- 1Programa de Pós Graduação em Zoologia, Departamento de Ciências Biológicas, Universidade Estadual de Santa Cruz, Ilhéus, Bahia, Brazil
- 2Tropical Herpetology Lab, Departamento de Ciências Biológicas, Universidade Estadual de Santa Cruz, Ilhéus, Bahia, Brazil
- 3Laboratório de Bioinformática e Biologia Evolutiva, Departamento de Genética, Universidade Federal de Pernambuco, Recife, Pernambuco, Brazil
- 4Herpetology Section, Zoologisches Forschungsmuseum Alexander Koenig, Bonn, Germany
Realistic predictions about the impacts of climate change onbiodiversity requires gathering ecophysiological data and the critical thermal maxima (CTMax) is the most frequently used index to assess the thermal vulnerability of species. In the present study, we performed a systematic review to understand how acclimation and altitude affect CTMax estimates for amphibian and non-avian reptile species. We retrieved CTMax data for anurans, salamanders, lizards, snakes, and turtles/terrapins. Data allowed to perform a multilevel random effects meta-analysis to answer how acclimation temperature affect CTMax of Anura, Caudata, and Squamata and also meta-regressions to assess the influence of altitude on CTMax of frogs and lizards. Acclimation temperature influenced CTMax estimates of tadpoles, adult anurans, salamanders, and lizards, but not of froglets. In general, the increase in acclimation temperature led to higher CTMax values. Altitudinal bioclimatic gradient had an inverse effect for estimating the CTMax of lizards and anuran amphibians. For lizards, CTMax was positively influenced by the mean temperature of the wettest quarter. For anurans, the relationship is inverse; we recover a trend of decreasing CTMax when max temperature of warmest month and precipitation seasonality increase. There is an urgent need for studies to investigate the thermal tolerance of subsampled groups or even for which we do not have any information such as Gymnophiona, Serpentes, Amphisbaena, and Testudines. Broader phylogenetic coverage is mandatory for more accurate analyses of macroecological and evolutionary patterns for thermal tolerance indices as CTMax.
Introduction
Amid the urgency of the global climate crisis, the volume of data on thermal ecology and physiology is increasing substantially (Bennett et al., 2018; Díaz-Ricaurte et al., 2022). Animal ecophysiology has been a hot topic in ecology and evolutionary biology, with scientists around the world trying to understand the impacts of rising temperatures on species survival (e.g., Sinervo et al., 2010; Hoffmann et al., 2013; Nowakowski et al., 2017; Diele-Viegas et al., 2020). In this scenario, understanding thermal traits, their natural history, and interaction with habitat conditions is paramount to forecast the consequences of climate change for species worldwide (Duarte et al., 2012; Nowakowski et al., 2018; Bennett et al., 2019; Carilo Filho et al., 2021; Turriago et al., 2022). The need to standardize experimental protocols has been a major issue in this area, especially when the comparison among several datasets is essential to understand patterns of thermal vulnerability in taxa (Lutterschmidt and Hutchison, 1997a,b; Taylor et al., 2021). There are a multitude of methods, variables and experimental protocols in use (see commentaries in Supplementary material in Sunday et al., 2014).
In this sense, the critical thermal maximum (CTMax) is the most employed thermal index among studies involving ectotherms (Duarte et al., 2012; Gutiérrez-Pesquera et al., 2016; Bennett et al., 2018; Leiva et al., 2019). A good proxy for upper thermal limits, CTMax is commonly measured through complete inactivity or muscular spasms of the individuals–the most consensual experimental end points. As other ecophysiological indexes, CTMax can be influenced by several factors such as natural history (Carilo Filho et al., 2021), microclimate (Duarte et al., 2012; Gutiérrez-Pesquera et al., 2016), ontogeny (Floyd, 1983; Bodensteiner et al., 2021), and phylogenetic relatedness (Duarte et al., 2012; Gutiérrez-Pesquera et al., 2016). In addition, characteristics related to the experimental design and methodological procedures can also affect the estimation of CTMax (e.g., acclimation, photoperiod, heating and ramping rates–see Hutchison and Kosh, 1965; Brattstrom, 1968; Mahoney and Hutchison, 1969; Rezende et al., 2011; Ribeiro et al., 2012; Simon et al., 2015).
Ecophysiological characteristics can be fixed in evolutionary history among families and orders (Clusella-Trullas and Chown, 2014; Gutiérrez-Pesquera et al., 2016; Leiva et al., 2019). However, within a given family or genus, the evolution of CTMax seems to be more related to the microhabitat occupied by species themselves, as suggested by literature for amphibians and lizards (Duarte et al., 2012; Gunderson et al., 2018; Carilo Filho et al., 2021). In amphibian's ontogeny, CTMax increases throughout larval development and usually decreases during the metamorphic climax–morphophysiological transition that occurs between the larval and adult stages (Floyd, 1983; Ruthsatz et al., 2022). Once the metamorphosis phase is over, the thermal tolerance values tend to increase again (Bodensteiner et al., 2021; Ruthsatz et al., 2022) but there are evidences that larval forms tend to have a CTMax greater than the adults for tropical species from Atlantic Forest (Carilo Filho et al., 2021). If the data in amphibians are not yet conclusive, in squamate reptiles the pattern is even less consistent (see Bodensteiner et al., 2021).
In thermal ecophysiology, acclimation is used as a methodological strategy to induce the adjustment of specimens to a given temperature (Brattstrom, 1968; Navas et al., 2008). Some studies use the thermal average and photoperiod of the collection sites to base the acclimation conditions of the specimens (e.g., Gutiérrez-Pesquera et al., 2016; Carilo Filho et al., 2021). In general, the acclimation temperature is directly proportional to the CTMax of the ectothermic vertebrate species (Navas et al., 2008; Clusella-Trullas and Chown, 2014; Campos et al., 2021). In reptiles the same trend is maintained with projections that each 1°C of acclimation corresponds to a 0.1°C increase in CTMax (Clusella-Trullas and Chown, 2014). For anurans, the effect of acclimation on thermal tolerance, although following the pattern discussed above, appears to be influenced by evolutionary history at the family level (Navas et al., 2008).
Altitudinal stratification drives diversification by creating a natural gradient of vegetation, temperature, and humidity (Cadena et al., 2012). In this sense, tropical species tend to occupy fewer bands of this gradient with less overlap in their distribution compared to temperate species (Cadena et al., 2012), with a similar pattern being found for thermal tolerance of a frog community in the Andes (von May et al., 2017). Many studies have tested intraspecific differences in thermal biology in lizards (GvoŽdík and Castilla, 2001; Muñoz et al., 2014; Belasen et al., 2017) and anuran amphibians (Christian et al., 1988; Zheng and Liu, 2010; Pintanel et al., 2019). Intraspecifically, no influence of altitudinal zonation on CTMax in amphibians and reptiles is yet recognizable. Studies tend to address these issues based on observations from a single species in many cases. In this sense, integrating the results of several researches in a meta-analytical panorama helps to investigate more broadly the maintenance or not of certain patterns such as acclimation and altitudinal climatic variation for thermal tolerance.
