- 1Smithsonian Environmental Research Center, Smithsonian Institution, Edgewater, MD, United States
- 2Department of Biology, Bryn Mawr College, Bryn Mawr, PA, United States
Many photosynthetic plants supplement photosynthetic carbon with fungal carbon, but the mechanisms that govern dependence on mycoheterotrophic carbon are poorly understood. We used exclusion shelters to manipulate water and light availability to plants of the terrestrial orchids Goodyera pubescens and Tipularia discolor. We tracked changes in δ13C from photosynthesis and δ15N acquired from soil-derived inorganic nitrogen versus mycoheterotrophy, along with direct measures of photosynthesis in T. discolor. We hypothesized that shade would increase dependence on mycoheterotrophy compared to reference plants, while drought would decrease both photosynthesis and the abundance of potential mycorrhizal fungi. Drought and shade enriched 13C and 15N in both G. pubescens and T. discolor, compared to control plants, indicating increased fungal contribution to orchid tissues. Physiological measurements of T. discolor leaves showed that dark respiration, water use efficiency, and relative electron transport rate did not vary significantly, but shaded plants had greater quantum efficiency, suggesting they were light-limited. Light saturated photosynthesis of T. discolor leaves was lower in both shaded and drought-treated plants, indicating lower photosynthetic capacity, and likely greater dependence on mycoheterotrophy and corresponding enrichment in 13C and 15N. This study documented changes in orchid dependence on fungal carbon in response to manipulated environmental conditions. Both shade and drought increased the dependence of both orchids on mycoheterotrophically derived carbon and nitrogen.
Introduction
Between 85 and 92% of land plants obtain nutrients and water from the soil through mycorrhizal associations (Wang and Qiu, 2006; Brundrett and Tedersoo, 2018). For most plants, this association is a two-way exchange, with the plant providing carbon to the fungus in exchange for other resources. Some non-photosynthetic plants, termed fully mycoheterotrophic, obtain carbon from their mycorrhizal fungi (Gebauer and Meyer, 2003; Lallemand et al., 2019), while other mycoheterotrophic plants only initially rely on fungal carbon until they produce green leaves (Leake and Cameron, 2010; Tĕšitel et al., 2018). However, recently many green photosynthetic plants have been shown to supplement photosynthetic carbon with fungal carbon, and are termed partially mycoheterotrophic (Gebauer and Meyer, 2003) or mixotrophic (e.g., Selosse and Roy, 2008; Hynson et al., 2009; Merckx et al., 2010; Selosse and Martos, 2014). The mechanisms that govern the extent to which plants depend on mycoheterotrophically derived carbon are poorly understood but may be important for understanding the evolution of mycorrhizal associations, especially mycoheterotrophy (Selosse and Roy, 2008; Leake and Cameron, 2010; Wang et al., 2021).
All orchids are mycoheterotrophic at the protocorm life history stage and depend entirely on fungi for carbon and other resources that are required for transition to later life history stages. During the later life history stages, the amount of carbon they derive mycoheterotrophically varies (e.g., Leake, 1994; Rasmussen and Rasmussen, 2007). Most orchids photosynthesize at maturity and are not obligate mycoheterotrophs. However, nearly all orchids continue to associate with mycorrhizal fungi, and largely autotrophic orchids can be partially mycoheterotrophic (e.g., Gebauer and Meyer, 2003; Liebel et al., 2010; Yagame et al., 2012; Selosse and Martos, 2014; Hynson, 2016; Schiebold et al., 2018; Schweiger et al., 2018). Studies of albino and variegated variants of green orchids have also been used to demonstrate the importance of resource movement from mycorrhizal fungi to orchids (Selosse et al., 2004; Lallemand et al., 2019; Suetsugu et al., 2019) and to demonstrate a linear relationship between leaf chlorophyll and fungal contributions to plant carbon (Stöckel et al., 2011). Until recently, orchids were solely assumed to be the beneficiaries of a non-mutualistic association, obtaining carbon from fungi but not providing anything in return (Alexander and Hadley, 1985; Smith and Read, 1997). However, recent studies have shown that species within the genus Goodyera provide carbon (C) to mycorrhizal fungi under specific laboratory conditions (Cameron et al., 2006, 2008; Hynson et al., 2009). While the circumstances that dictate the direction of C flow are unclear, it has been speculated that stressors that reduce a plant’s photosynthetic ability (e.g., limited light and moisture) may prevent autotrophic carbon acquisition to the extent that orchids will increase the level of resources gained through mycoheterotrophy (Gebauer, 2005; McCormick et al., 2006; Hynson et al., 2009; Preiss et al., 2010). Indeed, Preiss et al. (2010) and Schweiger et al. (2019) demonstrated a correlation between the light quantity and the proportion of carbon that orchids derived from fungi.
Almost all herbaceous species in forests are highly or obligately dependent on mycorrhizal fungi (Brundrett and Kendrick, 1988; Whigham, 2004), and the loss of plant-fungal interactions has negative consequences for physiological processes, including nutrient and water uptake (Hale et al., 2011). Plant-fungal interactions can be disrupted, and the quantity and direction of benefits altered. McCormick et al. (2006), for example, found that individuals of Goodyera pubescens lost their mycorrhizal fungi during a drought. Surviving plants subsequently associated with the same or different mycorrhizal fungi, but also suffered higher mortality. The presence of mycorrhizal fungi is also important in non-orchids. Bitterlich et al. (2019) found that mycorrhizal fungi supported increased photosynthesis in tomatoes, but only when there was sufficient light and moisture. In contrast, Zhu et al. (2011) and Cabral et al. (2016) found that mycorrhizal plants maintained higher photosynthetic rates and yield in response to heat stress. These findings suggest that stress responses by a wide range of plants are affected by fungal interactions, but there have been few instances where that hypothesis has been directly tested.
Stable isotope natural abundance analysis is a useful approach to the study of mycoheterotrophic nutrient pathways (e.g., Gebauer and Meyer, 2003; Trudell et al., 2003; Ogura-Tsujita et al., 2009). Heterotrophically derived nutrients reflect the isotopic composition of their source and, because fungal C and sometimes nitrogen (N) are typically enriched in heavy isotopes relative to photosynthetically fixed carbon and soil-derived inorganic nitrogen, C and N isotopes can be used to estimate the degree of mycoheterotrophy (e.g., Gebauer and Meyer, 2003; Zimmer et al., 2007; Suetsugu et al., 2019). The carbon and nitrogen isotopic distinctiveness of nutrient contributions from saprotrophic fungi is far less than for fungi that simultaneously form ectomycorrhizal associations. Hydrogen isotopes are now being used to overcome the limited power of C and N isotopes to quantify mycoheterotrophy and have demonstrated greater fungal contribution to plant nutrition than previously suspected (Gebauer et al., 2016), but these methods are still not widely applied, and they were unavailable when the reported study was conducted. If the amount of mycoheterotrophy changes with environmental conditions or stress, then plant isotopic enrichment would be expected to reflect that change. Such changes in the relative contribution of fungi to plant nutrition and isotopic composition could result from increasing fungal contribution, decreasing photosynthetic contribution, or both (Jacquemyn et al., 2021). Additionally, isotopic composition can shift with direct effects of environmental conditions on stomatal conductance and photosynthesis.
We tested the hypothesis that photosynthetic orchids increase reliance on mycorrhizal fungi for C and N acquisition during periods of resource limitation. We used C and N isotope analysis to determine whether two orchids with different life history characteristics, G. pubescens and Tipularia discolor, relied more on mycorrhizal fungi for N and C under conditions of light limitation and decreased water availability. Light availability has been previously shown to influence photosynthesis in Tipularia discolor (Tissue et al., 1995; Hughes et al., 2019) and carbon acquisition in a species of Goodyera (Liebel et al., 2015). Both direct effects of drought on plants and increased fungal contribution to plant carbon would be expected to increase plant δ13C, but only increased fungal contribution would be expected to increase enrichment in 15N. We hypothesized that increased shade would decrease photosynthesis without directly affecting fungi, resulting in orchid leaves enriched in 13C, reflecting increased fungal contribution to plant carbon. In contrast, we hypothesized that drought would affect photosynthesis through decreased stomatal conductance but would potentially decrease fungal contribution to plant carbon. We expected the two species to differ in mean isotopic enrichment, because they associated with different fungi and were active at different times of the year, but we expected isotopic enrichment to increase or decrease similarly in response to treatment conditions.
