- 1Faculty of Agrobiology, Food and Natural Resources, Department of Agroecology and Crop Production, Czech University of Life Sciences Prague, Prague, Czechia
- 2Faculty of Biology, University of Bialystok, Białystok, Poland
- 3Department of Botany and Zoology, Faculty of Science, Masaryk University, Brno, Czechia
Insects in the pupal stage are vulnerable to various predators because the pupa is immobile. The pupas of parasitoid ichneumonid wasps (Ichneumonidae) associated with spider hosts have evolved two lines of defense against predators, namely a cocoon spun by the parasitoid larva and a web provided by the spider host. The web is derived from a normal or modified spider web built by the spider under manipulation by the penultimate instar of the parasitoid wasp. In laboratory experiments, we tested the efficacy of these two defensive lines using six potential predators with two different types of mouthparts coming from three foraging guilds. The presence of the cocoon significantly reduced predation. Scavengers with chewing mouthparts, e.g., cockroaches and crickets, attacked and consumed pupas within both sparse and strong cocoon walls. Scavengers with piercing mouthparts were able to attack pupas in cocoons with a sparse wall, but not with a strong wall. Collectors and true predators showed no interest in cocoons. The presence of a web increased pupa protection by up to 80% when the web was on the ground and by up to 95% when the web was in the air. Only scavengers with chewing mouthparts were able to reach and consume pupas sheltered by the web. We provide the first evidence of how the two lines of defense contribute to parasitoid defense during the pupal stage.
Introduction
The pupal stage of insects is relatively immobile, leaving the insect with no possibility to escape from predators; however, it is not defenseless. Indeed, insect pupas have evolved a wide range of passive antipredator strategies (Lindstedt et al., 2019), such as hiding in vegetation, cryptic coloration, camouflage, and mimicry (Wiklund and Sillén-Tullberg, 1985; Skelhorn et al., 2010; Gaitonde et al., 2018). Many pupas have also evolved secondary defense mechanisms, which may be chemical, physical, or behavioral (Lindstedt et al., 2019). The production of a cocoon by the larva before the pupal stage is a common form of mechanical protection in holometabolan insects. Such a cocoon can protect them from predators, parasitoids, and unsuitable climatic conditions (Craig, 1997). The pupas of some insects are capable of limited movements that may have a potentially deimatic (frightening) function against predators. For example, Cole (1959) noted a lower rate of parasitoid oviposition on butterfly hosts whose pupas are capable of intensive wriggling movements. Pupas of other insects also utilize protection by other organisms (Pierce et al., 2002; Hammer and Moran, 2019). Caterpillars of the moth Platyperpia virginalis (Boisduval) utilize spines of plants for pupation. The survival of pupas was higher in spiny plants than when spines were removed experimentally (Grof-Tisza et al., 2015). The most unique way in which pupas gain protection is by manipulating the behavior of host species (Mokkonen and Lindstedt, 2016). Pupas of lycaenid butterflies have evolved acoustic mechanisms to manipulate ants’ guarding behavior (Pierce et al., 2002; Barbero et al., 2009). In addition to a pair of stridulatory organs, Lemonias caliginea (Butler) pupas possess a pair of glands on the thorax that may produce a chemical attractive to ants (Ross, 1964). Behavioral manipulation of the host in order to obtain protection for the pupa is also typical for ichneumonid wasps (Ichneumonidae) from the Polysphincta group of genera (polysphinctines), which are ectoparasitoids and koinobionts exclusively associated with spiders as hosts (Eberhard and Gonzaga, 2019). Koinobiont parasitoids attack hosts that continue feeding and growing during parasitism. Their larvae live on the surface of a host, from which they suck the haemolymph, and upon which they gradually mature to the final instar. During the larval stage, the ichneumonid wasp is protected by an active spider host, which is not paralyzed and uses various antipredatory strategies. This unique protection provided by the live spider host is lost when the final instar larva kills and consumes the spider to reach the next stage of its ontogeny, the pupal stage. Shortly before pupation, the larva may induce an alteration in the spider’s web building behavior, resulting in the production of a “cocoon web,” a silk structure which serves as protection for the parasitoid pupa (Eberhard, 2000; Korenko and Pekár, 2011; Korenko et al., 2014, 2018, 2022; Gonzaga et al., 2015; Kloss et al., 2016; Takasuka et al., 2017; Eberhard and Gonzaga, 2019). The architecture of cocoon webs often resembles that of molting webs of non-parasitized spiders (Eberhard and Gonzaga, 2019). Web-building spiders actively protect themselves against enemies and adverse environmental conditions by seeking a safe place and/or by building their own shelter (the production of silk structures). For example, spiders often build their own shelter by combining silk and surrounding material (Cloudsley-Thompson, 1995). Shelter construction is an important antipredatory behavior not only for spiders (Manicom et al., 2008), but also for their koinobiont parasitoids. Some spiders use leaves as shelters, and the pupas of some polysphinctines remain concealed and protected in these structures (Gonzaga and Sobczak, 2007; Sobczak et al., 2014; Gonzaga et al., 2016).
The strategy of pupa protection in ichneumonid wasps associated with spiders is composed of two lines of defense, these combining the defenses of both the parasitoid and the spider host. The parasitoid larva produces a protective cocoon and the spider host produces a web. Both should serve as protection for the parasitoid during the pupal stage. A spider under manipulation by the penultimate and final instar larva provides a normal or modified web, which is used as mechanical protection against potential enemies. The parasitoid manipulates the web building behavior of the spider host, which often builds a unique silk structure. Web manipulation by the parasitoid larva has been reported in a number of spider species (e.g., Kloss et al., 2017; Korenko et al., 2018, 2022; Eberhard and Gonzaga, 2019; Gonzaga et al., 2022). All studies on the manipulation of spider web building behavior work with the hypothesis that the purpose of the modified spider web is to protect the parasitoid during the pupal stage against natural elements and predators. This, however, has been supported by only three studies so far. Matsumoto (2009) reported that Agelena limbata Thorell (Agelenidae) individuals, when parasitized by Brachyzapus nikkoensis (Uchida), produced a silk-specific structure that blocked entry to crawling predators, such as ants, and thus reduced the predation risk faced by the parasitoid pupas. Gonzaga et al. (2010) documented that the reinforced cocoon webs produced by the spider Trichonephila clavipes (Linnaeus) increased protection for Hymenoepimecis wasps by preventing the cocoon from being knocked to the substrate. Kloss et al. (2016) observed that web modifications by Cyclosa spiders reduced the frequency of web ruptures and increased the chance of parasitoid adult emergence. Rain events were considered as the major cause of web ruptures and subsequent parasitoid mortality. In nature, parasitoid pupas can be predated by a range of arthropod and vertebrate predators (mainly birds). However, very limited evidence concerning the predation of the pupal stages of wasps from the Polysphincta group is available. We assume that predators that attack spiders and their cocoons (Pekár and Raspotnig, 2022) are potential predators of parasitoid pupas.
The protective efficiency against predators of the cocoon produced by the parasitoid pupa in conjunction with silk web structures provided by the spider host has not previously been investigated. We hypothesized that each of these two lines of defense provides a certain level of protection to the parasitoid pupa. We used several potential predators with various foraging strategies to test the effectiveness of these two defense lines. Ants, crickets, cockroaches and true bugs (Heteroptera) were used as model predators possessing the two most common types of mouthparts (chewing and sucking).