Herein, we evaluated the influence of acclimation temperature and the influence of altitudinal climate variation on the CTMax of amphibians and reptiles using metadata and bioclimatic variables to explore patterns and trends in both groups. Thus, we aim to determine whether: (i) individuals of amphibians and non-avian reptiles acclimated to higher temperatures tend to have higher CTMax than those acclimated at lower temperatures (sensu Brattstrom, 1968; Navas et al., 2008; Simon et al., 2015) and (ii) if altitudinal bioclimatic gradients constrain CTMax. Thus, amphibians and reptiles populations at cooler/wetter/higher locations have lower CTMax values than populations closer to sea level, or does CTMax not vary between different populations as it is phylogenetically conserved (Bennett et al., 2021).
Methods
Dataset
We performed a systematic review by searching for studies that evaluated the influence of acclimation and altitude on critical thermal maxima (CTMax) of amphibians and reptiles following the Preferred Reporting Items for Systematic reviews Meta-Analyses (PRISMA) protocol (Page et al., 2021). We searched for articles in the Scopus and Web of Science databases using the following words: (“thermal extremes” OR “thermal performance curve” OR “thermal safety margins” OR “thermal tolerance” OR “upper thermal limit” OR “CTMax” OR “cold tolerance” OR “lower thermal limit” OR “CTmin”) AND amphib* AND reptil*. These words were searched for in titles, abstracts and keywords in the articles published until August 2021. We present the number of records returned by the bibliographic search, and also sequential procedures of including/excluding the studies, on the PRISMA flow chart (Figure 1) (Page et al., 2021).
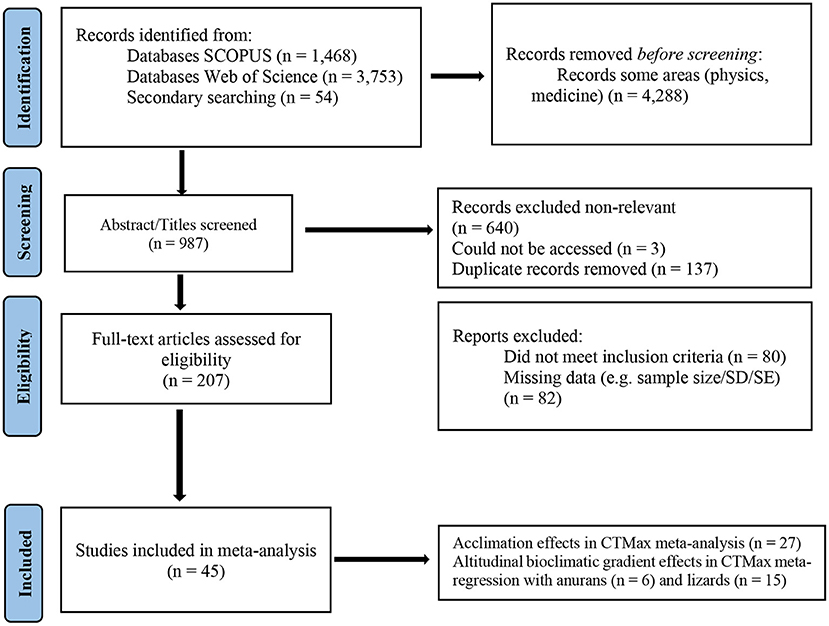
Figure 1. PRISMA flow diagram adapted from Page et al. (2021) showing literature search procedures and screening processes.
Inclusion criteria
We retrieved studies with amphibian and non-avian reptile species that presented estimates of mean, standard deviation/error, sample size and endpoint used for CTMax data. Studies with larval amphibians were included when larval stage was identified sensu the Gosner table (Gosner, 1960). We selected studies which compare CTMax estimates from conspecific individuals submitted to (a) different acclimation temperatures and (b) from different altitudes. Hence, within each study, we compare the CTMax from individuals exposed to lower temperature of acclimation with CTMax of conspecifics submitted to higher acclimation temperature (using the lowest acclimation temperature as a control). Similarly, we compare conspecific individuals collected at different altitudes but submitted to identical experimental procedures (e.g., same heating rates and acclimation temperature). To compile our database for each study, we extracted the number of individuals evaluated (sample size), the mean CTMax value, and standard deviation (original or converted from standard error) from each treatment. When information was only available in graphics, we extracted data using the (Get Data Graph Digitizer, 2021) program (www.getdata-graph-digitizer.com).
Phylogenetic non-independency control
We used phylogenetic information from the Tree of Life Web Project (http://tolweb.org/tree/) through the package rotl- version 3.0.14 (Michonneau et al., 2016), retaining in the topology only for the species included in our research (Supplementary Figure 1). We controlled for non-independence arising from the evolutionary history shared by the species, including phylogenetic covariance (i.e., using branch lengths) obtained from a phylogenetic correlation matrix, as a random variable within models (more details in next sections) (Adams, 2008). The branch lengths were calculated following Grafen (1989). Both the branch lengths and the correlation matrix of phylogenetic relatedness were estimated using the package ape–version 5.6-2 (Paradis and Schliep, 2019).
Analysis
We used Hedges'g equation to estimate the effect size from the data obtained from the studies, using the package metafor–version 3.8-1 (Viechtbauer, 2010). Considering that we retrieved estimates of effect size from different studies–with variable sample size, we used a random effect meta-analysis using a multilevel meta-analytical model to study whether different acclimation temperatures alter CTMax estimates in amphibians and reptiles. We controlled non-independence among effect sizes by using species and the phylogenetic relatedness as random variables (Nakagawa and Santos, 2012; Nakagawa et al., 2017) (Supplementary Figure 1). By using the species as a random variable, we also controlled for the influence of several studies for the same species while estimating the effect size. We built the model using function rma.mv and adjusted the models through the restricted maximum likelihood (REML) relating CTMax (response variable), temperature of acclimation and taxonomic groups (predictors). We organized the retrieved data in subgroups representing the upper taxonomic categories (e.g., Anura, Caudata, and Squamata–in our case lizards, excluding others groups phylogenetically recognized as Squamata e.g., amphisbaenians). Serpentes and Testudines were not included in the analyses due to the low sample size. Considering that most anuran species present biphasic lives (i.e., larvae stages mostly aquatic and adult's predominant terrestrial), we divided the anuran data into three subgroups according to the ontogenetic stage and following Gosner (1960): (a) tadpoles (pre-metamorphic stages), for stages until (<Gosner 42); (b) Froglets (≥ Gosner 42 to juveniles; adapted from Ruthsatz et al., 2022); and (c) adults (according with the retrieved information from studies). Lastly, we extracted the mean value of effect size (yi) and variance (xi) associated for each subgroup.
In a meta-regression we use a numerical explanatory variable that allows us to plot a regression graph, while in a conventional meta-analysis we use a forest plot based on categories or groups under analysis (Noble et al., 2017). We also performed separated meta-regressions for anurans and lizard species (as was done in the previous analysis) to test the influence of altitudinal climatic gradient on CTMax following a similar approach to those described above (i.e., species and phylogenetic relatedness were taken in account during analysis as moderators–Supplementary Figures 2A,B). Additionally, we used the different altitudes to obtain bioclimatic data that were used as models' moderators to verify the effect size variation of CTMax through climate gradient resulting of altitudinal variation. We extracted values from each occurrence point in the data gathered for the 19 bioclimatic variables (Bio 1 to Bio 19) in WorldClim (https://www.worldclim.org/)–version 2.1 (Fick and Hijmans, 2017), using point sample tools available in Qgis software–version 3.26.3 (QGIS Development Team, 2020). To avoid collinearity between the bioclimatic data, we retrieve variables based on a cut-off value of 0.75 for the Pearson correlation index. For the meta-regressions we used only data of the adults of anurans and lizard species, and from the variables selected after the collinearity test, we used those that made biological sense for both animal's groups. We selected best meta-regressions models based on Akaike information criteria (AIC), Bayesian information criteria (BIC) and Akaike information criteria for small samples (AICc). We used the package metafor implemented in the R environment in all analytical procedures (R Core Team, 2021) considering a significance value of α = 0.05.