Materials and methods
Study species
Goodyera pubescens R.Br is an evergreen orchid occurring in mid and late successional forests throughout eastern United States. Individual plants have a basal rosette of leaves with new leaves produced in the spring. Flowering occurs in mid-summer and the inflorescence emerges from the center of the basal rosette. After flowering, rhizomes may branch, allowing limited asexual reproduction, but clones remain small and did not extend beyond the experimental treatments. Pelotons of mycorrhizal fungi are present year-round in older roots and colonize newly produced roots (Rasmussen and Whigham, 2002). Plants associate exclusively with a single clade of Tulasnella spp. (McCormick et al., 2004) that decompose organic matter as their primary form of nutrition, and can switch fungi following drought (McCormick et al., 2006).
Tipularia discolor (Pursh) Nutt. is a winter-green orchid that produces a single leaf that appears in early autumn, typically September-October, and senesces in the spring, typically May. Flowering occurs at the end of July or beginning of August when leaves are not present. The species occurs primarily in hardwood forests throughout Eastern US. Fungal pelotons are present in roots throughout the year, with two fungi in the genus Protomerulius that support seed germination and protocorm growth. After becoming photosynthetic, the species associates with a wide range of fungi that belong to several distantly related Tulasnella clades (McCormick et al., 2004).
Study location
The experiments were conducted in six deciduous forest stands, three for each species of orchid, at the Smithsonian Environmental Research Center (SERC) in Edgewater, Maryland, USA. For both orchids, in each site we located 12 mature plants. For G. pubescens, the plants had rosettes that were ≥4 cm diameter and ≥five leaves. Tipularia discolor plants, which produce a single leaf per year, had leaves that were ≥3 cm wide. The orchids that were selected in each forest stand were separated by 1–2 meters to prevent sampling multiple plants associated with a single fungal organism. McCormick et al. (2006) found that orchids separated by more than 50 cm associated with different fungal individuals. We randomly assigned four individuals of each species to the shade and drought treatments (described below) and controls. We also selected 12 Fagus grandifolia Ehrh. seedlings (10–20 cm tall) associated with each selected orchid to serve as autotrophic reference plants. Fagus grandifolia was the only autotrophic species present across all study sites and <20 cm away from each selected orchid.
Experimental set-ups
Individual orchids subjected to drought or shade treatments were covered by exclosure shelters, each constructed of 1.9 cm diameter PVC pipe to form a 50 cm × 50 cm canopy with 28 cm legs. Shade structures used black 95% shade cloth as per Gorchov et al. (2011). Drought shelters were covered with UV-permeable rain barrier plastic (2-mil ACLAR 22A, Honeywell Specialty Films, Linden, NJ, USA), and were bordered on their uphill edge by a 50 cm length of landscape edging to divert surface runoff. Shelters for G. pubescens remained in place from June-October 2009 (5 months) and shelters over T. discolor remained in place from October 2009–February 2011 (16 months). Leaves of both species were collected for isotope analysis, described below, at the end of the study. In addition to the two orchids and reference F. grandifolia seedlings, we also collected leaves for isotope analysis from two seedlings of F. grandifolia that were growing beneath two of the T. discolor exclosures, one beneath a shade and the other beneath a drought treatment. While just two F. grandifolia seedlings make for a very small sample size, we had hoped to have far more F. grandifolia individuals, as well as other species, but few other plants grow in the shaded understory locations where the study took place and no other plants survived the treatments.
Environmental data
Soil moisture data associated with each shelter were collected at the beginning and end of each experiment to assess relative differences among sites, and to verify treatment efficacy. Percent soil moisture between 0 and 12 cm depth was measured at eight locations in each site to account for site differences in water availability (Hydrosense™ Moisture Meter, Campbell Scientific Australia Pty. Ltd., Garbutt, QLD, Australia). Photosynthetically active radiation (PAR) was measured using an AccuPAR PAR-80 1 m Sunfleck ceptometer (Decagon Devices, Pullman, WA, USA) at 50 cm above the ground, a height that reflected light levels that plants would have been experiencing prior to manipulation, at each plant location, and at random locations within each forest stand. PAR was highly variable (range 1–46% available PAR) among locations, sites, and beneath the light shelters and was never a significant factor in plant C, N, or isotopic enrichment (all P > 0.6), so it was not included in final analyses. Soil moisture values were compared among treatments (fixed independent variable) and sites (random independent variable) using an ANOVA. Experimental treatment and site effects were analyzed using two-way ANOVAs in Systat v 12.0 with site and treatment as main effects and interactions. Where treatment effects were significant, we conducted a post-hoc comparison among the treatment means using Tukey’s honestly significant difference test in Systat (12.0).
Plant growth and ecophysiology
For G. pubescens, initial plant size was measured by taking a photograph of each plant with a ruler for scale. Photos were printed, using the ruler to check for scale, and each leaf was cut out and area measured using a LI-3100 area meter (LiCor, Lincoln, NE, USA). At the end of the experiment, plants were again photographed, and area measured as before. Growth of each plant was calculated as the change in area from the beginning of the experiment until the end.
For T. discolor, we measured the length and width of each leaf (each plant produced a single leaf per year) using a ruler and converted to area using: Leaf Area = 2/3 (length × width). Growth of each leaf was based on area at the beginning and end of the experiment. For this species, we took advantage of the availability of a LiCor instrument that was not available when we conducted the study with G. pubescens. We measured the effects of drought and shade on T. discolor light saturated rates of photosynthesis (Asat) using a LiCor 6400 (LiCor Biosciences, Lincoln, NE, USA) on a warm (air temperature was 19.5°C) winter day, February 18, 2011. Hughes et al. (2019) found that February was when T. discolor had the highest rates of photosynthesis, reflecting a combination of increased light in the forest understory and physiological activity. Asat was measured with the instrument set to the following conditions: block temperature: 25°C, PAR: 1,000 micromols, as these represent optimum light and temperature conditions for this species (Tissue et al., 1995). After a minimum of 5 min under light saturated condition, we began to log data.
We then changed PAR to 0 to estimate dark respiration (Rd) and waited a minimum of 3 minutes until steady state conditions were achieved prior to logging data. Water use efficiency (WUE) under light saturated conditions was calculated as Asat/transpiration. We also performed rapid light curves using pulse amplitude modulated fluorometry (Mini PAM, Walz, Hamburg, Germany) to estimate quantum efficiency (α) and the maximum relative electron transport rate (rETR). Briefly, leaves were exposed to eight increasing levels of PAR for 10 seconds, followed by a 0.6 saturation pulse of light. Both α and rETR were fit in non-linear models in SAS (v 9.2) (proc nlin) as described by Ralph and Gademann (2005).
Experimental treatment and site effects on Asat, Rd, α, and rETR were analyzed using two-way ANOVAs in Systat v 12.0 with site and treatment as main effects and interactions. Where treatment effects were significant, we conducted a post-hoc comparison among the treatment means using Tukey’s honestly significant difference test in Systat (12.0).
Stable isotope abundances and nitrogen and carbon concentrations
Goodyera pubescens leaves were collected after 17 weeks of treatment and analyzed for relative isotopic abundance. We collected the youngest full-sized leaf from the center of each rosette. This ensured that we were collecting a leaf that had formed after the treatment was initiated, hence minimizing dilution of treatment effects through averaging over the lifespan of leaves that were already present when treatments began (e.g., Hynson et al., 2012). At the same time, we harvested the youngest full-sized leaves from the nearby F. grandifolia seedlings. After each leaf harvest, scissors used to cut each leaf were cleaned with 95% ethanol to prevent cross-contamination. Leaves were placed directly into sterile micro-centrifuge tubes, returned to the laboratory within 3 h, and stored at −20°C until they were prepared for isotope mass spectrometry (below).
Fully expanded leaves of T. discolor were collected in February 2011, 16 months after the experiment began. This ensured we were collecting leaves that initiated after treatment onset. The youngest full-sized leaves of the nearby F. grandifolia near the T. discolor shelters were collected earlier, October 2010. Fagus grandifolia leaves were collected at a different time because by the time T. discolor leaves were fully developed, the reference plants would not have had leaves. Leaves of the two F. grandifolia seedlings growing beneath T. discolor shelters (described above) were also sampled in October 2010.
Frozen leaf samples from the orchids and F. grandifolia were lyophilized, ground, and weighed (3–4 mg of ground foliar tissue) into 5 × 9 mm tin capsules (Costech Analytical Technologies, Valencia, CA, USA). Stable isotope ratio gas chromatography mass spectrometry (EA-IRMS) analyses were completed at the Smithsonian Museum Conservation Institute Stable Isotope/Mass Spectrometry Lab in Suitland, MD, using an Elemental Analyzer Model 4010 (Costech Analytical Technologies) coupled to a Delta V Advantage Isotopic Ratio Mass Spectrometer with Isodat NT Software (Thermo Fisher Scientific, Waltham, MA, USA).
