- 1Glasgow Polyomics, Wolfson Wohl Cancer Research Centre, College of Medical, Veterinary and Life Sciences, University of Glasgow, Glasgow, United Kingdom
- 2Sea Mammal Research Unit, School of Biology, University of St Andrews, St Andrews, United Kingdom
- 3College of Medical, Veterinary and Life Sciences, Institute of Biodiversity, Animal Health and Comparative Medicine, University of Glasgow, Glasgow, United Kingdom
Mammalian mothers usually provide their offspring with large quantities of immunoglobulins (antibodies) for circulation in blood, either trans-placentally before birth, via colostrum briefly thereafter, or, less commonly, from milk. Neonates of true, phocid seals, however, are peculiarly impoverished in serum immunoglobulins, the levels of which slowly increase but do not reach adult levels by the time of weaning. We investigated whether grey seal (Halichoerus grypus) neonates compensate through an elevation or rapid maturation in levels of serum innate immune factors, namely acute phase and complement proteins. Instead, their sera contained remarkably low levels of acute phase proteins (including C-reactive protein, haptoglobin, hemopexin, ceruloplasmin, orosomucoid), compared to their mothers, that barely increased to adult levels by weaning. For complement, there was a strong demarcation between the early activation and amplification cascade components (present at normal adult levels in pups) and the late lytic membrane attack complex and regulatory proteins (consistently at low relative levels). Phocid neonates therefore differ dramatically from land Carnivorans, such as dogs and cats, in early life immune protection. That neonatal phocids survive this apparent vulnerability to infections between birth and weaning prompts questions as to what other mechanisms protect them, and the adaptive value of their seeming vulnerability.
Introduction
At birth, the neonates of viviparous mammals move abruptly from a (near) sterile environment (Perez-Munoz et al., 2017; Lim et al., 2018; Rehbinder et al., 2018; Stinson et al., 2019; Campisciano et al., 2020; Singh and Mittal, 2020) of their mothers’ uteri into a world rich in potential pathogens. The survival of neonates depends on both their endogenous innate and newly engaged adaptive immune systems, and, more immediately, antibodies passively acquired from their mothers (Brambell, 1970). Mothers provide a sample of their own immunoglobulins directly into the circulation of their offspring either trans-placentally before birth, or during a brief postnatal period during which proteins in colostrum can traverse the gut epithelium directly into their blood (Brambell, 1970). Thereafter, and only in certain species, such as rats, immunoglobulins from milk may be translocated across the intestinal epithelium into the circulation by a receptor-mediated process similar to that in trans-placental transfer of immunoglobulin G (IgG) (Roopenian and Akilesh, 2007; Westrom et al., 2020). Meanwhile, the factors of the innate immune system act to provide continuous protective cover and to alert the adaptive immune system to breaches (Parham, 2021). If systemic infection occurs then innate immunity can respond rapidly, such as in the acute phase (fever) response. The main proteins in blood plasma that provide innate immune protection against infection are the acute phase proteins, complement components, and, less directly, coagulation factors (Parham, 2021).
Neonates of most mammal groups, exemplified by humans, cattle, pigs, camels, dogs, and cats, exhibit concentrations of maternal immunoglobulins in their blood equivalent to or even greater than their mothers by birth, or within a matter of hours thereafter, despite acquiring maternal immunoglobulins by different mechanisms (Brambell, 1970; Simister, 2003; Roopenian and Akilesh, 2007; Langer, 2008; Wooding and Burton, 2008; Langer, 2009; Westrom et al., 2020). The true, phocid, seals are exceptions to this, in that neonates exhibit levels of immunoglobulin that are a fraction of adult levels, even after taking colostrum (Ross et al., 1994; King et al., 1998; Hall et al., 2003; Ferreira et al., 2005). The concentrations of immunoglobulins then build slowly after birth, but barely reach adult levels by the time they are weaned (Ross et al., 1994; King et al., 1998; Hall et al., 2003; Ferreira et al., 2005). The source of these immunoglobulins (principally IgG) is not understood but suspected to be mainly or entirely produced by the neonate alone, and not gifted by the mother, although there is some evidence of trans-colostral transfer (Ross et al., 1994; King et al., 1998). This would conventionally mean that seal neonates should be peculiarly vulnerable to infections. A prediction would, therefore, be that the innate immune system of true seal pups should be unusually well-developed at birth, particularly given that they can rely on immune protection from milk for only a uniquely short time among mammal groups—phocids are developmentally precocious at birth and have the shortest lactation periods for their size amongst eutherian mammals (Schulz and Bowen, 2005)—another reason why reliance on, and rapid maturation of, innate immunity would be advantageous.
We sampled sera from mother and pup pairs of Atlantic grey seals, Halichoerus grypus, from shortly after birth until near weaning and here compared the relative levels of immune-related proteins. We did this using proteomic techniques that have not been used before to examine immunoglobulin levels in seals and found that grey seal pups, as previously reported, align with other phocids in acquiring little if any immunoglobulins from their mothers. Contrary to our prediction, however, pups did not exhibit compensatory high levels of innate immune factors, but quite the reverse—pups had remarkably low levels of the canonical acute phase proteins, particularly in the immediate perinatal period, and the same applied to the complement factors involved in direct lytic attack on pathogens.
The survival of phocid seal neonates is therefore puzzling in that they are gifted with little if any antibody protection by their mothers yet survive despite a paucity of endogenous innate immune factors. Whether this condition of seeming vulnerability is unique to phocid seals or is more widespread amongst marine Carnivora such as the otariids (sea lions, fur seals) and odobenids (walruses), remains to be seen, but it is clearly divergent from related land Carnivora typified by canids and felids.
Materials and Methods
Animals and Serum Samples
Blood samples were collected from five free-ranging mother/pup pairs (details in Supplementary Material B) on the Isle of May, Scotland (56.18°N 2.55°W). Four samples were collected from each mother and pup on days 2, 7, 13, and 17 or 18 or 19 days after birth—for simplicity, samples taken 17, 18, or 19 days are presented as being from day 18 throughout this report. In this species, mothers wean their pups between about 16 and 23 days after birth and return to sea, leaving their pups in the natal colony to continue development before they themselves go to sea independently after a further period of up to 40 days, during which time they do not feed (Bennett et al., 2010). All five pups had growth rates and weaned at masses within the normal range for UK grey seals (Pomeroy et al., 1996; Pomeroy et al., 1999). Their minimum durations of suckling ranged from 18 to 21 days. To avoid desertions by the mothers, the earliest samples were taken on day 2 post-partum. Mothers were tranquilised with an intramuscular mass-adjusted dose of Zoletil 100 (Virbac, Bury St Edmunds, Suffolk, United Kingdom), followed by intravenous oxytocin to stimulate milk let-down to provide milk samples as part of a larger study, and given a mass-adjusted intramuscular prophylactic dose of tetracycline after the day 7 and 18 samples (including blood) were taken, as reported previously. Blood was collected by venipuncture of either extradural vein or hind flipper plexus using 3.5in 16G spinal needle or 2in 19G Microlance needle (Becton Dickinson, United Kingdom) as appropriate into plain vacutainers and allowed to clot. Serum was separated by centrifugation within 4 h of collection, frozen at -20°C and stored at that temperature or below until sent to Glasgow for analysis. Samples from all five mother/pup pairs (pairs A to E) were used for the protein gel electrophoresis, and three pairs (B, D, and E) were used for the proteomics. At each capture, mothers and pups were checked externally for signs of ill health or abnormal growth of pups which may have indicated underlying problems. Pups on breeding colonies risk being bitten or suffer injuries from neighboring adults resulting in infections or trauma. No evidence of such was detected in our sample.
Protein Gel Electrophoresis
One-dimensional vertical sodium dodecyl sulfate polyacrylamide gel electrophoresis (SDS-PAGE) was carried out using the Invitrogen (Thermo Scientific, Paisley, United Kingdom) NuPAGE system with precast 4–12% gradient acrylamide gels, and β-mercaptoethanol as reducing agent when required. Pre-stained molecular mass/relative mobility (Mr) standard proteins were obtained from New England Biolabs, Ipswich, MA, United States (Cat. No. P77085). Gels were stained for protein using colloidal Coomassie Blue (InstantBlue; Expedion, Harston, United Kingdom), backlit with white light and images of gels recorded using a smartphone. Color images were converted to greyscale using IrfanView.1 The relative densities of specified bands were analysed using ImageJ software.2
Tandem Mass Tag Proteomics
This multiplex comparative proteomics technique provides relative abundances of detected proteins between six samples in one analysis run. Here, serum samples from one mother that were taken on days 2, 7, and 18 after birth were analyzed along with samples taken on the same days from her pup. Serum was sampled from three mother/pup pairs. Note that this method provides relative abundances between samples, not absolute values.
