- 1Institute of Ecology and Biodiversity (IEB), Ñuñoa, Santiago, Chile
- 2Cape Horn International Center (CHIC), Puerto Williams, Chile
- 3Millennium Institute Biodiversity of Antarctic and Subantarctic Ecosystems (BASE), Santiago, Chile
- 4British Antarctic Survey (BAS), Natural Environment Research Council, Cambridge, United Kingdom
- 5Natural History Museum of Denmark, University of Copenhagen, Copenhagen, Denmark
- 6Departamento de Zoología, Universidad de Concepción, Concepción, Chile
- 7Facultad de Ciencias, Centro FONDAP IDEAL, Instituto de Ciencias Marinas y Limnológicas (ICML), Universidad Austral de Chile, Valdivia, Chile
- 8Laboratorio de Estudios Dulceacuícolas Wankara, Programa de Conservación Biocultural Subantártica, Universidad de Magallanes, Punta Arenas, Chile
- 9Department of Zoology, University of Johannesburg, Johannesburg, South Africa
Two main hypotheses have been proposed to explain the contemporary distribution of Antarctic terrestrial biota. We assess whether the current distribution of maritime Antarctic populations of the freshwater copepod Boeckella poppei is the result of (1) a post-Last Glacial Maximum (LGM) colonization, or whether (2) the species survived in regional glacial refugia throughout the LGM and earlier glaciations. Using 438 specimens from 34 different sampling sites across Southern South America, South Georgia, South Orkney Islands, South Shetland Islands, and the Antarctic Peninsula, we analyzed mitochondrial and nuclear sequences to uncover patterns of genetic diversity and population structure. We also performed median-joining haplotype network, phylogenetic reconstruction, and divergence time analyses. Finally, we evaluated past demographic changes and historical scenarios using the Approximate Bayesian Computation (ABC) method. Our data support the existence of two clades with different and contrasting biogeographic histories. The first clade has been present in maritime Antarctica since at least the mid-Pleistocene, with the South Orkney Islands the most likely refugial area. The second clade has a broader distribution including southern South America, South Georgia, South Shetland Islands, and the Antarctic Peninsula. The ABC method identified long-distance dispersal (LDD) colonization event(s) from southern South America to South Georgia and the maritime Antarctic after the LGM deglaciation, supporting more recent colonization of Antarctic locations. The current Antarctic and sub-Antarctic distribution of B. poppei is likely derived from two independent biogeographic events. The combination of both (1) post-LGM colonization from southern South America and (2) longer-term persistence in in situ regional refugia throughout glacial periods challenges current understanding of the biogeographic history of Antarctic freshwater biota. Re-colonization of ice-impacted Antarctic areas would have occurred following a LDD and Establishment model, pointing to the existence of possible post-dispersal barriers, despite widely assumed high passive dispersal capacity in freshwater invertebrates.
Introduction
During the Quaternary, Antarctica experienced multiple glacial–interglacial cycles (Gersonde et al., 2005; Hassold et al., 2009). These cycles of ice sheet expansion and contraction across the Antarctic continent and its continental shelf are widely assumed to have caused severe extinction at high latitudes, leaving a legacy of impoverished polar and sub-polar terrestrial biotas. The contemporary freshwater fauna of the maritime Antarctic (i.e., Antarctic Peninsula and Scotia Arc archipelagos) and sub-Antarctic is extremely simple in terms of species richness and community structure, and generally includes only a very small number of insects and crustaceans, and smaller invertebrate groups such as tardigrades, rotifers, nematodes, and microscopic Protozoa (Chown and Convey, 2016; Convey, 2017).
Two main hypotheses have been proposed to explain how Quaternary events have shaped the contemporary distribution, demography, and genetic structure of higher latitude terrestrial and freshwater biota (Bennet et al., 1991; Taberlet et al., 1998). The dispersal hypothesis suggests that this biota results from postglacial colonization from lower latitudes, through range expansion during deglaciation (Hewitt, 2004). The contrasting in situ refugia hypothesis suggests that some high latitude biota survived in regional refugia throughout multiple Pleistocene glacial cycles, including the very extensive Last Glacial Maximum (LGM; ~20,000 years before present, YBP), and expanded from these following deglaciation (Provan and Bennett, 2008). Relatively few continental phylogeographic studies are available in the Southern Hemisphere, and freshwater ecosystems remain particularly under-researched (Avise and Ayala, 2017). Evidence of in situ refugia during at least the LGM and generally on much longer timescales has been suggested in mosses (Pisa et al., 2014; Biersma et al., 2018b, 2020a), springtails (Stevens and Hogg, 2003; McGaughran et al., 2010; Carapelli et al., 2017, 2020), nematodes (Maslen and Convey, 2006), midges (Allegrucci et al., 2012), mites (Mortimer et al., 2011; van Vuuren et al., 2018; Brunetti et al., 2021) and tardigrades (Short et al., 2022). On the other hand, although to a smaller number of phylogeographic studies in plants (Wouw et al., 2008; Fasanella et al., 2017; Biersma et al., 2020b), have proposed more recent post-LGM dispersal from northern locations.
In support of the post-LGM hypothesis, Pugh et al. (2002) listed only 101 freshwater crustacean species known to occur in Antarctica and on the Southern Ocean islands, representing <2% of the global non-marine crustacean fauna of ca. 6,000 species. In the apparently complete absence of paleoendemic crustaceans on the continent, they suggested that the Antarctic was likely (re-)colonized by freshwater fauna during the Holocene by dispersal from the sub-Antarctic islands and southern continents, primarily South America, or possibly even more recently through direct human assistance. However, recent evidence has questioned these conclusions, suggesting that freshwater Crustacea persisted on the Antarctic continent throughout glacial cycles. Cromer et al. (2006) documented the presence of the freshwater cladoceran Daphniopsis studeri throughout the entire sediment core of a lake in the Larsemann Hills, continental Antarctica, which has been in existence for up to 130,000 YBP, between the last interglacial period and the present day (Hodgson et al., 2005). This is, to date, the only documented example from the freshwater realm providing direct evidence supporting persistence and subsequent re-expansion of Antarctic freshwater fauna from in situ refugia.
The Southern Hemisphere copepod genus Boeckella includes 42 species distributed in Australia, New Zealand, Tasmania, New Caledonia, South America, maritime and continental Antarctica, and various sub-Antarctic and cool-temperate oceanic islands (Maturana et al., 2019). Fourteen species are recognized across South America, Antarctica, and the sub-Antarctic region. Among them, B. poppei (Mrázek, 1901) is the only species reported from lakes across sub-Antarctic islands, the maritime and continental Antarctic as well as from higher latitudes in southern South America (Maturana et al., 2019). Paleolimnological studies of post-LGM-formed lakes have revealed B. poppei in the basal sediments in lakes on Signy Island, South Orkney Islands, and on the northern Antarctic Peninsula, both dated to 5,500 years (Jones et al., 2000; Gibson and Zale, 2006). Similar analyses of the Amery Oasis lakes demonstrated that B. poppei has been present for at least 10,000 years (Bayly et al., 2003; Bissett et al., 2005).
Contemporary Antarctic inland fauna is restricted to ice-free areas, which contribute between only 0.18% (Burton-Johnson et al., 2016) and 0.44% (Brooks et al., 2019) of the entire continent. During periods of glacial maxima, ice-free areas were further reduced, particularly at low altitudes where the majority of terrestrial habitats occur today. Precise locations of refugia remain unclear (Convey et al., 2020), in part because of the relatively low spatial resolution in most existing glaciological models. However, there are candidate areas that fulfill the requirements for refugia across maritime and continental Antarctica. Areas subject to geothermal activity, which can maintain ice-free terrain in otherwise glaciated regions, have been proposed as potential refugial locations in some parts of Antarctica (Convey and Smith, 2006; Fraser et al., 2014). In parts of the maritime Antarctic, areas of volcanic activity existed during the Pleistocene; in particular, the South Sandwich Islands and parts of the South Shetland Islands are currently active or have been so since and during the LGM (Fraser et al., 2014). Although many lakes were frozen to their base during glacial maxima, some lakes with permanent ice cover may have provided plausible refugia over glacial–interglacial cycles for certain taxa, for example in East Antarctica (Cromer et al., 2006). However, the generality of lake persistence through full glacial cycles remains to be demonstrated.
Antarctic biota possess many adaptations to extreme environmental conditions. Cryptobiosis has been reported in different life stages of many terrestrial and freshwater taxa in response to extreme desiccation, freezing temperatures, anoxia, and osmotic stress. The presence of resistant eggs has been reported in B. poppei from the Antarctic Peninsula (Almada et al., 2004), Signy Island (Heywood, 1970), and the South Shetland Islands (Reed et al., 2021). Jiang et al. (2012) suggested that B. poppei eggs could survive for a mean of 47 y (maximum 196 y) in sediments of two South Shetland Islands lakes.
The presence of suitable habitats in combination with such survival adaptations further supports the potential for rapid colonization of lakes soon after their formation, consistent with the local refugial hypothesis. The presence of biota very soon after estimated times of glacial retreat has been interpreted as being consistent with local refugial sites from which recolonization would be rapid and straightforward, rather than requiring occasional and stochastic long-distance recolonization from remote refugia (Carapelli et al., 2017; Maturana et al., 2020). It has also been proposed that copepods such as B. poppei have very low autonomous dispersal potential, reducing the likelihood of long-range post-glacial dispersal (Maly and Bayly, 1991; Pugh et al., 2002; Scheihing et al., 2009). In analogous recent studies of many elements of the Antarctic terrestrial biota (including interstitial groups such as nematodes and tardigrades, whose species-level diversity overlaps in terrestrial and freshwater habitats), a strong pattern of long-term persistence over multi-million year timescales is emerging (Biersma et al., 2020a; see review in Convey et al., 2020; Baird et al., 2021). Hence, a hypothesis of long-term persistence in glacial refugia for B. poppei must be seriously considered.
To critically assess these two hypotheses (local extinction vs refugial survival) as applied to Antarctic freshwater crustaceans, we performed molecular and phylogeographic analyses, including widespread sampling of B. poppei in Antarctica, the sub-Antarctic islands, and southern South America. We evaluated the timescales of persistence of maritime and sub-Antarctic populations, assessing divergence times, phylogeographic structure, and past demographic changes across regions. The refugial hypothesis is examined in detail, with candidates for possible historical Antarctic freshwater refugia identified over multiple glacial cycles.
Materials and methods
Sample collection and taxonomic identification
Boeckella individuals were collected from lakes, ponds and other freshwater bodies using a zooplankton net (200 μm pore diameter) within five sampling areas (i-v) and three biogeographic regions (1–3): (1) maritime Antarctic (MA), including: (i) South Shetland Islands (62°S 61°W; SSI), (ii) Antarctic Peninsula (67°S 67°W; AP), and (iii) South Orkney Islands (60°S 45°W; SOI); (2) sub-Antarctic: (iv) South Georgia (54°S 36°W; SG); and (3) (v) southern South America (51°S 72°W; SA; Figure 1; for more detail see Supplementary Table S1). We used only sampling sites that contained specimens belonging to the “nominal B. poppei” clade representing the type species, as identified by Maturana et al. (2021). Subsequent morphological and molecular analyses were conducted following Maturana et al. (2021).
