- 1Leigh Marine Laboratory, Institute of Marine Science, University of Auckland, Leigh, New Zealand
- 2Department of Biology and Marine Biology, Center for Marine Science, University of North Carolina Wilmington, Wilmington, NC, United States
Introduction: The inner ear hair cells of fishes can provide insight into the early evolution of vertebrate inner ear structure. Fishes represent some of the first vertebrates to evolve auditory capacity, and the same basic structure, the sensory hair cell, provides the fundament for auditory and vestibular function in jawed vertebrates. Despite holding critical basal position in the evolutionary tree of gnathostomes, relatively little is known about inner ear hair cells in elasmobranchs. Specifically, the extent of plasticity in hair cell organization throughout ontogeny among different sensory epithelia and the degree of variation between species is unknown.
Methods: In this study, we characterized the inner ear hair cells of the New Zealand carpet shark Cephaloscyllium isabellum throughout ontogeny by quantifying macular area, number of hair cells, hair cell density, and hair cell orientations in the inner ear maculae from a range of body sizes.
Results: Similar to other elasmobranchs and bony fishes, macular area and the number of hair cells increased throughout ontogeny in the otolith organs. The orientations of hair cells within each maculae also was consistent with the limited data on other elasmobranchs. However, contrary to expectation, the macula neglecta did not increase in area or hair cell number throughout ontogeny, and hair cell density did not change with body size in any maculae.
Discussion: These findings suggest there may be variation between elasmobranch species in ontogenetic development of hair cell organization that may be related to hearing capabilities throughout life.
Introduction
All vertebrates share a common anatomical structure, the sensory hair cell (HC), which provides the basis for auditory and vestibular function (Hudspeth, 1983). Many basic auditory functions evolved early in vertebrate history and provide the foundation from which more complex auditory capabilities developed (Fay and Popper, 2000). Since fishes possess hearing mechanics that are thought to represent the ancestral state of vertebrates, studying the inner ear HCs of fishes can provide fundamental insight into the structure of early vertebrate auditory systems (Fay and Popper, 2000; Tricas, 2020). Moreover, the inner ears (including HCs) of fishes grow indeterminately (Corwin, 1983; Popper and Hoxter, 1984; Lombarte and Popper, 1994) and thus provide a unique opportunity to understand ontogenetic changes in the inner ear that may underlie changes in auditory capabilities throughout life in aquatic vertebrates. Despite this, only a handful of studies have investigated ontogenetic development of HCs in fishes (e.g., Corwin, 1983; Popper and Hoxter, 1984; Lombarte and Popper, 1994; Higgs et al., 2002; Wang et al., 2015; Chaves et al., 2017; Lozier and Sisneros, 2020; Sauer et al., 2022).
In fishes, there is likely substantial overlap of auditory and vestibular functions within the inner ear (Platt and Popper, 1981; Schulz-Mirbach et al., 2019). In fact, the early vertebrate inner ear likely served as a motion/body position sensor (vestibular organ) until an auditory role later evolved (Fritzsch et al., 2013; Platt and Straka, 2020). The inner ear of cyclostomes has either a single vertical semi-circular canal (hagfish) or two vertical canals (lampreys), along with a single otolith organ, the macula communis (Maklad et al., 2014). Lampreys also possess two horizontal canal ducts thought to have evolved in parallel to the horizontal canal characteristic of gnathostomes (Maklad et al., 2014). While the inner ear of cyclostomes is generally considered a vestibular organ, there is evidence for auditory sensitivity in the inner ear of lampreys (Mickle et al., 2019). In nearly all bony and cartilaginous fishes [with the exception of chimaeras (Fritzsch, 1992)], each inner ear is comprised of three semi-circular canals, three otolith organs (saccule, lagena, and utricle), and an otolith-lacking sensory organ called the macula neglecta (Figure 1). Each otolith organ contains an otolithic mass (i.e., otoconia or otolith) that overlies a sensory epithelium with HCs (termed macula) (Carlström, 1963). Collectively, the macula and otolithic mass function as a differential accelerometer, i.e., a mass that moves relative to a receptor in response to vibration (De Vries, 1950; Dijkgraaf, 1960; Pastras et al., 2020). The fish and the surrounding water have approximately the same density, while the otolithic mass has a greater density, causing a lag in vibrational disturbance between the macula and the otolithic mass; this deflects the HCs via shearing force and initiates a nervous system response (Flock, 1971; Hudspeth, 1983).
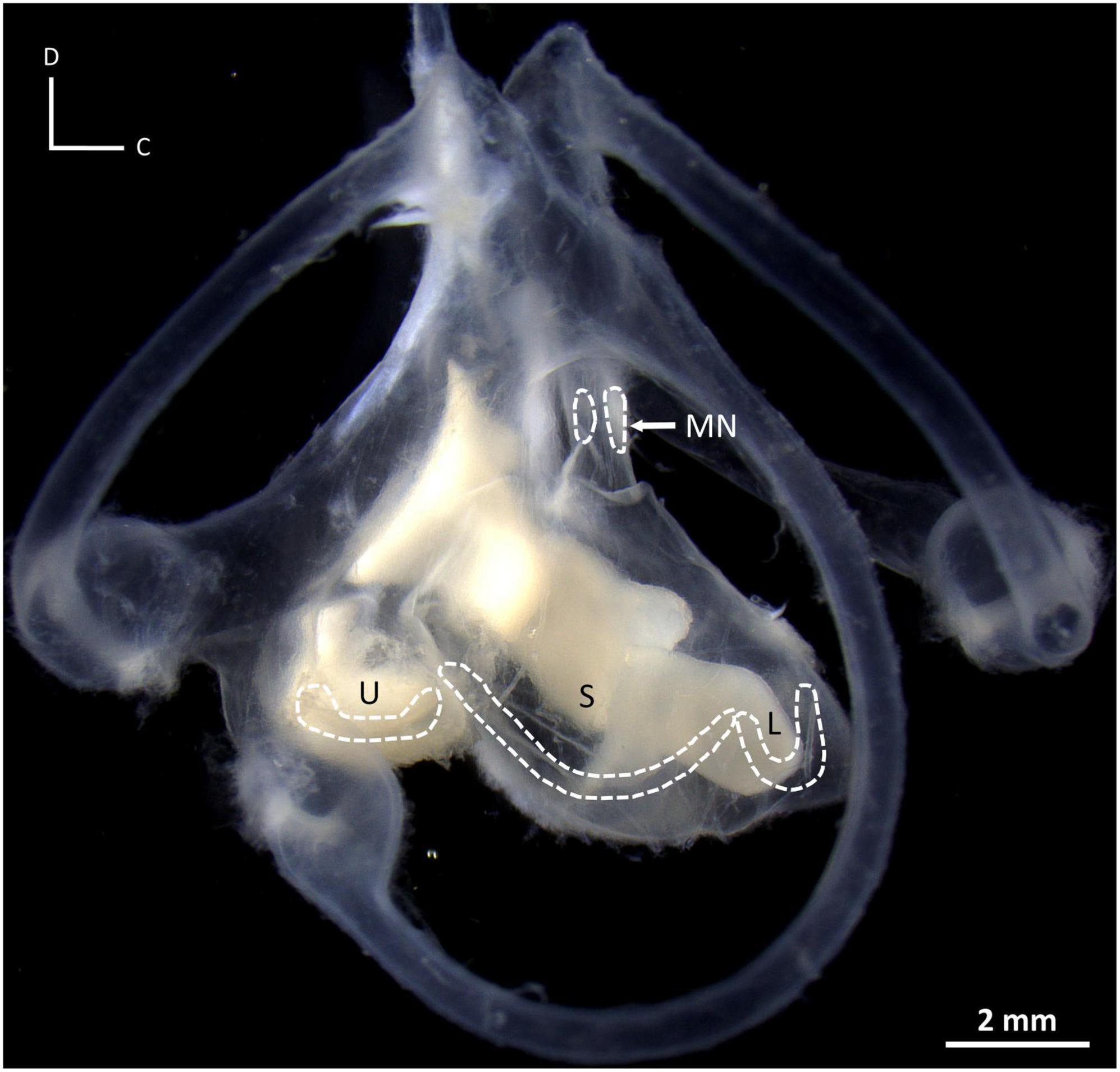
Figure 1. Photograph of the inner ear of a New Zealand carpet shark, Cephaloscyllium isabellum, illustrating the saccule (S), lagena (L), utricle (U), and macula neglecta (MN), and their associated maculae (dashed outlines). Note the maculae do not lie in the same plane in three-dimensional space. D, dorsal; C, caudal.