Measured isotope abundances are presented as δ-values and calculated using the equation: δ15N or δ13C = (Rsample/Rstandard −1) × 1000 [‰] (where Rsample and Rstandard are ratios of heavy:light isotope of each element in the sample or standard) (Gebauer and Meyer, 2003). Because stable isotope composition is affected by local climatic conditions, relative isotope ratios were normalized to site-specific enrichment factors for each species using the equation: ε = δxS −δxR, where δxS is the individual δ15N or δ13C value of a sample, and δxR is the mean δ15N or δ13C of the 12 autotrophic reference plants at the site in question (Preiss and Gebauer, 2008). Total %N and %C were measured on all leaf samples with the same instrument used for the isotope analysis.
Percent N and C data were analyzed for correlations with isotopic composition, and to determine whether they varied with sample date or experimental treatment. We compared the isotopic enrichment of drought, shade, and control plants using ANOVAs with ε15N and ε13C as dependent variables. Treatment (control, shade, drought) and species were fixed independent variables, and site (nested within species) was a random variable. After considering the effect of treatment overall, we compared drought and shade treatments in a second set of identical ANOVAs. All calculations were conducted using Systat 12 for Windows (Systat Software Inc., San Jose, CA, USA).
Results
Environmental data
Soil moisture varied among sites and treatments for both species (Table 1). The treatment effect was significant (F = 61.3, P < 0.001). Soil moisture differed among the species, which was expected, since the studies were carried out in different years and seasons, and was significantly lower inside the precipitation exclosures for both species, compared to shade or control locations (ANOVA: Species: F = 37.5, P < 0.001; Treatment: F = 61.3, P < 0.001; Species × Treatment: F = 2.05, P = 0.15, Species (Site): F = 15.6, P < 0.001; Supplementary Figure 1). Shaded and control soils did not differ significantly (F = 0.700, P = 0.41); Species × Treatment: F = 2.05, P = 0.15, Species (Site): F = 15.6, P < 0.001.
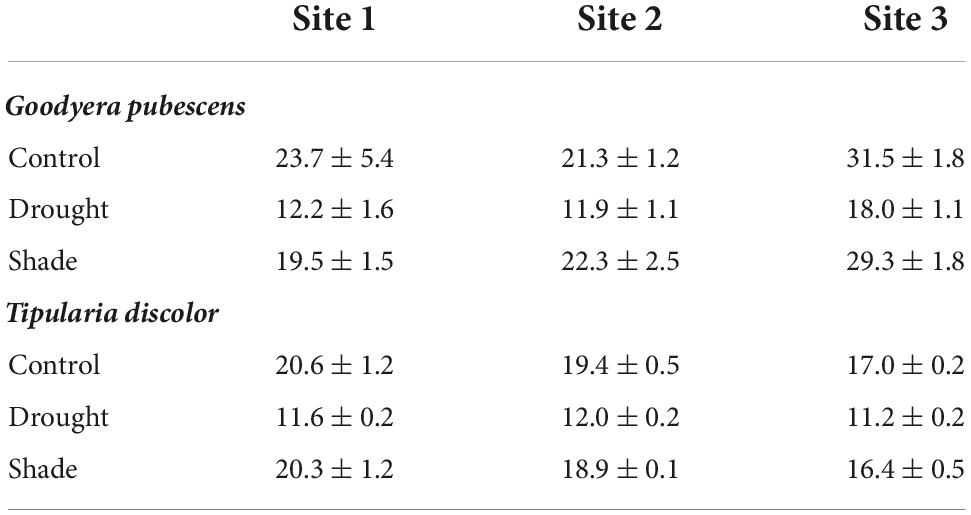
Table 1. Percent soil moisture (volumetric ± se) at each of the three sites and three treatments (control, drought, and shade) for Goodyera pubescens and Tipularia discolor.
Orchid growth
Relative leaf growth was significantly different between species (Figures 1A,B; ANOVA: Species: F = 13.5, P = 0.001) but the treatment and interaction effects were not significant (Treatment: F = 0.038, P = 0.96; Species × Treatment: F = 0.022, P = 0.98, Species (Site): F = 3.41, P = 0.014). Relative leaf growth was positive in all of the controls and the majority of treatments but there were noticeable differences in relative growth between sites within the two treatment sites (Figure 1A). Relative leaf growth was not different from zero, based on the size of the error bars, in one of the drought treatment plots, and negative in two of the shaded plots (Figure 1A).
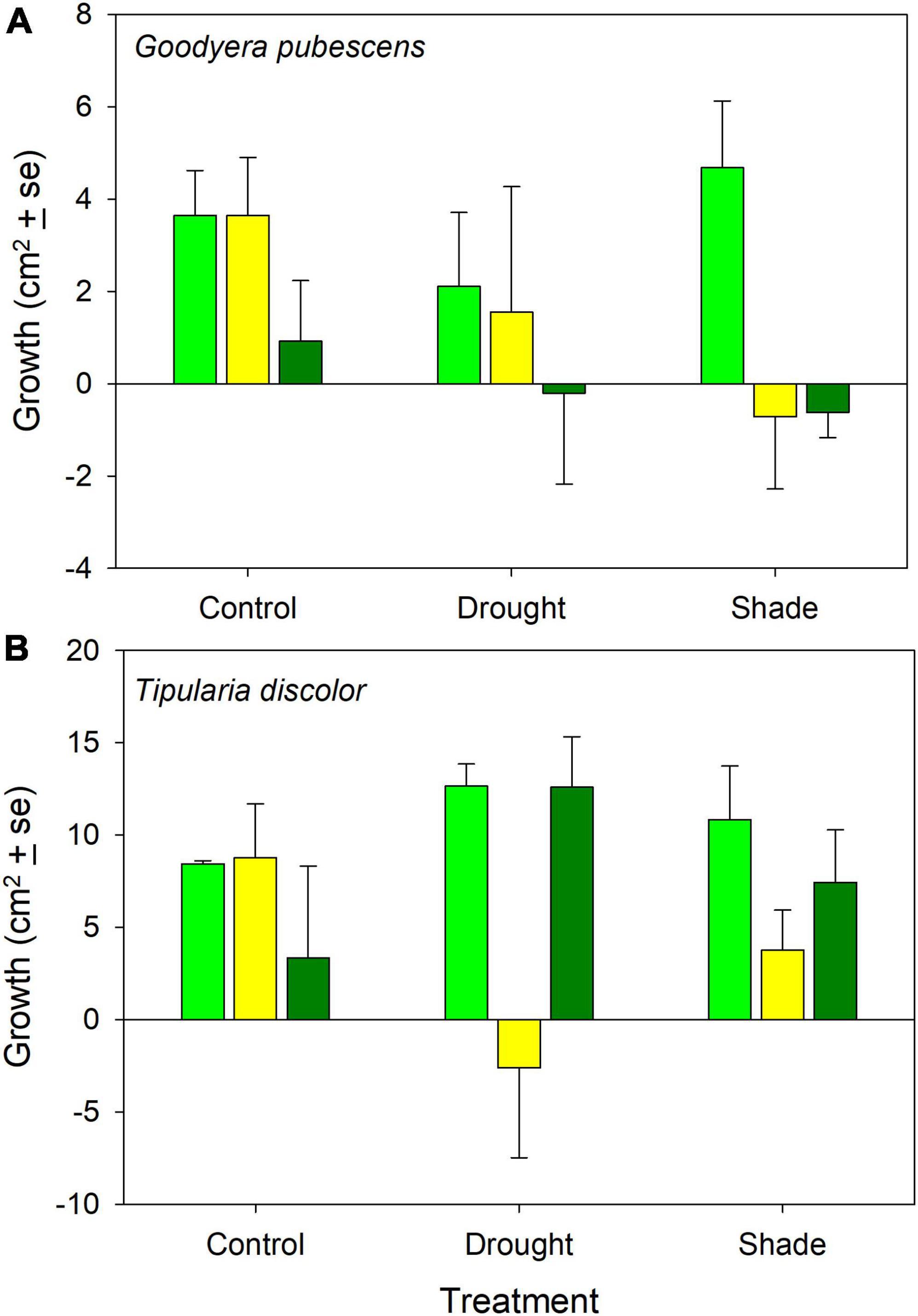
Figure 1. Relative leaf area growth (mean ± 1 SE) for (A) Goodyera pubescens and (B) Tipularia discolor control and treatment plots at each site. The three bars for the two treatments and controls represent different sites where the experiment was conducted, as described in the section “Materials and methods.”
Stable isotope abundances and nitrogen and carbon concentrations
There were no significant species, treatment, or sites effects for leaf C (Table 2; ANOVA: Species: F = 1.81, P = 0.18; Treatment: F = 1.57, P = 0.22), and the interactions between Species × Treatment (F = 0.808, P = 0.45) and Species (Site) (F = 0.890, P = 0.48) were not significant. Leaf N concentrations were significantly higher for T. discolor (Species: F = 16.0, P < 0.001) and drought-exposed plants had lower %N than control or shaded plants (F = 3.35, P = 0.042). The Species × Treatment (F = 0.011, P = 0.99) and Species (Site) (F = 1.61, P = 0.18) interactions were not significant for %N.