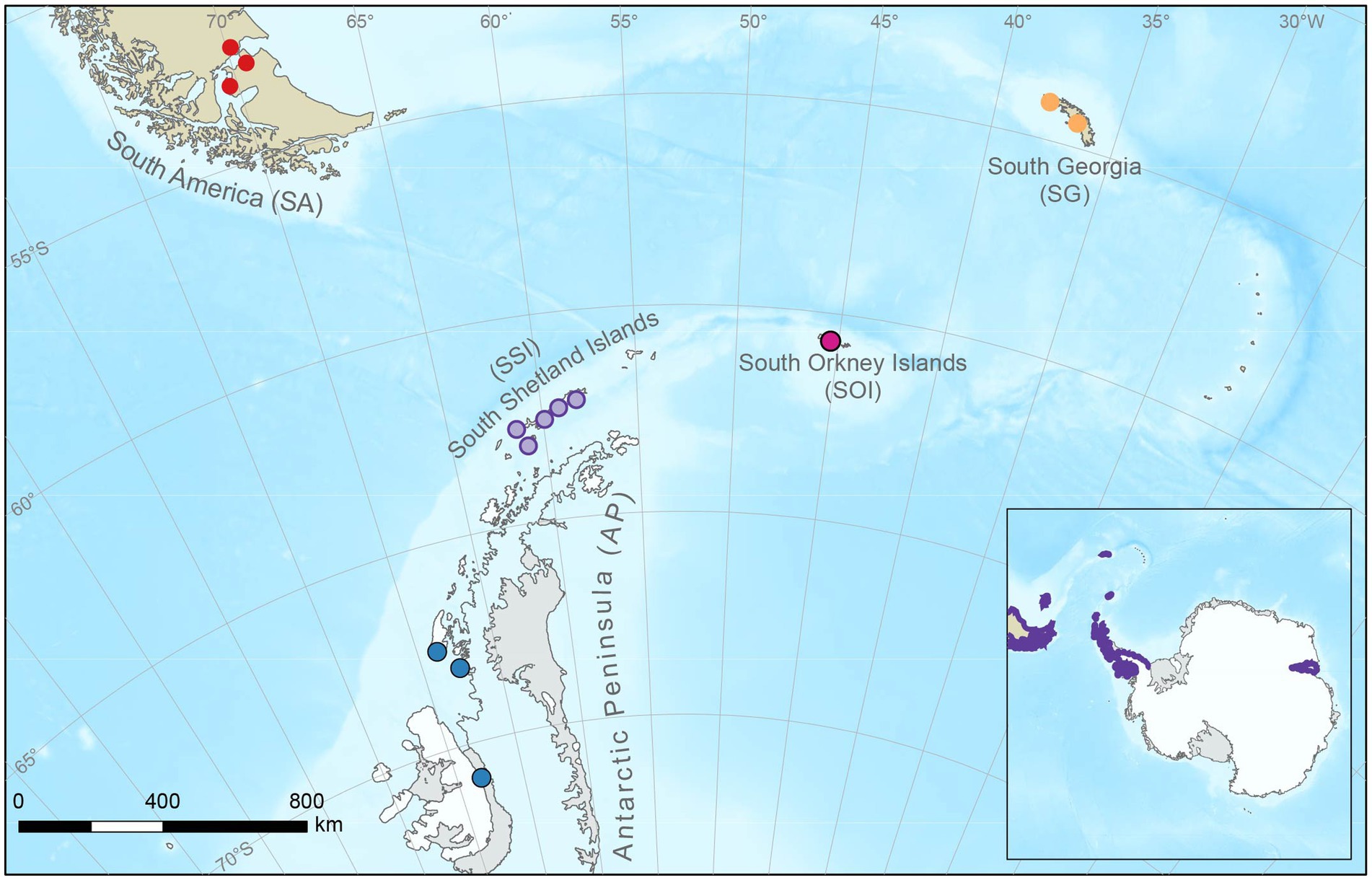
Figure 1. Boeckella poppei sampling areas. (1) Maritime Antarctic: (i) South Shetland Islands (violet): King George, Robert, Greenwich, Livingston, and Deception Islands, (ii) Antarctic Peninsula (blue): Avian, Horseshoe, and Alexander Islands, (iii) South Orkney Islands (orange): Signy Island; (2) sub-Antarctic: (iv) South Georgia (pink); and (3) southern South America (red). Inset: current distribution range of B. poppei is highlighted in purple. Base map produced by MAGIC, British Antarctic Survey. Bathymetry - GEBCO Compilation Group (2021) GEBCO 2021 Grid (doi:10.5285/c6612cbe-50b3-0cff-e053-6c86abc09f8f). Coastlines - data from the SCAR Antarctic Digital Database, accessed 2022.
DNA preparation and sequence editing
We extracted DNA from complete specimens using the DNeasy Blood & Tissue Kit protocol modified for a small amount of tissue (see Appendix S1 in Data Source). Three DNA loci were amplified: one segment of the fast-evolving mitochondrial cytochrome c oxidase subunit I (cox1), and two slower-evolving segments of the nuclear DNA (nucDNA) 28S rRNA gene and the Internal Transcribed Spacers 1 and 2 (ITS hereafter). For molecular protocols we followed Maturana et al. (2021) (see Appendix S2, S3 in Data Source). Sequences were manually quality controlled, examined, assembled, and edited using GENEIOUS 10.2.2 (Kearse et al., 2012). Alignments were obtained using MUSCLE (Edgar, 2004) with standard settings. For cox1, synonymous codon usage was determined using the “effective number of codons” value (ENC, Wright, 1990) from DnaSP 5.0 (Librado and Rozas, 2009). This statistic has a value between 20 (extremely biased codon usage) and 61 (totally random codon usage).
Genetic diversity and genealogical reconstruction in Boeckella poppei
Genetic polymorphism levels were determined for each locus using standard diversity indices including number of haplotypes (k), number of segregation sites (S), haplotype diversity (H), average number of pairwise differences (Π), and nucleotide diversity (π) for each of the five sampling areas, including the entire MA (SOI, SSI, and AP), sub-Antarctic SG, and SA using DnaSP v5.10.01 (Librado and Rozas, 2009). We estimated the number of private alleles per sampling area and region as a proxy to assess the potential existence of refugia (Maggs et al., 2008). Neutrality tests (Tajima’s D and Fu’s FS) were performed to assess deviation from the neutral model.
Genealogical relationships were estimated for mitochondrial and nuclear markers by constructing a median-joining haplotype network (Bandelt et al., 1999) using PopArt.1 Prior to this, haplotypes of nuclear sequences from heterozygous individuals were determined using the algorithms provided by PHASE (Stephens and Donnelly, 2003) in DnaSP.
Genetic and phylogeographic structure
We estimated levels of genetic differentiation for each locus among the five analyzed areas through mean pairwise differences (ΦST, using Kimura-2P genetic distances) and haplotype frequencies (FST) in ARLEQUIN v3.5.2.2 (Excoffier and Heckel, 2006), using 10,000 permutations to assess significance. To test statistical significance of differentiation, we performed a permutation test (20,000 iterations). The p-value for pairwise ΦST and FST between populations was corrected using the false discovery rate correction (FDR; Benjamini et al., 2005).
Phylogenetic reconstruction
We performed phylogenetic analyses using the entire cox1 + ITS + 28S rRNA dataset, combined in SequenceMatrix software v1.8 (Vaidya et al., 2011). Nucleotide substitution models and the optimal partitioning scheme were selected separately for each gene using the corrected Akaike Information Criteria (AICc) in PartitionFinder v2.1 (Guindon et al., 2010; Lanfear et al., 2017). The GTR + Ι + Γ model was used for cox1 and the HKY model for 28S rRNA and ITS. For analyses of cox1, 28S, and ITS, the tree was rooted with B. vallentini, based on previous phylogenetic reconstructions in Boeckella (Maturana et al., 2021).
Phylogenetic reconstructions were conducted in a maximum likelihood (ML) framework in MEGA X (Kumar et al., 2018), and via Bayesian Inference (BI) in MrBayes v.3.2.6 (Ronquist and Huelsenbeck, 2003). Tree node support values for ML analyses were assessed through bootstrap (BS) with the full heuristic search option and 1,000 pseudoreplicates (Felsenstein, 1981). Bayesian inference posterior probabilities (PP) were estimated using the Markov chain Monte Carlo algorithm (MCMC), running four chains for 50 × 106 generations with trees sampled every 500 generations. The initial 10% of the parameter values were discarded as “burn-in.” Convergence was assessed by checking that split frequencies had an average standard deviation below 0.01 and all parameters had effective sample sizes (ESS) > 200 using Tracer v.1.6 (Rambaut et al., 2014). Maximum clade credibility trees were generated using TREEANNOTATOR 2.4.7 (Bouckaert et al., 2014) and visualized with FigTree 1.4.2.2
Divergence time estimations
Phylogenetic reconstruction and divergence time analyses were performed in BEAST v.2.4.6 (Bouckaert et al., 2014) using the cox1 dataset and applying a relaxed lognormal molecular clock. The molecular clock model was selected following Bayesian Model Selection with nested sampling (Russel et al., 2019) using the NS package implemented in BEAST v.2.6.7. The nucleotide substitution model HKY + Ι + Γ was selected using the corrected Akaike Information Criteria (AICc) in PartitionFinder v2.1 as above. We included cox1 haplotypes of B. vallentini and B. brasiliensis as outgroups. A Birth-Death model speciation prior was used for branching rates in the phylogeny, four chains were run three times for 20 × 106 generations, and trees were sampled every 2,000 generations. Based on the absence of a fossil record for Boeckella and no direct estimates of mutation rates for cox1 being available for copepods, the time of the most recent common ancestor (TMRCA) was estimated using a conservative cox1 mutation rate of 1.8% per site per million years, as estimated for limnic amphipods (Copilaş-Ciocianu et al., 2019), and in accordance with other estimates for crustaceans (Knowlton and Weight, 1998; Lessios, 2008). Convergence was checked in Tracer v.1.6 to ensure all ESS values were >1,000. A maximum clade credibility with median node heights was generated using TREEANNOTATOR 2.4.7 and visualized with FigTree 1.4.2.
Inference of demographic history of Boeckella poppei
We estimated population dynamics through time for each demographic unit using the Bayesian Skyline Plot (BSP) method implemented in BEAST v2.4.7, based on the cox1 marker. We used a population substitution rate of tenfold the evolutionary rate (18% per million year) to estimate the TMRCA of each demographic unit, as this more accurately reflects the rate at which new haplotypes appear. This better accounts for the time-dependence of molecular evolution at population level, brings divergence estimates closer to the present, and avoids over-estimation of recent splits in intraspecific lineages (Ho et al., 2008, 2011). As suggested by these authors, molecular studies at population level display much higher substitution rates (i.e., within clades) than the mutation rates (i.e., fixed mutations among clades) inferred from phylogenetic analyses. Although this interpretation of time dependence remains controversial (Emerson and Hickerson, 2015; Ho et al., 2015; Cabrera, 2021), a growing number of studies provide empirical evidence that phylogenetic mutation rates overestimate the date of recent phylogeographic processes (Burridge et al., 2008; Ho et al., 2008; Ney et al., 2018). For example, in katydid insects the intraspecific mutation rate calibrated with a post-Pleistocene event was 14.4%–17.3% per million years, 13 times greater than the phylogenetic mutation rate (Ney et al., 2018). Similar corrections have been implemented in population-based studies of several Antarctic organisms, including fin whales (Pérez-Alvarez et al., 2021), copepods (Maturana et al., 2020), sea urchins (Diaz et al., 2018), gentoo penguins (Vianna et al., 2017), algae (Billard et al., 2015) and limpets (González-Wevar et al., 2011). The two independent MCMC calculations were run for 50 × 106 generations (sampled every 1,000 iterations), discarding the first 10% of parameter values as burn-in. The convergence of runs was confirmed with Tracer v1.6.
Historical scenarios
To assess the influence of glacial cycles on demographic history and genetic structure, we used DIYABC v.2.0.4 (Cornuet et al., 2008) for inference based on the Approximate Bayesian Computation (ABC) method (Beaumont et al., 2002) to evaluate the long-term persistence of maritime Antarctic populations of B. poppei and the existence of putative refugia within the species’ distribution. Different scenarios were built to examine competing hypotheses based on population structure, phylogenetic and demographic inference, using cox1 analyses (for more details see Appendix 1).