Tryptic peptides were generated from each serum sample using the Filter-Assisted Samples Preparation (FASP) method (Wisniewski et al., 2009), omitting LysC enzyme digestion steps and using a 30kDa ultrafiltration spin column. Equal volumes of the resulting peptide samples were labeled with TMT 6-plex reagents according to the manufacturer’s instructions (Thermo Scientific). Each of the six samples derived from each mother/pup pair were labeled with a set of six isobaric TMT reagents. Samples were then combined in equal volumes to constitute three separate 6-plex sets, comprising sera from one mother and her pup. Six microgram mixed, TMT-labeled peptides were dried and resolubilized in 20 μL 5% acetonitrile with 0.5% formic acid using the auto-sampler of a nanoflow uHPLC system (Thermo Scientific RSLCnano). Online detection of peptide ions was by electrospray ionization (ESI) mass spectrometry MS/MS with an Orbitrap Elite MS (Thermo Scientific). Ionization of LC eluent was performed by interfacing the LC coupling device to an NanoMate Triversa (Advion Bioscience) with an electrospray voltage of 1.7 kV. An injection volume of 5 μL of the reconstituted protein digest was desalted and concentrated for 12 min on trap column (0.3 × 5 mm) using a flow rate of 25 μl/min with 1% acetonitrile with 0.1% formic acid. Peptide separation was performed on a Pepmap C18 reversed phase column (50 cm × 75 μm, particle size 3 μm, pore size 100 Å, Thermo Scientific) using a solvent gradient at a fixed solvent flow rate of 0.3 ml/min for the analytical column. The solvent composition was (A) 0.1% formic acid in water (B) 0.08% formic acid in 80% acetonitrile 20% water. The solvent gradient was 4% B for 10 min, 4–60% for 170 min, 60–99% for 15 min, held at 99% for 5 min. A further 10 min at initial conditions for column re-equilibration were used before the next injection. The Orbitrap Elite was set to acquire a high-resolution precursor scan at 60,000 RP (over a mass range of m/z 380–1,800) followed by CID fragmentation and detection of the top 3 precursor ions from the MS scan in the linear ion trap. The 3 precursor ions are also subjected to HCD in the HCD collision cell followed by detection in the Orbitrap. Singly charged ions were excluded from selection, while selected precursors were added to a dynamic exclusion list for 180 s.
MS/MS spectra were analyzed for protein identification and quantification using Proteome Discoverer software 2.4 (Thermo Fisher Scientific). Protein identifications were assigned using the Mascot search engine (v2.6.2, Matrix Science) to interrogate protein sequences in the NCBIprot database using the taxonomy Caniformia and Phocidae with a precursor mass tolerance of 10ppm and fragment ion mass tolerance of 0.3Da. Set modifications were addition of TMT 6-plex labels to lysines and N-termini, carbamidomethylation of cysteine (fixed) and methionine oxidation (variable). Two missed cleavages for the trypsin digestion were permitted. Unique peptides were allowed for protein quantification in the consensus workflow, and normalization was designated for the total peptide amount with a scaling mode based on channels average per file. The quantification ratios were filtered based on fold change threshold equal to or greater than 1.5. The false discovery rate (FDR) was set at 5%.
Statistical Analysis and Graphing
Heatmaps with hierarchical clustering, variable importance projection (VIP) analysis, and Principal Component Analysis (PCA) were carried out using MetaboAnalyst 5.0,3 for which the data were assembled for upload in MS Excel and then log transformed online with MetaboAnalyst 5.0. ANOVA with Tukey’s procedure and graphing were carried out using MetaboAnalyst and OriginPro 2021 software (OriginLab, Northampton, Massachusetts, United States), and both were used to create the figures and graphs.
Results and Discussion
Immunoglobulins—Minimal or No Maternal Antibody?
We examined immunoglobulin levels in two ways—optical density scanning of stained protein electrophoresis gels, and by Tandem Mass Tag (TMT) proteomics. As applied here, both methods provide relative levels but not absolute concentrations of each protein, and we do not know whether the levels detected here in these mother seals are typical of adult males and non-breeding females in this population. It has been reported in grey seals that immunoglobulin G (IgG) levels in lactating females are lower than in adult males (King et al., 1994), but in elephant seals no difference between adult males and female has been found (Ferreira et al., 2005).
Protein electrophoresis gels showed remarkably low levels of proteins at the migration position expected for IgG (around 175 kDa) at 2 days after birth in pups, with a gradual increase culminating in about half or less of the amounts in maternal sera by weaning (Figures 1A,B). As a check that the cluster of protein bands at ∼175 kDa indeed comprised predominately IgG, they were, firstly, found to show characteristic disappearance under reducing conditions, yielding two proteins of sizes corresponding to heavy and light chains of IgG (Supplementary Figures 1A,B). Secondly, the identity of the main constituent of the ∼175 kDa band cluster as IgG was confirmed by proteomic analysis on excised bands (data not shown).
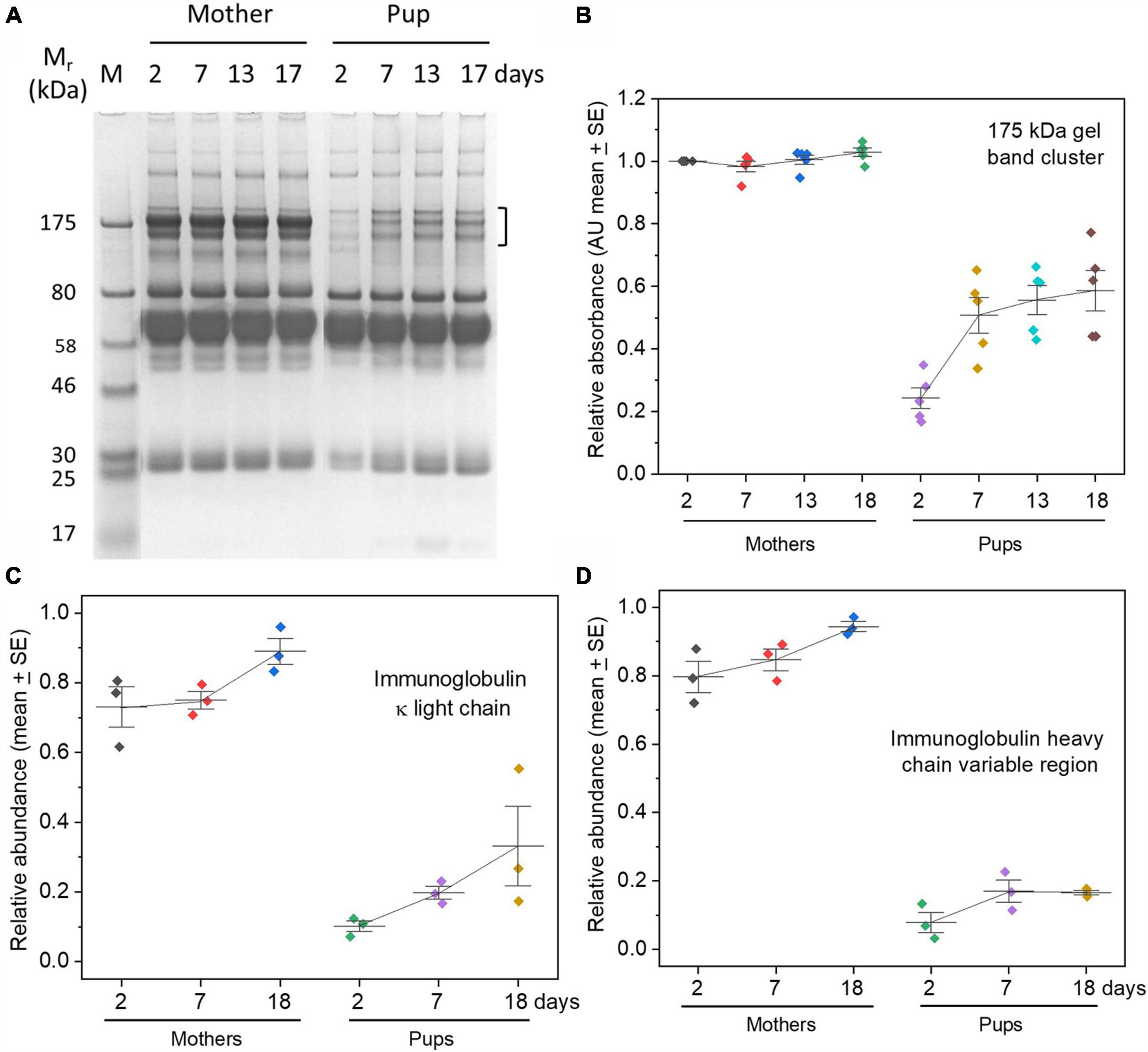
Figure 1. Low levels of IgG in grey seal pup sera relative to maternal levels with only gradual accumulation by weaning time. (A) Protein electrophoresis gel of sera from one mother/pup pair (pair B) sampled on the days indicated after birth. The three closely migrating protein bands enclosed by the square bracket migrate commensurately with isotypes of IgG, accounting for size and differential glycosylation. The cluster was excised from the maternal samples in the gel and confirmed to contain IgG by proteomics (data not shown), and by reduction and re-running on protein electrophoresis gels (Supplementary Figure 1B). Further confidence that the 175 kDa cluster contained IgG came from proteomics of similar bands in grey seal milk (Lowe et al., 2017). Mr, relative mobility of migration expressed in kiloDaltons (kDa); M, reference/marker proteins. (B) The clusters of gel bands indicated by the square bracket in (A) were optically scanned for each individual animal and optical density measurements plotted and expressed in arbitrary relative units. (C,D) Relative concentrations of immunoglobulin kappa light chain and heavy chain obtained from TMT proteomics, again indicating very low levels of immunoglobulins in pups’ sera. Similar results were obtained for lambda light chains and immunoglobulin J chain (Supplementary Figures 1C,D). Relative optical density (gel bands) or relative abundance (proteomics) values are expressed in arbitrary units.