Materials and methods
Parasitoids
We collected parasitized juvenile spiders in several localities in the Czech Republic, Slovakia, and Poland. We chose localities where the parasitism rate was high and where we were able to collect sufficient numbers of parasitized spiders (Table 1). We kept the parasitized spiders at 18–20°C under a natural photoperiod and fed them with wingless fruit flies Drosophila melanogaster Meigen. Spiders with a parasitoid were fed until the larva killed the spider. Several parasitoid individuals from each spider host species were reared to adulthood in order to identify the parasitoid used to species level. Wasps were identified using Fitton et al. (1988) and Zwakhals (2006). Others (N = 201) were reared only to the pupal stage to be used in experiments.
Predators
There are many potential predators of pupas, particularly among arthropods. As published evidence is limited (see above), we chose such model predators which (1) were common in the microhabitat of pupas occurrence, (2) were available in the required numbers, (3) represented various predatory strategies, and (4) possessed various types of mouthparts. Specifically, we used: (1) scavengers with chewing mouthparts: Shelfordella lateralis (Walker) cockroaches, and Gryllus assimilus (Fabricius) and Acheta domestica Linné crickets; (2) predators with sucking mouthparts: Platymeris biguttatus (Linnaeus) and Pyrrhocoris apterus (Linnaeus) bugs; and (3) collectors with chewing mouthparts: Lasius fuliginosus Latreille ants (Table 2). All predator individuals came from laboratory breeding cultures, except for L. fuliginosus and P. apterus, which were collected in nature. Predator size was measured as the distance between the anterior end of the head and the posterior end of the abdomen.
Treatments
We tested the efficiency of two defensive lines: (1) the one provided by the parasitoid, respectively by the cocoon, and (2) the one provided by spider host, respectively by its web. The cocoon was classified into two types on the basis of its morphology: (1) with a strong wall (produced by Acrodactyla carinator (Aubert), associated with orb web building spiders of the genus Tetragnatha, and by Zatypota albicoxa (Walker), associated with 3D tangle web building spiders of the genus Parasteatoda); and (2) with a soft wall (produced by Zatypota anomala (Holmgren) and Zatypota percontatoria (Muller), associated with 3D tangle web building spiders of the genera Dictyna and Theridion; Figure 1). As a control, we used bare pupas (Figure 1A), which were gently removed from the cocoon using fine scissors and tweezers (Figures 1A–E). For each treatment combination, 10–15 individuals of a given predator species were used (Table 2).
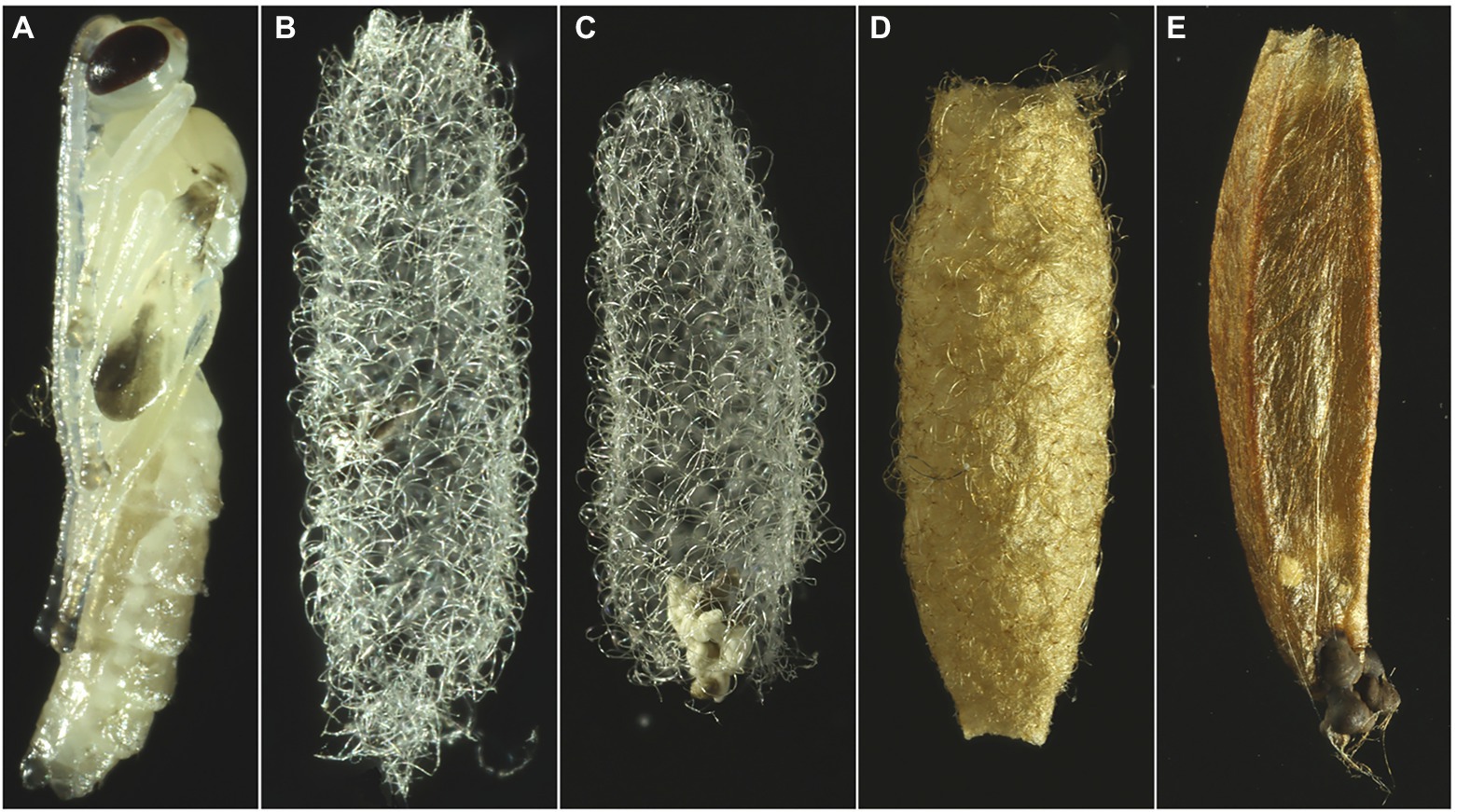
Figure 1. Tested bare pupa of Zatypota percontatoria (A) as control and the two tested cocoon types. Cocoons with a soft wall produced by Z. percontatoria (B) and Zatypota anomala (C), and cocoons with a strong wall produced by Zatypota albicoxa (D) and Acrodactyla carinator (E).
Further, we tested two traits of the spider web: (1) the web type (3D vs. none); and (2) the location of the web (in the air vs. on the ground). We simulated the situation in nature, where the pupas of parasitoids associated with 3D web building spiders are located at the centers of spider webs. Pupas of parasitoids associated with 3D web building spiders are protected by a 3D silk tangle provided by the spider host, which can originate only from the normal web, or can be modified by a manipulated spider host. Further, the position of the pupa above the ground appears to be another variable that contributes to its protection. We tested two hypothetical situations: (1) the pupa is protected by a 3D silk structure and located in an aerial web (Figure 2A); and (2) the pupa is protected only by a 3D silk structure and lies on the ground. In the first case, parasitized spiders were kept in an experimental arena to build cocoon webs. Once parasitoid larvas had formed a cocoon and pupated, the defensive function of the cocoon web was tested. In the latter case, we damaged one side of the 3D tangle web and thus the cocoon was touching the ground (Figure 2B). As a control, a cocoon located on the ground and unprotected by a 3D silk structure (i.e., the whole 3D web was removed) was used. Three-dimensional silk structures were provided by parasitized spiders producing 3D tangle webs – specifically, the spider hosts Dictyna sp. attacked by Z. anomala (unmodified 3D cocoon web), Parasteatoda lunata (Clerck) attacked by Z. albicoxa (unmodified 3D cocoon web), and Theridion varians Hahn attacked by Z. percontatoria (modified 3D cocoon web). Although the tested cocoon webs were represented by two different kinds of webs (modified and unmodified), their structures were very similar.
