- 1School of Life Sciences, University of Essex, Colchester, United Kingdom
- 2Fisheries Department, Falkland Islands Government, Stanley, Falkland Islands
- 3Marine Evolutionary Ecology, GEOMAR Helmholtz Centre for Ocean Research Kiel, Kiel, Germany
- 4Institut National de la Recherche Agronomique (INRA), Dijon, France
- 5Institute of Health and Wellbeing, University of Glasgow, Glasgow, United Kingdom
- 6The Centre for Environment and Climate Research, Lund University, Lund, Sweden
Cross-ecosystem subsidies are important as their recipients often rely on them to supplement in situ resource availability. Global warming has the potential to alter the quality and quantity of these subsidies, but our knowledge of these effects is currently limited. Here, we quantified the biomass and diversity of the invertebrates exchanged between freshwater streams and terrestrial grasslands in a natural warming experiment in Iceland. We sampled invertebrates emerging from the streams, those landing on the water surface, ground-dwelling invertebrates falling into the streams, and those drifting through the streams. Emerging invertebrate biomass or diversity did not change with increasing temperature, suggesting no effect of warming on aquatic subsidies to the terrestrial environment over the 1-month duration of the study. The biomass and diversity of aerial invertebrates of terrestrial origin landing on the streams increased with temperature, underpinned by increasing abundance and species richness, indicating that the greater productivity of the warmer streams may attract more foraging insects. The biomass of ground-dwelling invertebrates falling into the streams also increased with temperature, underpinned by increasing body mass and species evenness, suggesting that soil warming leads to terrestrial communities dominated by larger, more mobile organisms, and thus more in-fall to the streams. The biomass and diversity of terrestrial invertebrates in the drift decreased with temperature, however, underpinned by decreasing abundance and species richness, reflecting upstream consumption due to the higher energetic demands of aquatic consumers in warmer environments. These results highlight the potential for asynchronous responses to warming for reciprocal subsidies between aquatic and terrestrial environments and the importance of further research on warming impacts at the interface of these interdependent ecosystems.
Introduction
The Intergovernmental Panel on Climate Change (IPCC) has now unambiguously acknowledged the contribution of human activities to global warming, which is projected to surpass 1.5°C relative to Pre-industrial times over the next two decades (IPCC, 2021). These changes will not occur evenly across the planet, with land surfaces warming faster than the oceans and the Arctic region predicted to warm at more than two times the global rate (IPCC, 2021). Warming has already been demonstrated to have ecological consequences from the individual to the ecosystem level. For example, individuals, populations, and communities are widely shown to decrease in mean body size in response to warming (Daufresne et al., 2009; Gardner et al., 2011). Many taxa are also undergoing poleward shifts in their distributions (Hickling et al., 2006; Chen et al., 2011) and an earlier onset of reproductive events (Menzel et al., 2006) as they track their thermal optima in space and time, respectively. This will lead to novel communities and biodiversity loss (Lurgi et al., 2012; Trisos et al., 2020), as well as changes in community biomass and food web structure (Yvon-Durocher et al., 2011; O’Gorman et al., 2017, 2019).
Given the global nature of climate change, it is clear that the impacts of warming will not be constrained to any one ecosystem type, although the strength of the response may differ between marine, freshwater, and terrestrial systems (Forster et al., 2012; Häder and Barnes, 2019). Nonetheless, ecosystems are still largely studied as individual entities, disregarding the fact that many are tightly coupled by the energy, nutrients, and materials that flow across their boundaries (Spiller et al., 2010; Bartels et al., 2012; Bauer and Hoye, 2014). For example, riparian vegetation, fauna and other allochthonous inputs exert strong bottom-up effects on stream food webs (Bartels et al., 2012), whilst adult insects emerging from freshwaters play an important role as prey for terrestrial predators (Baxter et al., 2005; Gratton et al., 2008). This tight coupling of freshwater and terrestrial systems may result in subsidies constituting more than half the diet of the dominant predators in each environment (Nakano et al., 1999; Henschel et al., 2001). Increasing temperature may disrupt the reciprocal exchange of energy between ecosystems, however, with experiments in the tropics indicating that warming could reduce the flux of energy from aquatic to terrestrial environments, whilst enhancing the breakdown of terrestrial resources in freshwaters (Nash et al., 2021). Yet in spite of the emerging consensus that global change will alter cross-ecosystem linkages, we still know relatively little about the implications in a multispecies context (Larsen et al., 2016).
The timing of life cycle events can be highly synchronized between aquatic and terrestrial systems, with the emergence of aquatic invertebrates often occurring when resources are scarce in the riparian system (Nakano and Murakami, 2001). Warming could disrupt this link by speeding up aquatic larval development (Harper and Peckarsky, 2006), and the earlier emergence of adult flies (Hogg and Williams, 1996; Greig et al., 2012) could make a key aquatic subsidy unavailable at a time when terrestrial consumers are most reliant on it. Indeed, many terrestrial consumers have been shown to track the temporal dynamics of their aquatic resources (Kato et al., 2004; Paetzold et al., 2005) and may even experience reduced fitness in response to mis-matched peaks in the availability of those resources (Marczak and Richardson, 2008). The synchronicity of a subsidy with resource availability in the recipient ecosystem could thus determine the stability of consumer-resource dynamics (Takimoto et al., 2002; Leroux and Loreau, 2012). For instance, if peak aquatic insect emergence is seasonally offset with peak terrestrial invertebrate in-fall, the two neighboring ecosystems may fuel each other cyclically to maintain high levels of production (Nakano and Murakami, 2001).