Publication bias
We used the Egger regression to test for publication bias by controlling the random effect of the random variables and using species variance as moderators (Egger et al., 1997). We considered the data asymmetric, and thus with potential publication bias, when the intercept of the Egger regression significantly (i.e., p < 0.1) deviated from zero (Egger et al., 1997; Sterne and Egger, 2006).
Results
We retrieved 46 published studies that met the inclusion criteria (Figure 1). Studies were conducted between 1955 and 2020 in 18 countries. We analyzed 27 and 21 studies, respectively for the influence of acclimation and altitudinal climate variation on CTMax, with three studies providing data for both analyses. Data retrieved encompassed anurans, salamanders, lizards, snakes and freshwater turtles/terrapins (Supplementary Table 1). Therefore, we assembled data from 30 species including 96 effect sizes measured for acclimation analysis and 32 species with 120 values of effect sizes for the climate gradient meta-regression (Supplementary Tables 2–4). We did not include snake and turtle data in the meta-analysis due to their very low sample size–only one study with a single species for each group. We retrieved Bio 4, Bio 5, Bio 9, Bio 12 e Bio 15 for meta-regressions with anurans and Bio 3, Bio 8, Bio 10, Bio 12 and Bio 15 for analysis with lizard's species.
Meta-analysis on acclimation influence on CTMax
The Egger' regressions revealed no bias in the acclimation meta-analysis (intercept = −0.10; C.I. = −0.35–0.15; p = 0.44) and we recovered a high level of heterogeneity among studies (I2 = 82.4%). On the acclimation-CTMax meta-analysis, we retrieved a statistically relevant effect for lizards (Effect Size = 1.69; p = 0.001), salamanders (Effect Size = 1.68; p = < 0.0001), tadpoles (Effect Size = 2.72; p = < 0.0001) and adults of anurans (Effect Size = 1.34; p = < 0.0001) (Figure 2; Supplementary Table 5). Therefore, the increase in the acclimation temperature to lead to an increase in the CTMax values in all evaluated groups with exception of froglets (Figure 2).
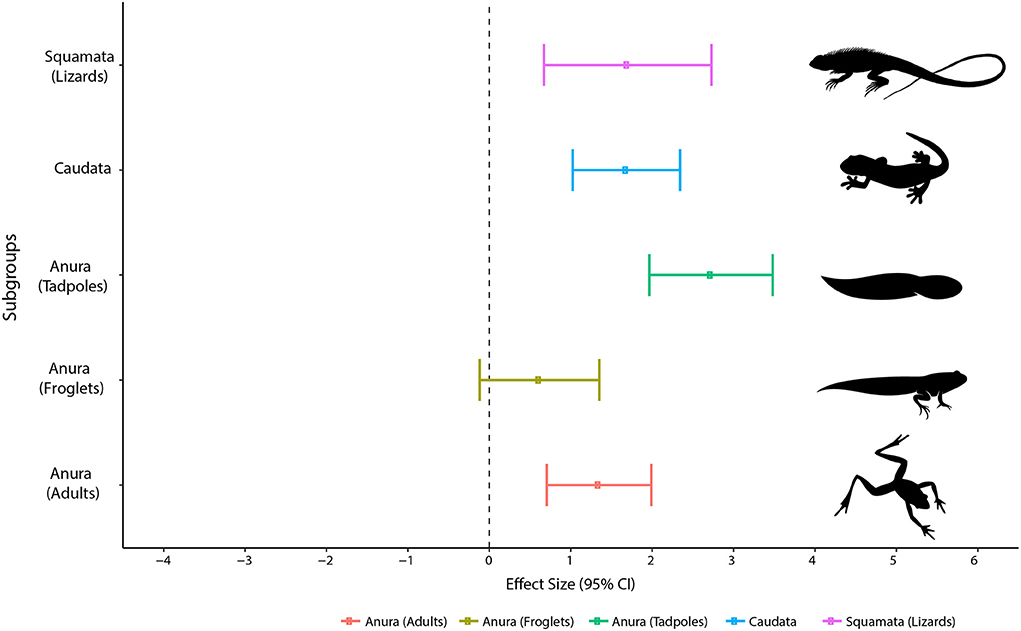
Figure 2. Effect size values measuring the influence of acclimation on the CTMax of Squamata (Lizards), Caudata and Anura (Tadpoles; Froglets; and Adults).
Meta-regressions on the influence of altitudinal climate gradient on CTMax
The analysis again revealed no apparent bias in the studies involving anurans (Supplementary Table 8) and lizards (Supplementary Table 9). Our meta-regressions revealed that bioclimatic gradient created by altitude may influence differently in CTMax values depending of the taxonomic group studied (Figure 3; Supplementary Table 6). In anurans we observe an increase in the CTMax as the Temperature Seasonality (Bio 4) and Annual Precipitation (Bio 12) values increase (Figures 3A,D; Supplementary Table 6). Otherwise, the higher the Max Temperature of Warmest Month (Bio 5) and Precipitation Seasonality (Bio 15) values, the lower the CTMax values (Figures 3B,E; Supplementary Table 6). We did not recover significant effect for Mean Temperature of Driest Quarter (Bio 9, Supplementary Table 6). Bio 5 and Bio 15 were the best supported by model selection criteria (Supplementary Table 10), indicating that the altitudinal climatic gradient promotes a decreasing in the CTMax of anurans.