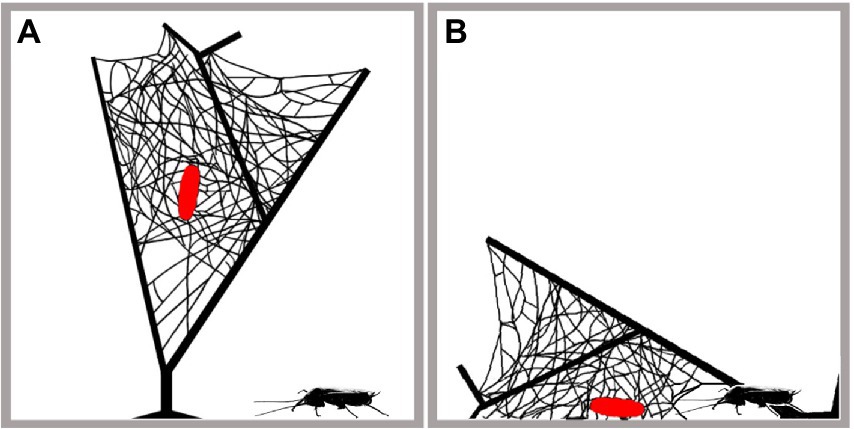
Figure 2. Situation in the experimental arena for testing the protection offered by web architecture against predators. The parasitoid cocoon with pupa (in red) was protected by a 3D silk structure. Cocoons were located either in an aerial web (A) or in a web that was placed on the ground (B).
Experimental design
To obtain pupas, parasitized spiders were reared individually in tubes (15 × 80 mm). A bare pupa, or pupa protected by a cocoon (of variable age), was placed on the ground in the center of an experimental area (100 × 100 mm base, 130 mm height), after which a predator was released into the arena. Pupa age was recorded as the number of days from the onset of the pupal stage, which was estimated to be 24 h after cocoon production.
To obtain a pupa protected by both a cocoon and a cocoon web, we kept spiders individually in experimental glass arenas (size as above) with an installed tree twig to provide support for a spider web. We moistened both tubes and arenas daily by spraying water.
After a potential predator was placed in the arena the predation success on the parasitoid pupa was recorded. Each predator was observed for 2 h continuously. If predation did not occur, then the predator was left in the arena for 24 h altogether, after which the trial was terminated. We recorded (1) the latency to an attack attempt, (2) the number of attacks, (3) capture success, and (4) feeding latency. Observations from experimental trials were recorded. Primary data used for statistical analyses are summarized in Supplementary Table S1.
Statistical analyses
The effects of six explanatory variables on the latency to attack, the latency to feed, and mortality were studied. Two explanatory variables were continuous (predator size, pupa age) and five categorical. Of these, two concerned the predator (predator species, mouthpart type) and two concerned the parasitoid species (cocoon type, protection type). We used generalized linear models (GLM), as the response variables came from different distributions (Pekár and Brabec, 2016). GLM with Gamma errors (GLM-g) were used to compare latencies, GLM with binomial errors (GLM-b) were used to compare mortalities. We tested the effect of host species in interaction with several explanatory variables, and, as it was not significant, we omitted it from the model. As the design was not fully orthogonal, we used the type II ANOVA table from the car package (Fox and Weisberg, 2019). The visreg package (Breheny and Burchett, 2017) was used to produce plots with estimated mean values and confidence intervals. Differences among factor levels were interpreted using confidence intervals.
Results
Parasitoid pupa as potential prey
We tested the acceptance of bare parasitoid pupa as prey by six potential predators. Of these, five predators caused the mortality of the bare pupa, but with significantly different probabilities (GLM-b, χ25 = 24.1, p = 0.0002): both Pyrrhocoris bugs and Lasius ants caused 100% mortality, followed by Acheta and Gryllus crickets, which each caused ~70% mortality, while Shelfordella cockroaches caused about 20% mortality and Platymeris bugs did not consume the pupa (Figure 3A). Pupa mortality was not affected by the age of the pupa (GLM-b, χ21 = 0.2, p = 0.65) but declined slightly, though significantly, with the increasing body size of potential predators (GLM-b, χ21 = 10.4, p = 0.0155, Figure 3B). Feeding latency was not affected by predator body size, predator species, or pupa age (GLM-g, F < 3.2, p > 0.17), and was, on average, 28 min and 57 s (SD = 33).
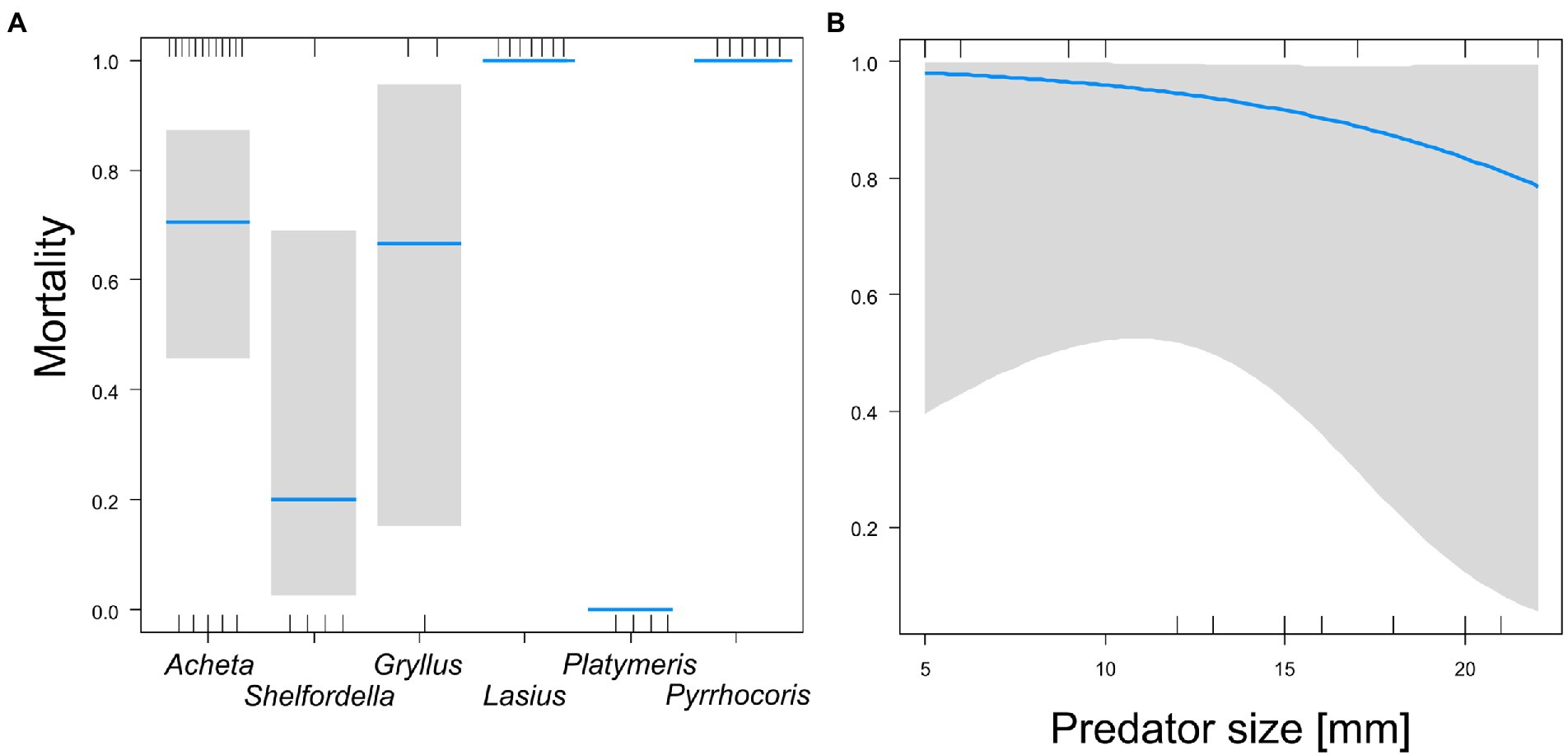
Figure 3. Comparison of bare pupa mortality caused by six predators (A); the effect of predator size on pupa mortality (B). Blue lines (A) are estimated means, gray bars are 95% confidence intervals of the mean. The blue line (B) is the estimated exponential model, the gray area is the 95% confidence band of the model. Marks on the horizontal axes represent surviving (upper axis) or dead individuals (lower axis).
