- Research Research Center on Animal Cognition (CRCA), Center for Integrative Biology (CBI), CNRS-University Paul Sabatier, Toulouse, France
It is often assumed that social life imposes specific cognitive demands for animals to communicate, cooperate and compete, ultimately requiring larger brains. The “social brain” hypothesis is supported by data in primates and some other vertebrates, but doubts have been raised over its applicability to other taxa, and in particular insects. Here, we review recent advances in insect cognition research and ask whether we can identify cognitive capacities that are specific to social species. One difficulty involved in testing the social brain hypothesis in insects is that many of the model species used in cognition studies are highly social (eusocial), and comparatively little work has been done in insects that live in less integrated social structures or that are solitary. As more species are studied, it is becoming clear that insects share a rich cognitive repertoire and that these abilities are not directly related to their level of social complexity. Moreover, some of the cognitive mechanisms involved in many social interactions may not differ from those involved in non-social behaviors. We discuss the need for a more comparative and neurobiologically grounded research agenda to better understand the evolution of insect brains and cognition.
1. Introduction
The considerable variation in brain size and cognitive abilities in the animal kingdom has been a long-standing question for biologists (Healy, 2021). It is broadly accepted that the brain evolved to process ecological information. However, it is an energetically expensive organ to operate and maintain. Therefore explanations for its evolution must reconcile these costs with fitness payoffs (Aiello and Wheeler, 1995). This presented an especially intriguing puzzle for primatologists who observed that the brains of primates were significantly larger than predicted, given their body size (Clutton-Brock and Harvey, 1980), and that classical explanations implicating their need for complex problem-solving (Gibson, 1986) did not account for these trends in all primate groups. The correlation between neocortex size and social group size in primates hinted at a link between brain size and sociality, later formalized as the “social brain hypothesis” (Dunbar, 1992; Dunbar, 1998). Following this hypothesis, increases in the frequency and complexity of social interactions, and the cognitive challenges of managing social relationships, may have required better information processing capabilities and larger brains. Over the past decades, research in primates has yielded experimental support for this hypothesis, reporting that neocortex size is also associated with intensity of reproductive competition (Lindenfors, 2005), frequency of coalition formation (Dunbar and Shultz, 2007), social play (Lewis, 2001), and social learning (Reader and Laland, 2002).
The story is a bit different in insects. While early description of insect anatomy and behavior also suggested a strong relationship between cognition and sociality (Dujardin, 1850), recent attempts to correlate brain size with metrics of social complexity are ambiguous (Riveros et al., 2012; O'Donnell et al., 2015; Farris, 2016; Kamhi et al., 2016; Gordon et al., 2019; Kamhi et al., 2019), emphasizing the need for more research (Lihoreau et al., 2012a; Simons and Tibbetts, 2019). Insects, like primates, display diverse social forms (Costa, 2006; Hölldobler and Wilson, 2009) which are accompanied by brain size variations (Strausfeld, 2012). However, the social organization of insect colonies can be dramatically different from that of vertebrates. In their most socially advanced forms (eusociality), insect colonies are characterized by extensive division of labor, cooperative brood rearing, and overlapping generations of adults (Batra, 1966; Michener, 1974; Wilson, 1975). This means that workers (i.e., the individuals on which most cognitive studies are performed) are sterile and fitness payoffs are experienced at the level of the group. Reproductive competition, mate selection and pair bonding - some of the primary drivers of brain evolution in social vertebrates (Healy, 2021) - therefore do not occur in these individuals. In fact, increases in insect social complexity are predicted to decrease overall brain size through obligate division of labor which allows for investment in functionally specialized brain regions (Gronenberg and Riveros, 2009; O'Donnell et al., 2015). In this way, the social structure of an insect colony may reduce the need for individual investment in neural tissue while improving overall performance in a few specialized tasks.
These recent theoretical developments, coupled with the observation that most of the impressive cognitive feats reported in insects have been identified in the context of individual foraging, and not social interactions (von Frisch, 1967; Giurfa, 2013; Wehner, 2020), draw attention to the emerging role of competition and foraging ecology in the evolution of insect cognition (Kamhi et al., 2016, 2019). For instance, a phylogenetic analysis has shown that the elaborated mushroom bodies in the insect brain (i.e., neuroanatomical structures involved in learning and memory), were acquired roughly 90MYR before the evolution of eusociality, coinciding with the switch from phytophagy to parasitoidism and the origin of central-place foraging (Farris and Schulmeister, 2011). This suggests the challenge of navigating and finding food in various types of landscapes drove the evolution of enhanced cognitive processes in what would later become eusocial insects (Jeanson and Weidenmüller, 2014; Farris, 2016). This observation thus raises the possibility that enhanced cognitive abilities may not be unique to just eusocial insects, but could be observed in any species that experiences the appropriate ecological conditions.
In this mini-review we consider whether many of the cognitive abilities once thought to be exclusive to social insects can evolve through non-social ecological processes. We argue that the primary drivers of insect cognition are processes related to reproductive competition and foraging. Using the extensive body of literature surrounding cognitive abilities in highly eusocial hymenopterans (i.e., honey bees, ants, and wasps) and the more recent, though rapidly expanding field of non-eusocial insect cognition (e.g., drosophila), we show that enhanced cognition abilities can evolve in other social structures (encompassing presocial, subsocial, semisocial, parasocial, quasisocial, facultatively social, cooperative breeding, gregarious, etc. species, sensu Michener, 1974; Wilson, 1975; Crespi and Yanega, 1995; Costa, 2006) and in solitary species.