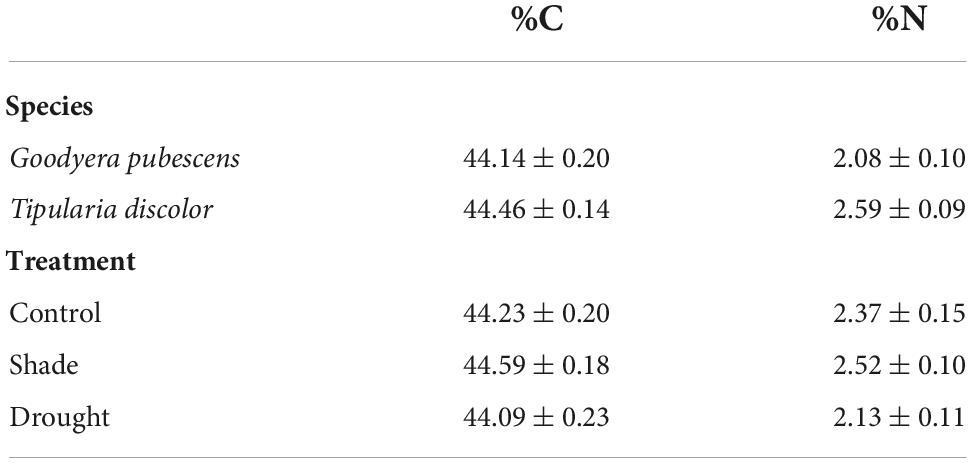
Table 2. Carbon (%C) and nitrogen (%N) concentrations (each mean ± se) of G. pubescens and T. discolor across all treatments (top two rows) and concentrations across treatments (bottom three rows).
Drought and shade treated plants of both orchids were enriched in both 13C and 15N compared to controls (Figure 2), but the species differences were only significant for ε13C (Table 3). For both species, there were between plot differences in ε13C and ε15N (Figure 3), but the difference between shade and drought treated plants was not significant (both P > 0.56). Leaves of both orchids differed from the control F. grandifolia leaves (Figure 2). As described in the Methods, we were able to sample a single F. grandifolia seeding in a shaded and drought T. discolor plot. The shaded seedling had ε13C = −0.51 and ε15N = 0.022, and the drought-exposed seedling had ε13C = 0.53 and ε15N = −0.06 (Figure 2).
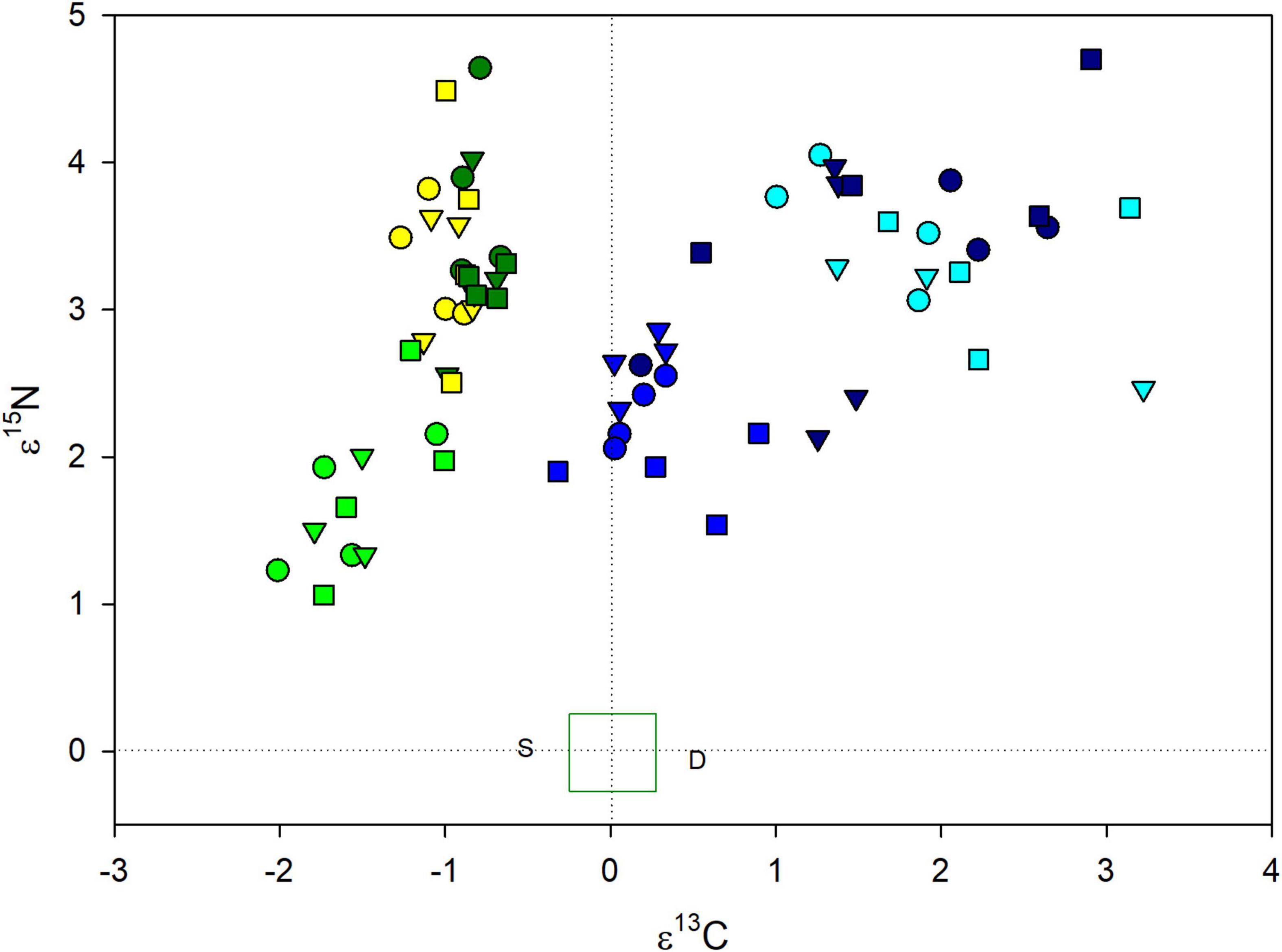
Figure 2. Isotopic enrichment factors for 13C and 15N orchids (G. pubescens: shades of green/yellow) and (T. discolor: shades of blue) exposed to control (medium green or blue), drought (yellow or light blue), and shade (dark green or dark blue) treatments. Within each species, values for orchids from the three sites are presented as different symbols, though overlapping symbol shapes for the two species do not indicate shared sites. The green box around 0,0 indicates the mean (defined as 0) and standard error of enrichment factors for autotrophic reference plants (Fagus grandifolia). The isotopic enrichment factors for the single surviving shaded and drought-treated F. grandifolia are indicated as S and D, respectively.