Further support for the paucity of IgG in pup sera came from TMT proteomics showing that the relative levels of immunoglobulin kappa light chains (which will be present in all isotypes of immunoglobulin), and heavy chain variable regions, were a small fraction of maternal levels and rose only slightly with time (Figures 1B,C). Lambda light chains yielded the same trend (Supplementary Figure 1C). We also detected immunoglobulin J chain, again at only very low relative levels (Supplementary Figure 1D); J chains are associated with IgM and IgA, but not IgG or other immunoglobulin isotypes. IgM is not known to be transferred across mammalian placentae from maternal circulation, but may cross into the circulation of neonates in species dependent on colostrum before the gut epithelium closes (Westrom et al., 2020). Both IgG and IgM are found in seal milk (along with the mucosa-protective IgA isotype) (Marquez et al., 2003; Lowe et al., 2017), so, while it is possible that what little of these isotypes is found in pup sera derives from colostrum or milk, it is tempting to consider merely that they are produced by the pups themselves (King et al., 1998).
Our finding of remarkably low levels of serum IgG in grey seal pups soon after birth is consistent with previous reports on this and other phocids (King et al., 1994; Ross et al., 1994; King et al., 1998; Hall et al., 2003; Ferreira et al., 2005). But, our use of proteomics and simultaneous measurements of mother and pup sera indicate that grey seals appear to present an extreme case in exhibiting such low relative levels until weaning. Phocids therefore contrast with other species of Carnivora, such as dogs and cats, in which serum IgG levels approach or even exceed maternal levels by the time the colostrum phase ends (Yamada et al., 1991; Claus et al., 2006; Pereira et al., 2019). The type of placenta known from Carnivora (endotheliochorial; Wooding and Burton, 2008; Gundling and Wildman, 2015) may allow very limited trans-placental transfer of IgG, or none—trans-placental transfer does not occur in cats (Claus et al., 2006), but in dogs there is evidence of limited transfer (Krakowka et al., 1978; Pereira et al., 2019). In dogs, indications of trans-placental transfer of IgG come from electron microscopical observations of the haemophagous zone of their endotheliochorial placentae (Stoffel et al., 2000), and from the existence of anti-viral IgG antibody in the serum of neonates before they ingest colostrum (Krakowka et al., 1978). There is no evidence of trans-placental transfer in another canid, foxes (Vos et al., 2003). In the Caniformia to which dogs, foxes and phocid seals belong, therefore, trans-placental transfer of immunoglobulins is slight or non-existent, implying that transfer in colostrum is crucial to survival. In dogs and in cats, colostrum antibody is essential for survival unless otherwise artificially supplemented (Poffenbarger et al., 1991; Levy et al., 2001; Claus et al., 2006; Pereira et al., 2019). Curiously, IgG levels in grey seal pups at weaning appear to correlate negatively with first year survival of grey seal pups but, as the authors admit, this finding does not account for the pups’ infection history (Hall et al., 2002).
The extremely low periparturient levels of immunoglobulins we found in grey seal pups could have been due to a rapid post-birth loss of maternally-derived antibody through consumption in anti-microbial defense. But, depletion down to the levels we observe within 2 days after birth would be unprecedented given that that is about the time at which maximum levels of maternal IgG appear in cat and dog neonates (Brambell, 1970; Claus et al., 2006). The subsequent gradual rise in levels is therefore likely due to endogenous production by the seal pups as their immune systems begin to respond to exposure to microbes and other environmental factors.
It is known from previous work that seal milk contains consistent levels of IgG throughout lactation (Marquez et al., 1995; Marquez et al., 2003; Lowe et al., 2017), so there could be continuous trans-intestinal acquisition by pups. If so, then transfer across the gut epithelium would likely require a receptor- and pinocytosis-mediated process such as occurs in trans-placental transport in haemochorial placentae (Simister, 2003; Roopenian and Akilesh, 2007). Such a receptor, FcRn (which mediates trans-placental transfer of IgG in human and rodents), is known in rats to transfer IgG from milk, but this does not occur in the gut of humans (Simister, 2003; Mayer et al., 2005; Roopenian and Akilesh, 2007; Westrom et al., 2020). Genes encoding an FcRn-like protein are known from Carnivora, including grey seals (NCBI accession XP_035924104.1), but it may have functions beyond reproduction (Roopenian and Akilesh, 2007; Rath et al., 2013), and it is not yet known whether it is expressed in the intestine of seal pups or, to our knowledge, other Carnivoran neonates.
Having found, using proteomics, that grey seal pups are endowed with little or no maternal immunoglobulins, we next investigated whether there is a compensatory elevation or rapid maturation in the innate immune armory.
Acute Phase Proteins—Paucity in Pups
The main acute phase proteins of mammals were detected in both mother and pup sera by TMT proteomics. Most of these were initially at very low levels that barely rose with time, although a few increased to levels approaching maternal serum by time of weaning (Figure 2). This distinct paucity applied to acute phase reactants that are widely used as markers of inflammation and infection in human and veterinary medicine such as C-reactive protein, haptoglobin, ceruloplasmin and orosomucoid (syn. alpha-1-acid glycoprotein) (Figure 2 and Supplementary Figure 2; Liberatori et al., 1997; Ceron et al., 2005; Cray et al., 2009; Eckersall and Bell, 2010; Ehlting et al., 2021). Notable exceptions were two proteinase inhibitors, alpha-2-macroglobulin and alpha-1-antitrypsin (but not alpha-1-antichymotrypsin), both of which initially exceeded maternal levels. Serum amyloid P-component and leucine-rich alpha-2-glycoprotein exhibited unusual patterns in being at low levels after birth, rapidly rising to above maternal levels, then falling.