2. Cognitive abilities shared by eusocial and non-eusocial insects
Advanced levels of sociality require cognitive abilities supporting precise social interactions (Byrne and Bates, 2007). For insects, this may mean being able to assess group identity, recognize specific individuals, remember past interactions with them, share information, find and learn the localization of food resources and navigate back to the colony nest (Wenseleers and van Zweden, 2017). Below we describe how many of these cognitive abilities, once thought to be unique to eusocial insects, have in fact recently been reported in some non-eusocial species and we discuss the possible explanations for how such traits could have evolved independently of sociality (see summary in Table 1).
2.1. Individual recognition
Targeted social interactions, such as cooperation, may require specific recognition systems to identify different types of individuals in a group or population (Anderson and McShea, 2001; Wenseleers and van Zweden, 2017). As such, eusocial insects have evolved recognition systems enabling them to discriminate nestmates and non-nestmates (van Zweden and d'Ettorre, 2010), as well as individuals from specific castes within the colony (van Oystaeyen et al., 2014), through the use of odor cues. In some small colonies where dominance hierarchies are established to determine reproductive priority, insects also possess the ability to recognize individual nestmates. This is the case of colonies founded by several reproductive females, like for instance the paper wasps Polistes fuscatus that have unique facial coloration patterns (Tibbetts, 2002), or the ants Pachycondylla villosa that carry individual chemical signatures (D’Ettorre and Heinze, 2005). Though these recognition mechanisms have been implicated in the ecological success of insect societies (Jeanson and Weidenmüller, 2014) they are not specific to eusocial species. Theoretical models predict that the capacity to recognize group members should peak at intermediate levels of sociality and decrease as societies become larger (Gronenberg and Riveros, 2009), possibly due to the inability to manage so many social interactions and the risk of genetic conflicts in large colonies with different patrilines (Ratnieks et al., 2006). Moreover, if cognitive processes involved in individual recognition evolved due to the demands of recognizing reproductive competitors (Tibbetts, 2002), insect societies composed of highly related individuals should not possess such abilities. In line with these predictions, recent work shows males of the fruit fly Drosophila melanogaster, which form temporary aggregations on food sources for feeding and mating, appear to recognize other males with whom they compete for access to females (Yurkovic et al., 2006), although the cues involved are still unknown. In the solitary decorated cricket (Gryllodes sigillatus), individuals acquire the scent of their partners after mating. Female crickets, who recognize their own odor through self-referent phenotype matching favour pairings with males with unfamiliar odors to maximize their number of mating partners (Capodeanu-Nägler et al., 2014). While more studies are needed to better understand the evolution of individual recognition across insects, these observations in eusocial and non-eusocial species suggest individual recognition may be more closely related to reproductive competition and mate selection, rather than just social life.
2.2. Numerosity
Social life may require the ability for individuals to assess quantities and estimate the number of social partners or potential competitors in a group (Rios and Kraenkel, 2017). Several studies suggest eusocial honey bees (Apis mellifera) can count landmarks (Chittka and Geiger, 1995), recognize the number of objects in an image (Gross et al., 2009), perform basic operations (Howard et al., 2019), and understand the concept of zero (Howard et al., 2018, but see MaBouDi et al., 2021). Desert ants (Cataglyphis fortis) seem to count steps to evaluate distances during path integration (Wittlinger et al., 2006). However, whether these abilities are linked to sociality is not clear as many other behaviors could also require numerosity, like monitoring brood and forager populations and increasing resource collection to feed a colony, may simply result from individual variability in response threshold to environmental stimuli (i.e., the presence of pheromones, empty brood cells) not numeric competency (Page and Erber, 2002). Moreover, eusocial insect colonies can be so large so as to make recognizing and tracking all social interactions between conspecifics very unlikely (Gronenberg and Riveros, 2009). Thus, we would expect cognitive traits related to numerosity to evolve when monitoring potential rivals or mates is both feasible, given colony/population size, and is associated with increased reproductive fitness. Accordingly, some studies suggest non-eusocial insects have some sense of numerosity. For instance, males of the gregarious beetle Tenebrio molitor are capable of adjusting the intensity of their mate guarding strategy according to the number of males encountered before mating (Carazo et al., 2009). In the ladybug Adalia bipunctata females reduce egg laying according to the number of larvae from other females already encountered (Hemptinne et al., 1992). These observations, though relatively rare, indicate that insect numerosity may be more closely related to the likelihood of encountering competitors and mates than just group size.
2.3. Non-elemental learning
Living in a group may also multiply the need for animals to develop different types of associative learning. As more individuals are encountered, more types of interactions, relationships, and common experiences may have to be stored in memories (Bond et al., 2003). Honey bees (A. mellifera), that live in colonies with thousands of workers, can learn many elemental associations between a stimulus and a reward, but also more sophisticated non-elemental associations involving ambiguous stimuli (Giurfa, 2013). For instance, bees can learn to respond to a reinforced odor A and not to a non-reinforced odor B, and then have to learn the opposite when stimulus contingencies are reversed (Boitard et al., 2015). Honey bees can also be trained to patterning problems, by associating a simple component (A or B) to a reward and the mixture (AB) to an absence of reward or vice versa (Devaud et al., 2015). So far, however all these experiments simulate learning in a foraging context, suggesting that this might be a stronger driver of associative learning than social life per se. Accordingly, the gregarious fruit fly D. melanogaster was recently shown to solve similar non-elemental cognitive operations such as reversal learning (Mancini et al., 2019) and negative patterning (Durrieu et al., 2020), a task related to foraging for food or oviposition sites. Given these observations in non-eusocial insects, it might well be that these types of learning are primarily required to find food or mating partners rather than just for navigating more social interactions.