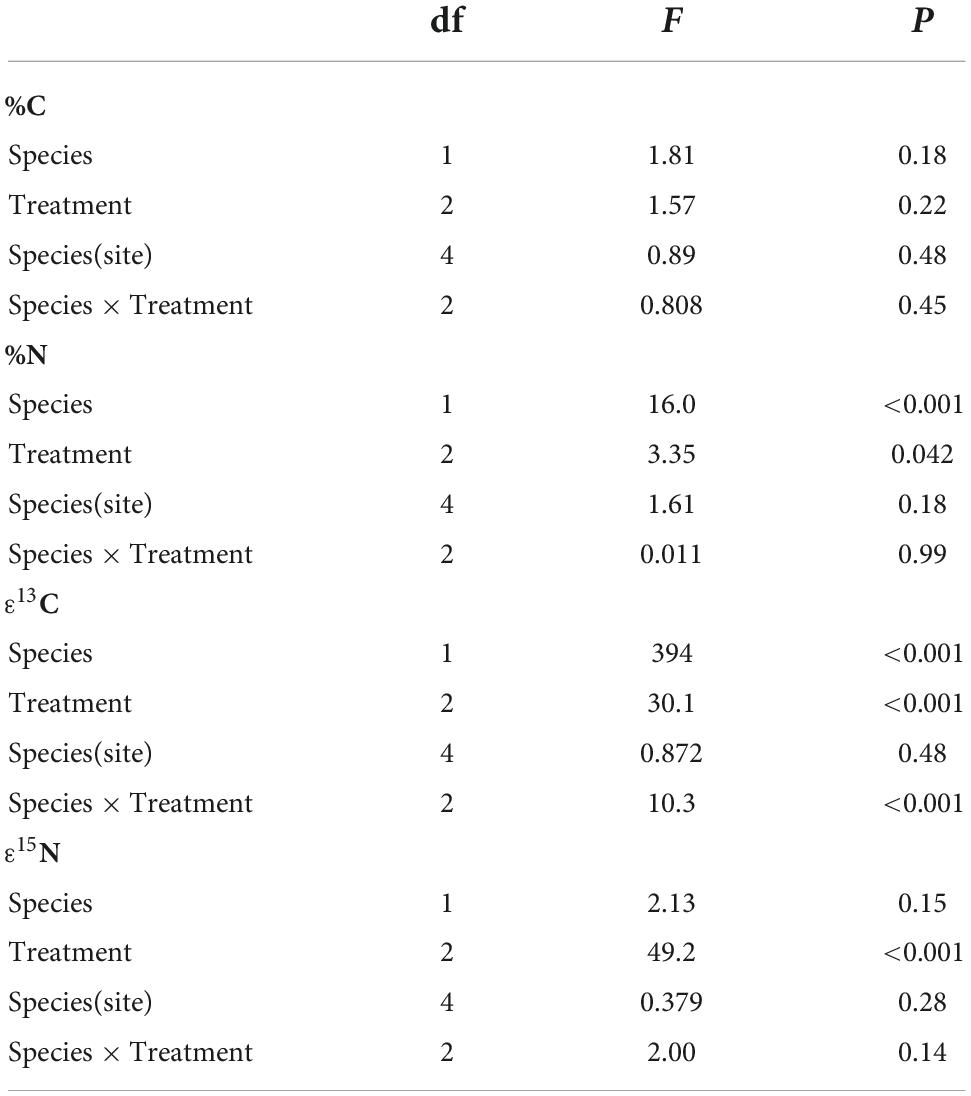
Table 3. The results of ANOVA tests for differences in carbon concentration (%C), nitrogen concentration (%N), and enrichment in 13C and 15N among orchid species, treatments (drought, shade, and control), sites within species, and species × treatment interactions.
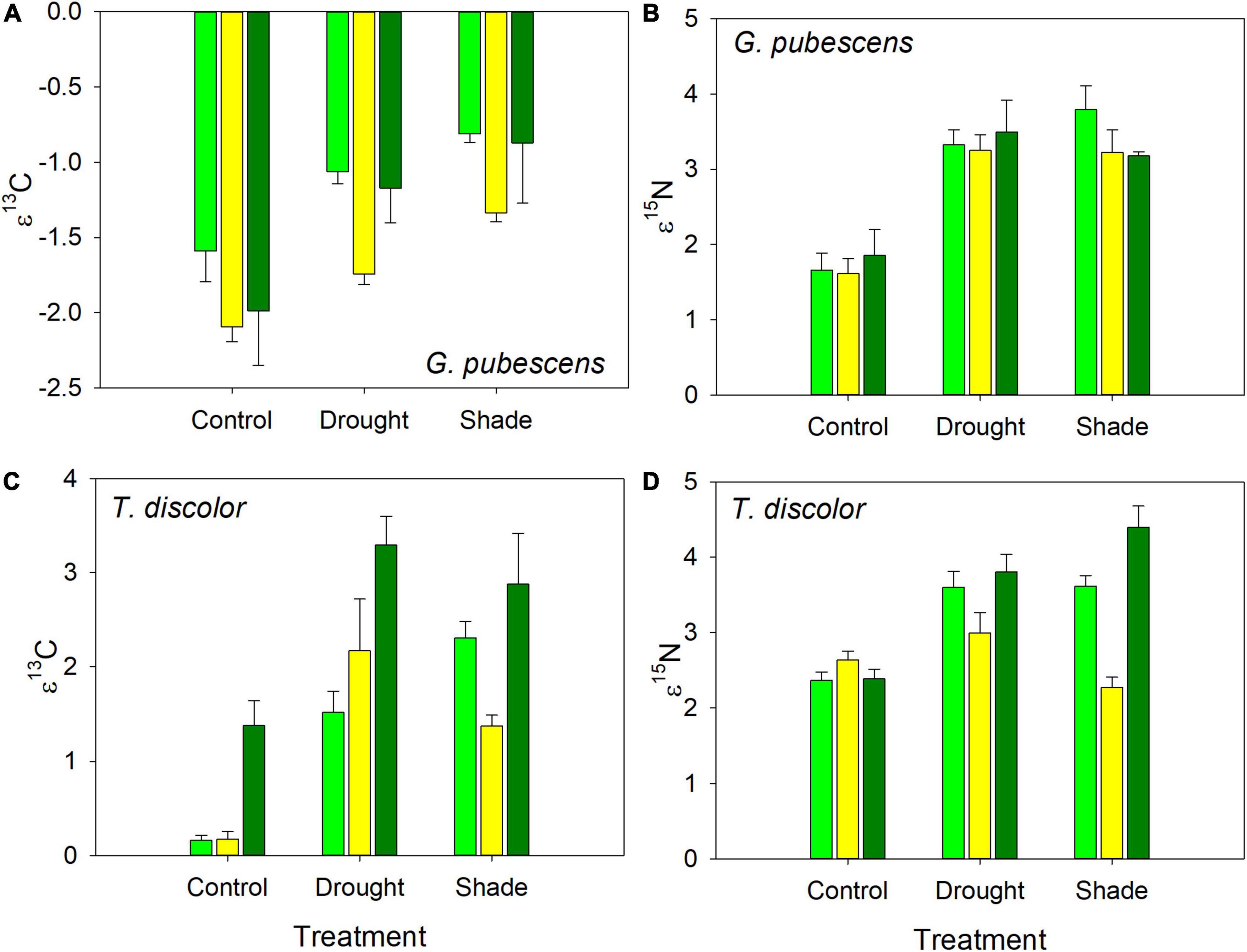
Figure 3. Isotopic enrichment factors for 13C and 15N for G. pubescens (A,B) and T. discolor (C,D) exposed to control, drought, and shade treatments. Mean enrichment factor ± se is shown. Different colored bars indicate different sites.
Ecophysiology
As expected, photosynthesis of T. discolor leaves differed between treatments, as demonstrated by significantly different quantum efficiency, α (F = 4.01, P = 0.04), and marginally significantly different rate of photosynthesis under light-saturating conditions, Asat (F = 3.48, P = 0.09). Dark respiration (Rd) was highly variable and not statistically different across sites or treatments (Table 4). Control plants had higher mean rates of light-saturated photosynthesis (Asat) than shaded or drought-treated plants (F = 8.93, P = 0.015), perhaps reflecting their lower stress level. Shaded plants had higher quantum efficiencies (α) than control and drought-treated plants (F = 7.07, P = 0.015), demonstrating the effects of light limitation. There were also significant differences among sites in rETR (F = 8.37, P = 0.002) and WUE (F = 4.25, P = 0.06) (Table 4).
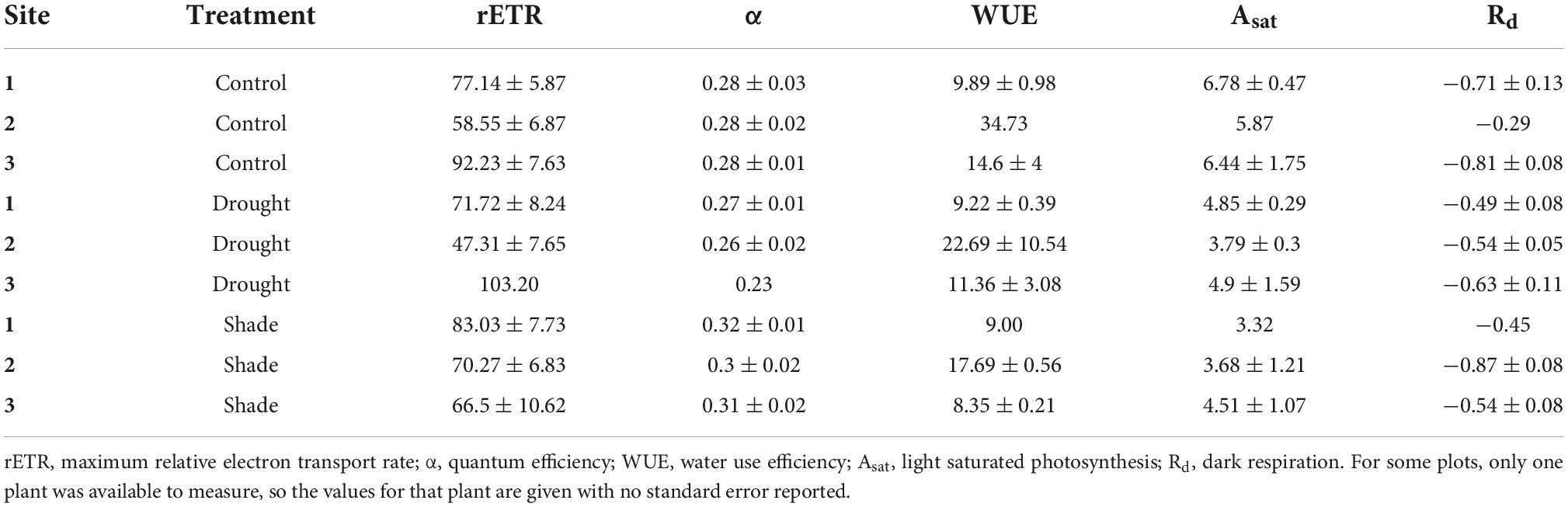
Table 4. Mean ecophysiological parameters (±se) for T. discolor in three sites under control conditions or exposed to drought or shade.