Cocoon protection (the first defensive line)
We investigated the defensive role of the cocoon against six potential predators. Four predators captured and consumed pupas protected by the cocoon, but with significantly different probabilities (GLM-b, χ25 = 22.4, p = 0.0004): the highest mortality was caused by Gryllus crickets, followed by Acheta crickets, Shelfordella cockroaches, and Pyrrhocoris bugs (Figure 4A). Pyrrhocoris bugs attacked only pupas with a soft wall (29%, N = 7). Generally, there was a significant difference in pupa mortality when comparing bare and protected pupa (GLM-b, χ25 = 21.6, p = 0.0006): Lasius ants, Pyrrhocoris bugs, and Acheta crickets caused lower mortality among protected pupas than among bare pupas.
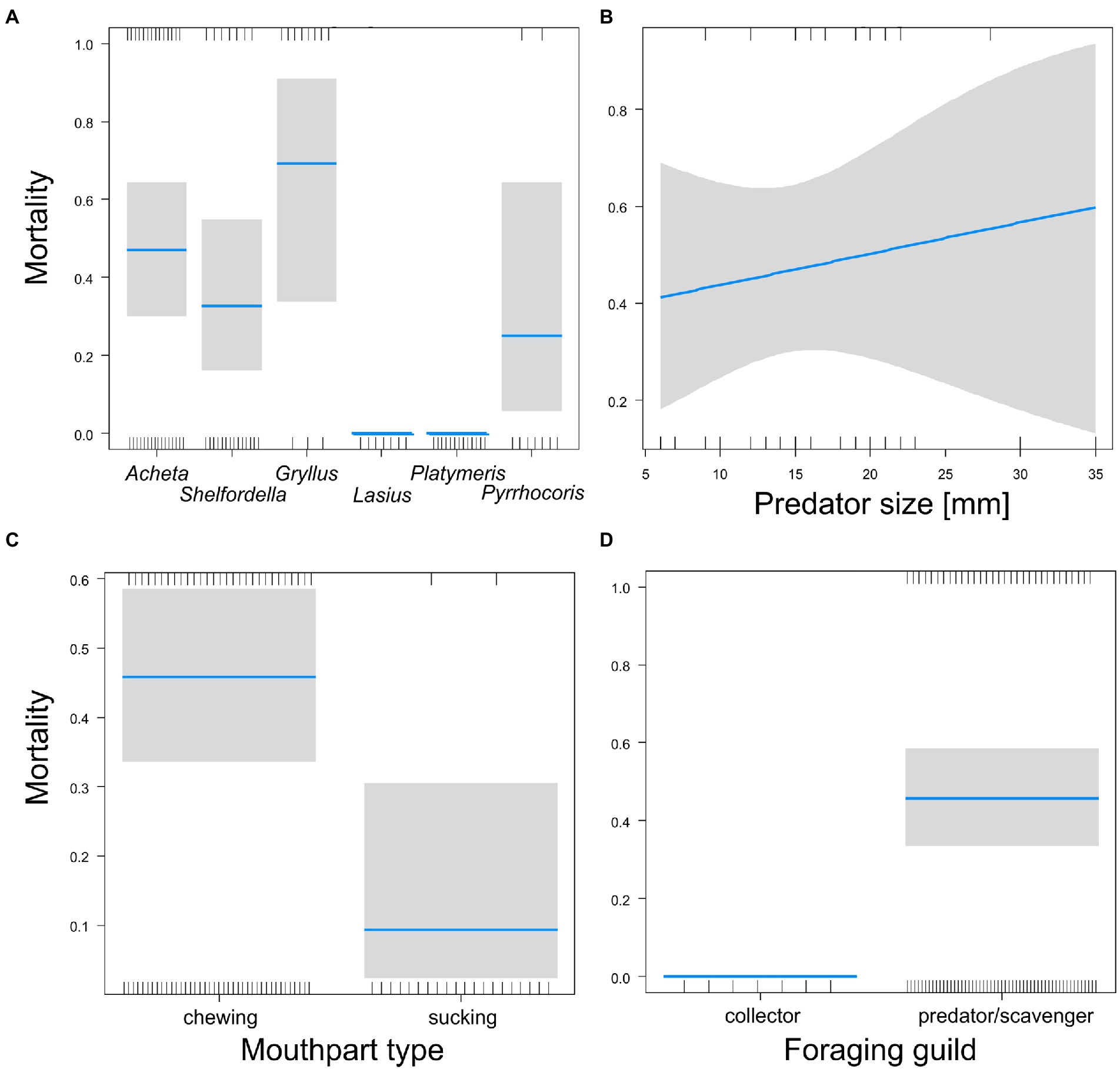
Figure 4. Comparison of the mortalities of pupas protected by cocoons caused by six predator species (A); the effect of predator size on mortality (B); comparison of the effects of two mouth parts (C) and two foraging guilds (D) of predators on pupa mortality. Blue lines (A,C,D) are estimated means, gray bars are 95% confidence intervals of the mean. Blue line (B) is the estimated sigmoid model, gray area is the 95% confidence band of the model. Marks on the horizontal axes represent surviving (upper axis) or dead individuals (lower axis).
The mortality of pupas protected by a cocoon was not affected by cocoon type (GLM-b, χ21 = 0.1, p = 0.72) or the interaction between cocoon type and mouthpart type (GLM-b, χ21 = 2.2, p = 0.14), but increased slightly, though significantly, with the increasing body size of predators (GLM-b, χ21 = 4.0, p = 0.045, Figure 4B). Mortality was significantly different for the two mouthpart types (GLM-b, χ21 = 8.6, p = 0.003, Figure 4C): predators with chewing mouthparts caused higher mortality than predators with sucking mouths. Scavengers caused significantly higher mortality than collectors (GLM-b, χ21 = 5.5, p = 0.019, Figure 4D). Feeding latency was not affected either by cocoon type, predator size, or predator species (GLM-g, F < 15, p > 0.16) and was, on average, 466 min 13 s (SD = 645). The latency to attack was not affected significantly by predator size, foraging guild, or mouthpart type (GLM-g, F < 47, p > 0.05), but was affected significantly by cocoon type (GLM-g, F1,51 = 76.1, p = 0.0155): pupas in cocoons with a soft wall were attacked with a short latency (Figure 5).