2.4. Social learning
Animals can learn about their environment by observing conspecifics (Laland, 2004). Information provided by others often improves individual decisions and is believed to be a major advantage of social life (Bourke, 2011). Famously, honey bees share information about the location of abundant food sources in the form of a symbolic waggle dance (von Frisch, 1967). Eusocial bumblebees can learn flower preferences through visual observation of more experienced conspecifics (Leadbeater and Chittka, 2005; Worden and Papaj, 2005) and new foraging techniques, such as pulling ropes (Alem et al., 2016) or rolling balls (Loukola et al., 2017). Interestingly, the study of the mechanisms underpinning flower choice copying in bumblebees showed this behavior can emerge from associative learning mechanisms that are not different from non-social learning (Dawson et al., 2013). It has thus been argued that social learning is just a form of individual learning with a social cue (Galef, 1995), and the emergence of social learning should correlate with the cost of information acquisition, not a direct result of group living. Supporting that theory, gregarious fruit flies D. melanogaster can also learn from their conspecifics. Females copy the choices of others for oviposition sites (Battesti et al., 2012) and mating partners (Danchin et al., 2018). Solitary wood crickets Nemobius sylvestris learn to hide after being in contact with conspecifics maintained under stressful conditions in the presence of spiders (Coolen et al., 2005). Thus, social learning and may employ associative mechanisms of non social learning can be observed in solitary species.
2.5. Tool use
In social groups with overlapping generations of individuals, social learning can lead to the emergence and cultural transmission of complex behaviors, like tool use (Shumaker et al., 2011). Such transmission is a form of heritable adaptation that is likely a key benefit of social life (Danchin et al., 2004). Several phenomena akin to tool use have been reported in eusocial insects (Pierce, 1986). For instance, workers of Pogonomyrmex and Aphaenogaster ant colonies occasionally use soil debris to transport liquid food that they could not otherwise bring back to their nests (Maak et al., 2020). However, examples of tool-users are also found in non-eusocial species. Antlion larvae throw sand to make their prey fall into the bottom of its pit (Oguma, 1930). African tree crickets pierce holes in leaves to create baffles and amplify their mating calls (Prozesky-Schulze et al., 1975). Solitary wasps use pebbles to compact the soil and close their burrows (Brockmann, 1985). Future research would benefit from identifying the specific learning mechanisms (i.e., social learning or trial and error) involved in tool use in these later examples remains unclear (Kenward et al., 2005). Once again, these abilities appear to be linked to foraging, sexual competition and defense, rather than the degree of sociality (Weir and Kacelnik, 2006).
2.6. Emotions
Many social interactions are regulated by emotional displays that enable communication between group members (van Kleef, 2009). In principle, being in a specific emotional state can improve responses to threats or social interactions and lead to more efficient collective responses. In this context, emotional states are elicited by rewarding and punishing goals, whereby rewards are stimuli that animals work to acquire and punishments are stimuli that they work to avoid Rolls (2014). Studies using judgment bias paradigms (Baciadonna and McElligott, 2015) indicate eusocial insects show emotional-like states. For instance, honey bees (A. mellifera) gently shaken just before the experiment showed pessimistic biases when faced the problem of classifying an ambiguous odor stimulus (Bateson et al., 2011) whereas bumblebees (Bombus terrestris) that received an unexpected reward showed consistent optimistic biases in a visual judgment task (Perry et al., 2016). However, similar emotional-like states were also recently reported in non-eusocial insects, such as in the gregarious fruit fly D. melanogaster where shaken flies showed a pessimistic bias in an ambiguous odor binary choice task, while control flies did not (Deakin et al., 2018). Again, this observation in non-eusocial fruit flies suggests emotional display is not specific to sociality. In fact, it is likely that these emotional states are triggered by basal neurohormonal mechanisms common across insect species (i.e., releases of biogenic amines) and increase efficiency of decision making in many contexts independent of sociality, such as foraging or predator avoidance.
2.7. Elaborate navigation
Social animals must intensely engage in foraging in order to gather and store large amounts of foods for their progeny. For central place foragers, this requires precise spatial memories and efficient navigation (Collett et al., 2013). Accordingly, eusocial insects are notoriously skilled navigators, learning near optimal routes between their colony nest and specific places based on cues in the local environment and on the panorama (Wehner, 2020). Honey bees and bumblebees can travel several kilometers to visit hundreds of flowers looking for nectar and pollen, and repeat these foraging trips over several days or weeks (von Frisch, 1967). When these resources are patchily distributed in space, foragers tend to develop multi-destination routes (i.e., traplines) by which individuals visit plant patches in stable sequences using the shortest possible path linking all these places (Lihoreau et al., 2012b; Buatois and Lihoreau, 2016). Strikingly however, many non-eusocial insects, including non-central place foraging species, are also excellent at these spatial cognitive tasks. In fact, the seminal experiment demonstrating place learning in insects were conducted in the non-eusocial wasps (Philanthus triangulum) in which moving the visual landmarks surrounding the nest of the wasp triggered search around landmarks (Tinbergen, 1932). Fruit flies D. melanogaster also develop visual place memories when forced to find a hidden cool tile in a warm arena based on patterns displayed on the panorama (Ofstad et al., 2011). Various butterflies have been observed developing traplines between distant plants (Young and Montgomery, 2020). Dung beetles use environmental cues to navigate in a straight line away from the dung pile as fast as possible (Dacke et al., 2019). Spatial cognition skills supporting efficient navigation are therefore not exclusive to eusocial insects and appear to rely more on ecological factors such as the variability of food sources in time and space rather than sociality per se.
3. Concluding remarks
Many cognitive abilities once thought to be required for social interactions and unique to eusocial insects are also being discovered in non-eusocial species (see summary in Table 1). This suggests factors related to foraging ecology, mate selection, reproductive competition, and defense not just sociality may give rise to the rich and elaborated cognitive capabilities found in insects. This also emphasizes the need for further theoretical and experimental research to clarify the relationship between ecology, sociality, and cognition in this group (Lihoreau et al., 2019). We argue this knowledge gap can be filled by overcoming methodological and conceptual challenges.