Discussion
This study offers experimental data supporting the hypothesis that light and drought stress can increase orchid dependence on fungal carbon. Selosse and Roy (2008) and Motomura et al. (2010) hypothesized that increasing mycoheterotrophy leads to the evolution of achlorophyllous, totally mycoheterotrophic plants, and that this might be triggered by very low light conditions. Others have shown a correlation between light availability and plant isotopic concentrations, indicative of fungal contribution to plant carbon (e.g., Gebauer, 2005; Liebel et al., 2010; Preiss et al., 2010), and a recent study used multiple isotopes to demonstrate a previously difficult to discern connection between light levels and partial mycoheterotrophy (Schweiger et al., 2019). In contrast, Tĕšitel et al. (2018) provided an argument for the retention of photosynthesis in partially mycoheterotrophic plants, as a support for seed set. None of these studies demonstrated the shifting of photosynthetic contributions with a manipulative experiment. We found that drought and shade stresses caused declines in parameters related to photosynthesis and resulted in increased reliance on mycoheterotrophy. We propose that the increased mycoheterotrophic contribution to orchid nutrition facilitated the maintenance of similar growth rates in both species and dark respiration in T. discolor despite the stress conditions.
The increased reliance on mycoheterotrophy was interpreted from increased enrichment in 13C and 15N, but isotopic composition can also be affected by other factors. Both drought and shade resulted in enrichment in 13C. However, both stressors can also have direct effects on 13C composition. In the absence of a changed fungal contribution to carbon, we would have expected that a direct effect of shade on plant carbon cycling would led to depleted 13C, as shown for a wide range of non-orchid autotrophs (Preiss et al., 2010; Liebel et al., 2015; Lallemand et al., 2018). This also appears to be borne out by the single shaded F. grandifolia seedling, which had a lower ε13C than the untreated autotrophic reference plants. Importantly, we found that the actual difference was in the opposite direction, demonstrating that the orchids were more enriched in 13C, not less. The results suggest that our measurements may have underestimated the true increases in mycoheterotrophy that occurred as a result of shading.
In contrast to the shade treatment, we hypothesized that drought-treated orchids would be unable to increase the fungal contribution to C. However, we measured enrichment in 13C in drought-treated orchids that was almost the same as for shade. Although we do not know the extent to which OMF are able to translocate water, a possible reason for not seeing the expected decrease in ε13C could be that the drought imposed by the shelters was too localized to affect the fungi, which might have been able to translocate water from outside the shelters. For example, Ruth et al. (2011) found that arbuscular mycorrhizal fungi were able to translocate water from one chamber to an associated plant in another chamber. Another possible explanation for the increase in ε13C could be the direct effects of drought. The δ13C of plants experiencing drought has been found to increase 1–2‰ as a result of stomatal closure to minimize water loss and increased internal recycling of CO2 (e.g., Pollastrini et al., 2010). However, the single F. grandifolia seedling that was exposed to drought was only slightly enriched in 13C compared to untreated autotrophic control plants, suggesting that while our isotopic measurements might have led to an overestimate of increases in mycoheterotrophy in response to drought, they do not seem to negate them.
Another possible cause for the observed enrichment of 13C is that drought could disrupt the orchid-fungus relationship and cause orchids to associate with different, perhaps more drought-resistant, fungi. McCormick et al. (2006) found that G. pubescens in locations near the present study sites switched to associate with different fungal genets following a drought. If such a switch happened in this experiment, the new fungi could have had different isotopic compositions than the original fungi, leading to shifts in orchid isotopic composition without corresponding changes in quantitative contributions to plant carbon. This is only a realistic possibility for T. discolor because it associates with diverse fungi that can include fungi that are ectomycorrhizal with surrounding trees, and so potentially isotopically very different (e.g., Gebauer and Meyer, 2003). However, in many T. discolor individuals sampled over the course of 10 years, we have not found any that were sufficiently enriched in 15N to suggest a considerable input from ectomycorrhizal fungi. In G. pubescens, fungal associates belong to a very narrow clade that we would expect to be isotopically very similar.
Patterns for 15N enrichment mirrored those for 13C, suggesting that both nitrogen and carbon were taken up from fungi, and that the uptake was affected by drought and shade stress. The strongest differences in 15N enrichment that reflect the extent of mycoheterotrophy have been seen in plants associating with ectomycorrhizal fungi, which are typically enriched in 15N relative to N obtained from inorganic nutrients in the soil (e.g., Hynson et al., 2013). Saprotrophic fungi often have less enriched 15N abundance, depending on what they decompose to obtain nutrients, and so the nitrogen “signal” for fungal contribution to plant nutrition is weaker (Bidartondo et al., 2004; Martos et al., 2009; Schweiger et al., 2019). Indeed, the observed increase in ε15N was far less than what has been observed for orchids associated with fungi that are simultaneously ectomycorrhizal with other plants, but it was still a detectable increase (e.g., Schiebold et al., 2018). This is particularly important for interpreting the direct effects of drought and shade on plant photosynthesis, and thus ε13C, because ε15N is expected to be unaffected by direct effects of drought (Peuke et al., 2006) and shade, yet we saw a similar increase in δ15N in drought-exposed and shaded plants. Accordingly, the two surviving F. grandifolia plants in the drought and shade treatments both had ε15N of nearly 0. However, drought-treated orchids also had lower overall nitrogen concentration, suggesting that less total nitrogen was taken up by the plants and that perhaps the fungal contribution to drought-treated plants, while a proportional increase compared to unstressed control orchids, nevertheless could have been less than for shaded plants.
The differences in photosynthetic parameters for T. discolor shed some light on how drought and shade affected plant physiology and complemented isotopic results. In particular, we found lower light saturated photosynthesis (Asat) in shade and drought stressed orchids; suggesting that photosynthetic rates were lower in stressed plants. Shaded plants were able to photosynthesize more efficiently (greater α), a common adaptation to low light levels, but this was likely not enough to make up for the greatly decreased light availability in this treatment. Other physiological parameters, WUE, and Rd, were unchanged, suggesting that T. discolor was able to maintain many aspects of their physiology, despite decreased photosynthetic ability. Both orchid species were also able to maintain similar growth rates despite shade and drought stress, perhaps pointing to the importance of increased mycoheterotrophy for surviving stressful conditions.
Our results suggest that increased mycoheterotrophy may be triggered by stresses that limit photosynthetic ability and not just by limited light. The effects of experimental treatments on plant ε13C and ε15N indicated that the orchids can increase reliance on carbon derived from fungi when photosynthetic capability was encumbered. Although it has previously been postulated that limited light availability could determine reliance on fungi for carbon (Gebauer, 2005; Hynson et al., 2009; Liebel et al., 2010; Preiss et al., 2010), this study provides experimental evidence that reduced light availability and drought can both increase mycorrhizal dependence in a partially mycoheterotrophic orchid. These results, combined with the evidence that photosynthesis contributes primarily to above-ground parts of partially mycoheterotrophic plants (Tĕšitel et al., 2018), provide the framework on which to build a more detailed understanding of the evolution of mycorrhizal associations. Further, with investigation of other green orchids and partially mycoheterotrophic plants, this promises to advance understanding of the dynamics of mycoheterotrophy in forest understories, and may help to explain why nearly all forest understory herbs are mycorrhizal (Whigham, 2004), despite very low light availability, which would be expected to limit the availability of photosynthetic carbon to contribute to a bidirectional mycorrhizal association.
Data availability statement
The raw data supporting the conclusions of this article will be made available by the authors, without undue reservation.
Author contributions
MM and KG conceived the bulk of the study with additions by DW and TM. KG (2009–2011), MM (2009–2022), and TM (2011–2022) carried out the study. MM analyzed the data. MM and KG wrote the manuscript. DW and TM edited the manuscript. All authors contributed to the article and approved the submitted version.
Funding
KG was funded by the Environmental Leadership Center at Warren Wilson College. MM was funded in part by the US National Park Service (PMIS 120082).