A single HC within the inner ear includes a bundle of numerous microvilli-like stereocilia and a single kinocilium (Wersäll, 1956). The kinocilium is located at one end of the bundle, making each HC morphologically and physiologically polarized. A HC’s axis of peak sensitivity (termed orientation) runs from the stereocilia toward the kinocilium (Lowenstein and Wersäll, 1959; Flock, 1964). Displacement in the direction of the kinocilium is excitatory, while displacement in the direction of the stereocilia is inhibitory (Hudspeth and Corey, 1977). In fishes, the HCs within the inner ear are distinctly grouped, such that their kinocilium and stereocilia are oriented in the same direction (Flock, 1964; Platt, 1977; Popper, 1977). While data are limited, it has been proposed that combining information across these orientation groups could enable directional hearing in fishes (Enger et al., 1973; Hawkins and Sand, 1977; Popper et al., 1988).
In fishes, growth of the inner ear is generally characterized by an increase in macula size and an increase in absolute number of HCs (Corwin, 1983; Popper and Hoxter, 1984; Lombarte and Popper, 1994; Chaves et al., 2017; Lozier and Sisneros, 2020; Sauer et al., 2022), decreasing density of HCs (Coffin et al., 2012; Chaves et al., 2017; Lozier and Sisneros, 2020; Sauer et al., 2022), and HC orientation groups that do not change (Lombarte and Popper, 1994; Chaves et al., 2017; Lozier and Sisneros, 2020; Sauer et al., 2022). While evidence for the functional significance of these changes is limited and inconsistent (Corwin, 1983; Higgs et al., 2002, 2003; Coffin et al., 2012; Lu and Desmidt, 2013; Wang et al., 2015), it seems likely that changes to the sensory epithelia will impact some aspect of auditory and/or vestibular capacity. However, the ontogenetic patterns above come from a small number of the c. 34,000 + extant species of fishes (Froese and Pauly, 2022) and are often limited to examination of a single macula within the inner ear (see review by Vasconcelos et al., 2016). Ontogenetic changes in HC organization need to be described in more species and maculae before an understanding of fish HC dynamics can be established.
Elasmobranchs (sharks, skates, and rays), represent some of the most basal jawed fishes, diverging over 400 million years ago (Whitenack et al., 2022). Given their position at the onset of gnathostomes, these fishes provide an opportunity to study an ancestral period in the evolution of jawed vertebrate inner ear structures. Furthermore, elasmobranchs possess a relatively well-developed macula neglecta, a sensory macula that is often diminutive in other fishes and may serve a critical role in hearing in fishes without a swim bladder (Lowenstein and Roberts, 1951; Tester et al., 1972; Fay et al., 1974; Corwin, 1989). This macula is composed of two patches of HCs in some species and lacks an otolithic mass, instead having a gelatinous cupula that seemingly functions likewise to the otolithic mass in the other inner ear organs (Tester et al., 1972; Fay et al., 1974; Corwin, 1977). Despite the opportunities that elasmobranchs impart on the study of fish inner ear maculae, much less is known about the HCs of elasmobranchs (Corwin, 1989; Myrberg, 2001; Chapuis and Collin, 2022; Mickle and Higgs, 2022) compared to bony fishes (Class: Osteichthyes), especially apart from the macula neglecta.
Limited data from elasmobranchs, predominantly from the macula neglecta, suggests that the inner ear maculae increase in size throughout life and that this growth is accompanied by the continual addition (and accumulation) of HCs (Corwin, 1981a,b, 1983, 1985; Barber et al., 1985; Parmentier et al., 2020). Only recently were these growth characteristics demonstrated comprehensively in all four maculae of a single species, where macular area and HC number increased throughout ontogeny in all inner ear organs in school sharks Galeorhinus galeus (Triakidae), with a concurrent ontogenetic decrease in the density of HCs and no growth-related changes in hair cell orientation groupings (Sauer et al., 2022). While these findings in G. galeus establish an important foundation for the study of elasmobranch inner ear HCs, whether these data are representative of all elasmobranchs is unknown.
The aim of this study was to describe the ontogenetic growth (macular area, total HC number, HC density, and HC orientations) in the four inner ear maculae of the New Zealand carpet shark Cephaloscyllium isabellum (Scyliorhinidae). We hypothesized that the inner ear maculae of C. isabellum would exhibit ontogenetic development similar to G. galeus, with increases in maculae size and HC number and decreases in HC density from juvenile to adult sizes.
Materials and methods
Fish collection
Eighteen C. isabellum (12 females, 6 males), ranging in size from 40.5 to 82.0 cm total length, were obtained from local commercial fishers in Leigh, New Zealand. Cephaloscyllium isabellum are born around 16 cm in total length (Graham, 1953), with sexual maturity occurring around 60 cm in males and 76 cm in females, and a maximum size of approximately 95 cm (Horn, 2016). Sex and body size information (to the nearest 0.1 cm) were recorded from fresh, unfixed samples. The otic capsules were excised from each shark and immersion-fixed in 4% paraformaldehyde in 0.1 M phosphate buffer (PB). These samples were post-fixed for a minimum of 7 days (maximum of 8 weeks) before the inner ears were extracted.
Fluorescence microscopy and imaging
The maculae within the inner ears were isolated and rinsed in 0.1 M PB solution before being stained in a solution containing 5 μl Oregon Green 488 Phalloidin (Invitrogen) and 200 μl PB (1:40 dilution). Phalloidin selectively binds to the actin in HCs, enabling visualization of individual HCs (Lu and Popper, 1998). Maculae were stained for 25 min, rinsed in PB, and then mounted onto a glass microscope slide using Prolong Glass Antifade Mountant (Invitrogen), with the apical surface of the HCs facing upward.
Maculae were observed with a Nikon ECLIPSE Ni-U microscope equipped with an Intensilight C-HGFI epi-fluorescence illuminator, FITC filter set (excitation 465–495 nm), and Nikon DS-Qi2 camera. NIS-BR Elements imaging software was used to image the maculae, digitally measure the area (μm2) of each macula, and count HCs. Macular area was measured from stitched 4x/0.13 N.A. objective images, HC counts were performed on 20x/0.50 N.A. objective images, and HC orientation patterns were established from 40x/0.75 N.A. objective images (Figure 2). Macular area measurements were not corrected for shrinkage due to fixation. In this study, HCs were defined as individual bundles of stereocilia and thus excluded any cells without stereocilia (i.e., early developing HCs). HC orientations were determined visually based on the direction of excitation (i.e., from the stereocilia toward the kinocilium). The kinocilium of each HC can readily be located because it lacks actin and appears as a dark semi-circle on fluorescently stained HCs (Lu and Popper, 1998; Figure 2C).