Foremost among these challenges is a scarcity of model organisms which limits our ability to assess how ecological and life history traits impact cognitive evolution. The large volume of insect behavioral and cognitive research is almost entirely based on highly eusocial ants, bees, and wasps. This is not surprising as these insects are easily maintained in both laboratory and natural environments, display a diversity of cognitive and neuroanatomical traits and have a well-established history of assays used to quantify cognitive variability (Giurfa, 2013). While there have been attempts to compare cognitive abilities across closely related, ecologically distinct, eusocial species (Sheehan and Tibbetts, 2011; Tait et al., 2021), examples are still rare and limited in their power due to the small number of species and phylogenetic groups usually considered in these studies (Harvey and Purvis, 1991). There are, however, other species of eusocial and non-eusocial insects, each with a unique ecology and life history but with very little information regarding their cognitive traits. Comparative analyses investigating such understudied insect clades could go a long way to improving our understanding of the role of ecology and sociality in the evolution of insect cognition, especially given the noticeable taxonomic bias revealed by our review of the literature (Figure 1). The network map of keywords extracted from the Web of Science (titles and abstracts) illustrates research biases for behavioral and learning in bees and ants (orange cluster), in contrast to a functional molecular bias in Drosophila studies (blue cluster).
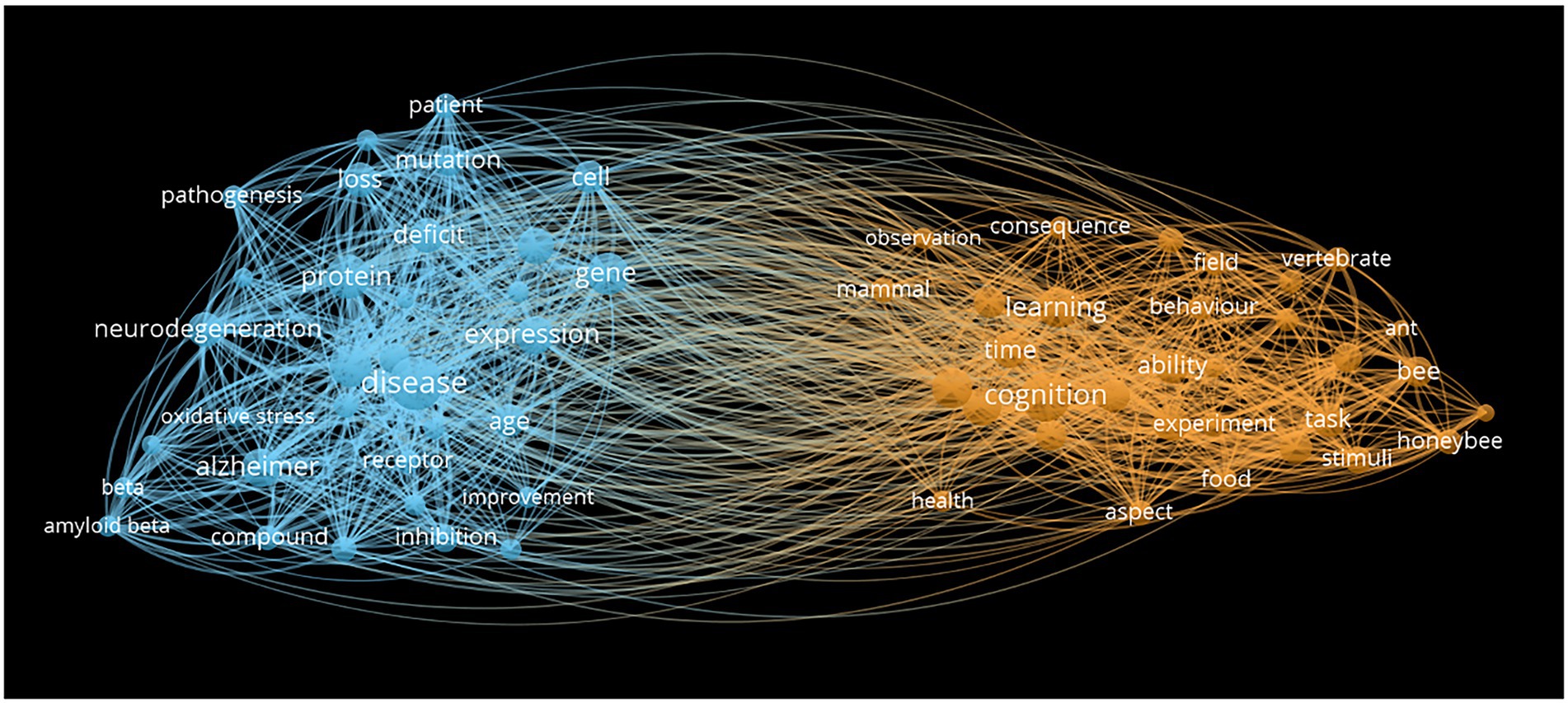
Figure 1. Literature search illustrating species biases in insect cognition research. Network map of words extracted from a Web of Science database, downloaded the September 21, 2021 from articles including “insect” and “cognition” either in the title or the abstract. We used VOSwiewer 1.6.16 with binary counting (only the presence or absence of a word counts), including each word that appeared 10 times or more, and removing or replacing irrelevant terms such as plural/singular, homonyms etc (see Supplementary Appendix). In the first cluster (orange), behavioral and learning studies were largely dominated by studies on bees and ants. In the second cluster (blue), molecular biology studies about brain functions were mostly dominated by studies on Drosophila.