Posterior probabilities of scenarios were estimated using historical, demographic, and mutational parameters drawn from prior distributions. Scenarios were compared in DIYABC using (1) a logistic regression of the 1% of simulated data closest to the observed data; (2) a “direct” principal component analysis to visualize similarity of the observed and simulated statistics for each analysis. We evaluated possible refugia for each of the main clades/haplogroups obtained from phylogenetic reconstruction.
Results
Occurrence of Boeckella poppei and molecular sequence data
Within the framework of this four-year study (2015–2018), we sampled 173 freshwater sites within the reported distribution of B. poppei. Among these, 85 were in southern SA, 10 in the Falkland/Malvinas Islands, 12 in SG and 66 in the MA (SOI = 16, SSI = 37, AP = 13). Since we used the “nominal B. poppei” clade following the type species criteria, Falkland/Malvinas Islands and many SA locations were excluded from further analyses. In total, 438 individuals of B. poppei were obtained from 34 different sampling sites (SA = 3, SG = 2, SSI = 18, AP = 4, SOI = 7; Figure 1; Supplementary Table S1).
The greatest number of sequences was obtained for cox1 (n = 438), followed by ITS (n = 157) and 28S (n = 97). We did not recover any missing data at each DNA marker. Alignments of cox1, 28S, and ITS consisted of 420, 655, and 649 bp, respectively. The cox1 alignment did not include insertions/deletions or stop codons, and no evidence for codon bias was detected (ENC = 39.20). The mitochondrial cox1 locus included 50 variable sites (11.9%), with 28 (6.7%) being parsimony informative. The nuclear regions showed less genetic variation, with 28S and ITS containing 1 and 6 variable sites and 1 and 2 parsimony informative sites, respectively.
Genetic diversity
Global mitochondrial haplotype diversity (Hd) was 0.882, with small variations among sampling locations, areas and biogeographic regions (Supplementary Table S2). Within the MA, SOI and AP exhibited the highest diversity (Hd = 0.816 and 0.812, respectively). Despite the higher number of individuals (n = 210), lakes (n = 18) and locations (five islands; Supplementary Table S1), the SSI had lower haplotype diversity (Hd = 0.726) compared with other locations within the MA. However, SSI displayed the highest number of nucleotide differences (Π) and nucleotide diversity (π). SG had the lowest haplotype and nucleotide diversity (0.647 and 0.002, respectively). In contrast, SA exhibited high genetic diversity for both metrics (Hd = 0.813, π = 0.007, n = 68).
The cox1 median-joining network identified 75 distinct haplotypes, 71 of which were private, including 49 unique haplotypes (Supplementary Table S2; Figure 2). Two main haplogroups separated by three mutational steps were distinguished (HG1 and HG2; Figure 2). HG1 included individuals from SSI (King George, Robert, Greenwich, and Deception Islands) and SOI. The central haplotype (H1) was frequent (17%) and shared by both localities (SSI = 92.3%, SOI = 7.7%). HG2 included individuals from SSI (King George, Robert, and Livingston Islands), AP, SG, and SA. This haplogroup also had a frequent and central haplotype (H = 27%) distributed in all localities where it occurred (SSI = 69%, SG = 17.6% and AP = 13.4%). Within HG2, SA populations did not share haplotypes with any MA populations. Each sampling area was characterized by the presence of a single haplogroup, except for SSI where both haplogroups were present. Within SSI, both haplogroups coexisted primarily on King George Island and to a lesser extent Robert Island. However, Deception, Livingston, and Greenwich islands contained only one of the two haplogroups (Supplementary Figure S3).
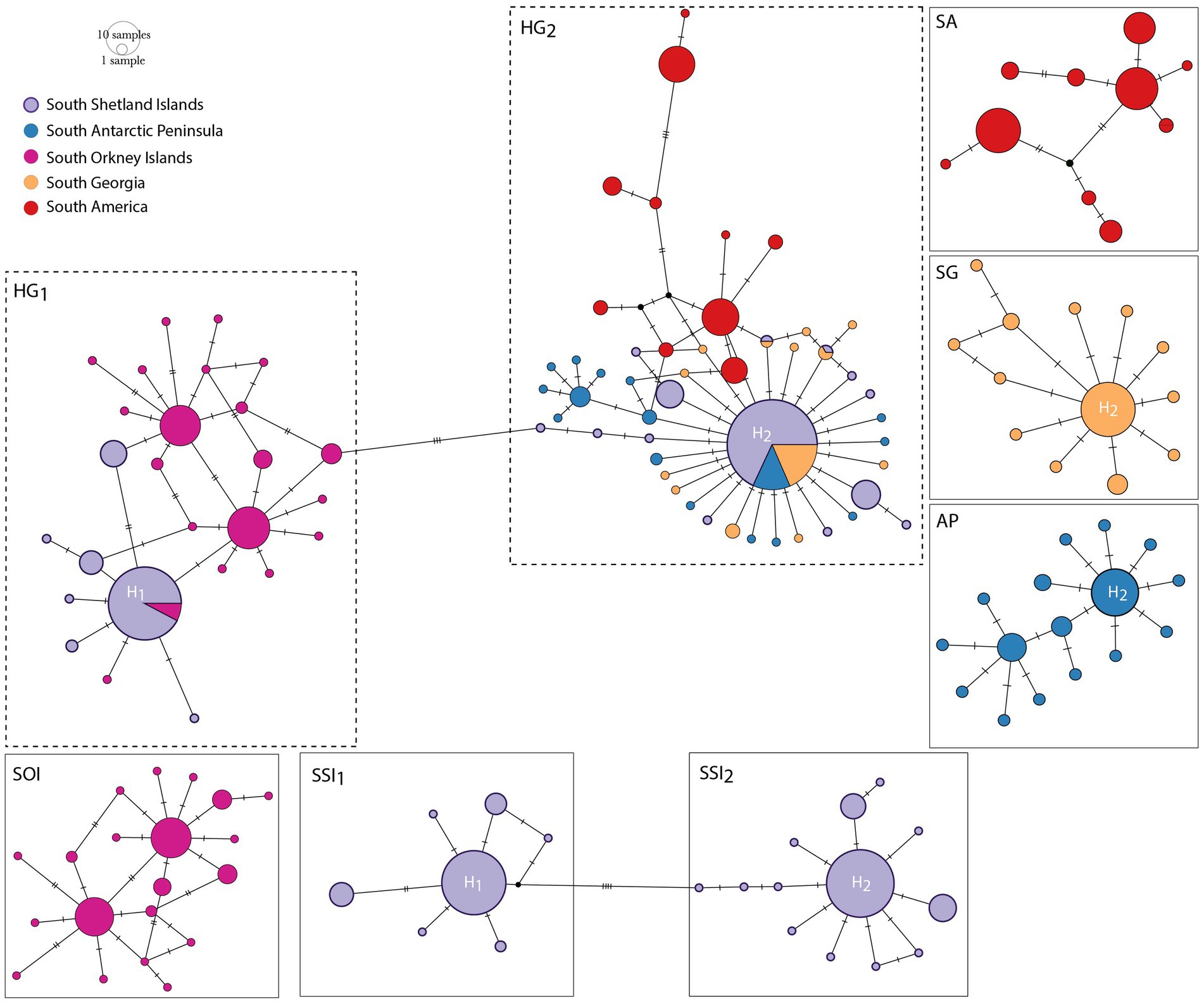
Figure 2. Haplotype network for Boeckella poppei, based on 438 mtDNA cox1 sequences spanning the species’ distribution. Neighbor-joining network illustrating the distribution of haplotypes across lakes in southern South America, South Georgia, the South Orkney Islands, the South Shetland Islands, and Antarctic Peninsula. Circles sizes are proportional to haplotype frequency. Color key indicates sampling areas illustrated in Figure 1.
The haplotype networks constructed for the five sampling areas (AP, SSI, SOI, SG, and SA) showed the different topologies more clearly (inset Figure 2). The SSI network represents the most expanded genealogy, with the two haplogroups separated by eight mutational steps. However, when the two haplogroups were examined separately (hereafter SSI1 and SSI2), each was characterized by a star-like topology and short genealogy. Similarly, the network from SG showed a star-like topology and short genealogy. Tajima’s and Fu’s neutrality tests were both negative and significant for these locations (Supplementary Table S2). In contrast, the networks from AP, SOI, and, particularly, SA exhibited more expanded genealogies.
The 28S locus had extremely low global genetic diversity with only two haplotypes (Supplementary Figure S4A). One was dominant, shared among all sampling areas, and the other was only present in SA. The global genetic diversity for ITS was higher (Supplementary Figure S4B), with six haplotypes. A dominant haplotype was shared across all five areas, but the other haplotypes were exclusively present in one of SSI, AP or SA.
Genetic and phylogeographic structure
We estimated genetic differentiation using only the cox1 dataset, since low genetic diversity in the nuclear markers precluded further analyses (Supplementary Table S2; Supplementary Figures S4A,B). Overall, B. poppei displayed strong genetic and phylogeographic structure across sampled areas, with all ΦST and FST values being highly significant, except for FST between SG and AP (Supplementary Table S5). SOI and SA had the highest, significant, values for all comparisons with both indices.
Phylogenetic and divergence time estimation
Multi-locus phylogenetic reconstruction identified the divergence of the two main clades (A and B) with high PP and BS support (Supplementary Figure S6). However, relationships within each clade were not completely resolved with low support values. Molecular dating analysis based on cox1 provided estimates of divergence times across the sampled regions (Figure 1).
Based on the interpretation of outgroup specimens, previous phylogenetic and ancestral area reconstruction analyses of the genus (Maturana et al., 2021), divergence time estimates suggest B. poppei originated from an SA ancestor, with Antarctic diversification during the mid-Pleistocene at 994,090 YBP (95% height posterior density (95HPD): 1.5 M–586,090 YBP). This period included an early major branching event with strong PP support (PP = 1) between a clade containing SSI1 and SOI specimens (hereafter clade A, Figure 3) and a clade containing the SSI2, AP, SG, and SA specimens (hereafter clade B, Figure 3).
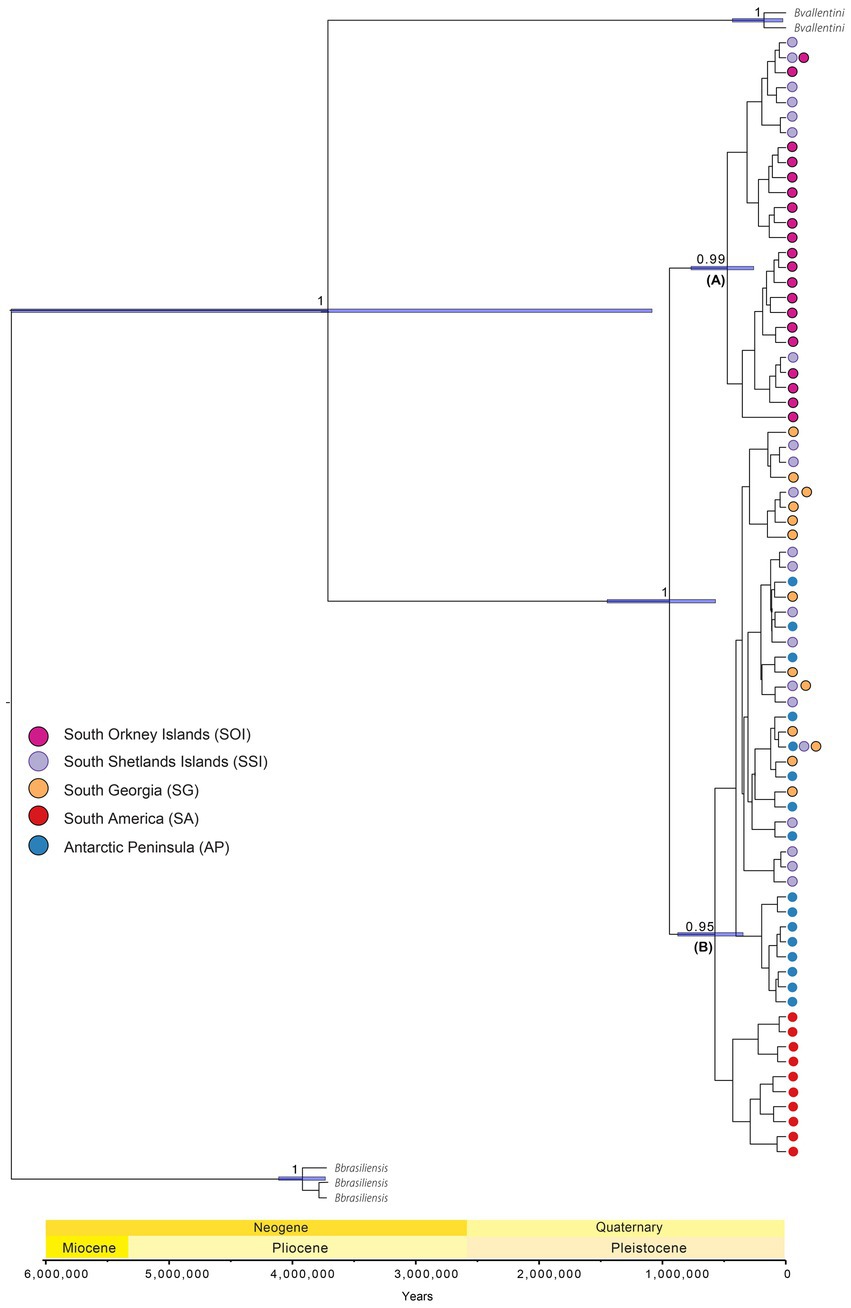
Figure 3. Historical biogeography and divergence times of B. poppei across South America, maritime and sub-Antarctic biogeographic regions using cox1. The maximum clade credibility tree shows the median divergence time estimates with 95% height posterior density (95HPD) calculated with a mutation rate of 1.8% per site per million years [based on Copilaş-Ciocianu et al. (2019)]. Different colors represent different regions and geographical ranges (also see Figure 1). Only high posterior probability support values (>0.85) are shown next to all major branches.