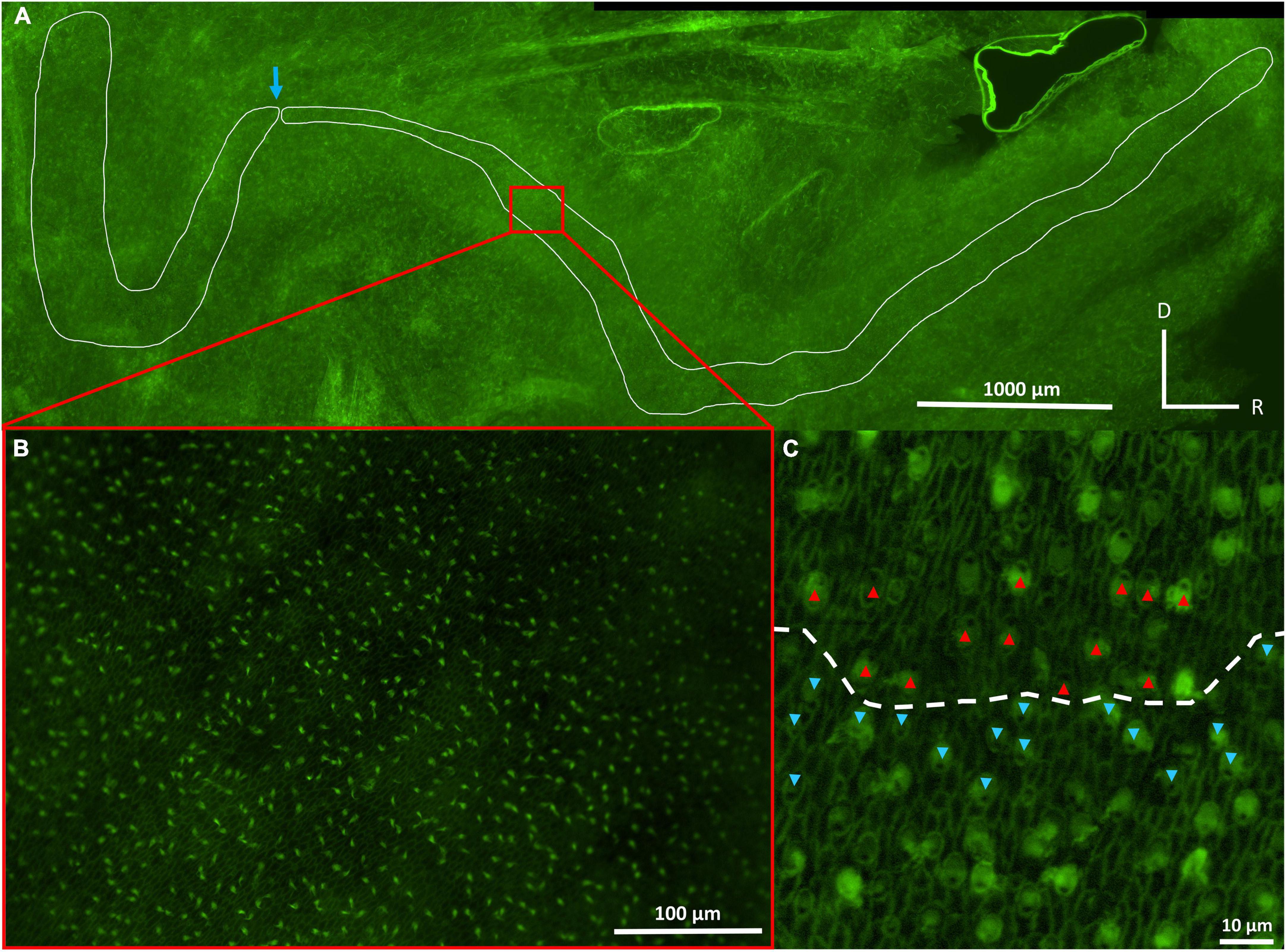
Figure 2. Photographs of the inner ear maculae of Cephaloscyllium isabellum, showing the saccular (right) and lagenar (left) maculae at 4× objective (A), saccular hair cells at 20× objective (B), and saccular hair cells at 40× objective (C). The blue arrow (A) indicates a natural bend in the lagenar/saccular maculae that was used as an anatomical marker to consistently differentiate and separate the two maculae. The kinocilium of each hair cell, which lacks actin, appears dark relative to the stereocilia (C). The red and blue arrows (C) indicate the orientation of individual hair cells, which is determined by the location of the kinocilium (dark spot) relative to the stereocilia. The dashed line (C) designates the approximate boundary between two distinct groups of hair cells. D, dorsal; R, rostral.
Morphological analysis
Hair cell density in the macula neglecta was quantified by dividing the total number of hair cells (HCT) by the macular area, while in the otolith organs HCD was estimated using a stereological approach (see Sauer et al., 2022). In brief, HCs were imaged and counted in three non-overlapping regions along the length of each macula (rostral, medial, and caudal) (see Supplementary Figure 1) that allowed for inclusion of the full width of the macula (both the central and marginal zones) and consistent counting of approximately 15% of the total macular area. HCD was calculated in each region by dividing the number of HCs (including those partially within a region) by the area of the region (HCs/μm2). HCD from the three regions was then averaged to estimate overall HCD for each macula. HC orientations were examined in all the quantified regions and in additional images acquired from the intermediate (between the rostral, medial, and caudal portions) and terminal parts of each macula to establish orientation groups throughout the entirety of each macula.
Since one-way analysis of variance (ANOVA) tests indicated no significant differences in HCD between the different regions (rostral, medial, and caudal) of the saccule (F2,42 = 0.62, p = 0.541), lagena (F2,48 = 2.50, p = 0.092), and utricle (F2,48 = 2.76, p = 0.073), HCT in these maculae was estimated by extrapolating the overall HCD to the total macular area. In the macula neglecta, all HCs in the entire macula were counted to determine HCT.
Statistical analyses
Given the fragility of inner ear tissues, measurements of macular area, HCT, and HCD could not be obtained from every maculae of all 18 individuals. All available data were included in our analyses, which included macular area measurements from 18 individuals (15 saccules, 16 lagenas, 17 utricles, and 18 macula neglectas), HCT measurements from 18 individuals (15 saccules, 16 lagenas, 17 utricles, and 18 macula neglectas), and HCD measurements from 18 individuals (15 saccules, 17 lagenas, 17 utricles, and 18 macula neglectas). Preliminary paired t-tests showed no significant differences in macular area or HCD between left and right maculae (see Supplementary material); therefore, left and right data were pooled to increase statistical power for analyses. Data from males and females were also pooled, since two-tailed t-tests did not detect any differences in macular area or HCD between sexes in any maculae (see Supplementary material). The macula neglecta was treated as a single macula since macular area and HCD did not significantly differ between the two patches of this macula (see Supplementary material).
The best fit model (linear, log10-transformed, or square-root transformed) was determined using corrected Akaike information criterion (AICc), which corrects for bias when using small sample sizes (Cavanaugh, 1997). Across all candidate models tested for macula area, HCD, and HCT against total length, a log10-transformation was the best fit, as determined by the lowest AICc value (Burnham and Anderson, 2002).
Macular area, HCT, and HCD were log10-transformed and the scaling relationships between these parameters and log10-transformed body size (total length) were assessed using generalized least squares (GLS) regression, with total length as the independent variable. A one-way analysis of covariance (ANCOVA) was performed to test for differences in GLS regression slopes and intercepts for macular area, HCD, and HCT, with total length as the continuous independent variable and macula as a categorical variable. Standardized residuals from linear regressions of total length and macular area, HCD, and HCT were calculated to remove the effect of size from each dependent variable. Standardized residuals were compared using Welch’s ANOVA to analyze variation in macular area and HCT between maculae, and one way ANOVA to analyze variation in HCD between maculae. Post hoc Games-Howell and Tukey HSD tests, respectively, were performed to determine significantly different means (α = 0.05). The Benjamini and Yekutieli correction method (Benjamini and Yekutieli, 2001) was used to adjust all p-values from sequential ANOVA and post hoc tests to control the False Discovery rate across multiple comparisons. The assumptions of all ANOVA analyses were met by using Anderson-Darling normality tests and Levene’s test for homogeneity of variances.
Results
Ontogenetic changes
Macular area increased significantly with body size (total length, TL) for the saccule, lagena, and utricle in C. isabellum (Figure 3A), whereby these inner ear maculae continually increase in size (area) throughout life. For example, the saccular macula nearly doubled in absolute size from 792,153 μm2 in a 40 cm juvenile fish to 1,524,930 μm2 in an 81 cm adult fish. There was, however, no significant relationship between TL and macular area in the macula neglecta, indicating a lack of ontogenetic growth of this macula. The macula neglecta was therefore not included in slope and intercept comparisons, but the slopes (F2,45 = 0.99, p = 0.379) and intercepts (F2,45 = 1.39, p = 0.260) of the regression lines from the saccule, lagena, and utricle were not significantly different.