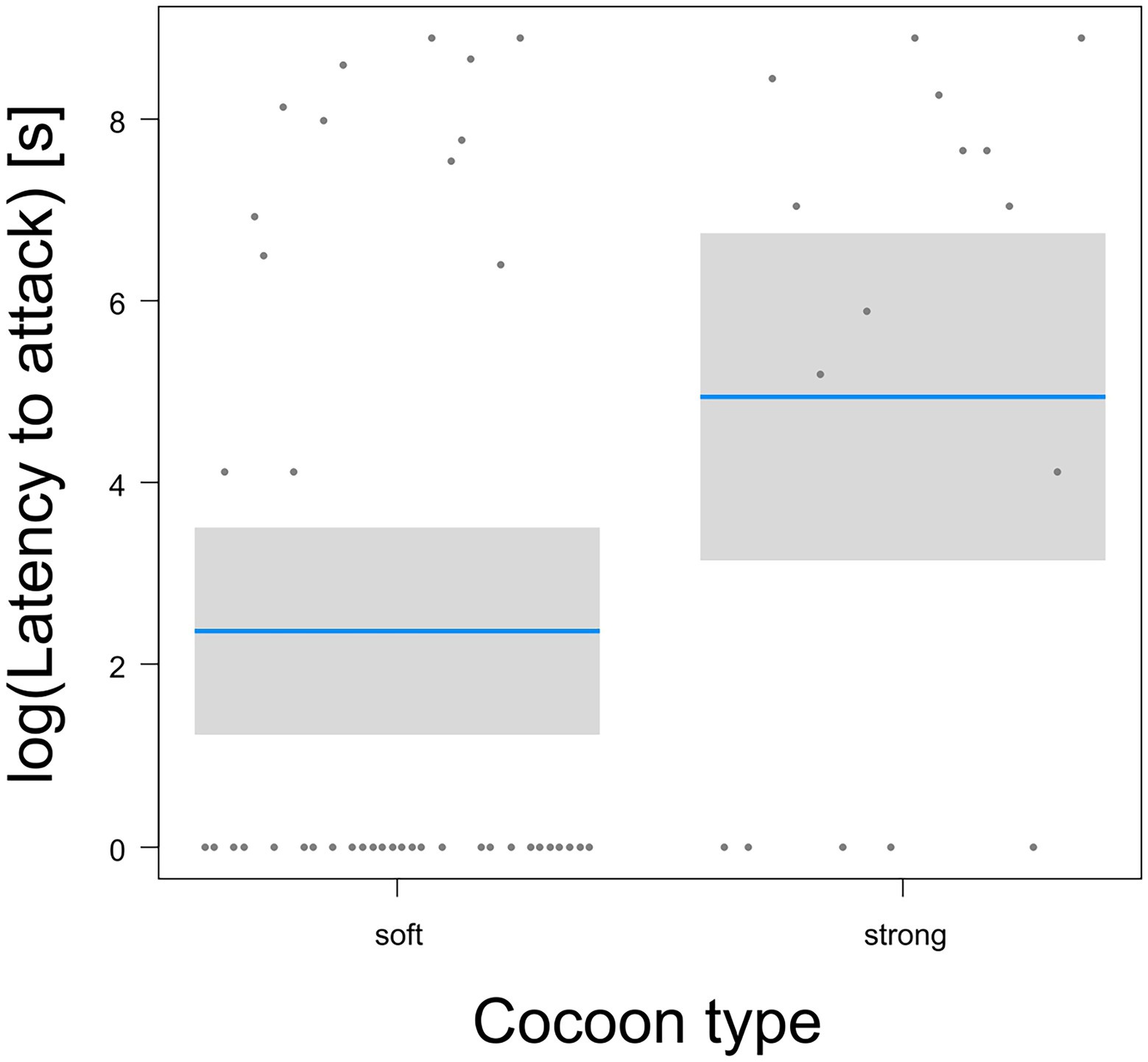
Figure 5. Comparison of latency to attack (on logarithmic scale) protected pupas between two cocoon types. Blue lines are estimated means, gray bars are 95% confidence intervals of the mean.
Web and cocoon location (the second defensive line)
We studied the defensive role of the web against six potential predators. The mortality of the pupa tended to differ between the locations of the web (GLM-b, χ21 = 3.5, p = 0.062): it was 5% for webs in the air compared to 20% for webs on the ground. Predators caused significantly higher mortality than collectors (GLM-b, χ21 = 9.8, p = 0.0018, Figure 6A). Predators with chewing mouthparts caused significantly higher mortality than suckers (GLM-b, χ21 = 7.2, p = 0.0073, Figure 6B).
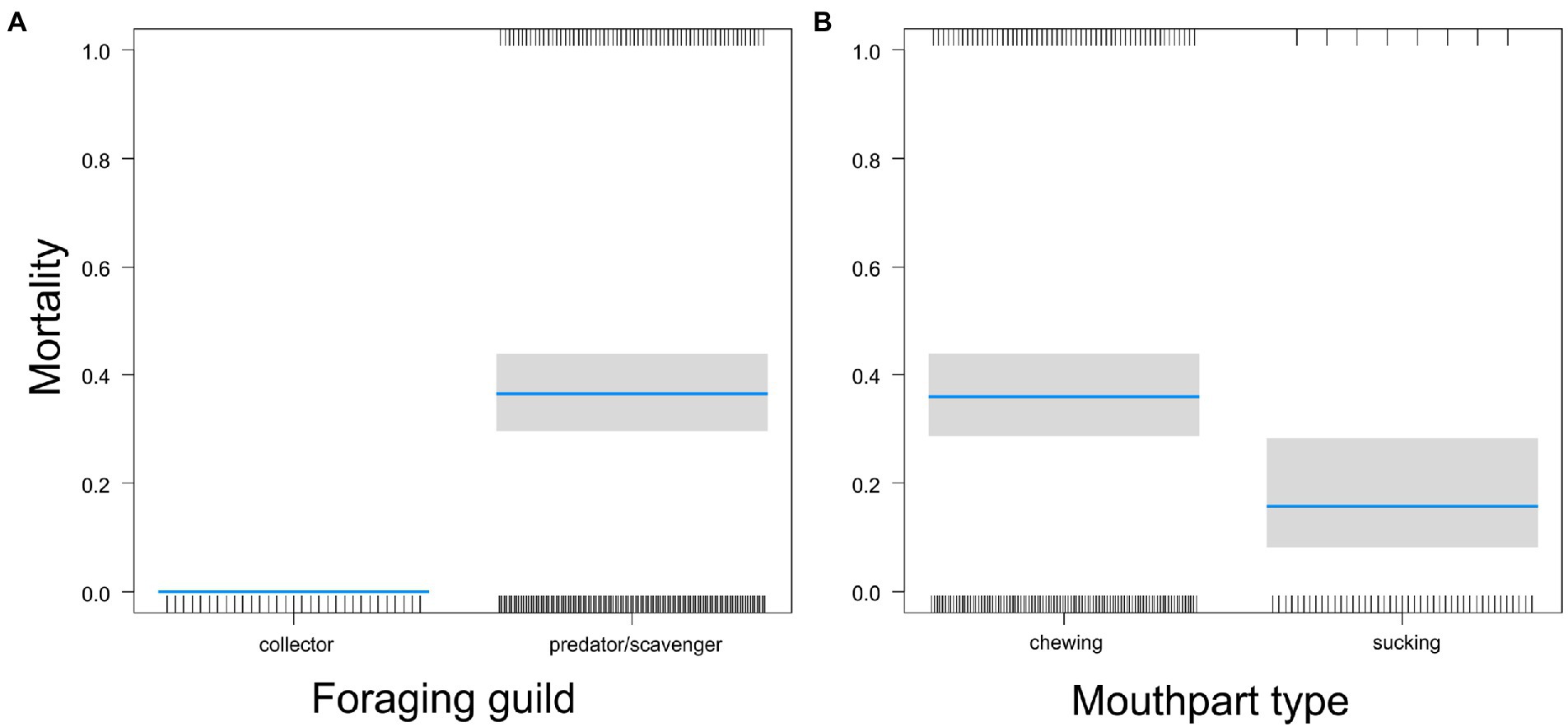
Figure 6. Comparison of the mortalities of pupas protected by webs between predators from different guilds (A) or possessing different mouthparts (B). Blue lines are estimated means, gray bars are 95% confidence intervals of the mean. Marks on the horizontal axes represent surviving (upper axis) or dead individuals (lower axis).