Another difficulty lies in the unverified assumption that larger behavioral repertoires require larger brains (Godfrey and Gronenberg, 2019). Many fundamental changes in the complexity of a nervous system may not result in measurable volumetric differences, and novel behavior may emerge from minimal rewiring of existing neurons (Chittka and Niven, 2009). Therefore, recent studies point towards a need to consider a new framework for insect comparative cognition, including a comprehensive discussion on their ecology beyond their social status (Lihoreau et al., 2012a; O'Donnell et al., 2015; Farris, 2016; Simons and Tibbetts, 2019). If we want to move from describing cognitive feats in insect models to understand why and how these cognitive abilities have evolved across insects in general, we need to develop more systematic comparative analyses of species with contrasted ecologies (including foraging, mating, and social interactions) and detailed anotomo-functional measures of their brains. It is only by identifying cognitive operations that are specific to social life, the neural circuits they involve, and the ecology and life history of the species, that the social brain hypothesis and its derivatives, as they are currently framed, can be tested and refined.
Author contributions
All authors listed have made a substantial, direct, and intellectual contribution to the work and approved it for publication.
Funding
This work was funded by grants from the Alexander von Humboldt Foundation (1223114) to LAP, the European Molecular Biology Organization (EMBO153-2022) to CT, and the European Commission (FEDER ECONECT MP0021763, ERC Cog BEE-MOVE GA101002644) to ML.
Conflict of interest
The authors declare that the research was conducted in the absence of any commercial or financial relationships that could be construed as a potential conflict of interest.
Publisher’s note
All claims expressed in this article are solely those of the authors and do not necessarily represent those of their affiliated organizations, or those of the publisher, the editors and the reviewers. Any product that may be evaluated in this article, or claim that may be made by its manufacturer, is not guaranteed or endorsed by the publisher.
Supplementary material
The Supplementary material for this article can be found online at: https://www.frontiersin.org/articles/10.3389/fevo.2022.1001045/full#supplementary-material
References
Aiello, L. C., and Wheeler, P. (1995). The expensive tissue hypothesis. Curr. Anthropol. 36, 199–221. doi: 10.1086/204350
Alem, S., Perry, C. J., Zhu, X., Loukola, O. J., Ingraham, T., Søvik, E., et al. (2016). Associative mechanisms allow for social learning and cultural transmission of string pulling in an insect. PLoS Biol. 14:e1002564. doi: 10.1371/journal.pbio.1002564
Anderson, C., and McShea, D. W. (2001). Individual versus social complexity, with particular reference to ant colonies. Biol. Rev. 76, 211–237. doi: 10.1017/S1464793101005656
Baciadonna, L., and McElligott, A. G. (2015). The use of judgment bias to assess welfare in farm livestock. Anim. Welf. 24, 81–91. doi: 10.7120/09627286.24.1.081
Bateson, M., Desire, S., Gartside, S. E., and Wright, G. A. (2011). Agitated honeybees exhibit pessimistic cognitive biases. Curr. Biol. 21, 1070–1073. doi: 10.1016/j.cub.2011.05.017
Batra, S. W. T. (1966). Nests and social behavior of halictine bees of India (hymenoptera: Haloctidae). Ind. J. Entomol. 28, 375–393.
Battesti, M., Moreno, C., Joly, D., and Mery, F. (2012). Spread of social information and dynamics of social transmission within drosophila groups. Curr. Biol. 22, 309–313. doi: 10.1016/j.cub.2011.12.050
Boitard, C., Devaud, J. M., Isabel, G., and Giurfa, M. (2015). GABAergic feedback signaling into the calyces of the mushroom bodies enables olfactory reversal learning in honey bees. Front. Behav. Neurosci. 9:198. doi: 10.3389/fnbeh.2015.00198
Bond, A. B., Kamil, A. C., and Balda, R. P. (2003). Social complexity and transitive inference in corvids. Anim. Behav. 65, 479–487. doi: 10.1006/anbe.2003.2101
Brockmann, H. J. (1985). Tool use in digger wasps (hymenoptera: Sphecinae). Psyche 92, 309–329. doi: 10.1155/1985/73184
Buatois, A., and Lihoreau, M. (2016). Evidence of trapline foraging in honeybees. J. Exp. Biol. 219, 2426–2429. doi: 10.1242/jeb.143214
Byrne, R. W., and Bates, L. A. (2007). Sociality, evolution and cognition. Curr. Biol. 17, R714–R723. doi: 10.1016/j.cub.2007.05.069
Capodeanu-Nägler, A., Rapkin, J., Sakaluk, S. K., Hunt, J., and Steiger, S. (2014). Self-recognition in crickets via on-line processing. Curr. Biol. 24, R1117–R1118. doi: 10.1016/j.cub.2014.10.050
Carazo, P., Font, E., Forteza-Behrendt, E., and Desfilis, E. (2009). Quantity discrimination in Tenebrio molitor: evidence of numerosity discrimination in an invertebrate? Anim. Cogn. 12, 463–470. doi: 10.1007/s10071-008-0207-7
Chittka, L., and Geiger, K. (1995). Can honey bees count landmarks? Anim. Behav. 49, 159–164. doi: 10.1016/0003-3472(95)80163-4
Chittka, L., and Niven, J. (2009). Are bigger brains better? Curr. Biol. 19, R995–R1008. doi: 10.1016/j.cub.2009.08.023