Acknowledgments
We thank Amy Boyd, Lou Weber, and Phil Jamison for their advice on study design and analysis and Jay O’Neill, Anne Chamberlain, Christine France, and Gary Peresta for their advice and technical support during the experiment. Isotopic samples were analyzed at the Smithsonian’s Museum Conservation Institute.
Conflict of interest
The authors declare that the research was conducted in the absence of any commercial or financial relationships that could be construed as a potential conflict of interest.
Publisher’s note
All claims expressed in this article are solely those of the authors and do not necessarily represent those of their affiliated organizations, or those of the publisher, the editors and the reviewers. Any product that may be evaluated in this article, or claim that may be made by its manufacturer, is not guaranteed or endorsed by the publisher.
Supplementary material
The Supplementary Material for this article can be found online at: https://www.frontiersin.org/articles/10.3389/fevo.2022.1047267/full#supplementary-material
References
Alexander, C., and Hadley, G. (1985). Carbon movement between host and mycorrhizal endophyte during the development of the orchid Goodyera repens. New Phytol. 101, 657–665. doi: 10.1111/j.1469-8137.1985.tb02871.x
Bidartondo, M., Burghardt, B., Gebauer, G., Bruns, T., and Read, D. (2004). Changing patterns in the dark, isotopic and molecular evidence of ectomycorrhizal liaisons between forest orchids and trees. Proc. Biol. Sci. 271, 1799–1806. doi: 10.1098/rspb.2004.2807
Bitterlich, M., Franken, P., and Graefe, J. (2019). Atmospheric drought and low light impede mycorrhizal effects on leaf photosynthesis–a glasshouse study on tomato under naturally fluctuating environmental conditions. Mycorrhiza 29, 13–28. doi: 10.1007/s00572-018-0872-6
Brundrett, M. C., and Kendrick, B. (1988). The mycorrhizal status, root anatomy, and phenology of plants in a sugar maple forest. Can. J. Bot. 66, 1153–1173. doi: 10.1139/b88-166
Brundrett, M. C., and Tedersoo, L. (2018). Evolutionary history of mycorrhizal symbioses and global host plant diversity. New Phytol. 220, 1108–1115. doi: 10.1111/nph.14976
Cabral, C., Ravnskov, S., Tringovska, I., and Wollenweber, B. (2016). Arbuscular mycorrhizal fungi modify nutrient allocation and composition in wheat (Triticum aestivum L.) subjected to heat-stress. Plant Soil 408, 385–399. doi: 10.1007/s11104-016-2942-x
Cameron, D. D., Johnson, I., Read, D. J., and Leake, J. R. (2008). Giving and receiving, measuring carbon cost of mycorrhizas in the green orchid, Goodyera repens. New Phytol. 180, 176–184. doi: 10.1111/j.1469-8137.2008.02533.x
Cameron, D. D., Leake, J. R., and Read, D. J. (2006). Mutualistic mycorrhiza in orchids, evidence from plant-fungus carbon and nitrogen transfers in the green-leaved terrestrial orchid Goodyera repens. New Phytol. 171, 405–416. doi: 10.1111/j.1469-8137.2006.01767.x
Gebauer, G. (2005). “Partnertausch im dunklen wald-stabile isotope geben neue einblicke in das ernährungsverhalten von orchideen,” in Rundgespräche der komission für ökologie, Vol. 30, ed. Bayerische Akademie der Wissenschaften (München: Verlag Dr. Friedrich Pfeil), 55–67.
Gebauer, G., and Meyer, M. (2003). 15N and 13C natural abundance of autotrophic and mycoheterotrophic orchids provides insight into nitrogen and carbon gain from fungal association. New Phytol. 160, 209–216. doi: 10.1046/j.1469-8137.2003.00872.x
Gebauer, G., Preiss, K., and Gebauer, A. C. (2016). Partial mycoheterotrophy is more widespread among orchids than previously assumed. New Phytol. 211, 11–15. doi: 10.1111/nph.13865
Gorchov, D. L., Thompson, E., O’Neill, J., Whigham, D., and Noe, D. A. (2011). Treefall gaps required for establishment, but not survival, of invasive Rubus phoenicolasius in deciduous forest, Maryland, USA. Plant Spec. Biol. 26, 221–234. doi: 10.1111/j.1442-1984.2011.00317.x
Hale, A. N., Tonsor, S. J., and Kalisz, S. (2011). Testing the mutualism disruption hypothesis, physiological mechanisms for invasion of intact perennial plant communities. Ecosphere 9:110. doi: 10.1890/ES11-00136.1
Hughes, N. M., Gigantino, G. M., Grace, M. H., Hoffman, K. M., Lila, M. A., Willans, B. N., et al. (2019). Photosynthetic profiles of green, purple, and spotted-leaf morphotypes of Tipularia discolor (Orchidaceae). Southeast. Nat. 18, 641–658. doi: 10.1656/058.018.0415
Hynson, N. A. (2016). The carbon and nitrogen ecophysiologies of two endemic tropical orchids mirrors those of their temperate relatives and the local environment. R. Soc. Open Sci. 3:160427. doi: 10.1098/rsos.160427
Hynson, N. A., Madsen, T. P., Selosse, M.-A., Adam, I. K. U., Ogura-Tsujita, Y., Roy, M., et al. (2013). “The physiological ecology of mycoheterotrophy,” in Mycoheterotrophy: The biology of plants living on fungi, ed. V. S. F. T. Merckx (New York, NY: Springer), 297–342. doi: 10.1007/978-1-4614-5209-6_8
Hynson, N. A., Mambelli, S., Amend, A., and Dawson, T. E. (2012). Measuring carbon gains from fungal networks in understory plants from the tribe Pyroleae (Ericaceae): A field manipulation and stable isotope approach. Oecologia 169, 307–317. doi: 10.1007/s00442-011-2198-3
Hynson, N. A., Preiss, K., and Gebauer, G. (2009). Is it better to give than receive? A stable isotope perspective to orchid-fungal carbon transport in the green orchid species Goodyera repens and G. oblongifolia. New Phytol. 182, 8–11. doi: 10.1111/j.1469-8137.2009.02778.x
Jacquemyn, H., Brys, R., Waud, M., Evans, A., Figura, T., and Selosse, M.-A. (2021). Mycorrhizal communities and isotopic signatures in two partially mycoheterotrophic orchids. Front. Plant Sci. 12:618140. doi: 10.3389/fpls.2021.618140
Lallemand, F., Figura, T., Damesin, C., Fresneau, C., Griveau, C., Fontaine, N., et al. (2019). Mixotrophic orchids do not use photosynthates for perennial underground organs. New Phytol. 221, 12–17. doi: 10.1111/nph.15443
Lallemand, F., Robionek, A., Courty, P.-E., and Selosse, M.-A. (2018). The 13C content of the orchid Epipactis palustris (L.) Crantz responds to light as in autotrophic plants. Bot. Lett. 165, 265–273. doi: 10.1080/23818107.2017.1418430
Leake, J. (1994). The biology of mycoheterotrophic (saprotrophic) plants. New Phytol. 127, 171–216. doi: 10.1111/j.1469-8137.1994.tb04272.x
Leake, J. R., and Cameron, D. D. (2010). Physiological ecology of mycoheterotrophy. New Phytol. 185, 601–605. doi: 10.1111/j.1469-8137.2009.03153.x
Liebel, H. T., Bidartondo, M. I., and Gebauer, G. (2015). Are carbon and nitrogen exchange between fungi and the orchid Goodyera repens affected by irradiance? Ann. Bot. London 115, 251–261. doi: 10.1093/aob/mcu240
Liebel, H. T., Bidartondo, M. I., Preiss, K., Segreto, R., Stöckel, M., Rodda, M., et al. (2010). C and N stable isotope signatures reveal constraints to nutritional modes in orchids from the Mediterranean and Macaronesia. Am. J. Bot. 97, 903–912. doi: 10.3732/ajb.0900354
Martos, F., Dulormne, M., Pailler, T., Bonfante, P., Faccio, A., Fournel, J., et al. (2009). Independent recruitment of saprotrophic fungi as mycorrhizal partners by tropical achlorophyllous orchids. New Phytol. 18, 668–681. doi: 10.1111/j.1469-8137.2009.02987.x
McCormick, M. K., Whigham, D. F., and O’Neill, J. (2004). Mycorrhizal diversity in photosynthetic terrestrial orchids. New Phytol. 163, 425–438. doi: 10.1111/j.1469-8137.2004.01114.x