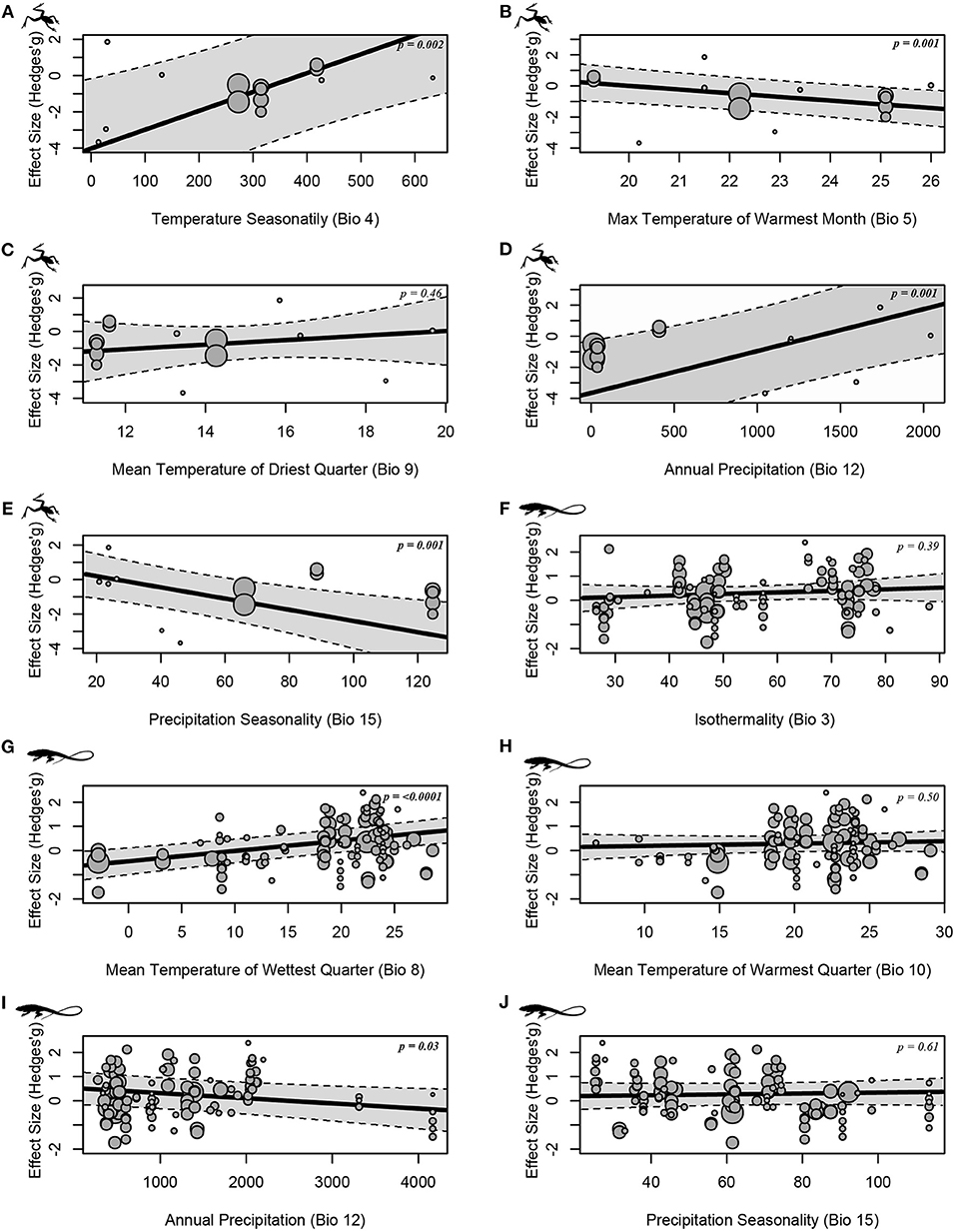
Figure 3. Effect size on the influence of altitudinal climatic variation to the CTMax in anurans (A–E) and lizards (F–J). Circle size denotes the precision of each estimate–larger circles representing more accurate estimates. The P-value (p) from each analysis is available inside the charts.
In lizard species CTMax increases with Mean Temperature of Wettest Quarter (Bio 8) and decreases with higher values of Annual Precipitation (Bio 12) (Figures 3D,G). Between Bio 8 and Bio 12, we selected the mean temperature of wettest quarter by model selection criteria (Supplementary Table 11). In this sense, high altitude tends to promote high values in the CTMax of lizards. We found no effect of Isothermality (Bio 3), Mean Temperature of Warmest Quarter (Bio 10) and Precipitation Seasonality (Bio 15) for lizards CTMax (Figures 3F,H,J; Supplementary Table 7).
Discussion
Here we evaluated the influence of the acclimation of reptiles and amphibians as well as the effect of altitude on the upper limits of these groups using meta-analytical procedures. Overall, our data indicated that non-avian reptiles and amphibians are capable of a certain physiological plasticity with respect to increasing temperature (Figure 2). These patterns previously raised by systematic reviews (e.g., Navas et al., 2008; Clusella-Trullas and Chown, 2014) were now retrieved using meta-analytic models. Our analysis also indicated that thermal gradient created by altitudinal variation has influence on the CTMax of the species studied in both groups evaluated, but with inverse patterns for anurans and lizards depending of bioclimatic variable in evidence (Figure 3). The pattern found for lizards reveals that mean temperature of the wettest quarter leads to high values on the upper thermal limits whereas warm associated precipitation seasonality constrains the CTMax of anurans in populations of high areas. In both groups, humidity plays an important role in the CTMax.
Acclimation
The acclimation potential of species is variable and directly related to the interaction of cellular, environmental and behavioral mechanisms (Huey and Slatkin, 1976; Huey, 1991; Huey and Kingsolver, 1993). Our data point to a trend towards an increase in CTMax of the species as the acclimation temperature increases. Although we obtained significant results for pre-metamorphic and adult amphibians, we did not observe the same pattern in froglets. However, it is plausible that the trend will be confirmed to this group as well, as the number of studies evaluating the ontogenetic responses of the anuran's species to acclimation increases.
In our systematic review, we noticed that the studies that evaluate the acclimation capacity in reptile and amphibian species are taxonomically concentrated. Only 14 amphibian families (Anura−8 families; Caudata−6 families) and two Squamate reptile families (i.e., Dactyloidae and Phrynosomatidae) are represented. Information regarding the influence of acclimation on the CTMax of aquatic ectothermic vertebrates (e.g., Osteichthyes) is more abundant in the literature (e.g., Comte and Olden, 2017; Campos et al., 2021) than for terrestrial taxa. Data in this sense are scarce for entire groups such as Serpentes and Testudines–(Scott et al., 1982; Xu et al., 2015) or even non-existent for taxonomic groups as Gymnophiona, Crocodylia, Sphenodontidae and Amphisbaenia–we did not retrieve studies for these groups in our research. Even for amphibians, the most studied group of terrestrial vertebrates regarding the relationship between CTMax and acclimation, data for the ontogenetic stages are still inconclusive as they are available for a reduced number of species and are mostly spatially concentrated for species from temperate zones (see Supplementary Table 1).
Thermal biology indexes may have different responses to acclimation even in closely related species as demonstrated by Ryan and Gunderson (2021) regarding the thermal preferences of two species of Anolis. There is a growing effort to fill these and other knowledge gaps in thermal biology especially for amphibians (Telemeco and Gangloff, 2021 and papers therein). As an example, Ruthsatz et al. (2022) analyzed the links of the thermal tolerance and acclimation capacity in Rana temporaria ontogeny. The pattern recovered by our analyses points in a similar way that those presented by Ruthsatz et al. (2022). Tadpoles showed a greater effect of acclimation on CTMax than the froglets, possibly showing a greater plasticity in acclimation in the stages that precede metamorphosis (Ruthsatz et al., 2022). Our analysis recovers plasticity in acclimation for adults, a result that does not coincide with those found by Ruthsatz et al. (2022). This fact may be related to the scarcity of studies evaluating this issue in amphibian adults, or even suggests that acclimation effects may vary interspecifically.
Species share an evolutionary history to a greater or lesser extent, therefore, more directly related species are generally expected to have similar aspects of their thermal physiology, including their thermal tolerance (Gutiérrez-Pesquera et al., 2016). Although we used phylogenetic data to account for the non-independence in the ecological species data, the number of studies that attended to our inclusion criteria were not suitable (n < 20) to make accurate considerations about the acclimation capacity and its influence on the CTMax of the species through an evolutionary approach (Garamszegi, 2014). Nevertheless, ectotherms vertebrates seem to demonstrate a phylogenetic conservatism tendency to exhibit higher CTMax values when exposed to upper acclimation temperatures (Losos, 2008). However, thermal plasticity appears to be insufficient to deal with temperature arise resulting from global climate change (Gunderson et al., 2017; Morley et al., 2019), as environmental temperatures are expected to increase between 2.6 and 5.0°C depending on location and warming scenario (IPCC, 2022). In this sense, we highlight the necessity of studies that estimate acclimation effects and that encompass transparency on methodological procedures (Taylor et al., 2021).