Clutton-Brock, T. H., and Harvey, P. H. (1980). P.H. primates, brains and ecology. J. Zool. 190, 309–323. doi: 10.1111/j.1469-7998.1980.tb01430.x
Collett, M., Chittka, L., and Collett, T. S. (2013). Spatial memory in insect navigation. Curr. Biol. 23, R789–R800. doi: 10.1016/j.cub.2013.07.020
Coolen, I., Dangles, O., and Casas, J. (2005). Social learning in noncolonial insects? Curr. Biol. 15, 1931–1935. doi: 10.1016/j.cub.2005.09.015
Crespi, B. J., and Yanega, D. (1995). The definition of eusociality. Behav. Ecol. 6, 109–115. doi: 10.1093/beheco/6.1.109
D’Ettorre, P., and Heinze, J. (2005). Individual recognition in ant queens. Curr. Biol. 15, 2170–2174. doi: 10.1016/j.cub.2005.10.067
Dacke, M., Bell, A. T. A., Foster, J. J., Baird, E. J., Strube-Bloss, M. F., Byrne, M. J., et al. (2019). Multimodal cue integration in the dung beetle compass. Proc. Natl. Acad. Sci. U. S. A. 116, 14248–14253. doi: 10.1073/pnas.1904308116
Danchin, E., Giraldeau, L. A., Valone, T. J., and Wagner, R. H. (2004). Public information: from nosy neighbors to cultural evolution. Science 305, 487–491. doi: 10.1126/science.1098254
Danchin, E., Nöbel, S., Pocheville, A., Dagaeff, A. C., Demay, L., Alphand, M., et al. (2018). Cultural flies: conformist social learning in fruitflies predicts long-lasting mate-choice traditions. Science 362, 1025–1030. doi: 10.1126/science.aat1590
Dawson, E. H., Avarguès-Weber, A., Chittka, L., and Leadbeater, E. (2013). Learning by observation emerges from simple associations in an insect model. Curr. Biol. 23, 727–730. doi: 10.1016/j.cub.2013.03.035
Deakin, A., Mendl, M., Browne, W. J., Paul, E. S., and Hodge, J. J. L. (2018). State-dependent judgment bias in drosophila: evidence for evolutionarily primitive affective processes. Biol. Lett. 14:20170779. doi: 10.1098/rsbl.2017.0779
Devaud, J. M., Papouin, T., Carcaud, J., Sandoz, J. C., Grünewald, B., and Giurfa, M. (2015). Neural substrate for higher-order learning in an insect: mushroom bodies are necessary for configural discriminations. Proc. Natl. Acad. Sci. U. S. A. 112, E5854–E5862. doi: 10.1073/pnas.1508422112
Dujardin, F. (1850). Mémoires sur le système nerveux des insectes. Annales des Sciences Naturelles Zoologie 14, 195–206.
Dunbar, R. I. M. (1992). Neocortex size as a constraint on group size in primates. J. Hum. Evol. 22, 469–493. doi: 10.1016/0047-2484(92)90081-J
Dunbar, R. I. M. (1998). The social brain hypothesis. Evol. Anthropol. 6, 178–190. doi: 10.1002/(SICI)1520-6505(1998)6:5<178::AID-EVAN5>3.0.CO;2-8
Dunbar, R. I. M., and Shultz, S. (2007). Evolution in the social brain. Science 317, 1344–1347. doi: 10.1126/science.1145463
Durrieu, M., Wystrach, A., Arrufat, P., Giurfa, M., and Isabel, G. (2020). Fruit flies can learn non-elemental olfactory discriminations. Proc. R. Soc. B 287:20201234. doi: 10.1098/rspb.2020.1234
Farris, S. M. (2016). Insect societies and the social brain. Curr. Opin. Insect Sci. 15, 1–8. doi: 10.1016/j.cois.2016.01.010
Farris, S. M., and Schulmeister, S. (2011). Parasitoidism, not sociality, is associated with eth evolution of eleaborate mushroom bodies in the brains of hymenopteran insects. Proc. R. Soc. Lond. B 278, 940–951. doi: 10.1098/rspb.2010.2161
Galef, B. G. (1995). Why behavior patterns that animals learn socially are locally adaptive. Anim. Behav. 49, 1325–1334. doi: 10.1006/anbe.1995.0164
Gibson, K. R. (1986). “Cognition, brain size and the extraction of embedded food resources,” in Primate ontogeny, cognition and social behavior. eds. J. Else and P. C. Lee (Cambridge, UK: Cambridge University Press), 93–104.
Giurfa, M. (2013). Cognition with few neurons: higher-order learning in insects. Trends Neurosci. 36, 285–294. doi: 10.1016/j.tins.2012.12.011
Godfrey, R. K., and Gronenberg, W. (2019). Brain evolution in social insects: advocating for the comparative approach. J. Comp. Physiol. A. 205, 13–32. doi: 10.1007/s00359-019-01315-7
Gordon, D. G., Zelaya, A., Arganda-Carreras, I., Arganda, S., and Traniello, J. F. A. (2019). Division of labor and brain evolution in insect societies: neurobiology of extreme specialization in the turtle ant Cephalotes varians. PLoS One 14:e0213618. doi: 10.1371/journal.pone.0213618
Gronenberg, W., and Riveros, A. J. Social brains and behavior: past and present (Ed. by J. Gadau and J. Fewell) in Organization of Insect Societies (eds. J. Gadau, J. Fewell, and E. D. Wilson) 377–401. Harvard University Press. (2009).
Gross, H. J., Pahl, M., Si, A., Zhu, H., Tautz, J., Zhang, S., et al. (2009). Number-based visual generalization in the honeybee. PLoS One 4:e4263. doi: 10.1371/journal.pone.0004263
Harvey, P. H., and Purvis, A. (1991). Comparative methods for explaining adaptations. Nature 351, 619–624. doi: 10.1038/351619a0
Hemptinne, J.-L., Dixon, A. F. G., and Coffin, J. (1992). Attack strategy of ladybird beetles (Coccinellidae): factors shaping their numerical response. Oecologia 90, 238–245. doi: 10.1007/BF00317181
Hölldobler, B., and Wilson, E. (2009). The superorganism: The beauty, elegance, and strangeness of insect societies. New-York City, New York: WW Norton & Company.