Warming may also increase the voltinism of aquatic insects, leading to more generations per year and a potentially greater biomass emerging into terrestrial environments (Hannesdóttir et al., 2013). Such increases in the flux of freshwater subsidies have been shown to dramatically increase terrestrial arthropod biomass (Hoekman et al., 2011). Similarly, climatic warming over the past four decades has led to greater voltinism in butterflies and moths, which could result in more aerial invertebrates landing on the surface of freshwaters. If soil warming increases the activity of ground-dwelling invertebrates (Ottesen, 1985), this may also increase the biomass of terrestrial organisms falling into the aquatic environment. These terrestrial supplements to the diet of drift feeding fish (e.g., salmonids) could bolster top predator biomass in aquatic systems, which may in turn exert greater top-down control on their aquatic prey (Baxter et al., 2005). Over longer time frames, stronger top-down control in aquatic environments could reduce insect emergence and thus indirectly alter the biomass of their predators or prey and associated ecosystem functions in the terrestrial environment (Knight et al., 2005).
Organismal body size is widely predicted to decline in response to warming (Daufresne et al., 2009), although numerous exceptions to this general rule have been identified (Gardner et al., 2011; Rueger and Sommer, 2012; O’Gorman et al., 2017). Indeed, most studies to date have shown minimal effect of warming on the overall mean body size of emerging insects from freshwater environments (but see Sardina et al., 2017), albeit underpinned by contrasting effects on individual taxa (Wesner, 2012; Jonsson et al., 2015; McCauley et al., 2018; Nash et al., 2021). Furthermore, some studies have demonstrated a reduction in the emergent adult body size of dominant larvae in freshwaters, such as midge flies, mosquitoes, and mayflies (Sardina et al., 2017; Nash et al., 2021), whilst others show the potential for an increased proportion of larger, predatory individuals emerging from freshwaters (Wesner, 2012; Jonsson et al., 2015). Terrestrial recipients of aquatic larvae emerging as adult flies can be highly selective in terms of the body size of their prey, where small and large consumers generally prefer small- and large-bodied prey, respectively (Hering and Plachter, 1997; Buchanan et al., 2006). Therefore, changes in the body size of emerging insects could mean a shift in the size structure of the terrestrial consumer community (Stenroth et al., 2015).
The diversity of reciprocal subsidies could also change in response to warming. There has been a sharp rise in species extinctions during the Anthropocene (Johnson et al., 2017; IPBES, 2019), with global warming projected to accelerate the rate of species loss in the coming decades (Urban, 2015; Warren et al., 2018; Trisos et al., 2020). Regional differences may occur, with the fastest rates of biodiversity loss occurring in the Southern hemisphere (Urban, 2015) whereas predictions for Europe show that species richness may actually increase with warming across a broad range of taxa and ecosystems (Pilotto et al., 2020). Diversity patterns may be complicated by individual thermal tolerances (Currie et al., 2004), with cold stenotherms potentially being replaced by thermophilic taxa (Daufresne et al., 2004). This may preserve total species richness but lead to changes in community composition and dominance patterns. For example, species richness is maintained across large geothermal stream temperature gradients, but evenness declines as highly abundant thermophiles dominate the warmest communities (Friberg et al., 2009). Changes in aquatic community composition could have negative effects on riparian specialists, which often rely on particular species emerging from the aquatic environment (Soininen et al., 2015). Loss of aquatic fish species has even been shown to alter pollination of riparian plants through cascading effects (Knight et al., 2005). Thus, warming-induced changes in diversity are likely to percolate across ecosystem boundaries.
Here, we used natural stream and soil temperature gradients to examine the reciprocal exchange of invertebrates between freshwater streams and their riparian grasslands. We hypothesized that: (1) the biomass of aquatic insects emerging into the terrestrial landscape, and of terrestrial invertebrates entering freshwater streams will increase with temperature, underpinned by increasing abundance but decreasing body size; (2) the diversity of aquatic insects emerging into the terrestrial landscape, and of terrestrial invertebrates entering freshwater streams will decrease with temperature, underpinned by fewer taxa and a less even distribution of abundances among taxa.
Materials and Methods
Study Site
The study was carried out in the Hengill geothermal area, near Reykjavík, Iceland (N 64°03; W 21°18), which has seen extensive characterization of community structure in the freshwater streams (Friberg et al., 2009; Woodward et al., 2010; O’Gorman et al., 2017, 2019) and surrounding terrestrial landscape (Robinson et al., 2018, 2021; Eitzinger et al., 2021; Warner et al., 2021) over the past decade. Differential heating by geothermal activity produces a gradient of stream and soil temperatures, but with minimal variation in their physical and chemical properties (Friberg et al., 2009; Robinson et al., 2021). The study site has thus been identified as an ideal natural experiment for studying impacts of warming on wild communities (Woodward et al., 2010; O’Gorman et al., 2014). For this study, we chose eight streams and the terrestrial habitat bordering them, which evenly spanned a temperature gradient of 5–20°C in both environments. Stream and soil temperatures were measured from May 13th to July 3rd (the duration of the field expedition) using temperature loggers (Maxim Integrated DS1921G Thermocron iButtons). One temperature logger was placed in each stream, with an additional logger placed 5 cm beneath the soil surface, 1 m from the stream edge at each of three sampling stations (an upper, mid, and lower reach, each separated by approximately 15 m) on the left and right banks of each stream. The mean temperature was calculated for each stream and each bank for the entire duration of the study period (Figure 1).