It is expected that the acclimation capacity of species from different biogeographic regions of the globe as well as belonging to different macro- and microhabitats show differences related to specific environmental filters and the influence of thermal niche partition with other species (Carilo Filho et al., 2021; Ryan and Gunderson, 2021). In the midst of an escalation in greenhouse gas emissions, it is essential to understand the extent to which biogeographic, evolutionary and ecological factors interact with the ability of species to acclimate to increasingly higher temperatures as a strategy to predict possible impacts generated by climate change (Lara-Reséndiz et al., 2021; Longhini et al., 2021). The effort to understand such interactions is even more urgent when we recognize that plasticity at thermal limits appears to be restricted in terrestrial ectotherms (Gunderson and Stillman, 2015).
Altitude
The decreasing in the CTMax of amphibians at higher altitudes may result from a physiological investment to support cooler rather than upper temperatures. Lower temperatures throughout evolutionary history possibly selected populations with lower CTMin to the detriment of physiological mechanisms that would eventually influence greater heat tolerance (i.e., higher CTMax), a similar pattern is already simulated for lizards and attested for and frog species (Telemeco et al., 2017; González-del-Pliego et al., 2020). Thus, these species would experience a conservation of their upper thermal limits and an adaptive selection of the lower limits of thermal tolerance (Losos, 2008). In lizards, altitude provided humidity conditions that had a significant and a positive effect on CTMax which can be attributed to several factors, as intraspecific variations, thermoregulation strategies or even the presence of selective pressure (Sunday et al., 2014; Telemeco et al., 2017; Herrando-Pérez et al., 2020). On the other hand, studies with species from altitudes above 3.000 m are underrepresented in the literature and in our analyses, which may denote a research gap or even the scarcity of species that inhabit these areas.
The effects of altitude on lizard's ecophysiology are possibly driven by the climatic gradient and provide a selective force for their CTMax. In this scenario, their survival in case of exposure to extreme temperatures would be directly related to their ability to actively thermoregulate (e.g., heliothermic species) or through the search for thermal refuges within their tolerance range (e.g., thermoconforming) (Sunday et al., 2014). However, thermoregulatory behavior may have a limited role in mitigating the effects of selective pressure from warmer habitats.
We found that higher temperatures associated with precipitation seasonality may constrain CTMax in anurans, leading to a thermal and evaporative stress. In Andean frogs of the genus Pristimantis the CTMax is directly dependent of the landscapes they occupy. Species that live in thermally variable habitat presented higher CTMax values than congenerics living in forests. Otherwise, forest-dweller species show lower CTMin indexes, suggesting in both case the occurrence of thermal selection by the environment (Pintanel et al., 2019). Macroecological analyses for ectotherms indicate that upper thermal limits have slower evolution rates than the lower limits, evidence that the upper limits tend to be phylogenetically conserved during evolutionary process (Araújo et al., 2013; Qu and Wiens, 2020; Bennett et al., 2021).
Conclusion
We confirm by a straightforward meta-analytical approach that reptiles and amphibians tend to have higher CTMax values with higher acclimation temperatures. Our study showed that many vertebrate groups remain underrepresented in the literature and that it is imperative to study the response of several lineages in terms of thermal patterns and indices. Despite their ecological importance for terrestrial ecosystems, reptiles and amphibians still show many knowledge gaps concerning their ecophysiology. Such panorama is even more clear for species that inhabit tropical regions and higher altitude areas worldwide (Winter et al., 2016; Carilo Filho et al., 2021; White et al., 2021). Notwithstanding, our evidence for acclimation capacity on squamate reptiles is based on a dataset composed solely by CTMax data of lizard species. Therefore, it is still premature to generalize our results to groups so divergent ecologically as snakes and amphisbaenians. The situation in amphibians is even more delicate, as the group of terrestrial vertebrates most threatened with extinction. The fact that most species show a biphasic life cycle makes it necessary to understand how adults and immature forms deal with the warming of their environments through the study of their thermal tolerance and their acclimation capacity. Our data is consistent with this assumption given we attest ontogenetic differences in acclimation capacity for anurans. In anurans at high elevations, max temperature of the warmest periods and precipitation seasonality decreasing CTMax. Thus, species on higher altitudinal quotes may be particularly vulnerable amid climate change.
In addition to the scarcity of data to assess acclimation or climatic altitudinal gradient, the existence of numerous experimental protocols hampers comparisons, as they introduce noise from the plurality of methods employed and intrinsic and extrinsic sources of bias in the analysis (see Taylor et al., 2021; Clusella-Trullas et al., 2021). One of the main challenges encountered for comprehensive ecophysiological studies is to reduce the influence of methodological bias while expanding biological sampling to obtain answers to macroecological and evolutionary questions (White et al., 2021). Once corrected to reduce the discrepancy in their measurement, even data obtained from different acclimation temperatures in different species may in the future provide a broader phylogenetic coverage in order to allow more sensitive analyses of macroecological and evolutionary patterns for traits of thermal biology.
Data availability statement
The original contributions presented in the study are included in the article/Supplementary material, further inquiries can be directed to the corresponding author.
Author contributions
LC contributed to conception, design of the study, and organized the database. LC and LG performed the statistical analysis and wrote the first draft of the manuscript. LC, LG, MK, MS, and VO wrote sections of the manuscript. All authors contributed to manuscript review, read, and approved the submitted version.
Acknowledgments
LC thanks the Fundação de Amparo à Pesquisa do Estado da Bahia (FAPESB) for a scholarship (BOL0418/2019). MS and VO acknowledge the Brazilian Council for Scientific and Technological Development (CNPq) for the productivity research grants (309365/2019-8 and 310467/2017-9, respectively).
Conflict of interest
The authors declare that the research was conducted in the absence of any commercial or financial relationships that could be construed as a potential conflict of interest.
Publisher's note
All claims expressed in this article are solely those of the authors and do not necessarily represent those of their affiliated organizations, or those of the publisher, the editors and the reviewers. Any product that may be evaluated in this article, or claim that may be made by its manufacturer, is not guaranteed or endorsed by the publisher.