Howard, S. R., Avarguès-Weber, A., Garcia, J. E., Greentree, A. D., and Dyer, A. G. (2018). Numerical ordering of zero in honey bees. Science 360, 1124–1126. doi: 10.1126/science.aar4975
Howard, S. R., Avarguès-Weber, A., Garcia, J. E., Greentree, A. D., and Dyer, A. G. (2019). Numerical cognition in honeybees enables addition and subtraction. Science. Advances 5:eaav0961. doi: 10.1126/sciadv.aav0961
Jeanson, R., and Weidenmüller, A. (2014). Interindividual variability in social insects - proximate causes and ultimate consequences. Biol. Rev. 89, 671–687. doi: 10.1111/brv.12074
Kamhi, J. F., Gronenberg, W., Robson, S. K. A., and Traniello, J. F. A. (2016). Social complexity influences brain investment and neural operation costs in ants. Proc. R. Soc. B Biol. Sci. 283:20161949. doi: 10.1098/rspb.2016.1949
Kamhi, J. F., Ilieş, I., and Traniello, J. F. A. (2019). Social complexity and brain evolution: comparative analysis of modularity and integration in ant brain organization. Brain Behav. Evol. 93, 4–18. doi: 10.1159/000497267
Kenward, B., Weir, A. A. S., Rutz, C., and Kacelnik, A. (2005). Tool manufacture by naive juvenile crows. Nature 433:121. doi: 10.1038/433121a
Laland, K. N. (2004). Social learning strategies. Anim. Learn. Behav. 32, 4–14. doi: 10.3758/BF03196002
Leadbeater, E., and Chittka, L. (2005). A new mode of information transfer in foraging bumblebees? Curr. Biol. 15, R447–R448. doi: 10.1016/j.cub.2005.06.011
Lewis, K. P. (2001). A comparative study of primate play behavior: implications for the study of cognition. Folia Primatol. 71, 417–421. doi: 10.1159/000052740
Lihoreau, M., Dubois, T., Gomez-Moracho, T., Kraus, S., Monchanin, C., and Pasquaretta, C. (2019). Putting the ecology back into insect cognition research. Adv. Insect. Physiol. 57, 1–25. doi: 10.1016/bs.aiip.2019.08.002
Lihoreau, M., Latty, T., and Chittka, L. (2012a). An exploration of the social brain hypothesis in insects. Front. Physiol. 3:442. doi: 10.3389/fphys.2012.00442
Lihoreau, M., Raine, N. E., Reynolds, A. M., Stelzer, R. J., Lim, K. S., Smith, A. D., et al. (2012b). Radar tracking and motion-sensitive cameras on flowers reveal the development of pollinator multi-destination routes over large spatial scales. PLoS Biol. 10:e1001392. doi: 10.1371/journal.pbio.1001392
Lindenfors, P. (2005). Neocortex evolution in primates: the “social brain” is for females. Biol. Lett. 1, 407–410. doi: 10.1098/rsbl.2005.0362
Loukola, O. J., Perry, C. J., Coscos, L., and Chittka, L. (2017). Bumblebees show cognitive flexibility by improving on an observed complex behavior. Science 355, 833–836. doi: 10.1126/science.aag2360
Maak, I., Roelandt, G., and D’Ettorre, P. (2020). A small number of workers with specific personality traits perform tool use in ants. elife 9, 1–22. doi: 10.7554/eLife.61298
MaBouDi, H., Barron, A. B., Li, S., Honkanen, M., Loukola, O. J., Peng, F., et al. (2021). Non-numerical strategies used by bees to solve numerical cognition tasks. Proc. R. Soc. B Biol. Sci. 288:20202711. doi: 10.1098/rspb.2020.2711
Mancini, N., Hranova, S., Weber, J., Weiglein, A., Schleyer, M., Weber, D., et al. (2019). Reversal learning in drosophila larvae. Learn. Mem. 26, 424–435. doi: 10.1101/lm.049510.119
Michener, C. D. (1974). The social behavior of bees: A comparative study. Cambridge, Massachusetts: Harvad University Press.
O'Donnell, S., Bulova, S. J., DeLeon, S., Khodak, P., Miller, S., and Sulger, E. (2015). Distributed cognition and social brains: reductions in mushroom body investment accompanied the origins of sociality in wasps (hymenoptera: Vespidae). Proc. R. Soc. B Biol. Sci. 282:20150791. doi: 10.1098/rspb.2015.0791
Ofstad, T. A., Zuker, C. S., and Reiser, M. B. (2011). Visual place learning in Drosophila melanogaster. Nature 474, 204–207. doi: 10.1038/nature10131
Page, R. E., and Erber, J. (2002). Levels of behavioral organization and the evolution of division of labor. Naturwissenschaften 89, 91–106. doi: 10.1007/s00114-002-0299-x
Perry, C. J., Baciadonna, L., and Chittka, L. (2016). Unexpected rewards induce dopamine-dependent positive emotion-like state changes in bumblebees. Science 353, 1529–1531. doi: 10.1126/science.aaf4454
Prozesky-Schulze, L., Prozesky, O. P. M., Anderson, F., and van der Merwe, G. J. J. (1975). Use of a self-made sound baffle by a tree cricket. Nature 255, 142–143. doi: 10.1038/255142a0
Ratnieks, F. L. W., Foster, K. R., and Wenseleers, T. (2006). Conflict resolution in insect societies. Annu. Rev. Entomol. 51, 581–608. doi: 10.1146/annurev.ento.51.110104.151003
Reader, S. M., and Laland, K. N. (2002). Social intelligence, innovation, and enhanced brain size in primates. Proc. Natl. Acad. Sci. U. S. A. 99, 4436–4441. doi: 10.1073/pnas.062041299
Rios, V. P., and Kraenkel, R. A. (2017). Do I know you? How individual recognition affects group formation and structure. PLoS One 12:e0170737. doi: 10.1371/journal.pone.0170737
Riveros, A. J., Seid, M. A., and Wcislo, W. T. (2012). Evolution of brain size in class-based societies of fungus-growing ants (Attini). Anim. Behav. 83, 1043–1049. doi: 10.1016/j.anbehav.2012.01.032
Rolls, E. T. (2014). Discussion forum emotion and decision-making explained: a précis. Cortex 59, 185–193. doi: 10.1016/j.cortex.2014.01.020
Sheehan, M. J., and Tibbetts, E. A. (2011). Specialized face learning is associated with individual recognition in paper wasps. Science 334, 1272–1275. doi: 10.1126/science.1211334
Shumaker, R. W., Walkup, K. R., and Beck, B. B. (2011). Animal Tool Behavior. eds. R. W. Shumaker, K. R. Walkup, B. B. Beck (Baltimore, MD: Johns Hopkins University Press).