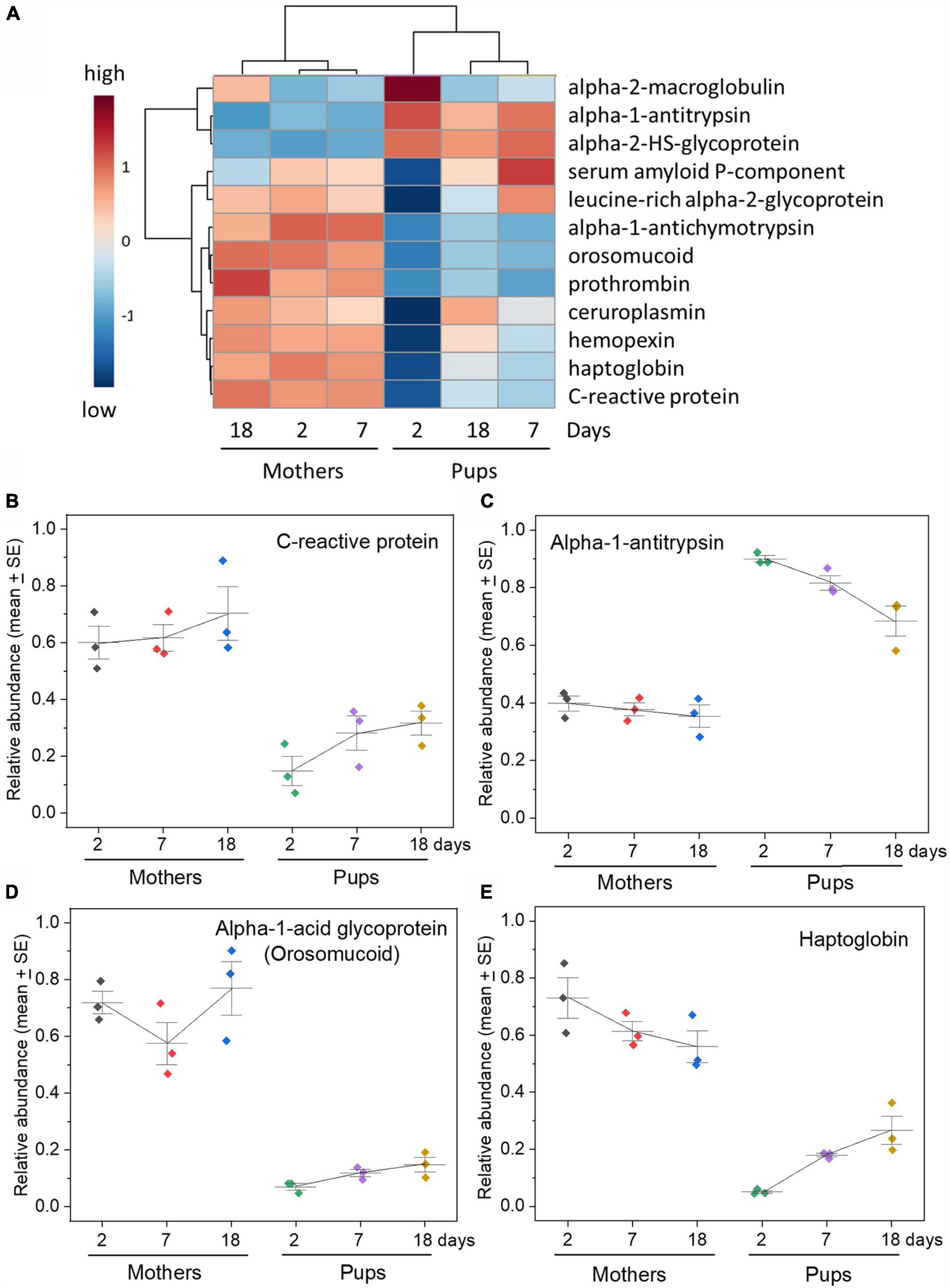
Figure 2. Grey seal pups have low levels of acute phase proteins in their sera relative to their mothers. (A) Heatmap with hierarchical clustering of all the acute phase proteins included in the analysis with relative abundance values obtained from TMT proteomics from three mother and pup pairs sampled on days 2, 7, and 18 after birth. The TMT data were log transformed, and the heatmap was created with group averages (three pups on a given day after birth, three mothers on a given day). See Supplementary Figure 2 for a heatmap from the same data but for individual mothers and pups. The cluster dendrogram above the heatmap clearly differentiates mother sera from pup sera at all days, and the dendrogram to the left splits the acute phase proteins into two distinct groups. Note that the columns in each heatmap column do not follow day order because the analysis yields the overall relationship between days—as reflected in the hierarchical cluster. Despite this the mother and pup samples easily group and segregate. (B–E) Relative concentration changes in selected acute phase proteins (C-reactive protein, haptoglobin, alpha-1-acid glycoprotein (syn. orosomucoid), and alpha-1-antitrypsin) in mother and pup sera with time after birth. See Supplementary Figure 2 for graphs of all the acute phase proteins examined and mentioned in the main text. Relative abundance values are expressed in arbitrary units.
The above proteins are all termed “positive” acute phase reactants in that their concentrations rise rapidly to as much as one or two orders of magnitude above resting levels in blood when mammals undergo an acute phase response to infection or inflammation (Parham, 2021). We saw no evidence of such increases during our sampling in any of the pups or their mothers, so we assume that they suffered no microbial or inflammatory disease during that time.
Proteins that may fall in concentration during acute phase responses, termed “negative” acute phase reactants, include serum albumin (but which was initially at higher than maternal levels, then fell), serotransferrin (which was also initially high and remained around maternal levels), and retinol (vitamin A)-binding protein (which was very low at first (Supplementary Figure 2). Serotransferrin was at similar levels in mothers and pups (Supplementary Figure 2) but may not be useful here as an indicator of innate immunity because it acts as an iron carrier in plasma, and its other roles in neonates may include to supply iron to muscles in the rapid increase in muscle myoglobin that occurs after birth in seals (Geiseler et al., 2013; Somo et al., 2015; Blix, 2018).
There are several other proteins that are not considered to be acute phase proteins per se but nevertheless appear to be associated with innate protective processes such as inflammation, healing, and tissue repair. One of these, Alpha 2-HS glycoprotein (fetuin-A) was of particular note in that it was present at exceptionally high levels in pups relative to their mothers (Supplementary Figure 2). While it has been argued to be an acute phase protein in some reports, it is usually found to decrease during such a response, so would be termed a negative acute phase reactant (Lebreton et al., 1979; Abiodun and Olomu, 1991; Dziegielewska et al., 1992). It is a multifunctional protein that has roles including in glucose metabolism and bone formation, so might be at high levels in pups in connection with birth and transition from total physiological dependency on their mothers to independence (Robinson and Teran-Garcia, 2016).
The heatmaps in Figure 2 and Supplementary Figure 2B emphasize the relative overall paucity of acute phase proteins in the pups that is particularly apparent at 2 days after birth, with only two notable exceptions (alpha-2-macroglobulin and alpha-1-antitrypsin). The hierarchical cluster included on the heatmap diagram emphasizes the separation between pups’ and mothers’ sera at all times. This is emphasized in a VIP analysis that also indicates which acute phase proteins best discriminate pup and maternal sera in terms of concentrations and how they change (Supplementary Figure 2C). PCA of the same data (Supplementary Figure 2D) further emphasizes the distinctiveness of pups’ sera at all times of sampling with only minor if any relative changes in the mothers.
How general are these findings? Perinatal levels of acute phase proteins have been observed to be low in several species of mammal, for instance rabbits, humans and sheep (Baker and Long, 1990; Kanakoudi et al., 1995; Rygg et al., 1996; Liberatori et al., 1997; Mcnamara and Alcorn, 2002; Mithal et al., 2017; Dinler et al., 2020), but to our knowledge this is the first evidence of a similar trend in a Carnivoran. The unique feature in grey and potentially other phocid seals is that this applies where protection against infection by antibodies may be initially non-existent and then minimal until parental care and lactational support ceases.
Complement—Depletion and Early and Late Pathway Divergence
The complement system bridges innate and adaptive immune systems, and complement proteins are abundant in blood plasma. The initiator proteins of the alternative pathway of complement are responsive to conserved pathogen molecular patterns (such as the surfaces of bacteria, fungi, and helminths), as is the lectin pathway, whereas the classical pathway is activated by antigen:antibody complexes (Parham, 2021). All three pathways converge on the central C3 component leading to the release of inflammatory mediators and activation of the late components. The late components assemble on the surface of a target cell to form the membrane attack complex that can lyse target cells, or to act as opsonins that provoke phagocytosis.
As with acute phase proteins, the complement proteins exhibited dramatic differences in the pattern of changes in the serum of grey seal pups with time after birth (Figure 3 and Supplementary Figure 3A). There was, however, a clear distinction between those of which there was a consistent relative scarcity and those that were consistently equivalent to maternal levels in pups throughout the sampling period. Moreover, this demarcation matched their position and roles in the activation and effector stages of complement pathways. The early components in both pathways that engage in the initial activation and amplification cascade (e.g., C1, C4, Factor B), and the central C3 protein, were consistently at levels similar to those in the mothers, or slightly higher. In marked contrast, the late components that assemble the membrane attack complex and release of inflammatory peptides were consistently at low levels (C5, C6, C7, C8, C9), as also were complement control/regulatory proteins (Factors H, Factor I, C4b-binding protein). There is very little literature on the ontogeny of serum complement factors in animals except for humans, in which the pattens we see in seal pups are similar (Georgountzou and Papadopoulos, 2017)—as already noted, humans are born with considerable antibody protection.