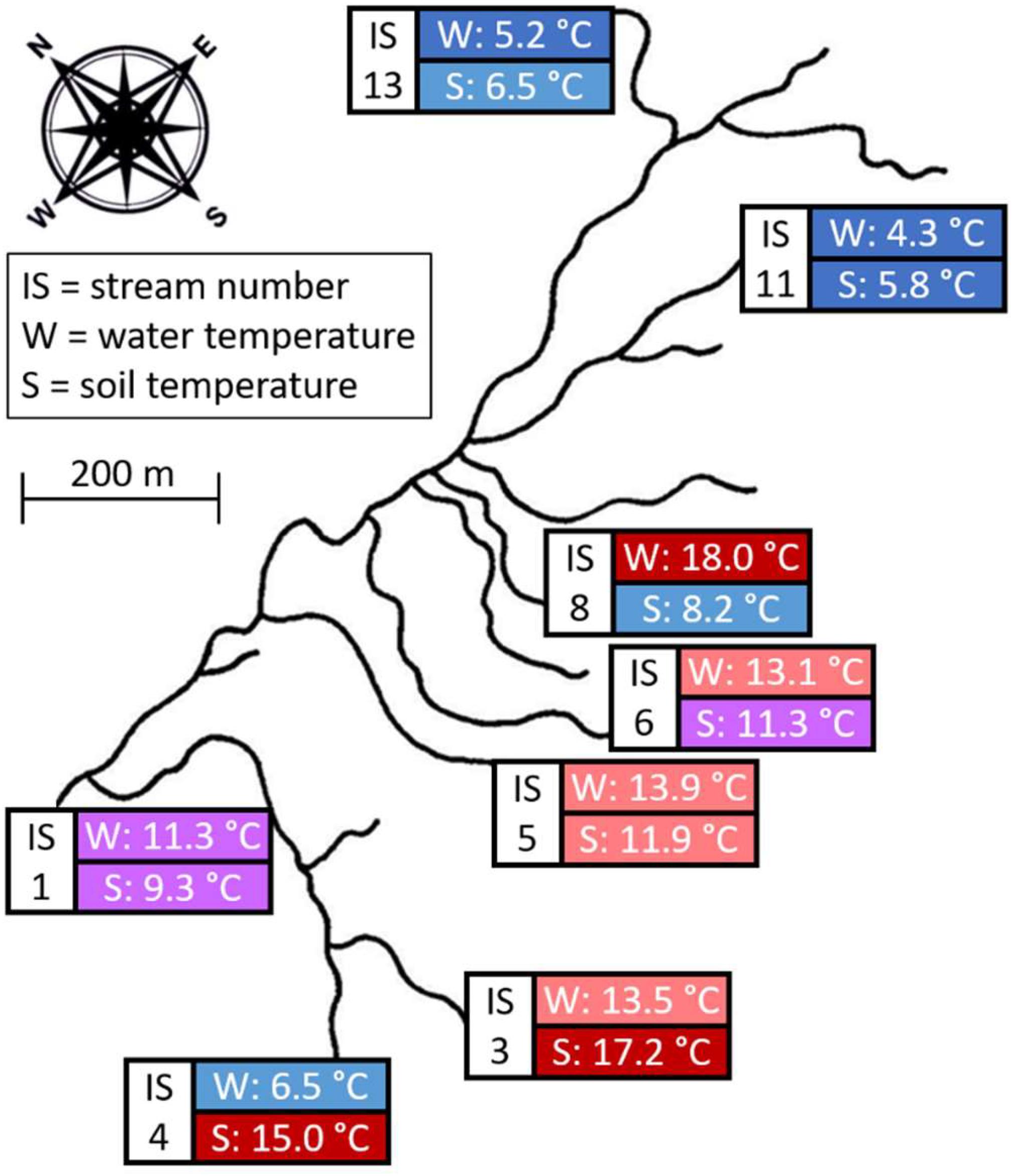
Figure 1. Map of the study site, with sampled streams and soil habitats indicated by colored boxes. Stream numbers correspond to the coding used in previous Hengill publications (e.g., Woodward et al., 2010). The mean water (W) and soil (S) temperatures during the study period are also provided and colored accordingly, ranging from dark blue (cold) to dark red (warm).
Field Sampling
The main aquatic subsidy to the terrestrial system is the emergence of larval insects as adults. Thus, emergence traps were used to quantify this output (60 × 60 × 60 cm; Figure 2a), with one trap placed in the middle and lower reaches of each stream. Steel bars were used to secure the four corners and stones were placed along the inner mesh lip to anchor each trap to the stream bed. Every trap contained a 250 ml collection jar, which was filled with 70% ethanol to kill and preserve all invertebrates and a few drops of surfactant (washing-up liquid) to break the surface tension. Emergence traps were established on June 2nd and samples were collected on July 3rd. Note that emergence of freshwater invertebrates in the Hengill system occurs from March to August, but the peak emergence for many aquatic invertebrate taxa coincides with this sampling period (Hannesdóttir et al., 2013).