Past demographic events
Based on Bayesian phylogenetic reconstruction, haplotype network, and phylogeographic structure analyses, we evaluated the two haplogroups from the SSI (SSI1 and SSI2) as two independent demographic units. SSI1 included haplotypes from Deception, Greenwich, Robert, and King George Islands, while SSI2 included haplotypes from Livingston, Robert, and King George Islands. The remaining four geographic areas were considered as independent demographic units.
Bayesian Skyline Plot analyses derived from the 10× corrected substitution rate (Figure 4) provided historical population dynamic patterns and dates for TMRCA for the six demographic units (SSI1, SSI2, SOI, SG, AP, and SA). TMRCA obtained for SSI1, SSI2 and SG, were very similar at ca. 10,000 YBP (10,861, 10,750, and 10,872, respectively). SOI had the oldest TMRCA at ca. 24,230 YBP (95% HPD 7,743–45,568 YBP), followed by AP (16,898 YBP; 95% HPD 4,746–32,589). Overall, the date for the onset of population expansion within most sampling areas from the maritime and sub-Antarctic regions was estimated at ca. 7,000 YBP (Figure 4), with the exception of both demographic units identified within SSI (SSI1 and SSI2), for which population expansion was estimated around 4,000 YBP. For the Patagonian B. poppei population, we did not detect a population expansion signal, and estimated a TMRCA of 38,095 YBP.
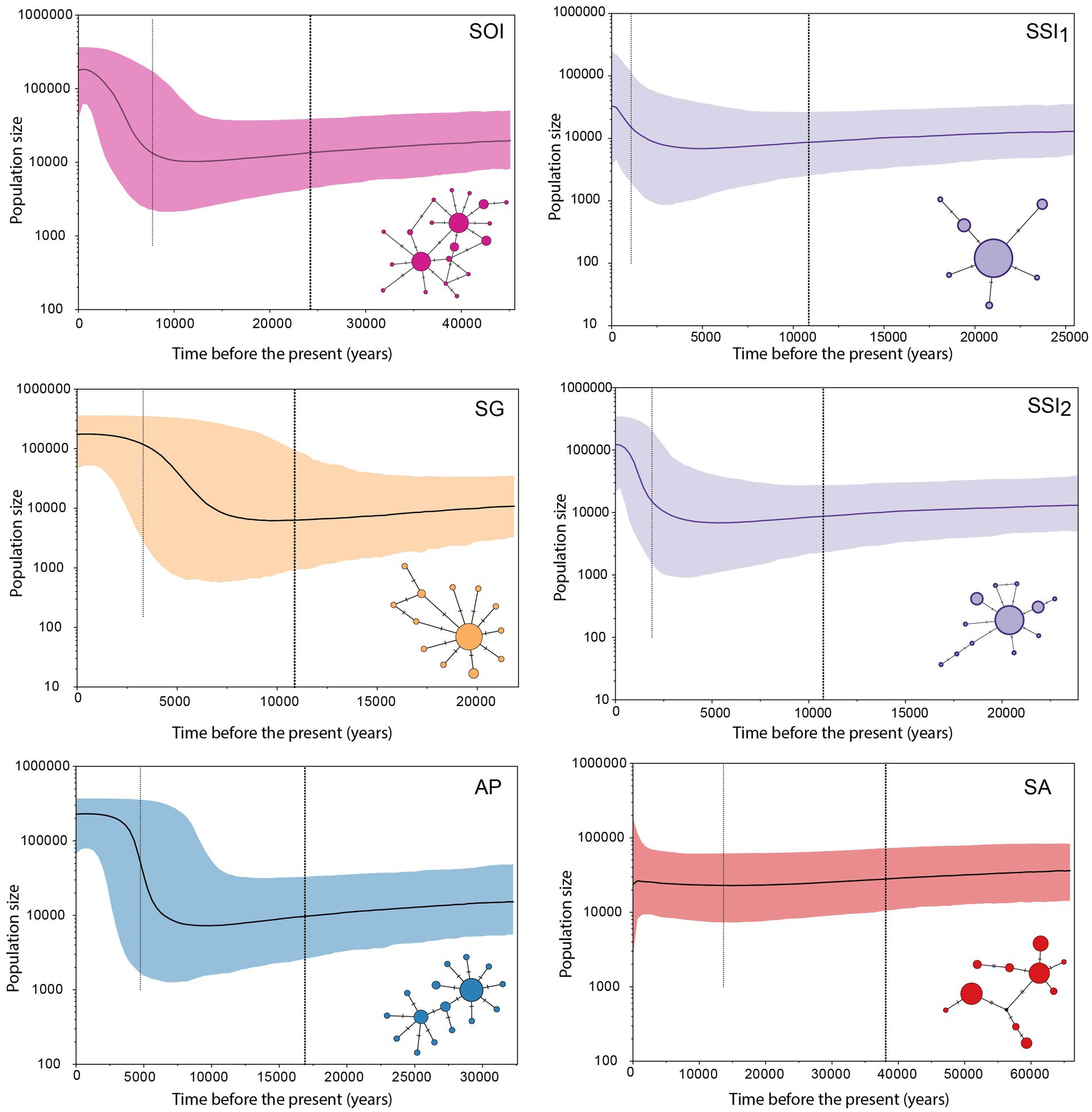
Figure 4. Historical demographic trends. Bayesian Skyline Plots (BSP) for genetic groups found in the five sampling areas of B. poppei, based on cox1. Effective population size trends (Ne) calculated using the 10× corrected substitution rate of the standard estimate. The y-axis shows the product of effective population size (Ne) and generation length in a log scale, while the x-axis shows time before present. The median estimate (solid black line) and 95% highest probability density (HPD) limits (colored area) are shown. The thick dashed line represents the time of the most recent common ancestor (TMRCA).
Historical scenarios
For clade A, we compared the posterior probabilities of two scenarios, reflecting the presence of this clade in only two locations (SOI and SSI). In Scenario 1, we proposed SOI as a refugium, with a post-glacial colonization of SS11 area, while Scenario 2 considered SSI1 as a refugium with postglacial colonization of SOI (Supplementary Figure S7, Clade A). For the more widespread clade B, we compared the posterior probability of refugia in the MA and sub-Antarctic regions (Scenario 1) with that of SA as the main refugium and post-glacial colonization of MA and sub-Antarctic regions (Scenario 2). Then, we evaluated which localities within the MA (SSI2 and AP) and SG were the most likely locations of refugia (under Scenario 1), or to be colonized from SA (Scenario 2). The historical constructed models are included in Supplementary Figure S7.
For clade A, ABC supported SOI, rather than SSI, hosting the most likely refugia, providing the highest posterior probability with both approaches (direct 0.988; logistic 0.997; Supplementary Table S8). For clade B, ABC supported post-glacial recolonization from SA to SG and MA, providing the highest posterior probability with both approaches (direct 1.000; logistic 0.999). For recolonization from SA, AP was the most likely area to be colonized first (direct 0.634; logistic 0.5810), followed by SG (direct 0.254; logistic 0.346) and finally SSI (direct 0.112; logistic 0.074).
Discussion
This study is the first molecular phylogeographic study of a freshwater crustacean across the maritime Antarctic, sub-Antarctic islands, and South America, as well as the first large-scale biogeographic analysis of a species within the freshwater copepod genus Boeckella. Our data are consistent with previous phylogenetic analyses and ancestral geographic reconstructions of Boeckella in suggesting a South American origin of B. poppei, with subsequent colonization of the Maritime Antarctic (South Shetland Is., South Orkney Is., and the Antarctic Peninsula) and sub-Antarctic South Georgia during the mid-Pleistocene. Based on mtDNA and nucDNA sequences, we identified two distinct clades currently distributed in (A) the SOI and SSI and (B) SSI, AP, SG, and SA. Based on intraspecific phylogeographic and demographic inferences and ABC-based biogeographic scenarios, this finding suggested two separate and independent southwards colonization events across the Drake Passage. The first occurred during the mid-Pleistocene (clade A), while the second was a post-LGM event (clade B). Furthermore, the existence of two clades whose origin considerably predates the LGM, albeit assessed using a conservative molecular evolutionary rate, suggests the presence of at least two historical refugia within the present distribution of the species.
Long-term persistence of Boeckella poppei in the maritime Antarctic
The exclusive distribution of clade A in the SSI and SOI suggests that some populations have persisted in local refugia within these archipelagos through multiple glacial cycles within at least the second half of the Pleistocene and, given the HPD range, plausibly longer. This is not compatible with a hypothesis that contemporary populations south of the Antarctic Polar Front (APF) are derived from Holocene recolonization events from South America. Rather, they support the long-term persistence of B. poppei in the maritime Antarctic, adding further support to the recently-recognized and recurring pattern of long-term persistence across a range of Antarctic terrestrial invertebrate, plant and microbial biota (see Convey et al., 2020, and citations therein, Verleyen et al., 2021).
South Orkney Islands as a putative refugium
Based on the high genetic diversity, extended network topology, oldest demographic growth, and TMRCA of Antarctic clade A, we infer that refugia were present in the SOI. The biogeographic scenario of colonization of the SSI from SOI refugia was also supported by ABC analyses. The SOI are a group of relatively small islands located south of the APF, between 60°36′S 46°13′W and 60°41′S 44°18′W, and harbor the northern-most occurrence of clade A. Hodgson et al. (2014), based on analyses of sediment cores from extant lakes on the island, inferred a minimum deglaciation age for Signy Island of 7,292–7,517 YBP. They also suggested, in line with Clapperton (1990), that the SOI would have been covered by LGM and/or earlier ice expansion. Such glacial history would appear to prohibit the existence of freshwater bodies, although alternative habitats may still have been available on snow and ice surfaces, and glacial moraines, that could host freshwater communities (Fountain et al., 2004). Such habitats, including cryoconite holes, ice-based and moraine-edge melt pools, and smaller moraine ponds, are likely to have existed in summer even during glacial maxima, and B. poppei occurs in some of these forms of water bodies today. Another possibility is provided by epishelf lake habitats which are freshwater directly overlying seawater, or freshwater systems with a direct marine connection through a conduit underneath an ice shelf (Laybourn-Parry and Pearce, 2007). Two contemporary epishelf lakes at Beaver Lake in the Amery Oasis (East Antarctica) and Ablation Lake, Alexander Island, host B. poppei populations (Heywood, 1977; Bayly et al., 2003; Bissett et al., 2005). Since we were unable to obtain samples from this part of the species’ distribution, we cannot confirm that these epishelf lakes were indeed refuge sites. However, evidence from morphology (Bayly et al., 2003), sediments (Cromer et al., 2006; Gibson and Bayly, 2007), and molecular data (Bissett et al., 2005) all suggest the long-term presence of B. poppei in this region. The putative existence of other refugia in situ within continental Antarctica overturns previous suggestions of the extinction of inland biota in the continent during not only the most recent glaciation but also many previous glaciations in the area.