Discussion
We found that the pupas of ichneumonid wasps are accepted as food by various potential predators, including insects with both chewing and sucking mouthparts. Of the six potential predators tested, from various foraging guilds, surprisingly, only the true predator, the Platymeris bug, showed no interest in the bare pupa. This lack of interest can be explained by the fact that many true predators hunt actively moving prey (Schmitz, 2010). Strangely, the granivorous Pyrrhocoris bug was an effective predator of ichneumonid wasp pupas. They presumably used pupas as an alternative source of nourishment and accepted this food probably due to its lack of movement, which is similar to seeds (Kristenová et al., 2011). The fact that pupal age, predator type, and predator size did not significantly affect the feeding latency with respect to bare pupas or the mortality of bare pupas shows that pupal protection is important for parasitoid survival.
Pupal mortality decreased considerably when the pupa was protected by a cocoon. Lasius ants successfully attacked bare pupas by carrying them away in their mandibles. Yet, when the pupa was protected by a cocoon, the ants lost interest. Insects are equipped with various highly sensitive food detectors (Hallem et al., 2006). It seems that the loss of direct contact between the ant and the pupa due to the barrier created by the cocoon probably resulted in a failure to detect potential prey. Predators with chewing mouthparts, namely the Gryllus cricket and the Shelfordella cocroach, caused high mortality to pupas protected by a cocoon. Protection by a cocoon significantly decreased pupa mortality only when Acheta crickets were used. A strong cocoon wall protected pupas against predators with sucking mouthparts, but was not effective against predators with chewing mouths. Cocroaches and crickets consumed both pupas and their protective cocoons. The protection provided by the cocoon was not sufficient, though it decreased the success of predation considerably in the case of suckers. A strong cocoon wall prolonged the latency to attack and theoretically increased pupa survival, though not against predators with chewing mouth parts. For example, the Pyrrhocoris bug was not able to suck pupas protected by a cocoon with a strong cocoon wall, though it was able to suck pupas in cocoons with a soft wall. True bugs possess a stylet which can pierce the prey’s cuticule and suck hemolymph (Alford, 1999). Our study focused on the effect of cocoon wall type, but the limited number of experiments with a particular predator did not allow us to sufficiently test predator responses to the cocoons of two different species. This is a challenge for future study.
Mortality during the pupal stage caused by predators has also been documented in various groups of holometabolan insects (Battisti et al., 2000; Heisswolf et al., 2009; Kollberg et al., 2014). Pupas use different methods of protection against predators, and their effectiveness often varies according to the predator taxa (Lindstedt et al., 2019). Pupas may avoid a predator attack through cryptic coloration (Wiklund and Sillén-Tullberg, 1985), chemical camouflage (Mizuno et al., 2018; Wang et al., 2018), or by pupating in the ground to avoid predators foraging on the surface (East, 1974). Alternatively, pupas can be aposematic in color, sound, or odor to announce their unsuitability as prey (Gaitonde et al., 2018; Lindstedt et al., 2019). Apart from the cocoon built by the parasitoid before pupation, physical defense in some insect pupas is also provided by hairs or spines originating at the larval stage. These can also play an important role in pupal defensive structures (Murphy et al., 2010; Sugiura and Yamazaki, 2014).
Different types of pupal defenses are combined to provide safety for the pupal development of various insects (Lindstedt et al., 2019). Ichneumonid wasp pupas use simple physical cocoon protection, which is common in other insects, but they have also evolved a unique technique to increase pupa survival – specifically, the manipulation of spider web building behavior to produce the so-called ‘cocoon web’ (Eberhard and Gonzaga, 2019). The phenomenon of host manipulation in parasitoids associated with spiders is manifested by the alteration of web building behavior (Eberhard and Gonzaga, 2019). Shortly before killing the spider, the penultimate instar larva of the parasitoid changes the web-building behavior of the spider host to produce a special silk (Eberhard and Gonzaga, 2019). Many parasitoids that associate with spiders building a dense 3D tangle web do not induce modification of the normal web (Fritzén and Fjellberg, 2014; Korenko, 2017). We tested here wasp species that mostly do not modify the web architecture. We found that the 3D silk structrures provided for the parasitoid pupas were highly protective against the tested predators. When the cocoon with the pupa was also protected by a spider web, pupa mortality decreased for all predators. Only predators with strong chewing mouthparts, namely crickets, were able to chew through the spider silk and attack the cocoon with the pupa. When the cocoon with the pupa was located in the air, the mortality decreased to only 5%. The cricket, A. domestica, was presumably able to climb the installed twig where the 3D web was located, damage it, and reach the pupa of Z. albicoxa, probably due to its smaller body size (16 mm).We did not consider here hyperparasitoids as predators. The protection of the pupa by the two lines of defense does not appear to be effective against the highly specialized hyperparasitoids attacking spider parasitoids. There are 27 documented cases of hyperparasitoids from the families Chalcididae, Eulophidae and Ichneumonidae attacking the pupas of ichneumonid wasps associated with spiders (Pádua et al., 2022). However, future observations in the field are needed to obtain insights into the impacts of predators on populations of ichneumonidwasp parasitoids associated with spiders.
We confirmed under laboratory conditions that ichneumonid wasps associated with spiders are accepted as prey by various predators. We found that insects with different foraging strategies and with different types of mouthparts are potential predators; however, further work needs to be done in the field in order to identify the true range of natural predators. This is because such observations have so far been scattered and very rare, and because targeted data collection from nature requires systematic long-term research. Future research should focus on spider predators, especially egg predators, because the parasitoid pupa is immobile like an egg sac and, indeed, can also resemble an egg sac to an egg predator or scavenger. The pupal stage is a risky period in the development of polysphincta ichneumonid wasps, and survival during this period depends on successful pupation and on remaining in (or on) the spider web, where the pupa is well protected not only by the cocoon created by the parasitoid final instar larva, but also by the web architecture created by the spider host. We provide the first evidence of the roles of these two lines of defense in the survival of ichneumonid wasp pupas. We found support for the hypothesis that the spider web is an important component of parasitoid defense during the pupal stage. This hypothesis is cardinal in the study of the behavioral manipulation of spiders induced by larvas of parasitoid ichneumonid wasps.
In brief, we found the two lines of pupa defense described above to have a significant function under laboratory conditions. Although conditions in nature differ from the artificial environment of the laboratory, predators from the tested foraging guilds are common in nature and therefore represent a potential danger for pupas. Thus, the existence of these defensive lines likely confirms the need to protect against predators in the pupal stage.