McCormick, M. K., Whigham, D. F., Sloan, D., O’Malley, K., and Hodkinson, B. (2006). Orchid-fungus fidelity, a marriage meant to last? Ecology 87, 903–911. doi: 10.1890/0012-9658(2006)87[903:OFAMMT]2.0.CO;2
Merckx, V., Stöckel, M., Fleischmann, A., Bruns, T. D., and Gebauer, G. (2010). 15N and 13C natural abundance of two mycoheterotrophic and a putative partially mycoheterotrophic species associated with arbuscular mycorrhizal fungi. New Phytol. 188, 590–596. doi: 10.1111/j.1469-8137.2010.03365.x
Motomura, H., Selosse, M.-A., Martos, F., Kagawa, A., and Yukawa, T. (2010). Mycoheterotrophy evolved from mixotrophic ancestors, evidence in Cymbidium (Orchidaceae). Ann. Bot. 106, 573–581. doi: 10.1093/aob/mcq156
Ogura-Tsujita, Y., Gebauer, G., Hashimoto, T., Umata, H., and Yukawa, T. (2009). Evidence for novel and specialised mycorrhizal parasitism, the orchid Gastrodia confusa gains carbon from saprotrophic Mycena. Proc. Biol. Sci. 276, 761–767. doi: 10.1098/rspb.2008.1225
Peuke, A. D., Gessler, A., and Rennenberg, H. (2006). The effect of drought on C and N stable isotopes in different fractions of leaves, stems, and roots of sensitive and tolerant beech ecotypes. Plant Cell Environ. 29, 823–835. doi: 10.1111/j.1365-3040.2005.01452.x
Pollastrini, M., Desotgiu, R., Cascio, C., Bussotti, F., Cherubini, P., Saurer, M., et al. (2010). Growth and physiological responses to ozone and mild drought stress of tree species with different ecological requirements. Trees Struct. Funct. 24, 695–704. doi: 10.1007/s00468-010-0439-4
Preiss, K., Adam, I. K. U., and Gebauer, G. (2010). Irradiance governs exploitation of fungi, fine-tuning of carbon gain by two partially myco-heterotrophic orchids. Proc. Biol. Sci. 277, 1333–1336. doi: 10.1098/rspb.2009.1966
Preiss, K., and Gebauer, G. (2008). A methodologial approach to improve estimates of nutrient gains by partially myco-heterotrophic plants. Isotopes Environ. Health. Stud. 44, 393–401. doi: 10.1080/10256010802507458
Ralph, P. J., and Gademann, R. (2005). Rapid light curves, a powerful tool to assess photosynthetic activity. Aquat. Bot. 82, 222–237. doi: 10.1016/j.aquabot.2005.02.006
Rasmussen, H. N., and Rasmussen, F. N. (2007). Trophic relationships in orchid mycorrhiza – diversity and implications for conservation. Lankesteriana 7, 334–341. doi: 10.15517/lank.v7i1-2.19560
Rasmussen, H. N., and Whigham, D. F. (2002). Phenology of roots and mycorrhiza in orchid species differing in phototrophic strategy. New Phytol. 154, 797–807. doi: 10.1046/j.1469-8137.2002.00422.x
Ruth, B., Khalvati, M., and Schmidhalter, U. (2011). Quantification of mycorrhizal water uptake via high-resolution on-line water content sensors. Plant Soil 342, 459–468. doi: 10.1007/s11104-010-0709-3
Schiebold, J. M. I., Bidartondo, M., Lenhard, F., Makiola, A., and Gebauer, G. (2018). Exploiting mycorrhizas in broad daylight, partial mycoheterotrophy is a common nutritional strategy in meadow orchids. J. Ecol. 106, 168–178. doi: 10.1111/1365-2745.12831
Schweiger, J. M.-I., Bidartondo, M. I., and Gebauer, G. (2018). Stable isotope signatures of underground seedlings reveal the organic matter gained by adult orchids from mycorrhizal fungi. Funct. Ecol. 32, 870–881. doi: 10.1111/1365-2435.13042
Schweiger, J. M.-I., Kemnade, C., Bidartondo, M. I., and Gebauer, G. (2019). Light limitation and partial mycoheterotrophy in rhizoctonia-associated orchids. Oecologia 189, 375–383. doi: 10.1007/s00442-019-04340-0
Selosse, M. -A., and Martos, F. (2014). Do chlorophyllous orchids heterotrophically use mycorrhizal fungal carbon? Trends Plant Sci. 19, 683–685. doi: 10.1016/j.tplants.2014.09.005
Selosse, M.-A., and Roy, M. (2008). Green plants that feed on fungi, facts and questions about mixotrophy. Trends Plant Sci. 14, 64–70. doi: 10.1016/j.tplants.2008.11.004
Selosse, M.-A., Faccio, A., Scappaticci, G., and Bonfante, P. (2004). Chlorophyllous and ahlorophyllous specimens of Epipactis microphylla (Neotieae, Orchidaceae) are associaed with ectomycorrhizal septomycetes, including truffles. Microb. Ecol. 47, 416–426. doi: 10.1007/s00248-003-2034-3
Stöckel, M., Meyer, C., and Gebauer, G. (2011). The degree of mycoheterotrophic carbon gain in green, variegated and vegetative albino individuals of Cephalanthera damasonium is related to leaf chlorophyll concentrations. New Phytol. 189, 790–796. doi: 10.1111/j.1469-8137.2010.03510.x
Suetsugu, K., Yamato, M., Masubayashi, J., and Tayasu, I. (2019). Comprative study of nutritional mode and mycorrhizal fungi in green and albino variants of Goodyera velutina, an orchid mainly utilizing saprotrophic rhizoctonia. Mol. Ecol. 28, 4290–4299. doi: 10.1111/mec.15213
Tĕšitel, J., Tĕšitelová, T., Minasiewicz, J., and Selosse, M.-A. (2018). Mixotrophy in land plants, Why to stay green? Trends Plant Sci. 23:8. doi: 10.1016/j.tplants.2018.05.010
Tissue, D. T., Skillman, J. B., McDonald, E. P., and Strain, B. R. (1995). Photosynthesis and carbon allocation in Tipularia discolor (Orchidaceae), a wintergreen understory herb. Am. J. Bot. 82, 1249–1256. doi: 10.1002/j.1537-2197.1995.tb12658.x
Trudell, S., Rygiewicz, P., and Edmonds, R. L. (2003). Nitrogen and carbon stable isotope abundances support the myco-heterotrophic nature and host- specificity of certain achlorophyllous plants. New Phytol. 160, 391–401. doi: 10.1046/j.1469-8137.2003.00876.x
Wang, B., and Qiu, Y.-L. (2006). Phylogenetic distribution and evolution of mycorrhizas in land plants. Mycorrhiza 16, 299–363. doi: 10.1007/s00572-005-0033-6
Wang, D., Jacquemyn, H., Gomes, S. I. F., Vos, R. A., and Merckx, V. S. F. T. (2021). Symbiont switching and trophic mode shifts in Orchidaceae. New Phytol. 231, 791–800. doi: 10.1111/nph.17414
Whigham, D. F. (2004). The ecology of woodland herbs. Annu. Rev. Ecol. Syst. 35, 583–621. doi: 10.1146/annurev.ecolsys.35.021103.105708
Yagame, T., Orihara, T., Selosse, M.-A., Yamato, M., and Iwase, K. (2012). Mixotrophy of Platanthera minor, an orchid associated with ectomycorrhiza-forming Ceratobasidiaceae fungi. New Phytol. 193, 178–187. doi: 10.1111/j.1469-8137.2011.03896.x
Zhu, X. C., Song, F. B., Liu, S. Q., and Liu, F. L. (2011). Effects of arbuscular mycorrhizal fungus on photosynthesis and water status of maize under high temperature stress. Plant Soil 346, 189–199. doi: 10.1007/s11104-011-0809-8
Keywords: Goodyera pubescens, Tipularia discolor, mycoheterotrophy, orchid, mycorrhizae, stable isotope
Citation: McCormick MK, Good KL, Mozdzer TJ and Whigham DF (2022) Shade and drought increase fungal contribution to partially mycoheterotrophic terrestrial orchids Goodyera pubescens and Tipularia discolor. Front. Ecol. Evol. 10:1047267. doi: 10.3389/fevo.2022.1047267
Received: 17 September 2022; Accepted: 10 November 2022;
Published: 29 November 2022.
Edited by:
Isabel Marques, University of Lisbon, PortugalReviewed by:
César Marín, Universidad Santo Tomás, ChileGerhard Gebauer, University of Bayreuth, Germany
Copyright © 2022 McCormick, Good, Mozdzer and Whigham. This is an open-access article distributed under the terms of the Creative Commons Attribution License (CC BY). The use, distribution or reproduction in other forums is permitted, provided the original author(s) and the copyright owner(s) are credited and that the original publication in this journal is cited, in accordance with accepted academic practice. No use, distribution or reproduction is permitted which does not comply with these terms.
*Correspondence: Melissa K. McCormick, bWNjb3JtaWNrbUBzaS5lZHU=