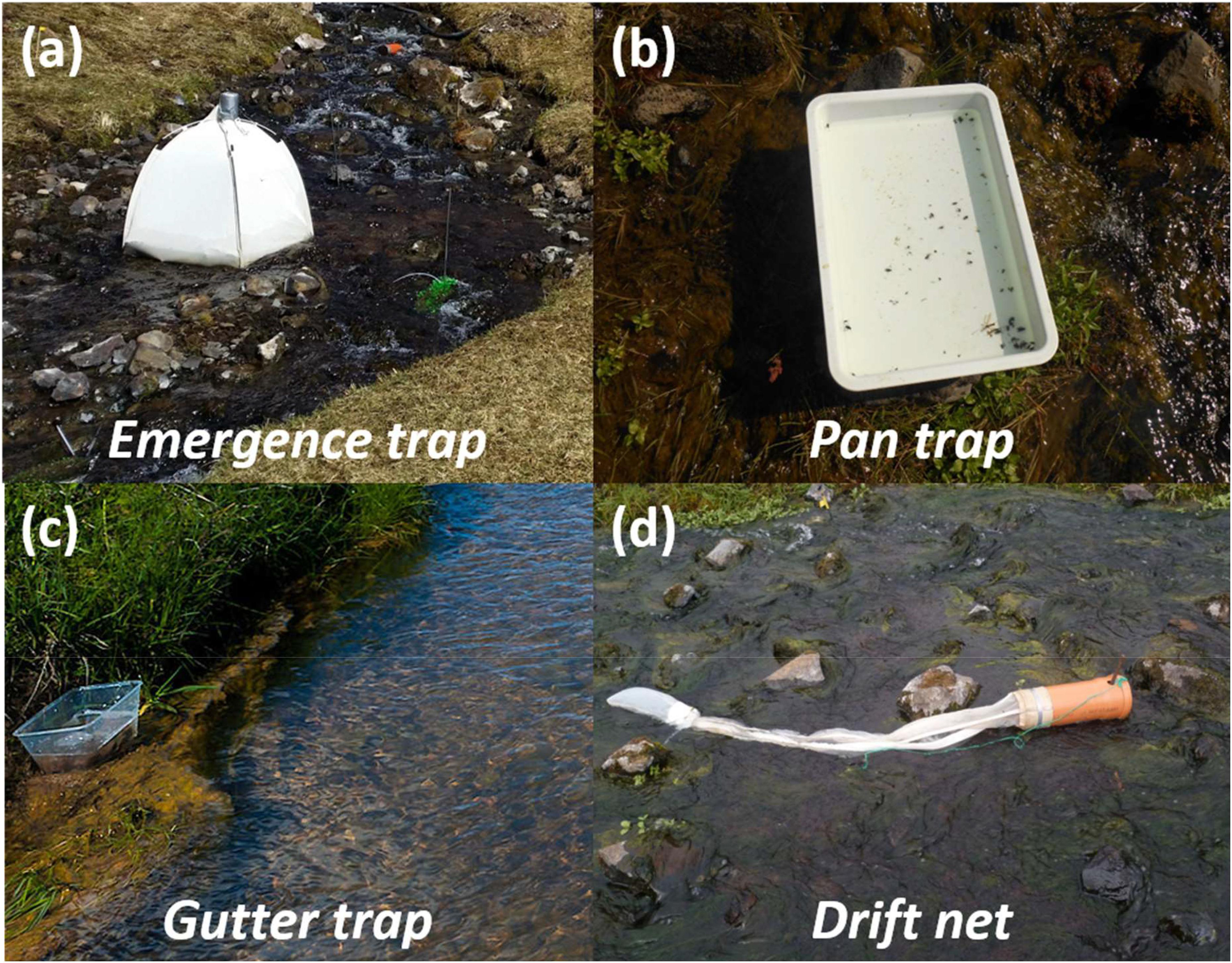
Figure 2. Photos of the traps used to sample reciprocal subsidies between the freshwater and terrestrial environments. (a) Emergence traps intercepted aquatic larvae emerging as adult flies into the terrestrial landscape. (b) Pan traps quantified terrestrial adult flies landing on the surface of the streams. (c) Gutter traps captured ground–dwelling invertebrates falling from the banks into the streams. (d) Drift nets collected terrestrial invertebrates drifting down the streams.
The vegetation in the Hengill region is representative of a low Arctic community, dominated by herbaceous perennial flowering plants and bryophytes, with no trees or shrubs and thus no leaf litter input to the streams. Therefore, the main terrestrial subsidy to the aquatic system consists of adult flies landing on the water surface, or ground-dwelling invertebrates falling into the streams, which were sampled using pan traps, gutter traps, and drift nets (see below). All of the sampling equipment used to quantify terrestrial input was set out for 48 h at a time when no rain was forecast to avoid overflow and loss of specimens.
The terrestrial subsidy of adult flies landing on the water surface was quantified using pan traps (Figure 2b), with one trap placed in the center of the lower, middle, and upper reach of each stream. The traps consisted of white plastic trays (35.6 × 25.4 × 5 cm), balanced on a pedestal built up from the stream bed with rocks, such that the base of the tray was just above the water surface. Each trap was half-filled with stream water and a few drops of surfactant. Upon collection, the pan traps were emptied into a 250 μm sieve, and the invertebrates were washed into a 50 ml tube with 70% ethanol. Whilst the pan traps also sampled aquatic flies landing on the streams, only material of terrestrial origin was quantified in this study.
The terrestrial subsidy of ground-dwelling invertebrates falling into the streams from the banks was quantified using gutter traps (Figure 2c). These consisted of clear plastic aquaria (18 × 11.7 × 11 cm), tightly aligned with the side of a bank bordering each stream and secured in place with rocks if needed. The traps were half-filled with stream water and a few drops of surfactant. One gutter trap was placed within the lower, middle, and upper reach of each stream, with the bank (left or right) randomly chosen on each occasion, ensuring that at least one trap was placed on each bank. Whilst aerial insects were also found in the gutter traps, only material of ground-dwelling terrestrial origin was quantified in this study.
The terrestrial subsidy of invertebrates drifting down the streams was quantified using drift nets, comprised of PVC tubes (40 cm long; 11 cm diameter), sealed at one end with a 250 μm mesh bag (Figure 2d). The drift nets were secured to the stream bed using a steel bar, with rocks propped beneath the tube to ensure it was only partially submerged, allowing stream water to flow freely through the opening. One drift net was established in the lower, middle, and upper reach of each stream. Whilst the drift nets also sampled aquatic invertebrates drifting through the streams, only material of terrestrial origin was quantified in this study.
Data Collection
Organisms were identified to species level where possible, except for slugs (genus level) flies and wasps (family level), and mites, springtails, and worms (order level). The body length of all invertebrates was measured from the tip of the mandibles to the base of the abdomen. Up to 50 individuals were measured per taxon per sample. Damaged individuals were not measured, but still enumerated by counting only the heads. New length-weight relationships were described for several families of flies by drying measured individuals in a drying oven at 80°C for 48 h and measuring body mass in milligrams of dry weight on a fine mass balance. Dry body masses were estimated for the remaining taxa from established length-weight relationships (Table 1).