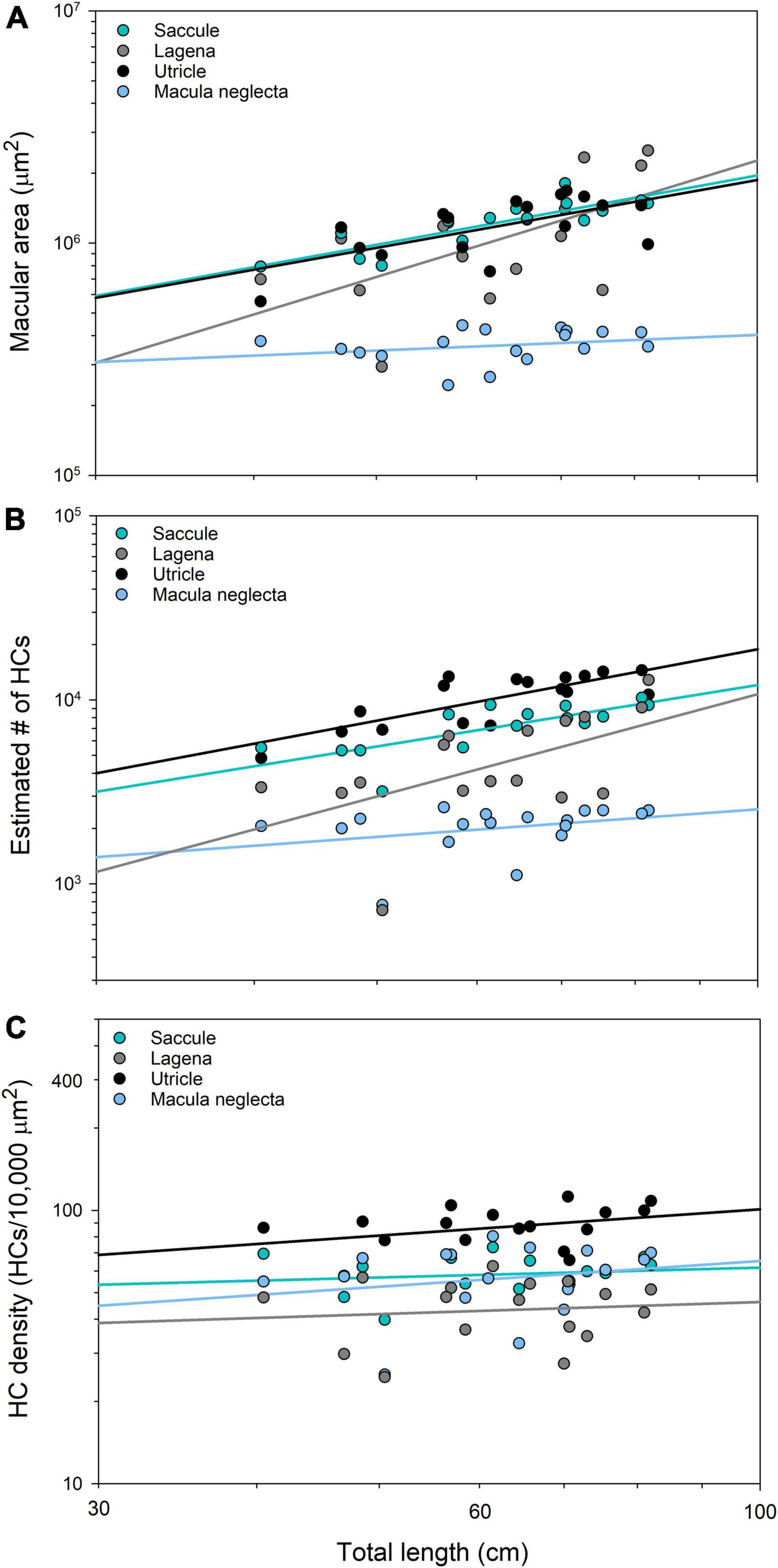
Figure 3. Regression of total length and macular area (A), total hair cell number (B), and hair cell density (C) (logarithmic axes) in the four inner ear maculae of Cephaloscyllium isabellum. Macular area significantly increases with total length in all maculae except the macula neglecta (Saccule = 0.99x + 4.31, F1,13 = 33.53, p < 0.001, r2 = 0.72; Lagena = 1.67x + 3.02, F1,14 = 7.93, p = 0.014, r2 = 0.36; Utricle = 0.97x + 4.33, F1,15 = 10.60, p = 0.005, r2 = 0.41; Macula neglecta = 0.22x + 5.16, F1,16 = 1.22, p = 0.286, r2 = 0.07). The total number of hair cells significantly increases with total length in all maculae except the macula neglecta (Saccule = 1.11x + 1.86, F1,13 = 16.22, p = 0.001, r2 = 0.56; Lagena = 1.85x + 0.34, F1,14 = 6.60, p = 0.022, r2 = 0.32; Utricle = 1.29x + 1.70, F1,15 = 24.94, p < 0.001, r2 = 0.62; Macula neglecta = 0.50x + 2.41, F1,16 = 1.75, p = 0.205, r2 = 0.10). There was no significant relationship between total length and hair cell density in any maculae (Saccule = 0.12x + 1.55, F1,13 = 0.32, p = 0.581, r2 = 0.02; Lagena = 0.15x + 1.37, F1,15 = 0.18, p = 0.678, r2 = 0.01; Utricle = 0.32x + 1.37, F1,15 = 2.24, p = 0.156, r2 = 0.13; Macula neglecta = 0.31x + 1.19, F1,16 = 0.714, p = 0.411, r2 = 0.04).
Total number of hair cells increased significantly with TL in the saccule, lagena, and utricle (Figure 3B), which indicates that the total number of HCs in these maculae increases throughout the life of the fish. For example, the smallest C. isabellum had an approximated 4,830 saccular HCs, while the largest had a 3 fold increase to approximately 14,492. However, there was no significant relationship between HCT and TL in the macula neglecta, again revealing a lack of ontogenetic growth; therefore, it was omitted from slope and intercept comparisons. The slopes of the regression lines of data from the saccule, lagena, and utricle were not significantly different (F2,45 = 0.63, p = 0.540), but the intercepts were significantly different (F2,45 = 21.66, p < 0.001).
There was no significant relationship between TL and HCD in the saccule, lagena, utricle, or macula neglecta (Figure 3C), whereby HCD did not change throughout ontogeny in any of the maculae. The slopes of the regression lines were not significantly different (F3,63 = 0.13, p = 0.945); however, the intercepts differed significantly (F3,63 = 24.36, p < 0.001).
Macula comparisons
Mean relative macular area (standardized residuals) was not significantly different between the saccule, lagena, and utricle; however, the macula neglecta was significantly smaller than all other maculae (F3,33 = 138.99, p < 0.001; Figure 4A). Mean relative HCT was greatest in the utricle, followed by the saccule and lagena, with the macula neglecta having the fewest HCs (F3,33 = 106.09, p < 0.001; Figure 4B). Mean relative HCD was the greatest in the utricle, followed by the saccule and macula neglecta, with the lagena having the lowest density of HCs (F3,63 = 25.86, p < 0.001; Figure 4C).