Simons, M., and Tibbetts, E. A. (2019). Insects as models for studying the evolution of animal cognition. Curr. Opin. Insect Sci. 34, 117–122. doi: 10.1016/j.cois.2019.05.009
Strausfeld, N. J. (2012). Arthropod Brains: Evolution, functional elegance, and historical significance. Cambridge, Massachusetts: Harvard University Press.
Tait, C., Brockmann, A., and Naug, D. (2021). Nesting ecology does not explain slow–fast cognitive differences among honeybee species. Anim. Cogn. 24, 1227–1235. doi: 10.1007/s10071-021-01515-2
Tibbetts, E. A. (2002). Visual signals of individual identity in the wasp Polistes fuscatus. Proc. R. Soc. B Biol. Sci. 269, 1423–1428. doi: 10.1098/rspb.2002.2031
Tinbergen, N. (1932). Über die Orientierung des Bienenwolfes (Philanthus triangulum Fabr.). Zeitschrift für Vergleichende. Physiologie 16, 305–334. doi: 10.1007/BF00338750
van Kleef, G. A. (2009). How emotions regulate social life: the emotions as social information (EASI) model. Curr. Dir. Psychol. Sci. 18, 184–188. doi: 10.1111/j.1467-8721.2009.01633.x
van Oystaeyen, A., Oliveira, R. C., Holman, L., van Zweden, J. S., Romero, C., Oi, C. A., et al. (2014). Conserved class of queen pheromones stops social insect workers from reproducing. Science 343, 287–290. doi: 10.1126/science.1244899
van Zweden, J. S., and d'Ettorre, P. (2010). “Nestmate recognition in social insects and the role of hydrocarbons,” in Insect hydrocarbons: Biology, biochemistry and chemical ecology. eds. G. J. Blomquist and A.-G. Bagnères (Cambridge UK: Cambridge University Press), 222–243.
von Frisch, K. The dance language and orientation of bees. (Cambridge, MA, United States: Harvard University Press. (1967).
Wehner, R. (2020). Desert Navigator: The journey of the ant. Cambridge, Massachusetts: Harvard University Press.
Weir, A. A. S., and Kacelnik, A. (2006). A new Caledonian crow (Corvus moneduloides) creatively re-designs tools by bending or unbending aluminium strips. Anim. Cogn. 9, 317–334. doi: 10.1007/s10071-006-0052-5
Wenseleers, T., and van Zweden, J. S. (2017). Sensory adaptations to social living in insect societies. Proc. Natl. Acad. Sci. U. S. A. 114, 6424–6426. doi: 10.1073/pnas.1707141114
Wittlinger, M., Wehner, R., and Wolf, H. (2006). The ant odometer: stepping on stilts and stumps. Science 312, 1965–1967. doi: 10.1126/science.1126912
Worden, B. D., and Papaj, D. R. (2005). Flower choice copying in bumblebees. Biol. Lett. 1, 504–507. doi: 10.1098/rsbl.2005.0368
Young, F. J., and Montgomery, S. H. (2020). Pollen feeding in Heliconius butterflies: the singular evolution of an adaptive suite. Proc. R. Soc. B 287:20201304. doi: 10.1098/rspb.2020.1304
Keywords: social brain hypothesis, insects, social evolution, comparative cognition, foraging, mate competition
Citation: Poissonnier L-A, Tait C and Lihoreau M (2023) What is really social about social insect cognition? Front. Ecol. Evol. 10:1001045. doi: 10.3389/fevo.2022.1001045
Edited by:
Simon M. Tierney, Western Sydney University, AustraliaReviewed by:
Andre Riveros, Universidad del Rosario, ColombiaCopyright © 2023 Poissonnier, Tait and Lihoreau. This is an open-access article distributed under the terms of the Creative Commons Attribution License (CC BY). The use, distribution or reproduction in other forums is permitted, provided the original author(s) and the copyright owner(s) are credited and that the original publication in this journal is cited, in accordance with accepted academic practice. No use, distribution or reproduction is permitted which does not comply with these terms.
*Correspondence: Mathieu Lihoreau, bWF0aGlldS5saWhvcmVhdUB1bml2LXRsc2UzLmZy
†ORCID: Laure-Anne Poissonnier https://orcid.org/0000-0003-2264-6964
Catherine Tait https://orcid.org/0000-0002-3642-082X
Mathieu Lihoreau https://orcid.org/0000-0002-2463-2040