A parallel line of inference can be drawn from the apparent contradiction between terrestrial biological and glaciological evidence from Alexander Island, the world’s second largest island (after Greenland), located off the west coast of the Antarctic Peninsula at the southern limit of the Maritime Antarctic. Multiple lines of evidence supporting long-term terrestrial biological persistence, including remarkably high levels of diversity and endemism of the soil nematode fauna (Maslen and Convey, 2006), the presence of the endemic and apparently ancient springtail Friesea topo (Greenslade, 1995; Carapelli et al., 2020) and the presence of a basal clade of the Antarctic tardigrade genus Mesobiotus (Short et al., 2022) in terrestrial oases in south-eastern Alexander Island (71–72°S), strongly challenge current glaciological reconstructions, which do not support this area being refugial.
Post-glacial (re-)colonization from South America
Representatives of clade B spanned a broader distribution than clade A, including the AP, SSI, SG, and southern SA, suggesting a more complex history. Patagonian populations of B. poppei were characterized by high genetic diversity, extended network topology, an older TMRCA, and had no signal of past demographic growth. Such genetic and demographic patterns suggest that B. poppei in this region maintained a significant effective population size during at least the last glacial cycle despite the advances of the Patagonian Ice Sheet (PIS) over large portions of the western side of southern Patagonia (Clapperton, 1993; Davies et al., 2020). Large populations could have persisted in this region even during glacial maxima, probably in freshwater systems such as ice-dammed lakes formed by glacial over-deepening to the east, moraines, and marginal melt water channels (Sagredo et al., 2011; Lovell et al., 2012). In contrast, contemporary populations representing clade B located south of the APF (SG, SSI2, and AP) exhibited lower genetic diversity, star-like topologies, and evidence of post–LGM demographic expansion. Such patterns may reflect past contraction-expansion processes in response to climate oscillations, as frequently noted in phylogeographic studies of species affected by the LGM (Hewitt, 2004; Provan and Bennett, 2008; Marko et al., 2010). Such patterns of demographic expansion detected during the glaciation process could have followed either (1) a strong genetic bottleneck due to drastic reductions in population size in SG or other maritime Antarctic refugia during the last glacial period, and/or (2) a founder effect associated with post-glacial recolonization of SG and/or the maritime Antarctic from southern SA.
Based on ABC simulations of these two biogeographic scenarios for clade B, we suggest the most likely scenario is postglacial recolonization from southern SA to SG and the MA. Under this scenario, this clade of B. poppei would have dispersed and colonized sub- and maritime Antarctic regions during the Holocene through long-distance dispersal and establishment (LDDE, Crisp et al., 2011). The precise location of these colonization event(s) is unclear, but the pattern of haplotype distribution on the Antarctic Peninsula, including private alleles currently only known from this region (red haplotype network inset in Figure 2), along with the oldest MRCA across the members of clade B, suggest the southern Antarctic Peninsula as the most likely candidate. Furthermore, among the alternative biogeographic scenarios tested using ABC simulations, the Antarctic Peninsula was assessed as the most probable area for recolonization from southern SA (63.4%), although SG (25.4%) could not be rejected (Supplementary Table S8; Supplementary Figure S7).
Support for the hypothesis of recent Antarctic recolonization of clade B from southern SA might raise doubt over the scenario of much longer-term persistence of clade A in the South Orkney Islands. The possibility exists that haplotypes from Clade A (Figures 2, 3, respectively) were not sampled in southern SA. However, our sampling took place over 4 years, across multiple locations in southern Patagonia and the Falkland/Malvinas Islands, including remote locations such as Horn Island (Cape Horn archipelago) and the Diego Ramirez Islands. Among 100 visited SA sites, we successfully located B. poppei in only three locations close to Tierra del Fuego (Chilean Patagonia). Moreover, shared haplotypes among these sampling sites would suggest no missed diversity. This unexpectedly low presence in southern SA decreases the likelihood that representatives of Clade A are present but overlooked in this region, and supports the hypothesis of long-term persistence of MA populations in SOI refugia.
Long-distance dispersal and establishment
For both clades the biogeographic scenarios proposed here, including the geographic expansion from in situ SOI refugia to SSI1 for clade A and the colonization of SG, SSI2, and AP from southern SA for clade B, require LDDE. For physically isolated ecosystems such as those that host B. poppei, the two main LDDE vectors generally proposed are wind and birds (Figuerola and Green, 2002; Hawes et al., 2007; Viana et al., 2016). LDDE also requires that propagules have physiological features supporting dispersal, such as zooplankton eggs being more resistant to harsh environmental conditions than their active forms in the water column (Marcus, 1996; Brendonck and de Meester, 2003). In B. poppei, the small resistant eggs (150.3 ± 8.2 μm) reported in the maritime Antarctic, which may provide an overwintering strategy (Jiang et al., 2012; Reed et al., 2021), can also survive desiccation and low temperature, thereby raising the possibility of dispersal over long distances, such as across the Drake Passage (~900 km) separating southern SA from the SSI.
Wind has often been invoked as a dispersal mechanism shaping plant and animal distributions in the Southern Hemisphere (Marshall and Convey, 1997; Muñoz et al., 2004; Hawes, 2009; Biersma et al., 2018a; Bohuslavová et al., 2018; McGaughran et al., 2019; Vega et al., 2019). The prevailing westerly wind systems at higher southern latitudes have been suggested to transport pollen and small moss propagules from southern SA into the MA (Biersma et al., 2018a). The hypothesis that resistant eggs of B. poppei could have achieved multiple long-distance colonization events through wind-related dispersal events is therefore plausible.
The native avifauna of the MA includes primarily seabirds belonging to the families Charadriidae (e.g., skuas and gulls) and Procellaridae (e.g., albatrosses, petrels, prions, fulmars, shearwaters), with vagrant species from SA also being regularly noted. Among these, the two skua species that occur in Antarctic regions (Catharacta maccormicki and C. antarctica lonnbergi) and the kelp gull (Larus dominicanus), spend much of their time on land, including frequenting freshwater bodies, both in the Antarctic and in the northerly parts of their migratory routes. Therefore, the inland behaviors of these species increase the chance of them being candidate vectors of long-distance dispersal for B. poppei at inter- and/or intracontinental scale.
Possible constraints and passages to new colonizers
Despite the existence of potential vectors, the strong phylogeographic structure and low number (clade A) or absence (clade B) of shared haplotypes between putative refugia and colonized areas suggest that these dispersal mechanisms do not permit connections with distant populations through regular gene flow but, rather, occur as extremely infrequent dispersal events. Alternatively, the strong genetic and phylogeographic structure may suggest the existence of effective constraints limiting the establishment of new colonizing individuals caused by the monopolization of resources and rapid local adaptation by the early arriving individuals: the “Monopolization Hypothesis” (De Meester et al., 2002; Orsini et al., 2013). This hypothesis provides a theoretical basis to explain the apparent paradox between high passive dispersal capacity and reduced gene flow among neighboring populations in freshwater communities (Gomez et al., 2000; Xu et al., 2009; Ventura et al., 2014; Maturana et al., 2020; Ortega-Mayagoitia et al., 2022). The distribution of clades A and B is consistent with this hypothesis, since individuals representing each clade generally do not occur in sympatry. As supported by our biogeographic scenario analyses, in Clade B SA individuals would have colonized SG, SSI and PA, but not SOI. The existence of a well-adapted resident population of B. poppei in the latter archipelago facilitating rapid local colonization of new suitable bodies (Maturana et al., 2020), would result in a combination of founder effect, rapid local adaptation, and monopolization of available resources, reducing the establishment success of any subsequent immigrants from SA. However, an alternative explanation of mismatch between phenotype (e.g., physiology, phenology, or life-history traits) and ecological conditions (e.g., chemical composition) of accessible lakes (Ortega-Mayagoitia et al., 2022) is also possible. While both clades A and B were found in the SSI, at finer scales the three lakes on Deception Island and one on Greenwich Island only hosted clade A while only clade B was found in two lakes on Livingston Island (see Supplementary Table S1; Supplementary Figure S3). On King George Island, among 10 sampling sites, two hosted only Clade A and six Clade B, with only two lakes hosting both clades. Suggestively, the latter two sites were located close to scientific stations and hence more vulnerable to human influence: Escudero (Fildes Bay) and Arctowski (Admiralty Bay). While there is no direct evidence of anthropogenic impacts on biodiversity in these lakes, there are increasing reports of negative impacts of human activity, almost exclusively in the vicinity of research stations and visitor sites (Hughes and Convey, 2010; Pertierra et al., 2019). Further, even though human–mediated movement of regionally non–indigenous species is the most recognized problem, the redistribution of indigenous Antarctic species between biologically distinct areas within the continent may also occur, which risks changing their fragile terrestrial and freshwater ecosystems irreversibly (Frenot et al., 2005; Convey et al., 2006).
Conclusion
We critically assessed two biogeographic hypotheses, namely in situ persistence and postglacial (re)colonization, that have been proposed to explain how Quaternary events have shaped the distribution, demography, and genetic structure of Antarctic terrestrial and freshwater biota. Boeckella poppei Clade A, found only in the maritime Antarctic, provided evidence of long-term persistence through multiple glacial cycles since at least the mid-Pleistocene, with a potential glacial refugial location in the South Orkney Islands from which the South Shetland Islands were then colonized. Our study provides the first report suggesting persistence through Pleistocene glacial cycles for a freshwater invertebrate in the maritime Antarctic, broadening the scope of conclusions of recent studies limited to terrestrial ecosystems. Clade B, with a much wider distribution, led us to infer exceptional and extremely infrequent LDDE event(s) from southern South America. Together, these findings reveal the complex biogeographic history of this Antarctic freshwater copepod and reconcile both in situ persistence and postglacial (re)colonization hypotheses.
Data availability statement
Cox1 haplotypes sequences of Boeckella poppei are available in GenBank under accession numbers (OP547785 – OP547859). For 28S rRNA and ITS, the haplotypes sequences are deposited in GenBank under accession numbers (OP547502 – OP547503) and (OP547515 – OP547520), respectively. Previously published data source cited in Methods section is available at https://doi.org/10.5281/zenodo.477909. New additional data cited across the manuscript are uploaded in Supplementary material. The occurrence dataset of Boeckella poppei across the Antarctic and sub-Antarctic regions is available from the GBIF repository: https://www.gbif.org/es/dataset/474ee54a-9eae-4b67-b79b-d8fc2d9ec884.
Author contributions
CM, EP, and PC conceived the idea and designed the analyses. CM performed the taxonomic revision and molecular work, phylogenetic and biogeographic analyses. CM, EP, PC, AD, EB, JJ, and TC conducted fieldwork. CM and TC designed and edited figures and maps. EB, EP, PC, JJ, and CG-W contributed to every analytical step interpreting the results and in preparing the manuscript. CM drafted the paper. EP, JJ, PC, EB, AD, CG-W, and TC discussed the results and contributed to the final manuscript. All authors contributed to the article and approved the submitted version.