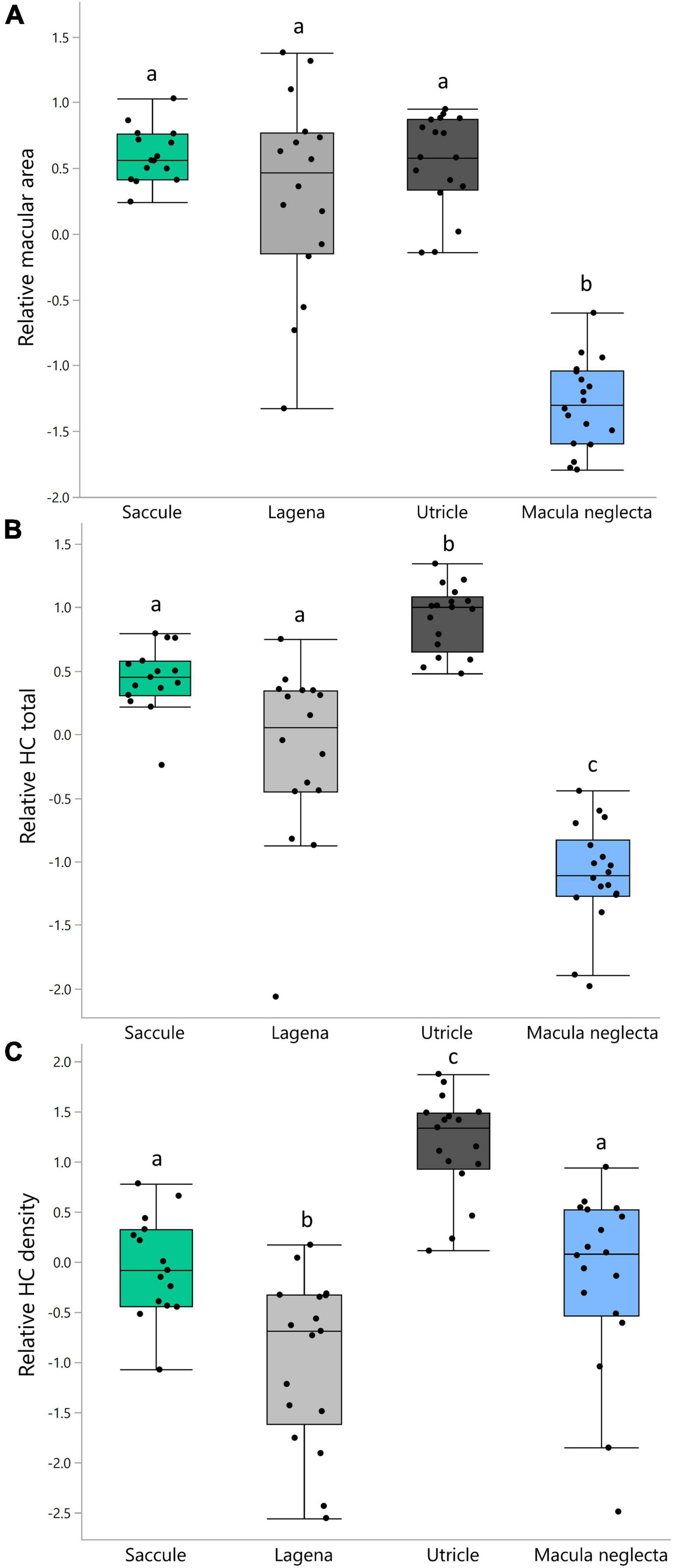
Figure 4. Boxplots of relative (standardized residuals) macular area (A), relative hair cell total (B), and relative hair cell density (C) in the four inner ear maculae of Cephaloscyllium isabellum. Significantly different means (α = 0.05) within each panel are indicated by differing lowercase letters.
Sensory hair cell orientations
Sensory hair cell orientation groups were the same in all C. isabellum specimens examined in this study; there was no ontogenetic variation. All maculae exhibited a bi-directional orientation pattern, where HCs are split into two groups with opposing (180° difference) orientations. The saccule and lagena have two groups of HCs that are oriented away (perpendicularly) from a midline that bisects the maculae (Figures 5A,B), while the utricle has two groups that are oriented toward a midline (Figure 5C). The macula neglecta is composed of two separate patches of HCs with opposing orientations (Figure 5D).
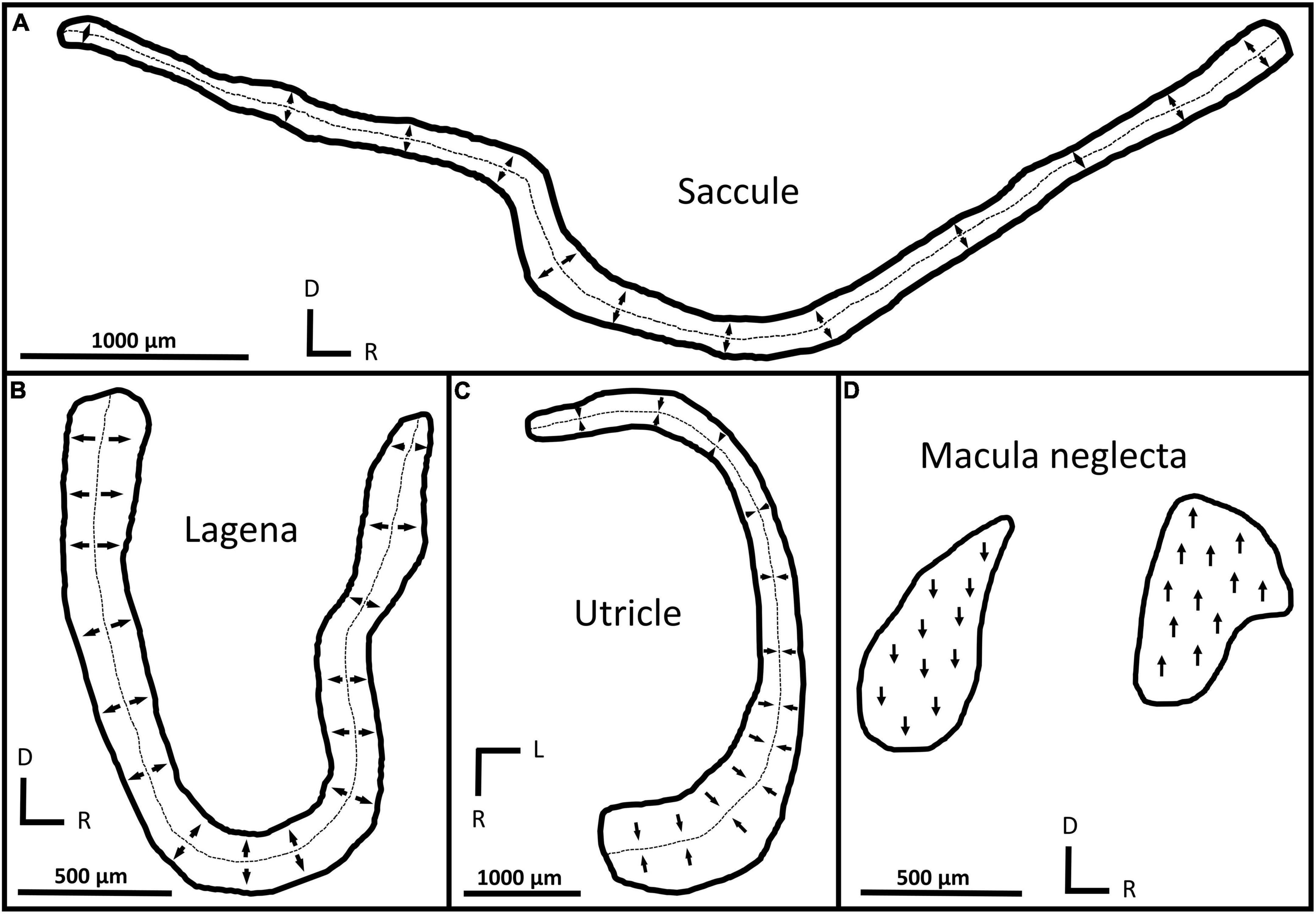
Figure 5. Tracings of macula shapes and orientation groups in the saccule (A), lagena (B), utricle (C), and macula neglecta (D) from C. isabellum. Arrows indicate the orientation of hair cells within different locations of the maculae and the dotted lines mark the approximate boundary between orientation groups. D, dorsal; R, rostral; L, lateral.
Discussion
The present study described the inner ear sensory maculae of a benthic elasmobranch species, the New Zealand carpet shark C. isabellum. Results show that macular area and number of hair cells (HCT) increased throughout ontogeny (except in the macula neglecta), but hair cell density (HCD) exhibited no ontogenetic change. There were significant differences in macular area, HCT, and HCD between the four inner ear maculae, while HC orientation groups were the same in all C. isabellum, regardless of size. These findings represent the first description of inner ear HC organization in C. isabellum and provide fundamental information about the organization and plasticity of inner ear HCs throughout life in elasmobranchs.
Both macular area and HCT increased linearly with body size in the saccule, lagena, and utricle of C. isabellum, with the steepest rate of growth in the lagena. Linear ontogenetic increases in macular area and HCT were also documented in the saccule, lagena, and utricle of G. galeus, the only other ontogenetic study of inner ear HCs in elasmobranch otolith organs (Sauer et al., 2022). Studies on various otolith organs of bony fishes have also reported ontogenetic increases in macular area and HCT (Popper and Hoxter, 1984; Lombarte and Popper, 1994; Higgs et al., 2002, 2003; Chaves et al., 2017; Lozier and Sisneros, 2020). While the functional significance of these ontogenetic changes is unclear, it has been suggested that increasing HCT may enhance auditory sensitivity as fishes grow (Corwin, 1983; Coffin et al., 2012; Lu and Desmidt, 2013; Wang et al., 2015) or could act to maintain stable hearing throughout ontogeny (Popper et al., 1988; Rogers et al., 1988; Higgs et al., 2003). Increasing HCT may also reflect vestibular requirements, such as maintaining stable body position at larger body sizes (Lowenstein and Roberts, 1949, 1951; Platt and Straka, 2020). Coupling the data here with auditory or vestibular sensitivity information throughout ontogeny in fishes, especially elasmobranchs, is critical to better understand how morphological changes relate to changes in inner ear capacity.