Data availability statement
The original contributions presented in the study are included in the article/Supplementary material, further inquiries can be directed to the corresponding author.
Author contributions
SK, JS, and AK-A collected model organisms and performed experiments. SK designed the experiments and responsible for the integrity of this work. SK and SP analyzed the data and wrote the paper. All authors contributed to the article and approved the submitted version.
Funding
The study was financially supported by grant LTAUSA19084 of the Ministry of Education Youth and Sports of the Czech Republic. JS was supported by Czech University of Life Sciences Prague, Internal Faculty of Agrobiology, Food and Natural Resources, FAPPZ grant – 21150/1312/3152.
Conflict of interest
The authors declare that the research was conducted in the absence of any commercial or financial relationships that could be construed as a potential conflict of interest.
Publisher’s note
All claims expressed in this article are solely those of the authors and do not necessarily represent those of their affiliated organizations, or those of the publisher, the editors and the reviewers. Any product that may be evaluated in this article, or claim that may be made by its manufacturer, is not guaranteed or endorsed by the publisher.
Supplementary material
The Supplementary material for this article can be found online at: https://www.frontiersin.org/articles/10.3389/fevo.2022.1061700/full#supplementary-material
References
Barbero, F., Thomas, J. A., Bonelli, S., Balletto, E., and Schönrogge, K. (2009). Queen ants make distinctive sounds that are mimicked by a butterfly social parasite. Science 323, 782–785. doi: 10.1126/science.1163583
Battisti, A., Bernardi, M., and Ghiraldo, C. (2000). Predation by the hoopoe (Upupa epops) on pupae of Thaumetopoea pityocampa and the likely influence on other natural enemies. BioControl 45, 311–323. doi: 10.1023/A:1009992321465
Breheny, P., and Burchett, W. (2017). Visualization of regression models using visreg. R J. 9, 56–71. doi: 10.32614/RJ-2017-046
Cloudsley-Thompson, J. (1995). A review of the anti-predator devices of spiders. Bull. Br. Arachnol. Soc. 10, 81–96.
Cole, L. R. (1959). On the defences of Lepidopterous pupae in relation to the oviposition behaviour of certain Ichneumonidae. J. Lepid. Soc. 13, 1–10.
Craig, C. L. (1997). Evolution of arthropod silks. Annu. Rev. Entomol. 42, 231–267. doi: 10.1146/annurev.ento.42.1.231
East, R. (1974). Predation on the soil-dwelling stages of the winter moth at Wytham woods, Berkshire. J. Anim. Ecol. 43, 611–626.
Eberhard, W. G. (2000). Spider manipulation by a wasp larva. Nature 406, 255–256. doi: 10.1038/35018636
Eberhard, W. G., and Gonzaga, M. O. (2019). Evidence that Polysphincta-group wasps (hymenoptera: Ichneumonidae) use ecdysteroids to manipulate the web-construction behaviour of their spider hosts. Biol. J. Linn. Soc. 127, 429–471. doi: 10.1093/biolinnean/blz044
Fitton, M. G., Shaw, M. R., and Gauld, I. D. (1988). “Pimpline ichneumon-flies: Hymenoptera, Ichneumonidae (Pimplinae),” in Handbooks for the Identification British Insects. London: Royal Entomological Society. Vol. 7. 1–110.
Fox, J., and Weisberg, S. (2019). An R Companion to Applied Regression, 3rd. Thousand Oaks: SAGE Publications.
Fritzén, N. R., and Fjellberg, A. (2014). Natural history of Oxyrrhexis zephyrus sp.n. (Hymenoptera: Ichneumonidae), a parasitoid of Enoplognatha serratosignata (Araneae: Theridiidae), with notes on taxonomy and other host species of Oxyrrhexis. Arthropoda Selecta 23, 135144–135140. doi: 10.15298/arthsel.23.2.05
Gaitonde, N., Joshi, J., and Kunte, K. (2018). Evolution of ontogenic change in color defenses of swallowtail butterflies. Ecol. Evol. 8, 9751–9763. doi: 10.1002/ece3.4426
Gonzaga, M. O., Loffredo, A. P., Penteado-Dias, A. M., and Cardoso, J. C. F. (2016). Host behavior modification of Achaearanea tingo (Araneae: Theridiidae) induced by the parasitoid wasp Zatypota alborhombarta (hymenoptera: Ichneumonidae). Entomol. Sci. 19, 133–137. doi: 10.1111/ens.12178
Gonzaga, M. O., Moura, R. R., Pêgo, P. T., Bang, D. L., and Meira, F. A. (2015). Changes to web architecture of Leucauge volupis (Araneae: Tetragnathidae) induced by the parasitoid Hymenoepimecis jordanensis (hymenoptera: Ichneumonidae). Behaviour 152, 181–193.
Gonzaga, M. O., Pádua, D. G., and Quero, A. (2022). Inclusion of an alien species in the host range of the Neotropical parasitoid Hymenoepimecis bicolor (Brullé, 1846) (hymenoptera, Ichneumonidae). J. Hymenopt. Res. 89, 9–18. doi: 10.3897/jhr.89.76620
Gonzaga, M. O., and Sobczak, J. F. (2007). Parasitoid-induced mortality of Araneus omnicolor (Araneae, Araneidae) by Hymenoepimecis sp. (hymenoptera, Ichneumonidae) in southeastern Brazil. Naturwissenschaften 94, 223–227. doi: 10.1007/s00114-006-0177-z
Gonzaga, M. O., Sobczak, J. F., Penteado-Dias, A. M., and Eberhard, W. G. (2010). Modification of Nephila clavipes (Araneae Nephilidae) webs induced by the parasitoids Hymenoepimecis bicolor and H. robertsae (hymenoptera Ichneumonidae). Ethol. Ecol. Evol. 22, 151–165. doi: 10.1080/03949371003707836
Grof-Tisza, P., Holyoak, M., Antell, E., and Karban, R. (2015). Predation and associational refuge drive ontogenetic niche shifts in an arctiid caterpillar. Ecology 96, 80–89. doi: 10.1890/14-1092.1
Hallem, E. A., Dahanukar, A., and Carlson, J. R. (2006). Insect odor and taste receptors. Annu. Rev. Entomol. 51, 113–135. doi: 10.1146/annurev.ento.51.051705.113646
Hammer, T. J., and Moran, N. A. (2019). Links between metamorphosis and symbiosis in holometabolous insects. Philos. Trans. R. Soc. B 374:20190068. doi: 10.1098/rstb.2019.0068
Heisswolf, A., Klemola, N., Ammunét, T., and Klemola, T. (2009). Responses of generalist invertebrate predators to pupal densities of autumnal and winter moths under field conditions. Ecol. Entomol. 34, 709–717. doi: 10.1111/j.1365-2311.2009.01121.x
Kloss, T. G., Gonzaga, M. O., Oliveira, L. L., and Sperber, C. F. (2017). Proximate mechanism of behavioral manipulation of an orb-weaver spider host by a parasitoid wasp. PLoS One 12:e0171336. doi: 10.1371/journal.pone.0174146
Kloss, T. G., Gonzaga, M. O., Roxinol, J. A. M., and Sperber, C. F. (2016). Host behavioural manipulation of two orb-weaver spiders by parasitoid wasps. Anim. Behav. 111, 289–296. doi: 10.1016/j.anbehav.2015.11.001
Kollberg, I., Bylund, H., Huitu, O., and Björkman, C. (2014). Regulation of forest defoliating insects through small mammal predation: reconsidering the mechanisms. Oecologia 176, 975–983. doi: 10.1007/s00442-014-3080-x
Korenko, S. (2017). First record from Italy of Zatypota anomala (Ichneumonidae, Ephialtini), a parasitoid of the cribellate spider Dictyna pusilla (Araneae, Dictynidae). Arachnol. Mitt. 54, 1–4.