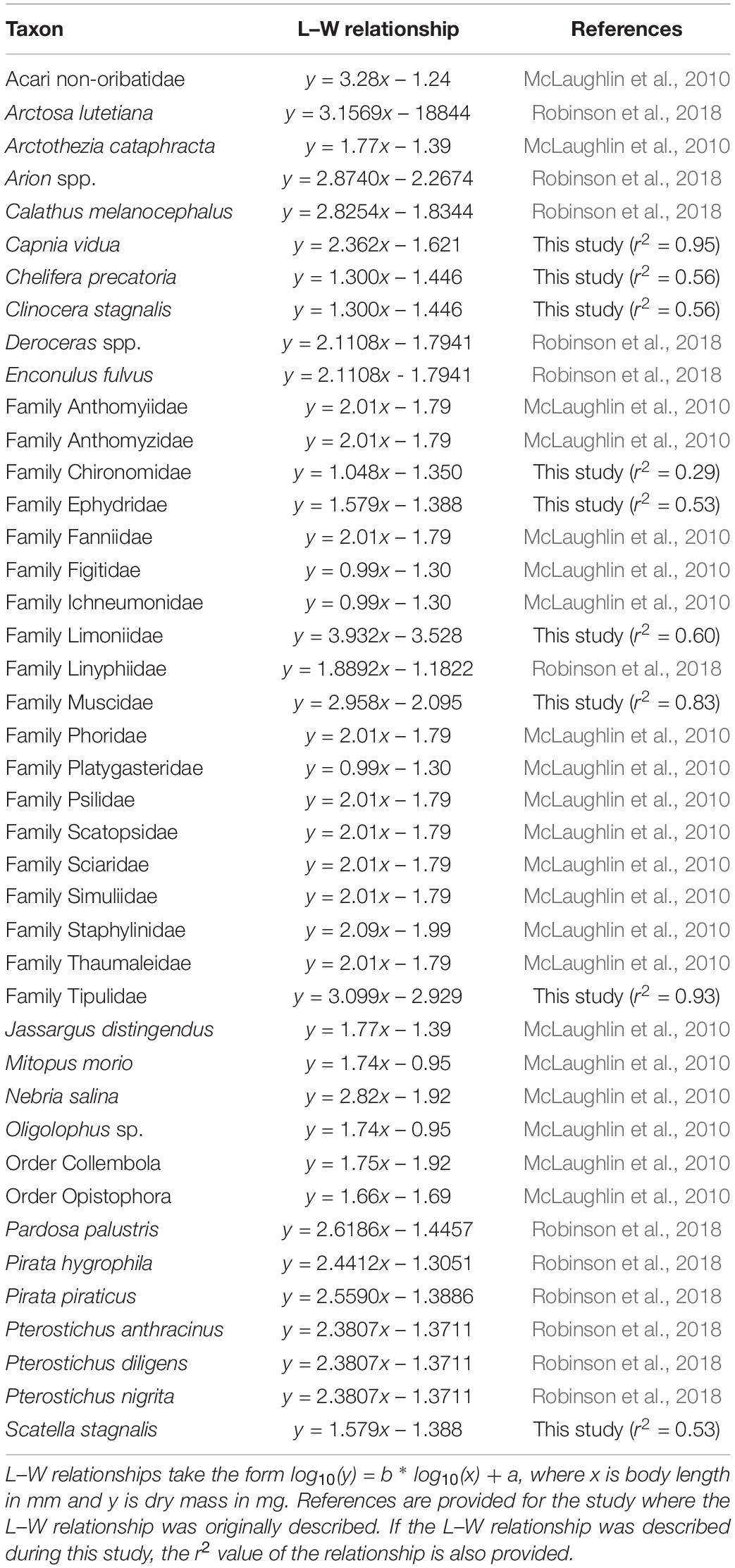
Table 1. Length–weight (L–W) relationships for estimating the body mass of invertebrate taxa sampled in the study.
The individual counts for each taxon were converted to densities (individuals m–2 h–1) by accounting for trap area and the duration of sampling in hours. The densities were summed to give the total community abundance per trap. The mean body mass of each taxon was estimated for each trap and the mean community body mass per trap was calculated as the abundance-weighted mean of all taxa. The biomass of each taxon was calculated as abundance multiplied by the mean body mass for that taxon, and total biomass per trap was the sum of all the biomasses. Shannon diversity, taxon richness, and Pielou’s evenness were also calculated, based on the list of unique taxa found in each trap and their relative abundances.
Statistical Analysis
All analyses were performed in R 4.0.2. There were six response variables in total: biomass, abundance, mean body mass, Shannon diversity, taxon richness, Pielou’s evenness. Mean stream temperature over the study period was considered as the explanatory variable for emergence traps because it alters the life cycles of developing invertebrates in the streams and thus their emergence as adult flies. Mean stream temperature was also considered as the explanatory variable for pan traps because air temperature is not affected by geothermal heating in the Hengill system and so flies landing on the surface of streams are most likely influenced by the water temperature or its effects on resource availability. Mean soil temperature over the study period was considered as the explanatory variable for gutter traps and drift nets because its effects on the activity of ground-dwelling invertebrates is most likely to determine their likelihood of falling into and drifting through the streams.
Two analyses were performed on every response variable: linear and second-order polynomial regression models, with stream or soil temperature as a continuous response variable in each case. When the second-order polynomial term was not significant, the results of the linear regression were presented instead. Normality, homogeneity, and independence of residuals were verified using Q-Q plots and exploration of residuals v fitted values. Biomass, abundance, and mean body mass were log10-transformed to meet the assumptions of the regression models.