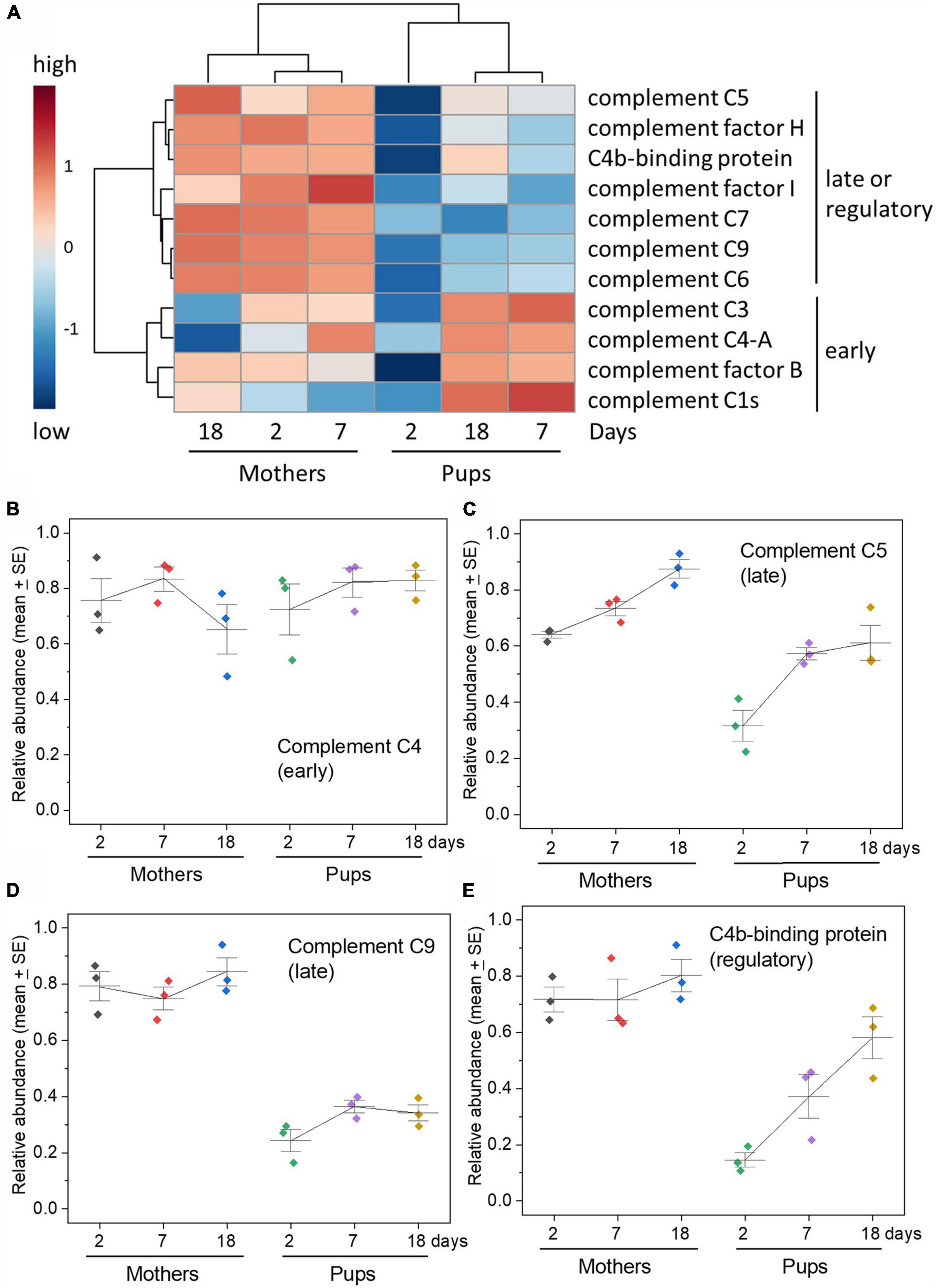
Figure 3. Complement pathways components—disparity between early versus late components of the pathways in relative abundance. (A) Heatmap of relative concentrations of complement pathway components obtained by TMT proteomics along with hierarchical clustering. Note the clear separation between mother and pup sera on all days indicated also by the cluster dendrogram above the heatmap columns. The clustering dendrogram to the left shows the clear bifurcation in abundance and change in the early activation and amplification components (C3, C4, factor B, C1) versus the late, lytic effector, inflammatory components, and control/inhibitory factors (C5, factor H, factor I, C7, C9, C6). (B–E) Changes in selected complement components to illustrate the difference between early and late components and also control/regulatory proteins. C4 is part of the amplification stage after initiation either by antigen:antibody complexes or the lectin pathway; C5 follows C3 activation and binds to a target cell upon which the membrane attack complex builds, which involves C9; C4b-binding protein is a complement control protein that inhibits activities of C4 and also C3. See Supplementary Figure 3 for graphs for all complement proteins detected. Relative abundance values are expressed in arbitrary units.
A heatmap comparing the temporal changes in complement components between mothers and pups illustrates the overall divide between the early and late components of the pathway and the separation between pups’ and mothers’ sera at all times, particularly soon after birth (Figure 3 and Supplementary Figure 3B). VIP analysis isolates which complement components best exhibit distinguishing features between maternal and pup sera, picking out the late components and complement control proteins for note (Supplementary Figure 3C). PCA shows that the pup complement protein profiles are distinct from the consistent profiles of the mothers, especially so on day 2 after birth (Supplementary Figure 3D).
Concluding Remarks
Despite their otherwise well-developed state at birth, and little or no systemic protection from maternal antibodies, the neonates of grey seals exhibit a conspicuously immature accumulation of innate immune proteins in their sera. This was clear at the earliest time after birth that we could sample and improved only gradually if at all before weaning and the termination of any continued immunological support garnered from milk.
The question now is—how do the pups of true seals survive without the considerable maternal antibody protection typical of land Carnivoran neonates in the apparent absence of an enhanced innate immunity? This question has recently become more pertinent with the discovery that terrestrial Carnivora appear to have undergone a loss of immune inflammasome pathways that should compromise innate and adaptive immunity (Digby et al., 2021), but whether this is also true in seals in unknown. The absence of antibody neonatally would normally be lethal to a land mammal (Brambell, 1970). It might be argued that many Phocinae seals, including some populations of grey seals, are ice-breeders that may not be exposed to many pathogens in their breeding sites. But that would be inconsistent with their success in environments where they would not be so protected, such as in crowded in temperate zone colonies that are ice-free, although pup survival is nevertheless good (Baker, 1988; Baker and Baker, 1988; Hall et al., 2001). Colonial breeding is likely to enhance transmission of pathogens, and colonially breeding birds (which obtain maternal antibody through egg yolk) appear to have evolved enhanced levels of immune protection (Moller and Erritzoe, 1996; Moller et al., 2001).
While one might expect that lower concentrations of innate immunity proteins would predispose to sensitivity to microbial infection, we did not carry out functional tests of the sera we sampled, such as bacterial killing, or opsonization to enhance phagocyte activity, and such assays would be a valuable future examination of the ontogeny of seal pup immunity. As already said, we saw no evidence of an acute phase response in any of our subjects indicated by a dramatic increase in innate immunity proteins. Although we are unwilling to do so on our wild seal pups, responses to an infection stimulus in neonates could be tested by administering an infective dose of a bacterium or a virus, or simple pyrogens such as bacterial lipopolysaccharide (LPS), whereupon dramatic increases in all acute phase proteins should occur, as has been done in other species (Voigt et al., 2020). A better way would be to sample animals undergoing natural infections. Of the latter, aside from the fundamental difference between seals and other eutherians being of intrinsic interest, the susceptibility of colonially breeding seal populations to viral epidemics such as phocine morbilliviruses (Duignan et al., 2014; Jo et al., 2018) indicates the importance of better understanding the distinctiveness of immune protection in their neonates and juveniles.