Supplementary material
The Supplementary Material for this article can be found online at: https://www.frontiersin.org/articles/10.3389/fevo.2022.1017255/full#supplementary-material
References
Adams, D. C. (2008). Phylogenetic meta-analysis. Evolution 62, 567–572. doi: 10.1111/j.1558-5646.2007.00314.x
Araújo, M. B., Ferri-Yáñez, F., Bozinovic, F., Marquet, P. A., Valladares, F., and Chown, S. L. (2013). Heat freezes niche evolution. Ecol. Lett. 16, 1206–1219. doi: 10.1111/ele.12155
Belasen, A., Brock, K., Li, B., Chremou, D., Valakos, E., Pafilis, P., et al. (2017). Fine with heat, problems with water: microclimate alters water loss in a thermally adapted insular lizard. Oikos 126, 447–457. doi: 10.1111/oik.03712
Bennett, J. M., Calosi, P., Clusella-Trullas, S., Martínez, B., Sunday, J., Algar, A. C., et al. (2018). GlobTherm, a global database on thermal tolerances for aquatic and terrestrial organisms. Sci. Data 5, 1–7. doi: 10.1038/sdata.2018.22
Bennett, J. M., Sunday, J., Calosi, P., Villalobos, F., Martínez, B., Molina-Venegas, R., et al. (2021). The evolution of critical thermal limits of life on Earth. Nature Comm. 12, 1–9. doi: 10.1038/s41467-021-21263-8
Bennett, S., Duarte, C. M., Marbà, N., and Wernberg, T. (2019). Integrating within-species variation in thermal physiology into climate change ecology. Philos. Trans. R. Soc. B. Biol. Sci. 374, 20180550. doi: 10.1098/rstb.2018.0550
Bodensteiner, B. L., Agudelo-Cantero, G. A., Arietta, A. A., Gunderson, A. R., Muñoz, M. M., Refsnider, J. M., et al. (2021). Thermal adaptation revisited: how conserved are thermal traits of reptiles and amphibians? J. Exp. Zool. Part A. Ecol. Integr. Physiol. 335, 173–194. doi: 10.1002/jez.2414
Brattstrom, B. H. (1968). Thermal acclimation in anuran amphibians as a function of latitude and altitude. Comp. Bioch. Physiol. 24, 93–111. doi: 10.1016/0010-406X(68)90961-4
Cadena, C. D., Kozak, K. H., Gomez, J. P., Parra, J. L., McCain, C. M., Bowie, R. C., et al. (2012). Latitude, elevational climatic zonation and speciation in New World vertebrates. Proc. R. Soc. B Biol. Sci. 279, 194–201. doi: 10.1098/rspb.2011.0720
Campos, D. F., Amanajás, R. D., Almeida-Val, V. M., and Val, A. L. (2021). Climate vulnerability of South American freshwater fish: thermal tolerance and acclimation. J. Exp. Zool. Part A. Ecol. Integr. Physiol. 335, 723–734. doi: 10.1002/jez.2452
Carilo Filho, L. M., de Carvalho, B. T., Azevedo, B. K., Gutiérrez-Pesquera, L. M., Mira-Mendes, C. V., Solé, M., et al. (2021). Natural history predicts patterns of thermal vulnerability in amphibians from the Atlantic rainforest of Brazil. Ecol. Evol. 11, 16462–16472. doi: 10.1002/ece3.7961
Christian, K. A., Nunez, F., Clos, L., and Diaz, L. (1988). Thermal relations of some tropical frogs along an altitudinal gradient. Biotropica 236–239. doi: 10.2307/2388239
Clusella-Trullas, S., and Chown, S. L. (2014). Lizard thermal trait variation at multiple scales: a review. J. Comp. Physiol. B. Biochem. Syst. Environ. Physiol. 184, 5–21. doi: 10.1007/s00360-013-0776-x
Clusella-Trullas, S., Garcia, R. A., Terblanche, J. S., and Hoffmann, A. A. (2021). How useful are thermal vulnerability indices? Trends Ecol. Evol. 36, 1000–1010. doi: 10.1016/j.tree.2021.07.001
Comte, L., and Olden, J. D. (2017). Evolutionary and environmental determinants of freshwater fish thermal tolerance and plasticity. Glob. Chang. Biol. 23, 728–736. doi: 10.1111/gcb.13427
Díaz-Ricaurte, J. C., Serrano, F. C., and Martins, M. (2022). VTMaxHerp: a data set of voluntary thermal maximum temperatures of amphibians and reptiles from two Brazilian hotspots. Ecology 103, e3602. doi: 10.1002/ecy.3602
Diele-Viegas, L. M., Figueroa, R. T., Vilela, B., and Rocha, C. F. D. (2020). Are reptiles toast? A worldwide evaluation of Lepidosauria vulnerability to climate change. Climat. Chang. 159, 581–599. doi: 10.1007/s10584-020-02687-5
Duarte, H., Tejedo, M., Katzenberger, M., Marangoni, F., Baldo, D., Beltrán, J. F., et al. (2012). Can amphibians take the heat? Vulnerability to climate warming in subtropical and temperate larval amphibian communities. Glob. Chang. Biol. 18, 412–421. doi: 10.1111/j.1365-2486.2011.02518.x
Egger, M., Smith, G. D., Schneider, M., and Minder, C. (1997). Bias in meta-analysis detected by a simple, graphical test. Br. Med. J. 315, 629–634. doi: 10.1136/bmj.315.7109.629
Fick, S. E., and Hijmans, R. J. (2017). WorldClim 2: new 1km spatial resolution climate surfaces for global land areas. Inter. J. Climatol. 37, 4302–4315. doi: 10.1002/joc.5086
Floyd, R. B. (1983). Ontogenetic change in the temperature tolerance of larval Bufo marinus (Anura: Bufonidae). Comp. Bioch. Physiol. Part A. Physiol. 75, 267–271. doi: 10.1016/0300-9629(83)90081-6
Garamszegi, L. Z. (2014). Modern Phylogenetic Comparative Methods and Their Application in Evolutionary Biology. Springer. p. 3–552. doi: 10.1007/978-3-662-43550-2
Get Data Graph Digitizer (2021). Available online at: www.getdata-graph-digitizer.com (accessed October, 2022).
González-del-Pliego, P., Scheffers, B. R., Freckleton, R. P., Basham, E. W., Araújo, M. B., Acosta-Galvis, A. R., et al. (2020). Thermal tolerance and the importance of microhabitats for Andean frogs in the context of land use and climate change. J. Anim. Ecol. 89, 2451–2460. doi: 10.1111/1365-2656.13309
Gosner, K. L. (1960). A simplified table for staging anuran embryos and larvae with notes on identification. Herpetologica 16, 183–190.
Grafen, A. (1989). The phylogenetic regression. Philos. Trans. R. Soc. B. Biol. Sci. 326, 119–157. doi: 10.1098/rstb.1989.0106
Gunderson, A. R., Dillon, M. E., and Stillman, J. H. (2017). Estimating the benefits of plasticity in ectotherm heat tolerance under natural thermal variability. Funct. Ecol. 31, 1529–1539. doi: 10.1111/1365-2435.12874
Gunderson, A. R., Mahler, D. L., and Leal, M. (2018). Thermal niche evolution across replicated Anolis lizard adaptive radiations. Proc. R. Soc. B. Biol. Sci. 285, 20172241. doi: 10.1098/rspb.2017.2241
Gunderson, A. R., and Stillman, J. H. (2015). Plasticity in thermal tolerance has limited potential to buffer ectotherms from global warming. Proc. R. Soc. B: Biol. Sci. 282, 20150401. doi: 10.1098/rspb.2015.0401
Gutiérrez-Pesquera, L. M., Tejedo, M., Olalla-Tárraga, M. Á., Duarte, H., Nicieza, A., and Solé, M. (2016). Testing the climate variability hypothesis in thermal tolerance limits of tropical and temperate tadpoles. J. Biogeog. 43, 1166–1178. doi: 10.1111/jbi.12700
GvoŽdík, L., and Castilla, A. M. (2001). A comparative study of preferred body temperatures and critical thermal tolerance limits among populations of Zootoca vivipara (Squamata: Lacertidae) along an altitudinal gradient. J. Herpetol. 35, 486–492. doi: 10.2307/1565967
Herrando-Pérez, S., Monasterio, C., Beukema, W., Gomes, V., Ferri-Yáñez, F., Vieites, D. R., et al. (2020). Heat tolerance is more variable than cold tolerance across species of Iberian lizards after controlling for intraspecific variation. Funct. Ecol. 34, 631–645. doi: 10.1111/1365-2435.13507
Hoffmann, A. A., Chown, S. L., and Clusella-Trullas, S. (2013). Upper thermal limits in terrestrial ectotherms: how constrained are they? Funct. Ecol. 27, 934–949. doi: 10.1111/j.1365-2435.2012.02036.x
Huey, R. B. (1991). Physiological consequences of habitat selection. Am. Nat. 137, S91–S115. doi: 10.1086/285141
Huey, R. B., and Kingsolver, J. G. (1993). Evolution of resistance to high temperature in ectotherms. Am. Nat. 142, S21–S46. doi: 10.1086/285521
Huey, R. B., and Slatkin, M. (1976). Cost and benefits of lizard thermoregulation. Quart. Rev. Biol. 51, 363–384. doi: 10.1086/409470
Hutchison, V. H., and Kosh, R. J. (1965). The effect of photoperiod on the critical thermal maxima of painted turtles (Chrysemys picta). Herpetologica 20, 233–238.