Results
Emergence Traps
There was no significant effect of stream temperature on any of the community-level metrics for the aquatic subsidy of freshwater larvae emerging as adult flies into the terrestrial landscape. Specifically, there was no effect of temperature on biomass [F(1, 15) = 0.895, p = 0.359; Figure 3A], abundance [F(1, 15) = 0.863, p = 0.368; Figure 3B], mean body mass [F(1, 13) = 1.107, p = 0.312; Figure 3C], Shannon diversity [F(1, 13) = 2.305, p = 0.153; Figure 3D], taxon richness [F(1, 15) = 0.001, p = 0.970; Figure 3E], or Pielou’s evenness [F(1, 13) = 2.689, p = 0.125; Figure 3F].
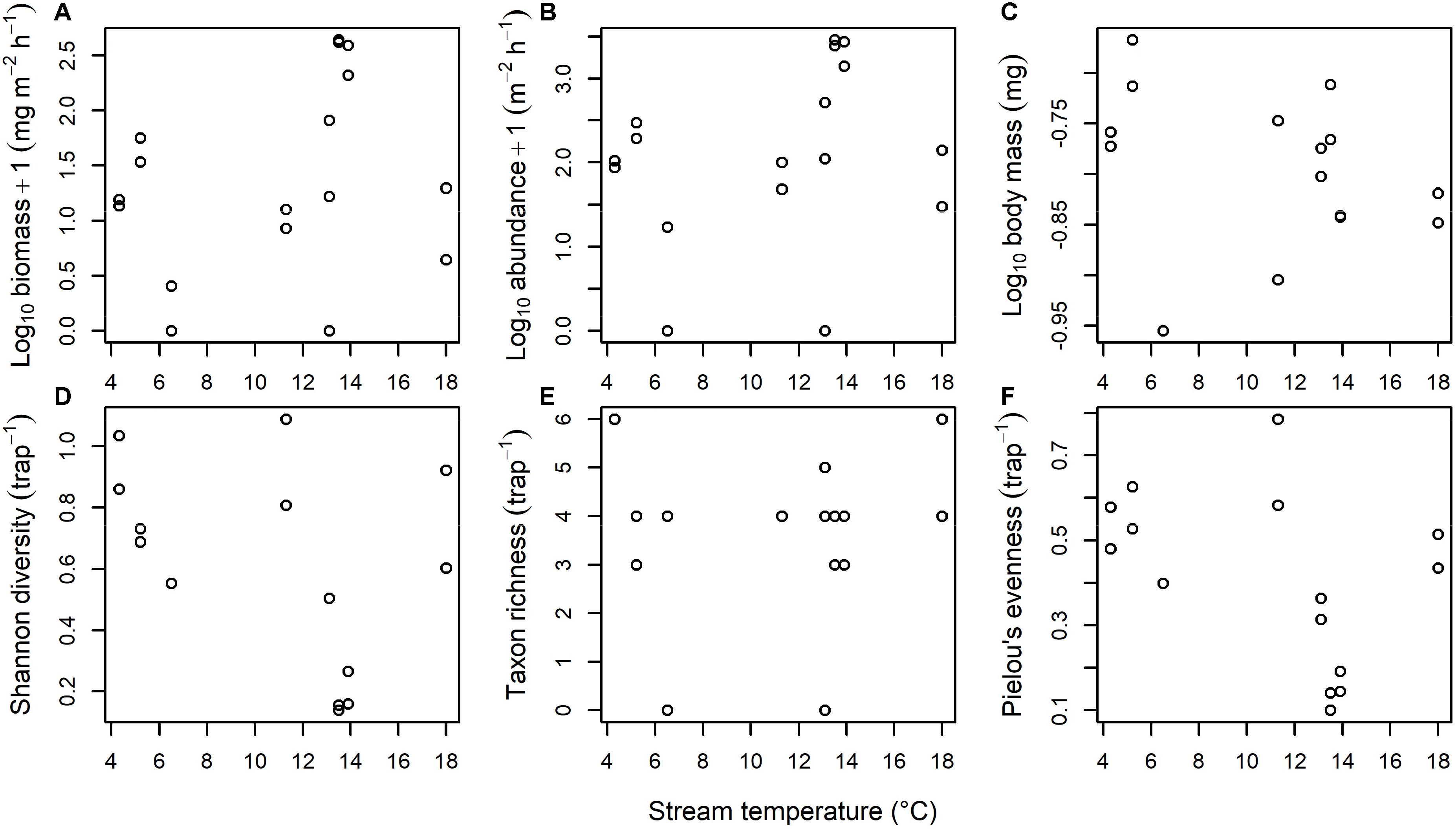
Figure 3. Effect of stream temperature on the freshwater subsidy of aquatic larvae emerging as adult flies into the terrestrial landscape. There was no significant effect of temperature on the (A) Biomass, (B) Abundance, (C) Body mass, (D) Shannon diversity, (E) Taxon richness, or (F) Pielou’s evenness of emerging flies.
Pan Traps
There was a significant increase of ∼210% in the biomass of flies landing on the water surface as stream temperature increased [F(1, 22) = 9.507, p = 0.005; Figure 4A]. This appeared to be driven by a ∼270% increase in the abundance of flies landing on the water surface [F(1, 22) = 7.600, p = 0.011; Figure 4B], with no significant effect of temperature on mean body mass [F(1, 21) = 0.409, p = 0.530; Figure 4C]. There was a significant increase of ∼200% in the Shannon diversity of flies landing on the water surface as stream temperature increased up to 12.5°C, with a subsequent leveling off or slight decline in diversity at higher stream temperatures [F(2, 20) = 10.76, p < 0.001; Figure 4D]. This appeared to be driven by an increase from an average of 1.1 to 3.4 fly taxa landing on the water surface as temperature increased up to 12.5°C [F(2, 21) = 9.423, p = 0.001; Figure 4E], with no significant effect of temperature on Pielou’s evenness [F(1, 18) = 0.095, p = 0.761; Figure 4F.