If other protective factors are at work in seals, then what are they? Seal milk will provide many protective factors, including immunoglobulins (Marquez et al., 2003; Lowe et al., 2017), but their effects are likely to be confined predominately to the gastrointestinal tract unless seals possess an FcRn-like system for translocation of IgG from the gut to blood as mentioned above. In other words, are the immune factors that are usually taken as crucial for survival more redundant than realized, and are other protective factors at play? One obvious candidate would be an enhanced cellular immunity and it has been reported in grey seals that the numbers of lymphocytes and neutrophils increase steadily until about day 15 after birth then plateau, and a similar situation applies in elephant seal pups which exhibit unusually high levels of circulating leukocytes (Hall et al., 2003). The unusual lack of immunoglobulins would, however, remain surprising given the degree to which the anti-microbial functions of neutrophils and monocytes, for instance, depend on antibodies (Parham, 2021).
So much for the detrimental effects that would be predicted from a paucity of innate and antibody-based adaptive immunity in phocid neonates. But why might phocids have diverged from the presumably better-protected terrestrial Carnivoran relatives (including other caniforms) in bulk transfer of maternal immunoglobulins? One advantage may be that without antibodies from their mothers (via placenta, colostrum, or milk), and a paucity of cell-lytic complement components, then they would not be at risk of hemolytic disease of the new-born. That phenomenon is due to differences in erythrocyte surface antigens (blood groups) between mother and offspring that can result in lethal hemolysis in a fetus or neonate—such as rhesus incompatibility in humans (Young et al., 1951; Brambell, 1970; Giger and Casal, 1997; Silvestre-Ferreira and Pastor, 2010; Parham, 2021). Blood group determinant molecules are associated with relative resistance or susceptibility to pathogens, such as malaria parasites in humans (Fumagalli et al., 2009; Zimmerman et al., 2013; Czerwinski, 2015; Abegaz, 2021; Arend, 2021). A balanced polymorphism within a population will, like all immune polymorphisms, be advantageous to a subset of members of an outbreeding population faced with diverse and rapidly evolving pathogens (Fumagalli et al., 2009). Having no risk of hemolytic disease of the new-born might therefore release a species from constraints that might select against polymorphism that may otherwise be useful. And this might be an advantage in the face of exposure to infective pathogens in a colonially breeding species that is exposed to pathogens in both the marine and terrestrial environments.
So, the main questions needing now to be addressed are, firstly, how do phocid seal pups survive pathogen assault whilst being seemingly so atypically unprotected amongst eutherians and even their close Carnivoran relatives, and, secondly, what might be the selective advantage to this divergence?
Data Availability Statement
The original contributions presented in the study are publicly available. This data can be found here: https://figshare.com/s/17f9d3f8f4188551faed.
Ethics Statement
Work involving animals in this study was licensed under the United Kingdom Home Office project 60/4009 or preceding versions and conformed to the United Kingdom Animals (Scientific Procedures) Act, 1986. Research was approved by the University of St Andrews Animal Welfare and Ethics Committee.
Author Contributions
MK and PP conceived, designed, and coordinated the study. PP organized collection of samples, carried out fieldwork, and acquired funding and fieldwork permits. MK carried out the protein gel electrophoresis and processed samples for submission to proteomics, carried out statistical analysis, and created the figures. MK wrote the manuscript with edits by PP. SMcG performed the TMT proteomics analysis and genome searches with advice from RB. MK and SMcG analyzed the data. All authors contributed amendments to drafts of the manuscript and approved the final version.
Funding
The work was funded from core support given to the Sea Mammal Research Unit, Scottish Oceans Institute from the Natural Environmental Research Council (United Kingdom).
Conflict of Interest
The authors declare that the research was conducted in the absence of any commercial or financial relationships that could be construed as a potential conflict of interest.
Publisher’s Note
All claims expressed in this article are solely those of the authors and do not necessarily represent those of their affiliated organizations, or those of the publisher, the editors and the reviewers. Any product that may be evaluated in this article, or claim that may be made by its manufacturer, is not guaranteed or endorsed by the publisher.
Acknowledgments
We are grateful to all involved in fieldwork, particularly Simon Moss. Scottish National Heritage granted permits for working on the Isle of May. We thank to Monika Mihm, Neil Evans, and David Watson for advice.
Supplementary Material
The Supplementary Material for this article can be found online at: https://www.frontiersin.org/articles/10.3389/fevo.2021.802510/full#supplementary-material
Footnotes
References
Abegaz, S. B. (2021). Human ABO Blood Groups and Their Associations with Different Diseases. Biomed. Res. Internat. 2021:2021. doi: 10.1155/2021/6629060
Abiodun, P. O., and Olomu, I. N. (1991). Serum alpha-2-HS-glycoprotein levels in neonatal infections. Biol. Neonat. 60, 114–117. doi: 10.1159/000243396
Arend, P. (2021). Why blood group A individuals are at risk whereas blood group O individuals are protected from SARS-CoV-2 (COVID-19) infection: A hypothesis regarding how the virus invades the human body via ABO(H) blood group-determining carbohydrates. Immunobiology 2021:226. doi: 10.1016/j.imbio.2020.152027
Baker, J. R. (1988). Further-studies on grey seal (Halichoerus grypus) pup mortality on North Rona. Br. Vet. J. 144, 497–506. doi: 10.1016/0007-1935(88)90090-5
Baker, J. R., and Baker, R. (1988). Effects of environment on grey seal (Halichoerus grypus) pup mortality - Studies on the Isle of May. J. Zool. 216, 529–537. doi: 10.1111/j.1469-7998.1988.tb02449.x
Baker, R. D., and Long, S. (1990). Acute phase proteins in neonatal rabbits - Diminished c-reactive protein response. J. Pediat. Gastro. Nutr. 11, 534–541. doi: 10.1097/00005176-199011000-00015
Bennett, K. A., Mcconnell, B. J., Moss, S. E. W., Speakman, J. R., Pomeroy, P. P., and Fedak, M. A. (2010). Effects of Age and Body Mass on Development of Diving Capabilities of Gray Seal Pups: Costs and Benefits of the Postweaning Fast. Physiolog. Biochem. Zool. 83, 911–923. doi: 10.1086/656925
Blix, A. S. (2018). Adaptations to deep and prolonged diving in phocid seals. J. Exp. Biol. 2018, 221. doi: 10.1242/jeb.182972
Brambell, F. W. R. (1970). The transmission of passive immunity from mother to young. New York, NY: American Elsevier Publishing Company, Inc.
Campisciano, G., Quadrifoglio, M., Comar, M., De Seta, F., Zanotta, N., Ottaviani, C., et al. (2020). Evidence of bacterial DNA presence in chorionic villi and amniotic fluid in the first and second trimester of pregnancy. Future Microb. 16, 801–810. doi: 10.2217/fmb-2020-0243
Ceron, J. J., Eckersall, P. D., and Martinez-Subiela, S. (2005). Acute phase proteins in dogs and cats: current knowledge and future perspectives. Vet. Clin. Pathol. 34, 85–99. doi: 10.1111/j.1939-165x.2005.tb00019.x
Claus, M. A., Levy, J. K., Macdonald, K., Tucker, S. J., and Crawford, P. C. (2006). Immunoglobulin concentrations in feline colostrum and milk, and the requirement of colostrum for passive transfer of immunity to neonatal kittens. J. Fel. Med. Surg. 8, 184–191. doi: 10.1016/j.jfms.2006.01.001
Cray, C., Zaias, J., and Altman, N. H. (2009). Acute Phase Response in Animals: A Review. Comparat. Med. 59, 517–526.