IPCC (2022). “Summary for policymakers,” in Climate Change 2022: Mitigation of Climate Change. Contribution of Working Group III to the Sixth Assessment Report of the Intergovernmental Panel on Climate Change, eds P. R. Shukla, J. Skea, R. Slade, A. Al Khourdajie, R. van Diemen, D. McCollum, M. Pathak, S. Some, P. Vyas, R. Fradera, M. Belkacemi, A. Hasija, G. Lisboa, S. Luz, and J. Malley (Cambridge, New York, NY; Cambridge University Press).
Lara-Reséndiz, R. A., Galina-Tessaro, P., Sinervo, B., Miles, D. B., Valdez-Villavicencio, J. H., Valle-Jiménez, F. I., et al. (2021). How will climate change impact fossorial lizard species? Two examples in the Baja California Peninsula. J. Therm. Biol. 95, 102811. doi: 10.1016/j.jtherbio.2020.102811
Leiva, F. P., Calosi, P., and Verberk, W. C. (2019). Scaling of thermal tolerance with body mass and genome size in ectotherms: a comparison between water-and air-breathers. Philos. Trans. R. Soc. B Biol. Sci. 374, 20190035. doi: 10.1098/rstb.2019.0035
Longhini, L. S., Zena, L. A., Polymeropoulos, E. T., Rocha, A. C., da Silva Leandro, G., Prado, C. P. A., et al. (2021). Thermal acclimation to the highest natural ambient temperature compromises physiological performance in tadpoles of a stream-breeding savanna tree frog. Front. Physiol. 12, 726440. doi: 10.3389/fphys.2021.726440
Losos, J. B. (2008). Phylogenetic niche conservatism, phylogenetic signal and the relationship between phylogenetic relatedness and ecological similarity among species. Ecol. Letters. 11, 995–1003. doi: 10.1111/j.1461-0248.2008.01229.x
Lutterschmidt, W. I., and Hutchison, V. H. (1997a). The critical thermal maximum: data to support the onset of spasms as the definitive end point. Can. J. Zool. 75, 1553–1560. doi: 10.1139/z97-782
Lutterschmidt, W. I., and Hutchison, V. H. (1997b). The critical thermal maximum: history and critique. Can. J. Zool. 75, 1561–1574. doi: 10.1139/z97-783
Mahoney, J. J., and Hutchison, V. H. (1969). Photoperiod acclimation and 24-hour variations in the critical thermal maxima of a tropical and a temperate frog. Oecologia 2, 143–161. doi: 10.1007/BF00379157
Michonneau, F., Brown, J. W., and Winter, D. J. (2016). rotl: an R package to interact with the open tree of life data. Methods Ecol. Evol. 7, 1476–1481. doi: 10.1111/2041-210X.12593
Morley, S. A., Peck, L. S., Sunday, J. M., Heiser, S., and Bates, A. E. (2019). Physiological acclimation and persistence of ectothermic species under extreme heat events. Glob. Ecol. Biog. 28, 1018–1037. doi: 10.1111/geb.12911
Muñoz, M. M., Stimola, M. A., Algar, A. C., Conover, A., Rodriguez, A. J., Landestoy, M. A., et al. (2014). Evolutionary stasis and lability in thermal physiology in a group of tropical lizards. Proc. R. Soc. B: Biol. Sci. 281, 20132433. doi: 10.1098/rspb.2013.2433
Nakagawa, S., Noble, D. W. A., Senior, A. M., and Lagisz, M. (2017). Meta-evaluation of meta-analysis: ten appraisal questions for biologists. BMC Biol. 15, 18. doi: 10.1186/s12915-017-0357-7
Nakagawa, S., and Santos, E. S. A. (2012). Methodological issues and advances in biological meta-analysis. Evol. Ecol. 26, 1253–1274. doi: 10.1007/s10682-012-9555-5
Navas, C. A., Gomes, F. R., and Carvalho, J. E. (2008). Thermal relationships and exercise physiology in anuran amphibians: integration and evolutionary implications. Comp. Bioch. Physiol. A. Mol. Integr. Physiol. 151, 344–362. doi: 10.1016/j.cbpa.2007.07.003
Noble, D. W., Lagisz, M., O'dea, R. E., and Nakagawa, S. (2017). Nonindependence and sensitivity analyses in ecological and evolutionary meta-analyses. Mol. Ecol. 26, 2410–2425. doi: 10.1111/mec.14031
Nowakowski, A. J., Frishkoff, L. O., Agha, M., Todd, B. D., and Scheffers, B. R. (2018). Changing thermal landscapes: merging climate science and landscape ecology through thermal biology. Cur. Land. Ecol. Rep. 3, 57–72. doi: 10.1007/s40823-018-0034-8
Nowakowski, A. J., Watling, J. I., Whitfield, S. M., Todd, B. D., Kurz, D. J., and Donnelly, M. A. (2017). Tropical amphibians in shifting thermal landscapes under land-use and climate change. Conserv. Biol. 31, 96–105. doi: 10.1111/cobi.12769
Page, M. J., McKenzie, J. E., Bossuyt, P. M., Boutron, I., Hoffmann, T. C., Mulrow, C. D., et al. (2021). Updating guidance for reporting systematic reviews: development of the PRISMA 2020 statement. J. Clin. Epidemiol. 134, 103–112. doi: 10.1016/j.jclinepi.2021.02.003
Paradis, E., and Schliep, K. (2019). ape 5.0: an environment for modern phylogenetics and evolutionary analyses in R. Bioinformatics 35, 526–528. doi: 10.1093/bioinformatics/bty633
Pintanel, P., Tejedo, M., Ron, S. R., Llorente, G. A., and Merino-Viteri, A. (2019). Elevational and microclimatic drivers of thermal tolerance in Andean Pristimantis frogs. J. Biogeog. 46, 1664–1675. doi: 10.1111/jbi.13596
QGIS Development Team (2020). QGIS Geographic Information System. Open-Source Geospatial Foundation Project, Beaverton. Available online at: http://qgis.osgeo.org (accessed October, 2022).