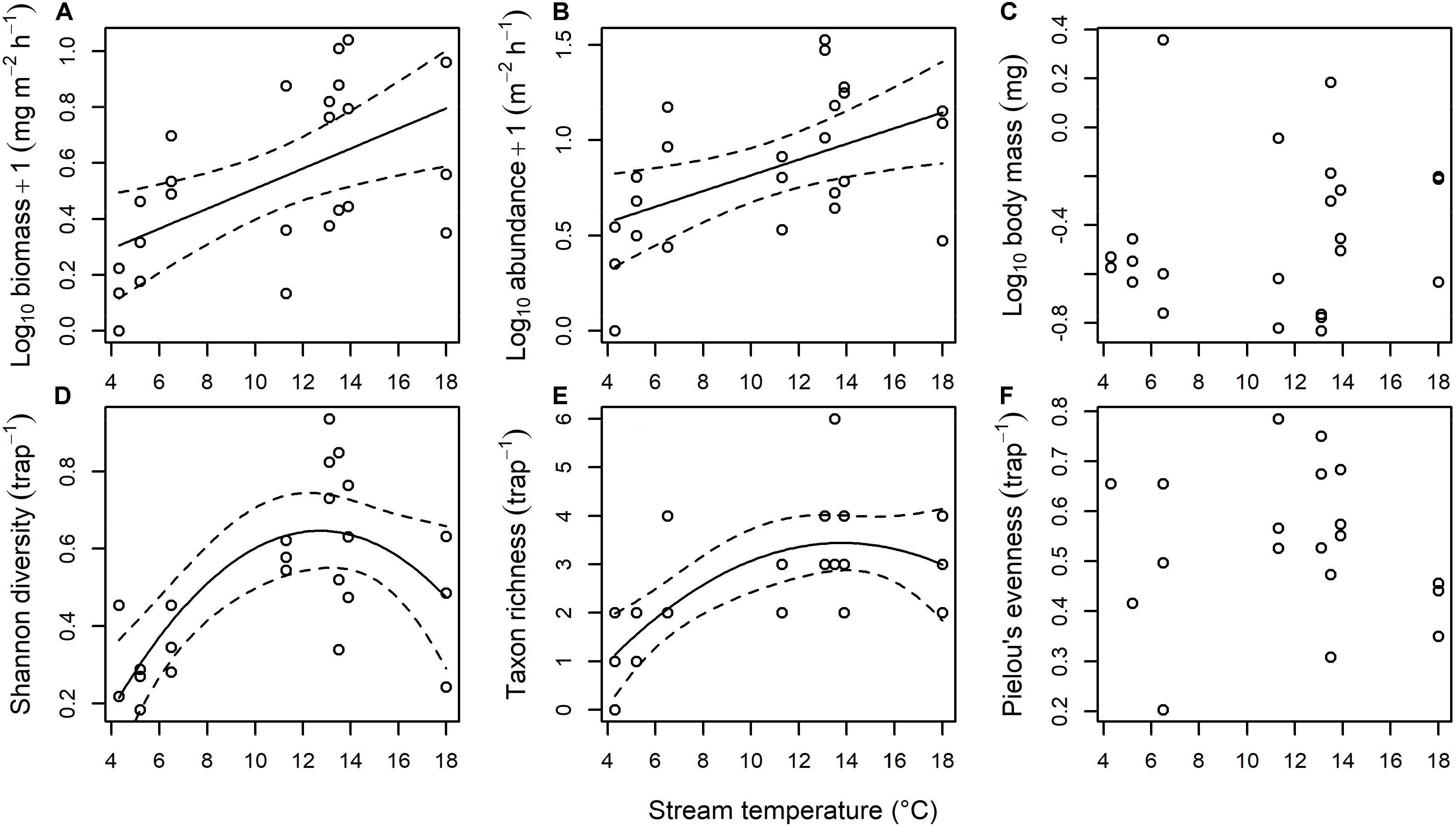
Figure 4. Effect of stream temperature on the terrestrial subsidy of adult flies landing on the surface of the streams. There was a significant effect of temperature on (A) Biomass (y = 0.151 + 0.036x, p = 0.005, r2 = 0.27), (B) abundance (y = 0.404 + 0.041x, p = 0.011, r2 = 0.22), (D) Shannon diversity (y = –0.342 + 0.156x – 0.006x2, p < 0.001, r2 = 0.47), and (E) taxon richness (y = –1.427 + 0.705x – 0.026x2, p = 0.001, r2 = 0.42), with no significant effect of temperature on (C) body mass or (F) Pielou’s evenness.
Gutter Traps
There was a significant increase of ∼465% in the biomass of ground-dwelling invertebrates falling into the streams as soil temperature increased [F(1, 22) = 8.027, p = 0.010; Figure 5A]. This appeared to be driven by an increase by > 2 orders of magnitude in the mean body mass of terrestrial invertebrates falling into the streams [F(1, 21) = 8.414, p = 0.009; Figure 5C], with no significant effect of temperature on abundance (F(1, 21) = 0.369, p = 0.550; Figure 5B]. There was a significant U-shaped response for the Shannon diversity of ground-dwelling invertebrates falling into the streams as soil temperature increased, with the highest diversity in the coldest and warmest soil habitats and the lowest diversity at approximately 11°C [F(2, 20) = 3.521, p = 0.049; Figure 5D]. This appeared to be driven by a similar U-shaped response of Pielou’s evenness to stream temperature [F(2, 20) = 6.630, p = 0.006; Figure 5F], with no significant effect of temperature on taxon richness [F(1, 22) = 2.561, p = 0.124; Figure 5E].