Contrary to the otolith organs, the macula neglecta, thought to serve an important role in sound detection in sharks (Fay et al., 1974; Corwin, 1981b), did not exhibit ontogenetic increases in macular area or HCT in C. isabellum; it maintained the same size and number of HCs despite animal growth (including in the other maculae). This result is unexpected, as previous studies have shown ontogenetic growth in the macula neglecta of other elasmobranchs (Corwin, 1983; Barber et al., 1985; Sauer et al., 2022). However, the present study examined individuals ranging from juveniles to sexually mature adults (40.5–82 cm total length) and did not include any neonates (16 cm length at birth; Graham, 1953). It is possible that a peak period of growth in the macula neglecta occurs in early ontogeny in C. isabellum. It has been suggested that benthic shark species, such as C. isabellum, may possess a smaller macula neglecta with fewer HCs compared to more pelagic species (Corwin, 1978); thus, growth of the macula neglecta may slow or even stop early in life. The comparatively small size and lack of ontogenetic growth of the macula neglecta in C. isabellum lend support to this notion, but more data are required from shark species, both benthic and pelagic, to empirically test this hypothesis.
In C. isabellum, HCD did not change throughout ontogeny in any inner ear maculae, suggesting that new HC’s proliferate at a rate concomitant with macular growth. These findings are in contrast with those from G. galeus, where ontogenetic decreases in HCD were documented in the saccule, lagena, and macula neglecta (but not the utricle) (Sauer et al., 2022). The smaller size range of C. isabellum (∼20–90 cm) compared to G. galeus (∼30–170 cm) might minimize ontogenetic changes in HCD, although Corwin (1977, 1981a) reported no differences in HCD of the macula neglecta between juvenile and adult blacktip and gray reef sharks (Carcharhinus melanopterus and Carcharhinus amblyrhynchos, respectively), two species with similar size ranges to G. galeus. There is likely variation in HCD patterns between elasmobranchs, similar to in bony fishes, where data indicate that ontogenetic changes in HCD may be species-specific, macula-specific, and even dependent on developmental stage (Popper and Hoxter, 1984; Lombarte and Popper, 1994; Higgs et al., 2002; Lu and Desmidt, 2013; Wang et al., 2015; Chaves et al., 2017; Lozier and Sisneros, 2020). Further quantification of HCD across full size ranges and in all inner ear maculae, especially in elasmobranchs, is needed to characterize postembryonic development of HCD in fishes.
Although HC orientations have been described in only a few species (Lowenstein et al., 1964; Corwin, 1977, 1978, 1981b; Barber and Emerson, 1980; Lovell et al., 2007), the inner ear maculae of elasmobranchs are generally thought to have two groups of HCs, with opposing orientations: a basal pattern from which others likely derived (Hawkins and Popper, 2018). Consistent with this notion, the maculae in C. isabellum contain two groups of HCs with opposing orientations, and these orientation groups did not change with body size. The orientation groups in C. isabellum are identical to those reported in the maculae of G. galeus (Sauer et al., 2022), and match those from the macula neglecta (Corwin, 1977, 1978) and saccule (Corwin, 1981b; Lovell et al., 2007) of other sharks. Conversely, the orientation patterns of C. isabellum do not resemble those in the macula neglecta (Lowenstein et al., 1964; Barber and Emerson, 1980; Corwin, 1983; Barber et al., 1985), lagena (Lowenstein et al., 1964; Barber and Emerson, 1980), or utricle (Lowenstein et al., 1964) of rays and skates, or those from another scyliorhinid shark, lesser-spotted dogfish (Scyliorhinus canicula) (Lovell et al., 2007), suggesting there is variability between elasmobranchs, even in closely related species. While the extent of diversity in HC orientation patterns among elasmobranchs is unknown, there is notable interspecific variation in gross morphology of the inner ear (Corwin, 1978; Evangelista et al., 2010), which suggests broad diversity in anatomy could be present at the cellular level too. Further examination of HC orientations in new elasmobranch species is needed to determine the degree and distribution of diversity among the clade.
The role(s) of each inner ear organ in regards to auditory and vestibular function is ambiguous in fishes (Platt and Popper, 1981; Schulz-Mirbach et al., 2019). The saccule has largely been considered the primary auditory organ in fishes, but it is likely that functional overlap exists within the inner ear organs, rather than a strict division of auditory and vestibular function (see review by Schulz-Mirbach et al., 2019). In C. isabellum, the significant differences in macular area, HCT, and HCD between the four maculae indicate that some maculae have distinct characteristics that may relate to specific roles in auditory and/or vestibular function. While the relative importance of these different characteristics (macular area, HCT, HCD) to inner ear function is unknown, differences in HC number and/or density may relate to auditory capacity (Corwin, 1983; Coffin et al., 2012; Lu and Desmidt, 2013; Wang et al., 2015; Chaves et al., 2017; Lozier and Sisneros, 2020). Accordingly, the utricle and saccule, which exhibited a relatively large size, HC number, and HC density in C. isabellum, may possess greater sensitivity to acoustical displacements compared to the other maculae. However, it is equally likely that variation in HC characteristics reflects vestibular requirements as body size increases and has minimal impact on auditory sensitivity (Higgs et al., 2002, 2003; Platt and Straka, 2020). More data, both morphological and physiological, are needed to assess the functional roles of the inner ear maculae in elasmobranchs.
Conclusions
Very little is known about how the inner ear varies throughout life in elasmobranchs. This study assessed the sensory HCs within all four inner ear organs of C. isabellum and characterized how they change throughout ontogeny. The inner ear maculae in C. isabellum display unique ontogenetic changes that suggest there could be variation between elasmobranchs, and may have implications for auditory and/or vestibular capacity throughout life. Inner ear ultrastructure suggests at least the potential for high relative importance of the saccule and utricle in C. isabellum in the detection of vibrational disturbances, and provides prerequisite information necessary for establishing structure-function relationships in the inner ear organs of elasmobranchs. Further assessment of the inner ear maculae within and across a range of elasmobranchs may help elucidate the relative importance of hearing and vestibular function throughout life and between species in this ancient group of vertebrates.
Data availability statement
The raw data supporting the conclusions of this article will be made available by the authors, without undue reservation.
Ethics statement
This animal study was reviewed and approved by the University of Auckland Animal Ethics Committee.
Author contributions
DS, KY, and CR conceived and designed the research. DS collected and analyzed the data and led the writing of the manuscript, with review and editing by KY and CR. All authors gave approval for publication of the manuscript.
Funding
This research was supported by the University of Auckland Doctoral Scholarship to DS and the Royal Society Te Apārangi Marsden Fund (UOA1808) awarded to CR and KY.
Acknowledgments
We would like to sincerely thank Errol Murray, Maria Mugica, and Kate Shears for technical support throughout this project, and the commercial and recreational fishers in Leigh for donation of specimens, with special mention to Jake Brebner and Gavin Perry. We would also like to acknowledge the University of Auckland and Royal Society Te Apārangi for funding that made this work possible.
Conflict of interest
The authors declare that the research was conducted in the absence of any commercial or financial relationships that could be construed as a potential conflict of interest.
Publisher’s note
All claims expressed in this article are solely those of the authors and do not necessarily represent those of their affiliated organizations, or those of the publisher, the editors and the reviewers. Any product that may be evaluated in this article, or claim that may be made by its manufacturer, is not guaranteed or endorsed by the publisher.
Supplementary material
The Supplementary Material for this article can be found online at: https://www.frontiersin.org/articles/10.3389/fevo.2022.1034891/full#supplementary-material
Abbreviations
HCs, sensory hair cells; HCT, total number of hair cells; HCD, hair cell density.