Korenko, S., Černecká, Ľ., Dorková, M., Sýkora, J., and Gajdoš, P. (2022). Sinarachna nigricornis and genus-specific host utilization of Araneus spiders by the genus Sinarachna (hymenoptera: Ichneumonidae). J. Arachnol. 50, 51–55. doi: 10.1636/JoA-S-21-012
Korenko, S., Isaia, M., Satrapová, J., and Pekár, S. (2014). Parazitoid genus-specific manipulation of orb-web host spiders (Araneae, Araneidae). Ecol. Entomol 39, 30–38. doi: 10.1111/een.12067
Korenko, S., and Pekár, S. (2011). A parasitoid wasp induces overwintering behaviour in its spider host. PLoS One 6:e24628. doi: 10.1371/journal.pone.0024628
Korenko, S., Pekár, S., Walter, G. H., Korenková, V., Hamouzová, K., Kolářová, M., et al. (2018). One generalist or several specialist species? Wide host range and diverse manipulations of the hosts’ web building behaviour in the true spider parasitoid Zatypota kauros (hymenoptera: Ichneumonidae). Insect Conserv. Divers. 11, 587–599. doi: 10.1111/icad.12307
Kristenová, M., Exnerová, A., and Štyds, P. (2011). Seed preferences of Pyrrhocoris apterus (Heteroptera: Pyrrhocoridae): are there specialized trophic populations? Eur. J. Entomol. 108, 581–586. doi: 10.14411/eje.2011.075
Lindstedt, C., Murphy, L., and Mappes, J. (2019). Antipredator strategies of pupas: how to avoid predation in an immobile life stage? Philos. Trans. R. Soc. Lond. B 374:20190069. doi: 10.1098/rstb.2019.0069
Manicom, C., Schwarzkopf, L., Alford, R. A., and Schoener, T. W. (2008). Self-made shelters protect spiders from predation. Proc. Natl. Acad. Sci. U. S. A. 105, 14903–14907. doi: 10.1073/pnas.0807107105
Matsumoto, R. (2009). “Veils” against predators: modified web structure of a host spider induced by an ichneumonid parasitiod, Brachyzapus nikkoensis (Uchida) (Hymenoptera). J. Insect Behav. 22, 39–48. doi: 10.1007/s10905-008-9152-1
Mizuno, T., Hagiwara, Y., and Akino, T. (2018). Chemical tactic of facultative myrmecophilous lycaenid pupa to suppress ant aggression. Chemoecology 28, 173–182. doi: 10.1007/s00049-018-0270-8
Mokkonen, M., and Lindstedt, C. (2016). The evolutionary ecology of deception. Biol. Rev. 91, 1020–1035. doi: 10.1111/brv.12208
Murphy, S. M., Leahy, S. M., Williams, L. S., and Lill, J. T. (2010). Stinging spines protect slug caterpillars (Limacodidae) from multiple generalist predators. Behav. Ecol. 21, 153–160. doi: 10.1093/beheco/arp166
Pádua, D. G., Kloss, T. G., Tavares, M. T., Santos, B. F., Araujo, R. O., Schoeninger, K., et al. (2022). Hyperparasitoids of polysphinctine Darwin wasps (Hymenoptera: Ichneumonidae) in South America. Austral Entomol. 61, 170–186. doi: 10.1111/aen.12593
Pekár, S., and Brabec, M. (2016). Modern Analysis of Biological Data. Generalised Linear Models in R. Brno: Masaryk University Press.
Pekár, S., and Raspotnig, G. (2022). Defences of arachnids: diversified arsenal used against range of enemies. Entomol. Gen. 42, 663–679. doi: 10.1127/entomologia/2022/1531
Pierce, N. E., Braby, M. F., Heath, A., Lohman, D. J., Mathew, J., Rand, D. B., et al. (2002). The ecology and evolution of ant association in the Lycaenidae (Lepidoptera). Annu. Rev. Entomol. 47, 733–771. doi: 10.1146/annurev.ento.47.091201.145257
Ross, G. N. (1964). Life history studies on Mexican butterflies. III. Early stages of Anatole rossi, a new myrmecophilous metalmark. J. Res. Lepid. 3, 81–94. doi: 10.5962/p.333475
Skelhorn, J., Rowland, H. M., Speed, M. P., and Ruxton, G. D. (2010). Masquerade: camouflage without crypsis. Science 327:51. doi: 10.1126/science.1181931
Sobczak, J. F., Moura-Sobczak, J. C. M. S., Messas, Y. F., Souza, H. S., and Vasconcellos-Neto, J. (2014). A new record of a host-parasitoid interaction: Hymenoepimecis veranii Lofredo & Penteado-Dias, 2009 (Hymenoptera: Ichneumonidae) parasitizing Araneus orgaos Levi, 1991 (Araneae: Araneidae). J. Insect Behav. 27, 753–758. doi: 10.1007/s10905-014-9467-z
Sugiura, S., and Yamazaki, K. (2014). Caterpillar hair as a physical barrier against invertebrate predators. Behav. Ecol. 25, 975–983. doi: 10.1093/beheco/aru080
Takasuka, K., Korenko, S., Štefánik, M., Černecká, Ľ., Mihál, I., Dolejš, P., et al. (2017). Host utilization of koinobiont spider-ectoparasitoids (Ichneumonidae, Ephialtini, Polysphincta genus-group) associated with Cyclosa spp. (Araneae, Araneidae) across Palaearctic. Zool. Anz. 267, 8–14. doi: 10.1016/j.jcz.2017.01.001
Wang, B., Lu, M., Cook, J. M., Yang, D.-R., Dunn, D. W., and Wang, R.-W. (2018). Chemical camouflage: a key process in shaping an ant–treehopper and fig–fig wasp mutualistic network. Sci. Rep. 8:1833. doi: 10.1038/s41598-018-20310-7
Wiklund, C., and Sillén-Tullberg, B. (1985). Why distasteful butterflies have aposematic larvae and adults, but cryptic pupas: evidence from predation experiments on the monarch and the European swallowtail. Evolution 39, 1155–1158. doi: 10.1111/j.1558-5646.1985.tb00456.x
Keywords: host parasitoid interaction, coevolution, spider host, Araneae, Polysphincta group of genera
Citation: Korenko S, Sýkora J, Kostro-Ambroziak A and Pekár S (2022) Two lines of defense in the pupas of ichneumonid wasp parasitoids associated with spider hosts. Front. Ecol. Evol. 10:1061700. doi: 10.3389/fevo.2022.1061700
Edited by:
Alfredo G. Nicieza, University of Oviedo, SpainReviewed by:
William Eberhard, University of Costa Rica, Costa RicaYael D. Lubin, Ben-Gurion University of the Negev, Israel
Marcelo Oliveira Gonzaga, Federal University of Uberlandia, Brazil
Copyright © 2022 Korenko, Sýkora, Kostro-Ambroziak and Pekár. This is an open-access article distributed under the terms of the Creative Commons Attribution License (CC BY). The use, distribution or reproduction in other forums is permitted, provided the original author(s) and the copyright owner(s) are credited and that the original publication in this journal is cited, in accordance with accepted academic practice. No use, distribution or reproduction is permitted which does not comply with these terms.
*Correspondence: Stanislav Korenko, a29yZW5rb0BhZi5jenUuY3o=