Funding
This work was funded by ANID—Millennium Science Initiative Program—ICN2021_002, ANID/BASAL FB210018 from Cape Horn International Centre (CHIC), and Grant ANID ACE210006 from the Institute of Ecology and Biodiversity (IEB). Postdoctoral grant 3210063 to CSM and NERC core funding to the BAS ‘Ecosystems’ and ‘Biodiversity, Evolution and Adaptation’ Teams to JJ, PC, and EB. EB was also funded by NERC-CONICYT grant NE/P003079/1 and Carlsberg Foundation grant CF18-0267.
Acknowledgments
We recognize the Chilean Antarctic Institute (INACH) for the logistic support during the Chilean Antarctic Expeditions (ECA 53, 54, and 55), the South Atlantic Environmental Research Institute (SAERI) for their logistic support of sampling in the Falkland/Malvinas Islands and South Georgia, and Laboratorio de Ecosistemas Marinos Antárticos y Subantárticos (LEMAS) for their constant support of our fieldwork in Punta Arenas and Tierra del Fuego. Finally, CSM would like to particularly thank Moisés Valladares, Zambra López, Karin Gérard, and Sebastian Rosenfeld for valuable sampling, discussion, and support in the development of this study.
Conflict of interest
The authors declare that the research was conducted in the absence of any commercial or financial relationships that could be construed as a potential conflict of interest.
Publisher’s note
All claims expressed in this article are solely those of the authors and do not necessarily represent those of their affiliated organizations, or those of the publisher, the editors and the reviewers. Any product that may be evaluated in this article, or claim that may be made by its manufacturer, is not guaranteed or endorsed by the publisher.
Supplementary material
The Supplementary material for this article can be found online at: https://www.frontiersin.org/articless/10.3389/fevo.2022.1012852/full#supplementary-material
Footnotes
References
Allegrucci, G., Carchini, G., Convey, P., and Sbordoni, V. (2012). Evolutionary geographic relationships among orthocladine chironomid midges from marine Antarctic and sub-Antarctic islands. Biol. J. Linn. Soc. 106, 258–274. doi: 10.1111/J.1095-8312.2012.01864.X
Almada, P., Allende, L., Tell, G., and Izaguirre, I. (2004). Experimental evidence of the grazing impact of Boeckella poppei on phytoplankton in a maritime Antarctic lake. Polar Biol. 28, 39–46. doi: 10.1007/S00300-004-0644-Y
Avise, J. C., and Ayala, F. J. (2017). Comparative Phylogeography. Washington, DC: The National Academies Press.
Baird, H. P., Shin, S., Oberprieler, R. G., Hulle, M., Vernon, P., Moon, K. L., et al. (2021). Fifty million years of beetle evolution along the Antarctic Polar Front. Proc. Natl. Acad. Sci. U. S. A. 118, 1–10. doi: 10.1073/Pnas.2017384118
Bandelt, H. J., Forster, P., and Rohl, A. (1999). Median-joining networks for inferring intraspecific phylogenies. Mol. Biol. Evol. 16, 37–48. doi: 10.1093/Oxfordjournals.Molbev.A026036
Bayly, I. A. E., Gibson, J. A. E., Wagner, B., and Swadling, K. M. (2003). Taxonomy, ecology and zoogeography of two east Antarctic freshwater calanoid copepod species: Boeckella poppei and Gladioferens antarcticus. Antarct. Sci. 15, 439–448. doi: 10.1017/S0954102003001548
Beaumont, M. A., Zhang, W., and Balding, D. J. (2002). Approximate Bayesian computation in population genetics. Genetics 162, 2025–2035. doi: 10.1093/genetics/162.4.2025
Benjamini, Y., Krieger, A. M., and Yekutieli, D. (2005). Adaptive linear step-up procedures that control the false discovery rate. Biometrika 93, 491–507. doi: 10.1093/biomet/93.3.491
Bennet, K. D., Tzedakis, P. C., and Willis, K. J. (1991). Quaternary refugia of north European trees. J. Biogeogr. 18, 103–115. doi: 10.2307/2845248
Biersma, E. M., Convey, P., Wyber, R., Robinson, S. A., Dowton, M., van de Vijver, B., et al. (2020a). Latitudinal biogeographic structuring in the globally distributed moss Ceratodon purpureus. Front. Plant Sci. 11:502359. doi: 10.3389/Fpls.2020.502359
Biersma, E. M., Jackson, J. A., Bracegirdle, T. J., Griffiths, H., Linse, K., and Convey, P. (2018a). Low genetic variation between South American and Antarctic populations of the bank-forming moss Chorisodontium aciphyllum (Dicranaceae). Polar Biol. 41, 599–610. doi: 10.1007/S00300-017-2221-1
Biersma, E. M., Jackson, J. A., Stech, M., Griffiths, H., Linse, K., and Convey, P. (2018b). Molecular data duggest long-term in situ Antarctic persistence within Antarctica's most speciose plant genus, Schistidium. Front. Ecol. Evol. 6:77. doi: 10.3389/Fevo.2018.00077
Biersma, E. M., Torres-Diaz, C., Molina-Montenegro, M. A., Newsham, K. K., Vidal, M. A., Collado, G. A., et al. (2020b). Multiple late-Pleistocene colonisation events of the Antarctic pearlwort Colobanthus quitensis (Caryophyllaceae) reveal the recent arrival of native Antarctic vascular flora. J. Biogeogr. 47, 1663–1673. doi: 10.1111/Jbi.13843
Billard, E., Reyes, J., Mansilla, A., Faugeron, S., and Guillemin, M.-L. (2015). Deep genetic divergence between austral populations of the red alga Gigartina skottsbergii reveals a cryptic species endemic to the Antarctic continent. Polar Biol. 38, 2021–2034. doi: 10.1007/S00300-015-1762-4
Bissett, A., Gibson, J. A. E., Jarman, S. N., Swadling, K. M., and Cromer, L. (2005). Isolation, amplification, and identification of ancient copepod DNA from lake sediments. Limnology Oceanography-Methods 3, 533–542. doi: 10.4319/Lom.2005.3.533
Bohuslavová, O., Macek, P., Redčenko, O., Láska, K., Nedbalová, L., and Elster, J. (2018). Dispersal of lichens along a successional gradient after deglaciation of volcanic mesas on northern James Ross Island, Antarctic Peninsula. Polar Biol. 41, 2221–2232. doi: 10.1007/S00300-018-2357-7
Bouckaert, R., Heled, J., Kuhnert, D., Vaughan, T., Wu, C. H., Xie, D., et al. (2014). Beast 2: a software platform for Bayesian evolutionary analysis. PloS Comp. Biol. 10:E1003537. doi: 10.1371/Journal.Pcbi.1003537
Brendonck, L., and De Meester, L. (2003). Egg banks in freshwater zooplankton: evolutionary and ecological archives in the sediment. Hydrobiologia 491, 65–84. doi: 10.1023/A:1024454905119
Brooks, S. T., Jabour, J., Van Den Hoff, J., and Bergstrom, D. M. (2019). Our footprint on Antarctica competes with nature for rare ice-free land. Nature Sustainability 2, 185–190. doi: 10.1038/S41893-019-0237-Y
Brunetti, C., Siepel, H., Convey, P., Fanciulli, P. P., Nardi, F., and Carapelli, A. (2021). Overlooked species diversity and distribution in the Antarctic mite genus Stereotydeus. Diversity 13, 1–26. doi: 10.3390/D13100506
Burridge, C. P., Craw, D., Fletcher, D., and Waters, J. M. (2008). Geological dates and molecular rates: fish DNA sheds light on time dependency. Mol. Biol. Evol. 25, 624–633. doi: 10.1093/Molbev/Msm271
Burton-Johnson, A., Black, M., Fretwell, P. T., and Kaluza-Gilbert, J. (2016). An automated methodology for differentiating rock from snow, clouds and sea in Antarctica from Landsat 8 imagery: a new rock outcrop map and area estimation for the entire Antarctic continent. Cryosphere 10, 1665–1677. doi: 10.5194/tc-10-1665-2016
Cabrera, V. M. (2021). Human molecular evolutionary rate, time dependency and transient polymorphism effects viewed through ancient and modern mitochondrial DNA genomes. Sci. Rep. 11:5036. doi: 10.1038/S41598-021-84583-1
Carapelli, A., Convey, P., Frati, F., Spinsanti, G., and Fanciulli, P. P. (2017). Population genetics of three sympatric springtail species (Hexapoda: Collembola) from the South Shetland Islands: evidence for a common biogeographic pattern. Biol. J. Linn. Soc. 120, 788–803. doi: 10.1093/Biolinnean/Blw004
Carapelli, A., Cucini, C., Fanciulli, P. P., Frati, F., Convey, P., and Nardi, F. (2020). Molecular comparison among Three Antarctic Endemic Springtail Species and Description of the Mitochondrial Genome of Friesea Gretae (Hexapoda, Collembola). Diversity 12, 1–13. doi: 10.3390/D12120450
Chown, S. L., and Convey, P. (2016). Antarctic Entomology. Annu. Rev. Entomol. 61, 119–137. doi: 10.1146/Annurev-Ento-010715-023537
Clapperton, C. M. (1990). Quaternary glaciation in the Southern Ocean and Antarctic Peninsula area. Quat. Sci. Rev. 9, 229–252. doi: 10.1016/0277-3791(90)90020-B
Clapperton, C. M. (1993). Nature of environmental changes in South America at the Last Glacial Maximum. Palaeogeogr., Palaeoclimatol. Palaeoecol. 101, 189–208. doi: 10.1016/0031-0182(93)90012-8
Convey, P. (2017). “Antarctic Ecosystems” in Reference Module in Life Science (Cambridge: Elsevier), 179–188.
Convey, P., Biersma, E. M., Casanova-Katny, A., and Maturana, C. (2020). “Refuges of Antarctic diversity” in Past Antarctica. eds. M. Oliva and J. Ruiz-Fernandez (Elsevier), 181–200.
Convey, P., Frenot, Y., Gremmen, N., and Bergstrom, D. M. (2006). “Biological invasions” in Trends in Antarctic Terrestrial and Limnetic Ecosystems: Antarctica as a Global Indicator. eds. D. M. Bergstrom, P. Convey, and A. H. L. Huiskes (Dordhrecht: Springer), 193–220.