References
Barber, V. C., and Emerson, C. J. (1980). Scanning electron microscopic observations on the inner ear of the skate, Raja ocellata. Cell Tissue Res. 205, 199–215. doi: 10.1007/BF00234680
Barber, V. C., Yake, K. I., Clark, V. F., and Pungur, J. (1985). Quantitative analyses of sex and size differences in the macula neglecta and ramus neglectus in the inner ear of the skate, Raja ocellata. Cell Tissue Res. 241, 597–605. doi: 10.1007/BF00214581
Benjamini, Y., and Yekutieli, D. (2001). The control of the false discovery rate in multiple testing under dependency. Ann. Stat. 29, 1165–1188. doi: 10.1214/aos/1013699998
Burnham, K. P., and Anderson, D. R. (2002). “A practical information-theoretic approach,” in Model Selection and Multimodel Inference, eds K. P. Burnham and D. R. Anderson (Berlin: Springer), 70–71. doi: 10.1007/b97636
Carlström, D. (1963). A crystallographic study of vertebrate otoliths. Biol. Bull. 123, 441–463. doi: 10.2307/1539358
Cavanaugh, J. E. (1997). Unifying the derivations for the Akaike and corrected Akaike information criteria. Stat. Probab. Lett. 33, 201–208. doi: 10.1016/s0167-7152(96)00128-9
Chapuis, L., and Collin, S. P. (2022). The auditory system of cartilaginous fishes. Rev. Fish Biol. Fish. 32, 521–554. doi: 10.1007/s11160-022-09698-8
Chaves, P. P., Valdoria, C. M. C., Amorim, M. C. P., and Vasconcelos, R. O. (2017). Ontogenetic development of the inner ear saccule and utricle in the Lusitanian toadfish: Potential implications for auditory sensitivity. Hear. Res. 353, 112–121. doi: 10.1016/j.heares.2017.06.008
Coffin, A. B., Mohr, R. A., and Sisneros, J. A. (2012). Saccular-specific hair cell addition correlates with reproductive state-dependent changes in the auditory saccular sensitivity of a vocal fish. J. Neurosci. 32, 1366–1376. doi: 10.1523/JNEUROSCI.4928-11.2012
Corwin, J. T. (1977). Morphology of the macula neglecta in sharks of the genus Carcharhinus. J. Morphol. 152, 341–361. doi: 10.1002/jmor.1051520306
Corwin, J. T. (1978). The relation of inner ear structure to the feeding behavior in sharks and rays. Scan. Electron Microsc. 2, 1105–1112.
Corwin, J. T. (1981a). Postembryonic production and aging of inner ear hair cells in sharks. J. Comp. Neurol. 201, 541–553. doi: 10.1002/cne.902010406
Corwin, J. T. (1981b). Peripheral auditory physiology in the lemon shark: Evidence of parallel otolithic and non-otolithic sound detection. J. Comp. Physiol. A 142, 379–390. doi: 10.1007/BF00605450
Corwin, J. T. (1983). Postembryonic growth of the macula neglecta auditory detector in the ray, Raja clavata: Continual increases in hair cell number, neural convergence, and physiological sensitivity. J. Comp. Neurol. 217, 345–356. doi: 10.1002/cne.902170309
Corwin, J. T. (1985). Auditory neurons expand their terminal arbors throughout life and orient toward the site of postembryonic hair cell production in the macula neglecta in elasmobranchs. J. Comp. Neurol. 239, 445–452. doi: 10.1002/cne.902390410
Corwin, J. T. (1989). Functional anatomy of the auditory system in sharks and rays. J. Exp. Zool. 252, 62–74. doi: 10.1002/jez.1402520408
De Vries, H. (1950). The mechanics of the labyrinth otoliths. Acta Otolaryngol. 38, 262–273. doi: 10.3109/00016485009118384
Dijkgraaf, S. (1960). Hearing in bony fishes. Proc. R. Soc. London B. Biol. Sci. 152, 51–54. doi: 10.1098/rspb.1960.0022
Enger, P. S., Hawkins, A. D., Sand, O., and Chapman, C. J. (1973). Directional sensitivity of saccular microphonic potentials in the haddock. J. Exp. Biol. 59, 425–433. doi: 10.1242/jeb.59.2.425
Evangelista, C., Mills, M., Siebeck, U. E., and Collin, S. P. (2010). A comparison of the external morphology of the membranous inner ear in elasmobranchs. J. Morphol. 271, 483–495. doi: 10.1002/jmor.10812
Fay, R. R., Kendall, J. I., Popper, A. N., and Tester, A. L. (1974). Vibration detection by the macula neglecta of sharks. Comp. Biochem. Physiol. Part A Physiol. 47, 1235–1240. doi: 10.1016/0300-9629(74)90097-8
Fay, R. R., and Popper, A. N. (2000). Evolution of hearing in vertebrates: The inner ears and processing. Hear. Res. 149, 1–10. doi: 10.1016/S0378-5955(00)00168-4
Flock, Å (1964). Structure of the macula utriculi with special reference to directional interplay of sensory responses as revealed by morphological polarization. J. Cell Biol. 22, 413–431. doi: 10.1083/jcb.22.2.413
Flock, Å (1971). “Sensory transduction in hair cells,” in Principles of Receptor Physiology. Handbook of Sensory Physiology, ed. W. R. Loewenstein (Heidelberg: Springer), 396–441. doi: 10.1007/978-3-642-65063-5_14
Fritzsch, B. (1992). “The water-to-land transition: Evolution of the tetrapod basilar papilla, middle ear, and auditory nuclei,” in The Evolutionary Biology of Hearing, eds D. B. Webster, A. N. Popper, and R. R. Fay (New York, NY: Springer), doi: 10.1007/978-1-4612-2784-7_22
Fritzsch, B., Pan, N., Jahan, I., Duncan, J. S., Kopecky, B. J., Elliott, K. L., et al. (2013). Evolution and development of the tetrapod auditory system: an organ of Corti-centric perspective. Evol. Dev. 15, 63–79. doi: 10.1111/ede.12015
Froese, R., and Pauly, D. (eds) (2022). FishBase. World Wide Web Electronic Publication. Berlin: Springer.