Czerwinski, M. (2015). Blood groups - minuses and pluses Do the blood group antigens protect us from infectious diseases? Post. Hig. I Med. Dosw. 69, 703–722. doi: 10.5604/17322693.1158795
Digby, Z., Tourlomousis, P., Rooney, J., Boyle, J. P., Bibo-Verdugo, B., Pickering, R. J., et al. (2021). Evolutionary loss of inflammasomes in the Carnivora and implications for the carriage of zoonotic infections. Cell Rep. 2021:36. doi: 10.1016/j.celrep.2021.109614
Dinler, C., Tuna, G. E., Ay, E., Ulutas, B., Voyvoda, H., and Ulutas, P. A. (2020). Reference intervals for serum amyloid A, haptoglobin, ceruloplasmin, and fibrinogen in apparently healthy neonatal lambs. Vet. Clin. Pathol. 49, 484–490. doi: 10.1111/vcp.12883
Duignan, P. J., Van Bressem, M.-F., Baker, J. D., Barbieri, M., Colegrove, K. M., De Guise, S., et al. (2014). Phocine Distemper Virus: Current Knowledge and Future Directions. Viruses-Basel 6, 5093–5134. doi: 10.3390/v6125093
Dziegielewska, K. M., Brown, W. M., Gould, C. C., Matthews, N., Sedgwick, J. E. C., and Saunders, N. R. (1992). Fetuin - An acute phase protein in cattle. J. Comp. Physiol. B-Biochem. Syst. Env. Physiol. 162, 168–171. doi: 10.1007/BF00398343
Eckersall, P. D., and Bell, R. (2010). Acute phase proteins: Biomarkers of infection and inflammation in veterinary medicine. Vet. J. 185, 23–27. doi: 10.1016/j.tvjl.2010.04.009
Ehlting, C., Wolf, S. D., and Bode, J. G. (2021). Acute-phase protein synthesis: a key feature of innate immune functions of the liver. Biolog. Chem. 402, 1129–1145. doi: 10.1515/hsz-2021-0209
Ferreira, A. P. S., Martinez, P. E., Colares, E. P., Robaldo, R. B., Berne, M. E. A., Filho, K. C. M., et al. (2005). Serum immunoglobulin G concentration in Southern elephant seal, Mirounga leonina (Linnaeus, 1758), from Elephant island (Antarctica): Sexual and adrenal steroid hormones effects. Vet. Immunol. Immunopathol. 106, 239–245. doi: 10.1016/j.vetimm.2005.02.024
Fumagalli, M., Cagliani, R., Pozzoli, U., Riva, S., Comi, G. P., Menozzi, G., et al. (2009). Widespread balancing selection and pathogen-driven selection at blood group antigen genes. Genome Res. 19, 199–212. doi: 10.1101/gr.082768.108
Geiseler, S. J., Blix, A. S., Burns, J. M., and Folkow, L. P. (2013). Rapid postnatal development of myoglobin from large liver iron stores in hooded seals. J. Exp. Biol. 216, 1793–1798. doi: 10.1242/jeb.082099
Georgountzou, A., and Papadopoulos, N. G. (2017). Postnatal innate immune Development: From Birth to Adulthood. Front. Immunol. 2017:8. doi: 10.3389/fimmu.2017.00957
Giger, U., and Casal, M. L. (1997). Feline colostrum - friend or foe: maternal antibodies in queens and kittens. J. Reprod. Fert. 1997, 313–316.
Gundling, W. E. Jr., and Wildman, D. E. (2015). A review of inter- and intraspecific variation in the eutherian placenta. Philosoph. Trans. R. Soc. B-Biol. Sci. 2015:370. doi: 10.1098/rstb.2014.0072
Hall, A. J., Englehard, G. H., Brasseur, S., Vecchione, A., Burton, H. R., and Reijnders, P. J. H. (2003). The immunocompetence handicap hypothesis in two sexually dimorphic pinniped species - is there a sex difference in immunity during early development? Dev. Comp. Immunol. 27, 629–637. doi: 10.1016/s0145-305x(03)00029-6
Hall, A. J., Mcconnell, B. J., and Barker, R. J. (2001). Factors affecting first-year survival in grey seals and their implications for life history strategy. J. Anim. Ecol. 70, 138–149. doi: 10.1111/j.1365-2656.2001.00468.x
Hall, A. J., Mcconnell, B. J., and Barker, R. J. (2002). The effect of total immunoglobulin levels, mass and condition on the first-year survival of Grey Seal pups. Funct. Ecol. 16, 462–474. doi: 10.1046/j.1365-2435.2002.00649.x
Jo, W. K., Osterhaus, A. D. M. E., and Ludlow, M. (2018). Transmission of morbilliviruses within and among marine mammal species. Curr. Opin. Virol. 28, 133–141. doi: 10.1016/j.coviro.2017.12.005
Kanakoudi, F., Drossou, V., Tzimouli, V., Diamanti, E., Konstantinidis, T., Germenis, A., et al. (1995). Serum concentrations of 10 acute-phase proteins in healthy term and preterm infants from birth to age 6 months. Clin. Chem. 41, 605–608. doi: 10.1093/clinchem/41.4.605
King, D. P., Lowe, K. A., Hay, A. W. M., and Evans, S. W. (1994). Identification, characterization, and measurement of immunoglobulin concentrations in grey (Haliocherus grypus) and common (Phoca vitulina) seals. Dev. Comp. Immunol. 18, 433–442. doi: 10.1016/0145-305x(94)90008-6
King, D. P., Sanders, J. L., Nomura, C. T., Stoddard, R. A., Ortiz, C. L., and Evans, S. W. (1998). Ontogeny of humoral immunity in northern elephant seal (Mirounga angustirostris) neonates. Comparat. Biochem. Physiol. B-Biochem. Mole. Biol. 121, 363–368. doi: 10.1016/s0305-0491(98)10118-9
Krakowka, S., Long, D., and Koestner, A. (1978). Influence of transplacentally acquired antibody on neonatal susceptibility to canine distemper virus in gnotobiotic dogs. J. Infect. Dis. 137, 605–608. doi: 10.1093/infdis/137.5.605
Langer, P. (2008). The phases of maternal investment in eutherian mammals. Zoology 111, 148–162. doi: 10.1016/j.zool.2007.06.007
Langer, P. (2009). Differences in the composition of colostrum and milk in eutherians reflect differences in immunoglobulin transfer. J. Mamm. 90, 332–339. doi: 10.1644/08-mamm-a-071.1
Lebreton, J. P., Joisel, F., Raoult, J. P., Lannuzel, B., Rogez, J. P., and Humbert, G. (1979). Serum concentration of human alpha-2-HS glycoprotein during the inflammatory process - evidence that alpha-2-HS glycoprotein is a negative acute-phase reactant. J. Clin. Investig. 64, 1118–1129. doi: 10.1172/JCI109551
Levy, J. K., Crawford, P. C., Collante, W. R., and Papich, M. G. (2001). Use of adult cat serum to correct failure of passive transfer in kittens. J. Am. Vet. Med. Assoc. 219, 1401–1405. doi: 10.2460/javma.2001.219.1401
Liberatori, S., Bini, L., Defelice, C., Magi, B., Marzocchi, B., Raggiaschi, R., et al. (1997). Acute-phase proteins in perinatal human plasma. Electrophoresis 18, 520–526. doi: 10.1002/elps.1150180331
Lim, E. S., Rodriguez, C., and Holtz, L. R. (2018). Amniotic fluid from healthy term pregnancies does not harbor a detectable microbial community. Microbiome 2018:6.
Lowe, A. D., Bawazeer, S., Watson, D. G., Mcgill, S., Burchmore, R. J. S., Pomeroy, P. P., et al. (2017). Rapid changes in Atlantic grey seal milk from birth to weaning - immune factors and indicators of metabolic strain. Sci. Rep. 2017:7. doi: 10.1038/s41598-017-16187-7
Marquez, M. E. I, Carlini, A. R., Baroni, A. V., De Ferrer, P. A. R., Slobodianik, N. H., and Godoy, M. F. (2003). Shifts in immunoglobulin (IgG, IgM and IgA) levels in the milk of southern elephant seals, at Potter Peninsula, King George Island, Antarctica. Polar Biol. 26, 151–156. doi: 10.1007/s00300-002-0471-y
Marquez, M. E. I, Slobodianik, N. H., Deferrer, P. A. R., Carlini, A. R., Vergani, D. F., and Daneri, G. A. (1995). Immunoglobulin-a levels in southern elephant seal (Mirounga leonina) milk during the suckling period. Comparat. Biochem. Physiol. B-Biochem. Mole. Biol. 112, 569–572. doi: 10.1016/0305-0491(95)00101-8
Mayer, B., Doleschall, M., Bender, B., Bartyik, J., Bosze, Z., Frenyo, L. V., et al. (2005). Expression of the neonatal Fc receptor (FcRn) in the bovine mammary gland. J. Dairy Res. 72, 107–112. doi: 10.1017/s0022029905001135
Mcnamara, P. J., and Alcorn, J. (2002). Protein binding predictions in infants. Aaps Pharmsci. 2002:4. doi: 10.1208/ps040104
Mithal, L. B., Palac, H. L., Yogev, R., Ernst, L. M., and Mestan, K. K. (2017). Cord Blood Acute Phase Reactants Predict Early Onset Neonatal Sepsis in Preterm Infants. PLoS One 2017:12. doi: 10.1371/journal.pone.0168677
Moller, A. P., and Erritzoe, J. (1996). Parasite virulence and host immune defense: Host immune response is related to nest reuse in birds. Evolution 50, 2066–2072. doi: 10.1111/j.1558-5646.1996.tb03592.x
Moller, A. P., Merino, S., Brown, C. R., and Robertson, R. J. (2001). Immune Defense and Host Sociality: A Comparative Study of Swallows and Martins. Am. Natural. 158:136. doi: 10.1086/321308
Pereira, M., Valerio-Bolas, A., Saraiva-Marques, C., Alexandre-Pires, G., Da Fonseca, I. P., and Santos-Gomes, G. (2019). Development of Dog Immune System: From in Uterus to Elderly. Vet. Sci. 2019:6. doi: 10.3390/vetsci6040083
Perez-Munoz, M. E., Arrieta, M.-C., Ramer-Tait, A. E., and Walter, J. (2017). A critical assessment of the “sterile womb” and “in utero colonization” hypotheses: implications for research on the pioneer infant microbiome. Microbiome 2017:5. doi: 10.1186/s40168-017-0268-4
Poffenbarger, E. M., Olson, P. N., Chandler, M. L., Seim, H. B., and Varman, M. (1991). Use of adult dog serum as a substitute for colostrum in the neonatal dog. Am. J. Vet. Res. 52, 1221–1224.