Convey, P., and Smith, R. I. L. (2006). Geothermal bryophyte habitats in the South Sandwich Islands, maritime Antarctic. J. Veg. Sci. 17, 529–538. doi: 10.1111/j.1654-1103.2006.tb02474.x
Copilaş-Ciocianu, D., Sidorov, D., and Gontcharov, A. (2019). Adrift across tectonic plates: molecular phylogenetics supports the ancient Laurasian origin of old limnic crangonyctid amphipods. Org. Diversity Evol. 19, 191–207. doi: 10.1007/S13127-019-00401-7
Cornuet, J. M., Santos, F., Beaumont, M. A., Robert, C. P., Marin, J. M., Balding, D. J., et al. (2008). Inferring population history with DIY ABC: a user-friendly approach to approximate Bayesian computation. Bioinformatics 24, 2713–2719. doi: 10.1093/Bioinformatics/Btn514
Crisp, M. D., Trewick, S. A., and Cook, L. G. (2011). Hypothesis testing in biogeography. Trends Ecol. Evol. 26, 66–72. doi: 10.1016/J.Tree.2010.11.005
Cromer, L., Gibson, J. A. E., Swadling, K. M., and Hodgson, D. A. (2006). Evidence for a lacustrine faunal refuge in the Larsemann Hills, East Antarctica, during the Last Glacial Maximum. J. Biogeogr. 33, 1314–1323. doi: 10.1111/J.1365-2699.2006.01490.X
Davies, B. J., Darvill, C. M., Lovell, H., Bendle, J. M., Dowdeswell, J. A., Fabel, D., et al. (2020). The evolution of the Patagonian Ice Sheet from 35 ka to the present day (PATICE). Earth-Sci. Rev. 204:103152. doi: 10.1016/J.Earscirev.2020.103152
De Meester, L., Gómez, A., Okamura, B., and Schwenk, K. (2002). The Monopolization Hypothesis and the dispersal-gene flow paradox in aquatic organism. Acta Oecol. 23, 121–135. doi: 10.1016/S1146-609X(02)01145-1
Diaz, A., Gerard, K., Gonzalez-Wevar, C., Maturana, C., Feral, J. P., David, B., et al. (2018). Genetic structure and demographic inference of the regular sea urchin Sterechinus neumayeri (Meissner, 1900) in the Southern Ocean: The role of the last glaciation. PLoS One 13:E0197611. doi: 10.1371/Journal.Pone.0197611
Edgar, R. C. (2004). MUSCLE: a multiple sequence alignment method with reduced time and space complexity. BMC Bioinformatics 5, 1–19. doi: 10.1186/1471-2105-5-113
Emerson, B. C., and Hickerson, M. J. (2015). Lack of support for the time-dependent molecular evolution hypothesis. Mol. Ecol. 24, 702–709. doi: 10.1111/mec.13070
Excoffier, L., and Heckel, G. (2006). Computer programs for population genetics data analysis: a survival guide. Nat. Rev. Genet. 7, 745–758. doi: 10.1038/Nrg1904
Fasanella, M., Premoli, A. C., Urdampilleta, J. D., Gonzalez, M. L., and Chiapella, J. O. (2017). How did a grass reach Antarctica? The Patagonian connection of Deschampsia antarctica (Poaceae). Botanical J. Linnean Society 185, 511–524. doi: 10.1093/botlinnean/box070
Felsenstein, J. (1981). Evolutionary trees from DNA sequences: a maximum likelihood approach. J. Mol. Evol. 17, 368–376. doi: 10.1007/BF01734359
Figuerola, J., and Green, A. J. (2002). Dispersal of aquatic organisms by waterbirds: a review of past research and priorities for future studies. Freshwat. Biol. 47, 483–494. doi: 10.1046/j.1365-2427.2002.00829.x
Fountain, A. G., Tranter, M., Nylen, T. H., Lewis, K. J., and Mueller, D. R. (2004). Evolution of cryoconite holes and their contribution to meltwater runoff from glaciers in the McMurdo Dry Valleys, Antarctica. J. Glaciol. 50, 35–45. doi: 10.3189/172756504781830312
Fraser, C. I., Terauds, A., Smellie, J., Convey, P., and Chown, S. L. (2014). Geothermal activity helps life survive glacial cycles. Proc. Natl. Acad. Sci. U. S. A. 111, 5634–5639. doi: 10.1073/Pnas.1321437111
Frenot, Y., Chown, S. L., Whinam, J., Selkirk, P. M., Convey, P., Skotnicki, M., et al. (2005). Biological invasions in the Antarctic: extent, impacts and implications. Biol. Rev. Camb. Philos. Soc. 80, 45–72. doi: 10.1017/S1464793104006542
Gersonde, R., Crosta, X., Abelmann, A., and Armand, L. (2005). Sea-surface temperature and sea ice distribution of the Southern Ocean at the EPILOG Last Glacial Maximum—a circum-Antarctic view based on siliceous microfossil records. Quat. Sci. Rev. 24, 869–896. doi: 10.1016/J.Quascirev.2004.07.015
Gibson, J. A. E., and Bayly, I. A. E. (2007). New insights into the origins of crustaceans of Antarctic lakes. Antarct. Sci. 19, 157–163.
Gibson, J. A., and Zale, R. (2006). Holocene development of the fauna of Lake Boeckella, northern Antarctic Peninsula. Holocene 16, 625–634. doi: 10.1191/0959683606hl959rp
Gomez, A., Carvalho, G., and Lunt, D. (2000). Phylogeography and regional endemism of a passively dispersing zooplankter: mtDNA variation of rotifer resting egg banks. Proc. Royal Soc. Lond. B 267, 2189–2197. doi: 10.1098/rspb.2000.1268
González-Wevar, C. A., David, B., and Poulin, E. (2011). Phylogeography and demographic inference in Nacella (Patinigera) concinna (Strebel, 1908) in the western Antarctic peninsula. Deep-Sea Res. II Top. Stud. Oceanogr. 58, 220–229. doi: 10.1016/J.Dsr2.2010.05.026
Greenslade, P. (1995). Collembola from the Scotia Arc and Antarctic peninsula including descriptions of two new species and notes on biogeography. Bulletin Entomologique de Pologne 64, 305–319.
Guindon, S., Dufayard, J. F., Lefort, V., Anisimova, M., Hordijk, W., and Gascuel, O. (2010). New algorithms and methods to estimate maximum-likelihood phylogenies: assessing the performance of PhyML 3.0. Syst. Biol. 59, 307–321. doi: 10.1093/Sysbio/Syq010
Hassold, N. J. C., Rea, D. K., Van Der Pluijm, B. A., and Parés, J. M. (2009). A physical record of the Antarctic Circumpolar Current: Late Miocene to recent slowing of abyssal circulation. Palaeogeogr. Palaeoclimatol. Palaeoecol. 275, 28–36. doi: 10.1016/J.Palaeo.2009.01.011
Hawes, T. C. (2009). Origins and dispersal of the Antarctic fairy shrimp. Antarct. Sci. 21, 477–482. doi: 10.1017/S095410200900203x
Hawes, T. C., Worland, M. R., Convey, P., and Bale, J. S. (2007). Aerial dispersal of springtails on the Antarctic Peninsula: implications for local distribution and demography. Antarct. Sci. 19, 3–10. doi: 10.1017/S0954102007000028
Hewitt, G. M. (2004). Genetic consequences of climatic oscillations in the Quaternary. Philos. Trans. R. Soc. Lond. Ser. B Biol. Sci. 359, 183–195. doi: 10.1098/Rstb.2003.1388
Heywood, R. B. (1970). Ecology of the freshwater lakes of Signy Island, South Orkney Island: III Biology of the copepod Pseudoboeckella silvestri Daday (Calanoida, Centropagidae). Br. Antarct. Surv. Bull. 23, 1–17.
Heywood, R. B. (1977). A limnological survey of the Ablation Point area, Alexander Island, Antartica. Philos. Trans. R. Society Series B 279, 39–54.
Ho, S. Y., Duchene, S., Molak, M., and Shapiro, B. (2015). Time-dependent estimates of molecular evolutionary rates: evidence and causes. Mol. Ecol. 24, 6007–6012. doi: 10.1111/mec.13450
Ho, S. Y., Lanfear, R., Bromham, L., Phillips, M. J., Soubrier, J., Rodrigo, A. G., et al. (2011). Time-dependent rates of molecular evolution. Mol. Ecol. 20, 3087–3101. doi: 10.1111/J.1365-294x.2011.05178.X
Ho, S. Y., Saarma, U., Barnett, R., Haile, J., and Shapiro, B. (2008). The effect of inappropriate calibration: three case studies in molecular ecology. PLoS One 3:E1615. doi: 10.1371/Journal.Pone.0001615
Hodgson, D. A., Graham, A. G. C., Roberts, S. J., Bentley, M. J., Cofaigh, C. Ó., Verleyen, E., et al. (2014). Terrestrial and submarine evidence for the extent and timing of the Last Glacial Maximum and the onset of deglaciation on the maritime-Antarctic and sub-Antarctic islands. Quat. Sci. Rev. 100, 137–158. doi: 10.1016/j.quascirev.2013.12.001
Hodgson, D. A., Verleyen, E., Sabbe, K., Squier, A. H., Keely, B. J., Leng, M. J., et al. (2005). Late Quaternary climate-driven environmental change in the Larsemann Hills, East Antarctica, multi-proxy evidence from a Lake sediment Core. Quat. Res. 64, 83–99. doi: 10.1016/J.Yqres.2005.04.002
Hughes, K. A., and Convey, P. (2010). The protection of Antarctic terrestrial ecosystems from inter-and intra-continental transfer of non-indigenous species by human activities: a review of current systems and practices. Glob. Environ. Chang. 20, 96–112. doi: 10.1016/J.Gloenvcha.2009.09.005
Jiang, X., Zhao, S., Xu, Z., Wang, G., He, J., and Cai, M. (2012). Abundance and age of viable resting eggs of the calanoid copepod Boeckella poppei Mrázek in sediments: evidence of egg banks in two Antarctic maritime lakes. Polar Biol. 35, 1525–1531. doi: 10.1007/s00300-012-1192-5
Jones, V. J., Hodgson, D. A., and Lusty-Chepstow, A. (2000). Palaeolimnological evidence for marked Holocene environmental changes on Signy Island, Antarctica. Holocene 10, 43–60. doi: 10.1191/095968300673046662
Kearse, M., Moir, R., Wilson, A., Stones-Havas, S., Cheung, M., Sturrock, S., et al. (2012). Geneious basic: an integrated and extendable desktop software platform for the organization and analysis of sequence data. Bioinformatics 28, 1647–1649. doi: 10.1093/bioinformatics/bts199
Knowlton, N., and Weight, L. A. (1998). New dates and new rates for divergence across the Isthmus of Panama. Proc. R. Soc. Lond. Ser. B Biol. Sci. 265, 2257–2263. doi: 10.1098/rspb.1998.0568
Kumar, S., Stecher, G., Li, M., Knyaz, C., Tamura, K., and Battistuzzi, F. U. (2018). MEGA X: molecular evolutionary genetics analysis across computing platforms. Mol. Biol. Evol. 35, 1547–1549. doi: 10.1093/Molbev/Msy096
Lanfear, R., Frandsen, P. B., Wright, A. M., Senfeld, T., and Calcott, B. (2017). Partitionfinder 2: new methods for selecting partitioned models of evolution for molecular and morphological phylogenetic analyses. Mol. Biol. Evol. 34, 772–773. doi: 10.1093/Molbev/Msw260
Laybourn-Parry, J., and Pearce, D. A. (2007). The biodiversity and ecology of Antarctic lakes: models for evolution. Philos. Trans. R. Soc. Lond., Ser. B: Biol. Sci. 362, 2273–2289. doi: 10.1098/Rstb.2006.1945
Lessios, H. A. (2008). The Great American Schism: divergence of marine organisms after the rise of Central American Isthmus. Annu. Rev. Ecol. Syst. 39, 63–91. doi: 10.1146/annurev.ecolsys.38.091206.095815
Librado, P., and Rozas, J. (2009). DnaSP V5: a software for comprehensive analysis of DNA polymorphism data. Bioinformatics 25, 1451–1452. doi: 10.1093/bioinformatics/btp187
Lovell, H., Stokes, C. R., Bentley, M. J., and Benn, D. I. (2012). Evidence for rapid ice flow and proglacial lake evolution around the central strait of Magellan region, southernmost Patagonia. J. Quat. Sci. 27, 625–638. doi: 10.1002/Jqs.2555
Maggs, C. A., Castilho, R., Foltz, D., Henzler, C., Jolly, M. T., and Kelly, J. (2008). Evaluating signature of glacial refugia for north Atlantic benthic marine taxa. Ecology 89, S108–S122. doi: 10.1890/08-0257.1