Qu, Y. F., and Wiens, J. J. (2020). Higher temperatures lower rates of physiological and niche evolution. Proc. R. Soc. B: Biol. Sci. 287, 20200823. doi: 10.1098/rspb.2020.0823
R Core Team (2021). R: A Language and Environment for Statistical Computing. R Foundation for Statistical Computing. Available online at: https://www.R-project.org/ (accessed October, 2022).
Rezende, E. L., Tejedo, M., and Santos, M. (2011). Estimating the adaptive potential of critical thermal limits: methodological problems and evolutionary implications. Funct. Ecol. 25, 111–121. doi: 10.1111/j.1365-2435.2010.01778.x
Ribeiro, P. L., Camacho, A., and Navas, C. A. (2012). Considerations for assessing maximum critical temperatures in small ectothermic animals: insights from leaf-cutting ants. PLoS ONE 7, e32083. doi: 10.1371/journal.pone.0032083
Ruthsatz, K., Dausmann, K. H., Peck, M. A., and Glos, J. (2022). Thermal tolerance and acclimation capacity in the European common frog (Rana temporaria) change throughout ontogeny. J. Exp. Zool. Part A: Ecol. Integ. Physiol. 337, 477–490. doi: 10.1002/jez.2582
Ryan, L. M., and Gunderson, A. R. (2021). Competing native and invasive Anolis lizards exhibit thermal preference plasticity in opposite directions. J. Exp. Zool. Part A: Ecol. Integ. Physiol. 335, 118–125. doi: 10.1002/jez.2420
Scott, J. R., Tracy, C. R., and Pettus, D. (1982). A biophysical analysis of daily and seasonal utilization of climate space by a montane snake. Ecology. 6, 482–493. doi: 10.2307/1938965
Simon, M. N., Ribeiro, P. L., and Navas, C. A. (2015). Upper thermal tolerance plasticity in tropical amphibian species from contrasting habitats: implications for warming impact prediction. J. Therm. Biol. 48, 36–44. doi: 10.1016/j.jtherbio.2014.12.008
Sinervo, B., Mendez-De-La-Cruz, F., Miles, D. B., Heulin, B., Bastiaans, E., Villagrán-Santa Cruz, M., et al. (2010). Erosion of lizard diversity by climate change and altered thermal niches. Science. 328, 894–899. doi: 10.1126/science.1184695
Sterne, J. A. C., and Egger, M. (2006). “Regression methods to detect publication and other bias in meta-analysis,” in Publication Bias in Meta-Analysis: Prevention, Assessment and Adjustments, eds. H. R. Rothstein, A. J. Sutton, and M. Borenstein (Hoboken, NJ: Wiley), 99–110. doi: 10.1002/0470870168.ch6
Sunday, J. M., Bates, A. E., Kearney, M. R., Colwell, R. K., Dulvy, N. K., Longino, J. T., et al. (2014). Thermal-safety margins and the necessity of thermoregulatory behavior across latitude and elevation. Proc. Nat. Acad. Sci. 111, 5610–5615. doi: 10.1073/pnas.1316145111
Taylor, E. N., Diele-Viegas, L. M., Gangloff, E. J., Hall, J. M., Halpern, B., Massey, M. D., et al. (2021). The thermal ecology and physiology of reptiles and amphibians: a user's guide. J. Exp. Zool. Part A. Ecol. Integ. Physiol. 335, 13–44. doi: 10.1002/jez.2396
Telemeco, R. S., Fletcher, B., Levy, O., Riley, A., Rodriguez-Sanchez, Y., Smith, C., et al. (2017). Lizards fail to plastically adjust nesting behavior or thermal tolerance as needed to buffer populations from climate warming. Glob. Chang. Biol. 23, 1075–1084. doi: 10.1111/gcb.13476
Telemeco, R. S., and Gangloff, E. J. (2021). Introduction to the special issue-Beyond CTMAX and CTMIN: Advances in studying the thermal limits of reptiles and amphibians. J. Exp. Zoology Ecol. Integrat. Physiol. 335, 5–12. doi: 10.1002/jez.2447
Turriago, J. L., Tejedo, M., Hoyos, J. M., and Bernal, M. H. (2022). The effect of thermal microenvironment in upper thermal tolerance plasticity in tropical tadpoles. Implications for vulnerability to climate warming. J. Exp. Zool. Part A. Ecol. Integ. Physiol. 337, 746–759. doi: 10.1002/jez.2632
Viechtbauer, W. (2010). Conducting meta-analyses in R with the metafor. J. Stat. Softw. 36, 1–48. doi: 10.18637/jss.v036.i03
von May, R., Catenazzi, A., Corl, A., Santa-Cruz, R., Carnaval, A. C., and Moritz, C. (2017). Divergence of thermal physiological traits in terrestrial breeding frogs along a tropical elevational gradient. Ecol. Evol. 7, 3257–3267. doi: 10.1002/ece3.2929
White, C. R., Marshall, D. J., Chown, S. L., Clusella-Trullas, S., Portugal, S. J., Franklin, C. E., et al. (2021). Geographical bias in physiological data limits predictions of global change impacts. Funct. Ecol. 35, 1572–1578. doi: 10.1111/1365-2435.13807
Winter, M., Fiedler, W., Hochachka, W. M., Koehncke, A., Meiri, S., and De la Riva, I. (2016). Patterns and biases in climate change research on amphibians and reptiles: a systematic review. R. Soc. O. Sci. 3, 160158. doi: 10.1098/rsos.160158
Xu, W., Dang, W., Geng, J., and Lu, H. L. (2015). Thermal preference, thermal resistance, and metabolic rate of juvenile Chinese pond turtles Mauremys reevesii acclimated to different temperatures. J. Therm. Biol. 53, 119–124. doi: 10.1016/j.jtherbio.2015.09.003
Keywords: Anura, Caudata, CTMax, thermal biology, meta-analysis, meta-regression, Squamata
Citation: Carilo Filho LM, Gomes L, Katzenberger M, Solé M and Orrico VGD (2022) There and back again: A meta-analytical approach on the influence of acclimation and altitude in the upper thermal tolerance of amphibians and reptiles. Front. Ecol. Evol. 10:1017255. doi: 10.3389/fevo.2022.1017255
Received: 11 August 2022; Accepted: 20 October 2022;
Published: 04 November 2022.
Edited by:
Chun-Sen Ma, Institute of Plant Protection (CAAS), ChinaReviewed by:
Julian Chen, Institute of Plant Protection (CAAS), ChinaGeorgios Georgalis, Institute of Systematics and Evolution of Animals (PAN), Poland
Liang Zhu, Institute of Plant Protection (CAAS), China
Copyright © 2022 Carilo Filho, Gomes, Katzenberger, Solé and Orrico. This is an open-access article distributed under the terms of the Creative Commons Attribution License (CC BY). The use, distribution or reproduction in other forums is permitted, provided the original author(s) and the copyright owner(s) are credited and that the original publication in this journal is cited, in accordance with accepted academic practice. No use, distribution or reproduction is permitted which does not comply with these terms.
*Correspondence: Leildo M. Carilo Filho, bGVpbGRvY2FyaWxvJiN4MDAwNDA7Z21haWwuY29t