Pomeroy, P. P., Fedak, M. A., Rothery, P., and Anderson, S. (1999). Consequences of maternal size for reproductive expenditure and pupping success of grey seals at North Rona, Scotland. J. Anim. Ecol. 68, 235–253. doi: 10.1046/j.1365-2656.1999.00281.x
Pomeroy, P. P., Green, N., Hall, A. J., Walton, M., Jones, K., and Harwood, J. (1996). Congener-specific exposure of grey seal (Halichoerus grypus) pups to chlorinated biphenyls during lactation. Can. J. Fish. Aquat. Sci. 53, 1526–1534. doi: 10.1139/f96-087
Rath, T., Kuo, T. T., Baker, K., Qiao, S.-W., Kobayashi, K., Yoshida, M., et al. (2013). The Immunologic Functions of the Neonatal Fc Receptor for IgG. J. Clin. Immunol. 33, S9–S17. doi: 10.1007/s10875-012-9768-y
Rehbinder, E. M., Carlsen, K. C. L., Staff, A. C., Angell, I. L., Landro, L., Hilde, K., et al. (2018). Is amniotic fluid of women with uncomplicated term pregnancies free of bacteria? Am. J. Obstetr. Gynecol. 2018:219. doi: 10.1016/j.ajog.2018.05.028
Robinson, K. N., and Teran-Garcia, M. (2016). From infancy to aging: Biological and behavioral modifiers of Fetuin-A. Biochimie 124, 141–149. doi: 10.1016/j.biochi.2015.12.016
Roopenian, D. C., and Akilesh, S. (2007). FcRn: the neonatal Fc receptor comes of age. Nat. Rev. Immunol. 7, 715–725. doi: 10.1038/nri2155
Ross, P. S., Deswart, R. L., Visser, I. K. G., Vedder, L. J., Murk, W., Bowen, W. D., et al. (1994). Relative immunocompetence of the newborn harbor seal, Phoca vitulina. Vet. Immunol. Immunopathol. 42, 331–348. doi: 10.1016/0165-2427(94)90077-9
Rygg, M., Alstad, H. K., and Marhaug, G. (1996). Developmental regulation of expression of rabbit C-reactive protein and serum amyloid A genes. Biochimica Et Biophysica Acta-Gene Struct. Expr. 1307, 89–96. doi: 10.1016/0167-4781(96)00019-x
Schulz, T. M., and Bowen, W. D. (2005). The evolution of lactation strategies in pinnipeds: A phylogenetic analysis. Ecol. Monogr. 75, 159–177. doi: 10.1890/04-0319
Silvestre-Ferreira, A. C., and Pastor, J. (2010). Feline neonatal isoerythrolysis and the importance of feline blood types. Vet. Med. Internat. 2010, 753726–753726. doi: 10.4061/2010/753726
Singh, A., and Mittal, M. (2020). Neonatal microbiome - a brief review. J. Mat. Fetal Neonatal Med. 33, 3841–3848. doi: 10.1080/14767058.2019.1583738
Somo, D. A., Ensminger, D. C., Sharick, J. T., Kanatous, S. B., and Crocker, D. E. (2015). Development of Dive Capacity in Northern Elephant Seals (Mirounga angustirostris): Reduced Body Reserves at Weaning Are Associated with Elevated Body Oxygen Stores during the Postweaning Fast. Physiol. Biochem. Zool. 88, 471–482. doi: 10.1086/682386
Stinson, L. F., Boyce, M. C., Payne, M. S., and Keelan, J. A. (2019). The Not-so-Sterile Womb: Evidence That the Human Fetus Is Exposed to Bacteria Prior to Birth. Front. Microb. 2019:10. doi: 10.3389/fmicb.2019.01124
Stoffel, M. H., Friess, A. E., and Hartmann, S. H. (2000). Ultrastructural evidence of transplacental transport of immunoglobulin G in bitches. J. Reproduct. Fert. 118, 315–326. doi: 10.1530/reprod/118.2.315
Voigt, C. C., Fritze, M., Lindecke, O., Costantini, D., Petersons, G., and Czirjak, G. A. (2020). The immune response of bats differs between pre-migration and migration seasons. Sci. Rep. 2020:10. doi: 10.1038/s41598-020-74473-3
Vos, A., Schaarschmidt, U., Muluneh, A., and Muller, I. (2003). Origin of maternally transferred antibodies against rabies in foxes (Vulpes vulpes). Vet. Rec. 153, 16–18. doi: 10.1136/vr.153.1.16
Westrom, B., Sureda, E. A., Pierzynowska, K., Pierzynowski, S. G., and Perez-Cano, F.-J. (2020). The Immature Gut Barrier and Its Importance in Establishing Immunity in Newborn Mammals. Front. Immunol. 2020:11. doi: 10.3389/fimmu.2020.01153
Wisniewski, J. R., Zougman, A., Nagaraj, N., and Mann, M. (2009). Universal sample preparation method for proteome analysis. Nat. Methods 6, 359–U360.
Wooding, P., and Burton, G. J. (2008). Comparative Placentation: Structures, Functions and Evolution. Berlin: Springer Verlag.
Yamada, T., Nagai, Y., and Matsuda, M. (1991). Changes in serum immunoglobulin values in kittens after ingestion of colostrum. Am. J. Vet. Res. 52, 393–396.
Young, L. E., Christian, R. M., Ervin, D. M., Davis, R. W., Obrien, W. A., Swisher, S. N., et al. (1951). Hemolytic disease in newborn dogs. Blood 6, 291–313.
Keywords: Atlantic grey seals, neonates, Halichoerus grypus, immunoglobulins, acute phase proteins, complement factors, innate immunity
Citation: McGill S, Burchmore RJS, Pomeroy PP and Kennedy MW (2022) Is a Little Enough? Paucity of Immune Proteins in Serum of Precocial Neonates of a Marine Carnivoran—the Atlantic Grey Seal. Front. Ecol. Evol. 9:802510. doi: 10.3389/fevo.2021.802510
Received: 26 October 2021; Accepted: 10 December 2021;
Published: 27 January 2022.
Edited by:
Jose A. Masero, University of Extremadura, SpainReviewed by:
Gábor Árpád Czirják, Leibniz Institute for Zoo and Wildlife Research (LG), GermanyMandy Keogh, NOAA Fisheries Alaska Region Protected Resources Division, United States
Copyright © 2022 McGill, Burchmore, Pomeroy and Kennedy. This is an open-access article distributed under the terms of the Creative Commons Attribution License (CC BY). The use, distribution or reproduction in other forums is permitted, provided the original author(s) and the copyright owner(s) are credited and that the original publication in this journal is cited, in accordance with accepted academic practice. No use, distribution or reproduction is permitted which does not comply with these terms.
*Correspondence: Patrick P. Pomeroy, pp6@st-andrews.ac.uk; Malcolm W. Kennedy, malcolm.kennedy@glasgow.ac.uk