Maly, E. J., and Bayly, I. A. E. (1991). Factors influencing biogeographic patterns of Australasian centropagid copepods. J. Biogeogr. 18, 455–461. doi: 10.2307/2845486
Marcus, N. H. (1996). Ecological and evolutionary significance of resting eggs in marine copepods: past, present, and future studies. Hydrobiologia 320, 141–152. doi: 10.1007/BF00016815
Marko, P. B., Hoffman, J. I., Emme, S. A., Mcgovern, T. M., Keever, C. C., and Cox, L. N. (2010). The ‘Expansion–Contraction’ model of Pleistocene biogeography: rocky shores suffer a sea change? Mol. Ecol. 19, 146–169. doi: 10.1111/J.1365-294x.2009.04417.X
Marshall, A. W., and Convey, P. (1997). Dispersal of moss propagules on Signy Island, Maritime Antarctic. Polar Biol. 18, 376–383. doi: 10.1007/s003000050203
Maslen, N. R., and Convey, P. (2006). Nematode diversity and distribution in the southern maritime Antarctic—clues to history? Soil Biol. Biochem. 38, 3141–3151. doi: 10.1016/j.soilbio.2005.12.007
Maturana, C. S., Rosenfeld, S., Biersma, E. M., Segovia, N. I., González-Wevar, C. A., Díaz, A., et al. (2021). Historical biogeography of the Gondwanan freshwater genus Boeckella (Crustacea): timing and modes of speciation in the Southern Hemisphere. Divers. Distrib. 27, 2330–2343. doi: 10.1111/Ddi.13405
Maturana, C. S., Rosenfeld, S., Naretto, J., Convey, P., and Poulin, E. (2019). Distribution of the genus Boeckella (Crustacea, Copepoda, Calanoida, Centropagidae) at high latitudes in South America and the main Antarctic biogeographic regions. Zookeys 854, 1–15. doi: 10.3897/Zookeys.854.29614
Maturana, C. S., Segovia, N. I., González-Wevar, C. A., Díaz, A., Rosenfeld, S., Poulin, E., et al. (2020). Evidence of strong small-scale population structure in the Antarctic freshwater copepod Boeckella poppei in lakes on Signy Island, South Orkney Islands. Limnol. Oceanogr 65, 2024–2040. doi: 10.1002/lno.11435
Mcgaughran, A., Terauds, A., Convey, P., and Fraser, C. I. (2019). Genome-wide SNP data reveal improved evidence for Antarctic glacial refugia and dispersal of terrestrial invertebrates. Mol. Ecol. 28, 4941–4957. doi: 10.1111/Mec.15269
Mcgaughran, A., Torricelli, G., Carapelli, A., Frati, F., Stevens, M. I., Convey, P., et al. (2010). Contrasting phylogeographical patterns for springtails reflect different evolutionary histories between the Antarctic Peninsula and continental Antarctica. J. Biogeogr. 37, 103–119. doi: 10.1111/J.1365-2699.2009.02178.X
Mortimer, E., Jansen Van Vuuren, B., Lee, J. E., Marshall, D. J., Convey, P., and Chown, S. L. (2011). Mite dispersal among the Southern Ocean Islands and Antarctica before the last glacial maximum. Proc. Biol. Sci. 278, 1247–1255. doi: 10.1098/Rspb.2010.1779
Muñoz, J., Felicísimo, A. M., Cabezas, F., Burgaz, A. R., and Martínez, I. (2004). Wind as a long-distance dispersal vehicle in the Southern Hemisphere. Science 304, 1144–1147. doi: 10.1126/science.1095210
Ney, G., Frederick, K., and Schul, J. (2018). A post-pleistocene calibrated mutation rate from insect museum specimens. Plos Curr 10, 1–20. doi: 10.1371/Currents.Tol.Aba557de56be881793261f7e1565cf35
Orsini, L., Vanoverbeke, J., Swillen, I., Mergeay, J., and De Meester, L. (2013). Drivers of population genetic differentiation in the wild: isolation by dispersal limitation, isolation by adaptation and isolation by colonization. Mol. Ecol. 22, 5983–5999. doi: 10.1111/Mec.12561
Ortega-Mayagoitia, E., Alcántara-Rodríguez, J. A., Urbán-Olivares, J., Campos, J. E., and Ciros-Pérez, J. (2022). Genomic signatures of adaptive divergence in lacustrine copepods. Freshwat. Biol. 67, 1045–1062. doi: 10.1111/Fwb.13900
Pérez-Alvarez, M., Kraft, S., Segovia, N. I., Olavarría, C., Nigenda-Morales, S., Urbán, R. J., et al. (2021). Contrasting Phylogeographic Patterns Among Northern and Southern Hemisphere Fin Whale Populations With New Data From the Southern Pacific. Front. Mar. Sci. 8:630233. doi: 10.3389/Fmars.2021.630233
Pertierra, L. R., Bartlett, J. C., Duffy, G. A., Vega, G. C., Hughes, K. A., Hayward, S. A. L., et al. (2019). Combining correlative and mechanistic niche models with human activity data to elucidate the invasive potential of a sub-Antarctic insect. J. Biogeogr. 47, 658–673. doi: 10.1111/Jbi.13780
Pisa, S., Biersma, E. M., Convey, P., Patiño, J., Vanderpoorten, A., Werner, O., et al. (2014). The cosmopolitan moss Bryum argenteum in Antarctica: recent colonisation or in situ survival? Polar Biol. 37, 1469–1477. doi: 10.1007/S00300-014-1537-3
Provan, J., and Bennett, K. D. (2008). Phylogeographic insights into cryptic glacial refugia. Trends Ecol. Evol. 23, 564–571. doi: 10.1016/J.Tree.2008.06.010
Pugh, P. J. A., Dartnall, H. J. G., and Mcinnes, S. J. (2002). The non-marine Crustacea of Antarctica and the islands of the Southern Ocean: biodiversity and biogeography. J. Nat. Hist. 36, 1047–1103. doi: 10.1080/00222930110039602
Rambaut, A., Suchard, M. A., Xie, D., and Drummond, A. J. (2014). Tracer v1.6. Available at: http://Beast.Bio.Ed.Ac.Uk/Tracer
Reed, K. A., Lee, S. G., Lee, J. H., Park, H., and Covi, J. A. (2021). The ultrastructure of resurrection: Post-diapause development in an Antarctic freshwater copepod. J. Struct. Biol. 213:107705. doi: 10.1016/J.Jsb.2021.107705
Ronquist, F., and Huelsenbeck, J. P. (2003). MrBayes 3: Bayesian phylogenetic inference under mixed models. Bioinformatics 19, 1572–1574. doi: 10.1093/Bioinformatics/Btg180
Russel, P. M., Brewer, B. J., Klaere, S., and Bouckaert, R. R. (2019). Model selection and parameter inference in phylogenetics using nested sampling. Syst. Biol. 68, 219–233. doi: 10.1093/Sysbio/Syy050
Sagredo, E. A., Moreno, P. I., Villa-Martínez, R., Kaplan, M. R., Kubik, P. W., and Stern, C. R. (2011). Fluctuations of the Última Esperanza ice lobe (52°S), Chilean Patagonia, during the last glacial maximum and termination 1. Geomorphology 125, 92–108. doi: 10.1016/J.Geomorph.2010.09.007
Scheihing, R., Cardenas, L., Nespolo, R. F., Krall, P., Walz, K., Kohshima, S., et al. (2009). Morphological and molecular analysis of centropagids from the high Andean plateau (Copepoda: Calanoidea). Hydrobiologia 637, 45–52. doi: 10.1007/S10750-009-9983-6
Short, K. A., Sands, C. J., Mcinnes, S. J., Pisani, D., Stevens, M. I., and Convey, P. (2022). An ancient, Antarctic-specific species complex: large divergences between multiple Antarctic lineages of the tardigrade genus Mesobiotus. Mol. Phylogenet. Evol. 170:107429. doi: 10.1016/J.Ympev.2022.107429
Stephens, M., and Donnelly, P. (2003). A comparison of bayesian methods for haplotype reconstruction from population genotype data. Am. J. Hum. Genet. 73, 1162–1169. doi: 10.1086/379378
Stevens, M. I., and Hogg, I. D. (2003). Long-term isolation and recent range expansion from glacial refugia revealed for the endemic springtail Gomphiocephalus hodgsoni from Victoria land. Antarctica. Mol. Ecol. 12, 2357–2369. doi: 10.1046/j.1365-294X.2003.01907.x
Taberlet, P., Fumagalli, L., Wust-Saucy, A.-G., and Cosson, J.-F. (1998). Comparative phylogeography and postglacial colonization routes in Europe. Mol. Ecol. 7, 453–464. doi: 10.1046/J.1365-294x.1998.00289.X
Vaidya, G., Lohman, D. J., and Meier, R. (2011). SequenceMatrix: concatenation software for the fast assembly of multi-gene dataset with character set and codon information. Cladistics 27, 171–180. doi: 10.1111/j.1096-0031.2010.00329.x
van Vuuren, B. J., Lee, J. E., Convey, P., and Chown, S. L. (2018). Conservation implications of spatial genetic structure in two species of oribatid mites from the Antarctic Peninsula and the Scotia Arc. Antarct. Sci. 30, 105–114. doi: 10.1017/S0954102017000529
Vega, G. C., Convey, P., Hughes, K. A., and Olalla-Tárraga, M. Á. (2019). Humans and wind, shaping Antarctic soil arthropod biodiversity. Insect Conserv. Diversity 13, 63–76. doi: 10.1111/Icad.12375
Ventura, M., Petrusek, A., Miró, A., Hamrová, E., Buñay, C., de Meester, L., et al. (2014). Local and regional founder effects in lake zooplankton persist after thousands of years despite high dispersal potential. Mol. Ecol. 23, 1014–1027. doi: 10.1111/Mec.12656
Verleyen, E., Van de Vijver, B., Tytgat, B., Pinseel, E., Hodgson, D. A., Kopalová, K., et al. (2021). Diatoms define a novel freshwater biogeography of the Antarctic. Ecography 44, 548–560. doi: 10.1111/Ecog.05374
Viana, D. S., Santamaria, L., and Figuerola, J. (2016). Migratory birds as global dispersal vectors. Trends Ecol. Evol. 31, 763–775. doi: 10.1016/J.Tree.2016.07.005
Vianna, J. A., Noll, D., Dantas, G. P. M., Petry, M. V., Barbosa, A., Gonzalez-Acuna, D., et al. (2017). Marked phylogeographic structure of Gentoo penguin reveals an ongoing diversification process along the Southern Ocean. Mol. Phylogenet. Evol. 107, 486–498. doi: 10.1016/J.Ympev.2016.12.003
Wouw, M. V. D., Dijk, P. V., and Huiskes, A. H. L. (2008). Regional genetic diversity patterns in Antarctic hairgrass (Deschampsia Antarctica Desv.). J. Biogeogr. 35, 365–376. doi: 10.1111/J.1365-2699.2007.01784.X
Xu, S., Hebert, P. D., Kotov, A. A., and Cristescu, M. E. (2009). The noncosmopolitanism paradigm of freshwater zooplankton: insights from the global phylogeography of the predatory cladoceran Polyphemus pediculus (Linnaeus, 1761) (Crustacea, Onychopoda). Mol. Ecol. 18, 5161–5179. doi: 10.1111/J.1365-294x.2009.04422.X
Keywords: Antarctic-Magellan connection, centropagidae, last glacial maximum, monopolization hypothesis, phylogeography, refugia
Citation: Maturana CS, Biersma EM, Díaz A, González-Wevar C, Contador T, Convey P, Jackson JA and Poulin E (2022) Survivors and colonizers: Contrasting biogeographic histories reconciled in the Antarctic freshwater copepod Boeckella poppei. Front. Ecol. Evol. 10:1012852. doi: 10.3389/fevo.2022.1012852
Edited by:
Marco A. Molina-Montenegro, University of Talca, ChileReviewed by:
Marcelo Michel Rivadeneira, Universidad Católica del Norte, ChileEduardo Castro-Nallar, University of Talca, Chile
Copyright © 2022 Maturana, Biersma, Diaz, Gonzalez-Wevar, Contador, Convey, Jackson and Poulin. This is an open-access article distributed under the terms of the Creative Commons Attribution License (CC BY). The use, distribution or reproduction in other forums is permitted, provided the original author(s) and the copyright owner(s) are credited and that the original publication in this journal is cited, in accordance with accepted academic practice. No use, distribution or reproduction is permitted which does not comply with these terms.
*Correspondence: Claudia S. Maturana, Y2xhdWRpYW1hdHVyYW5hQHVjaGlsZS5jbA==