Hawkins, A. D., and Popper, A. N. (2018). Directional hearing and sound source localization by fishes. J. Acoust. Soc. Am. 144, 3329–3350. doi: 10.1121/1.5082306
Hawkins, A. D., and Sand, O. (1977). Directional hearing in the median vertical plane by the cod. J. Comp. Physiol. A 122, 1–8. doi: 10.1007/BF00611244
Higgs, D. M., Rollo, A. K., Souza, M. J., and Popper, A. N. (2003). Development of form and function in peripheral auditory structures of the zebrafish (Danio rerio). J. Acoust. Soc. Am. 113, 1145–1154. doi: 10.1121/1.1536185
Higgs, D. M., Souza, M. J., Wilkins, H. R., Presson, J. C., and Popper, A. N. (2002). Age- and size-related changes in the inner ear and hearing ability of the adult zebrafish (Danio rerio). J. Assoc. Res. Otolaryngol. 3, 174–184. doi: 10.1007/s101620020035
Horn, P. L. (2016). Biology of the New Zealand carpet shark Cephaloscyllium isabellum (Scyliorhinidae). J. Ichthyol. 56, 336–347. doi: 10.1134/S0032945216030048
Hudspeth, A. J. (1983). The hair cells of the inner ear. Sci. Am. 248, 54–64. doi: 10.1038/scientificamerican0183-54
Hudspeth, A. J., and Corey, D. P. (1977). Sensitivity, polarity, and conductance change in the response of vertebrate hair cells to controlled mechanical stimuli. Proc. Natl. Acad. Sci. U.S.A. 74, 2407–2411. doi: 10.1073/pnas.74.6.2407
Lombarte, A., and Popper, A. N. (1994). Quantitative analyses of postembryonic hair cell addition in the otolithic endorgans of the inner ear of the european hake. Merluccius merluccius (gadiformes, teleostei). J. Comp. Neurol. 345, 419–428. doi: 10.1002/cne.903450308
Lovell, J. M., Findlay, M. M., Harper, G. M., and Moate, R. M. (2007). The polarization of hair cells from the inner ear of the lesser spotted dogfish Scyliorhinus canicula. J. Fish Biol. 70, 362–373. doi: 10.1111/j.1095-8649.2006.01304.x
Lowenstein, O., Osborne, M. P., and Wersaäll, J. (1964). Structure and innervation of the sensory epithelia of the labyrinth in the thornback ray (Raja clavata). Proc. R. Soc. L. Ser. B Biol. Sci. 160, 1–12. doi: 10.1098/rspb.1964.0026
Lowenstein, O., and Roberts, T. D. (1949). The equilibrium function of the otolith organs of the thornback ray (Raja clavata). J Physiol. 110, 392–415. doi: 10.1113/jphysiol.1949.sp004448
Lowenstein, O., and Roberts, T. D. (1951). The localization and analysis of the responses to vibration from the isolated elasmobranch labyrinth. A contribution to the problem of the evolution of hearing in vertebrates. J. Physiol. 114, 471–489. doi: 10.1113/jphysiol.1951.sp004638
Lowenstein, O., and Wersäll, J. (1959). A functional interpretation of the electron-microscopic structure of the sensory hairs in the cristae of the elasmobranch Raja clavata in terms of directional sensitivity. Nature 184, 1807–1808. doi: 10.1038/1841807a0
Lozier, N. R., and Sisneros, J. A. (2020). Ontogeny of inner ear saccular development in the plainfin midshipman (Porichthys notatus). Brain Behav. Evol. 95, 330–340. doi: 10.1159/000516477
Lu, Z., and Desmidt, A. A. (2013). Early development of hearing in zebrafish. J. Assoc. Res. Otolaryngol. 14, 509–521. doi: 10.1007/s10162-013-0386-z
Lu, Z., and Popper, A. N. (1998). Morphological polarizations of sensory hair cells in the three otolithic organs of a teleost fish: Fluorescent imaging of ciliary bundles. Hear. Res. 126, 47–57. doi: 10.1016/S0378-5955(98)00149-X
Maklad, A., Reed, C., Johnson, N. S., and Fritzsch, B. (2014). Anatomy of the lamprey ear: morphological evidence for occurrence of horizontal semicircular ducts in the labyrinth of Petromyzon marinus. J. Anat. 224, 432–446. doi: 10.1111/joa.12159
Mickle, M. F., and Higgs, D. M. (2022). Towards a new understanding of elasmobranch hearing. Mar. Biol. 169, 1–13. doi: 10.1007/s00227-021-03996-8
Mickle, M. F., Miehls, S. M., Johnson, N. S., and Higgs, D. M. (2019). Hearing capabilities and behavioural response of sea lamprey (Petromyzon marinus) to low-frequency sounds. Can. J. Fish. Aquat. 76, 1541–1548. doi: 10.1139/cjfas-2018-0359
Myrberg, A. A. (2001). “The acoustical biology of elasmobranchs,” in The Behavior and Sensory Biology of Elasmobranch Fishes: An Anthology in Memory of Donald Richard Nelson. Developments in Environmental Biology of Fishes, eds T. C. Tricas and S. Gruber (Dordrecht: Springer), 31–45. doi: 10.1023/A:1007647021634
Parmentier, E., Banse, M., Boistel, R., Compère, P., Bertucci, F., and Colleye, O. (2020). The development of hearing abilities in the shark Scyliorhinus canicula. J. Anat. 237, 1–10. doi: 10.1111/joa.13212
Pastras, C. J., Stefani, S. P., Curthoys, I. S., et al. (2020). Utricular sensitivity during hydrodynamic displacements of the macula. JARO 21, 409–423. doi: 10.1007/s10162-020-00769-w
Platt, C. (1977). Hair cell distribution and orientation in goldfish otolith organs. J. Comp. Neurol. 172, 283–297. doi: 10.1002/cne.901720207
Platt, C., and Popper, A. N. (1981). “Fine structure and function of the ear,” in Hearing and Sound Communication in Fishes. Proceedings in Life Sciences, eds W. N. Tavolga, A. N. Popper, and R. R. Fay (New York, NY: Springer), 3–38. doi: 10.1007/978-1-4615-7186-5_1
Platt, C., and Straka, H. (2020). “Vestibular endorgans in vertebrates and adequate sensory stimuli,” in The Senses: A Comprehensive Reference (Second Edition), ed. F. Bernd (San Diego: Elsevier, Academic Press), 108–128. doi: 10.1016/B978-0-12-809324-5.24140-0
Popper, A. N. (1977). A scanning electron microscopic study of the sacculus and lagena in the ears of fifteen species of teleost fishes. J. Morphol. 153, 397–417. doi: 10.1002/jmor.1051530306
Popper, A. N., and Hoxter, B. (1984). Growth of a fish ear: 1. Quantitative analysis of hair cell and ganglion cell proliferation. Hear. Res. 15, 133–142. doi: 10.1016/0378-5955(84)90044-3
Popper, A. N., Rogers, P. H., Saidel, W. M., and Cox, M. (1988). “Role of the fish ear in sound processing,” in Sensory Biology of Aquatic Animals, eds J. Atema, R. R. Fay, A. N. Popper, and W. N. Tavolga (New York, NY: Springer), doi: 10.1007/978-1-4612-3714-3_27
Rogers, P. H., Popper, A. N., Hastings, M. C., and Saidel, W. M. (1988). Processing of acoustic signals in the auditory system of bony fish. J. Acoust. Soc. Am. 83, 338–349. doi: 10.1121/1.396444
Sauer, D. J., Yopak, K. E., and Radford, C. A. (2022). Ontogeny of the inner ear maculae in school sharks (Galeorhinus galeus). Hear. Res. 424:108600. doi: 10.1016/j.heares.2022.108600
Schulz-Mirbach, T., Ladich, F., Plath, M., and Heß, M. (2019). Enigmatic ear stones: what we know about the functional role and evolution of fish otoliths. Biol. Rev. 94, 457–482. doi: 10.1111/brv.12463
Tester, A., Kendall, J., and Milisen, W. (1972). Morphology of the ear of the shark genus carcharhinus, with particular reference to the macula neglecta. Pacific Sci. 26, 264–274.
Tricas, T. C. (2020). “Acoustic Ecology, Communication and Peripheral Signal Processing in Fishes,” in The Senses: A Comprehensive Reference (Second Edition), ed. B. Fritzsch (San Diego: Elsevier, Academic Press), 114–137. doi: 10.1016/B978-0-12-809324-5.24220-X
Vasconcelos, R. O., Alderks, P. W., and Sisneros, J. A. (2016). “Development of structure and sensitivity of the fish inner ear,” in Fish Hearing and Bioacoustics, ed. J. A. Sisneros (Cham: Springer), 291–318. doi: 10.1007/978-3-319-21059-9_14
Wang, J., Song, Q., Yu, D., Yang, G., Xia, L., Su, K., et al. (2015). Ontogenetic development of the auditory sensory organ in zebrafish (Danio rerio): Changes in hearing sensitivity and related morphology. Sci. Rep. 5, 1–15. doi: 10.1038/srep15943
Wersäll, J. (1956). Studies on the structure and innervation of the sensory epithelium of the cristae ampullaris in the guinea pig. Acta Otolaryngol. Suppl. 126, 1–85.
Keywords: elasmobranch, ontogeny, epithelia, macula, auditory
Citation: Sauer DJ, Yopak KE and Radford CA (2022) Ontogenetic development of inner ear hair cell organization in the New Zealand carpet shark Cephaloscyllium isabellum. Front. Ecol. Evol. 10:1034891. doi: 10.3389/fevo.2022.1034891
Received: 02 September 2022; Accepted: 11 November 2022;
Published: 24 November 2022.
Edited by:
Wayne Iwan Lee Davies, Umeå University, SwedenReviewed by:
Bernd Fritzsch, The University of Iowa, United StatesHans Straka, Ludwig Maximilian University of Munich, Germany
Copyright © 2022 Sauer, Yopak and Radford. This is an open-access article distributed under the terms of the Creative Commons Attribution License (CC BY). The use, distribution or reproduction in other forums is permitted, provided the original author(s) and the copyright owner(s) are credited and that the original publication in this journal is cited, in accordance with accepted academic practice. No use, distribution or reproduction is permitted which does not comply with these terms.
*Correspondence: Derek J. Sauer, ZHNhdTcyOUBhdWNrbGFuZHVuaS5hYy5ueg==