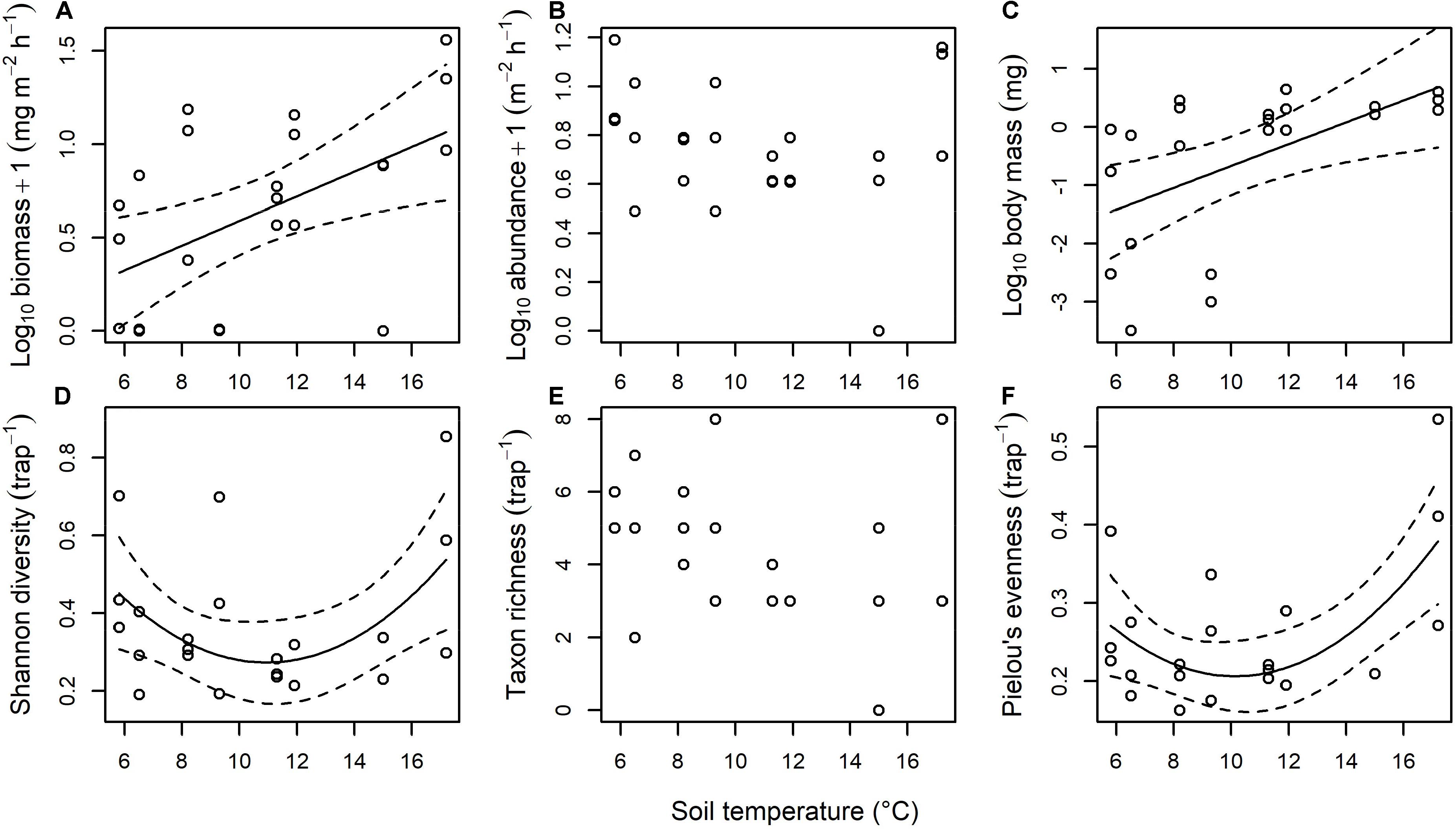
Figure 5. Effect of stream temperature on the terrestrial subsidy of ground-dwelling invertebrates falling into the streams. There was a significant effect of temperature on (A) biomass (y = −0.071 + 0.066x, p = 0.010, r2 = 0.23), (C) body mass (y = −2.543 + 0.187x, p = 0.008, r2 = 0.25), (D) Shannon diversity (y = 1.080 – 0.147x + 0.007x2, p = 0.049, r2 = 0.19), and (F) Pielou’s evenness (y = 0.561 – 0.070x + 0.003x2, p = 0.006, r2 = 0.34), with no significant effect of temperature on (B) abundance or (E) taxon richness.
Drift Nets
There was a significant decrease of ∼135% in the biomass of invertebrates drifting through the streams as soil temperature increased [F(1, 18) = 6.050, p = 0.024; Figure 6A]. This appeared to be driven by an order of magnitude reduction in the abundance of invertebrates drifting through the streams as soil temperature increased [F(1, 18) = 8.820, p = 0.008; Figure 6B], with no significant effect of temperature on mean body mass [F(1, 13) = 0.240, p = 0.633; Figure 6C]. There was a significant decrease of ∼600% in the Shannon diversity of invertebrates drifting through the streams as soil temperature increased [F(1, 13) = 4.897, p = 0.045; Figure 6D]. This appeared to be driven by a decrease from an average of 3.0 to 0.4 invertebrate taxa drifting through the streams as soil temperature increased [F(1, 18) = 5.170, p = 0.035; Figure 6E], with no significant effect of temperature on Pielou’s evenness [F(1, 13) = 2.544, p = 0.135; Figure 6F].
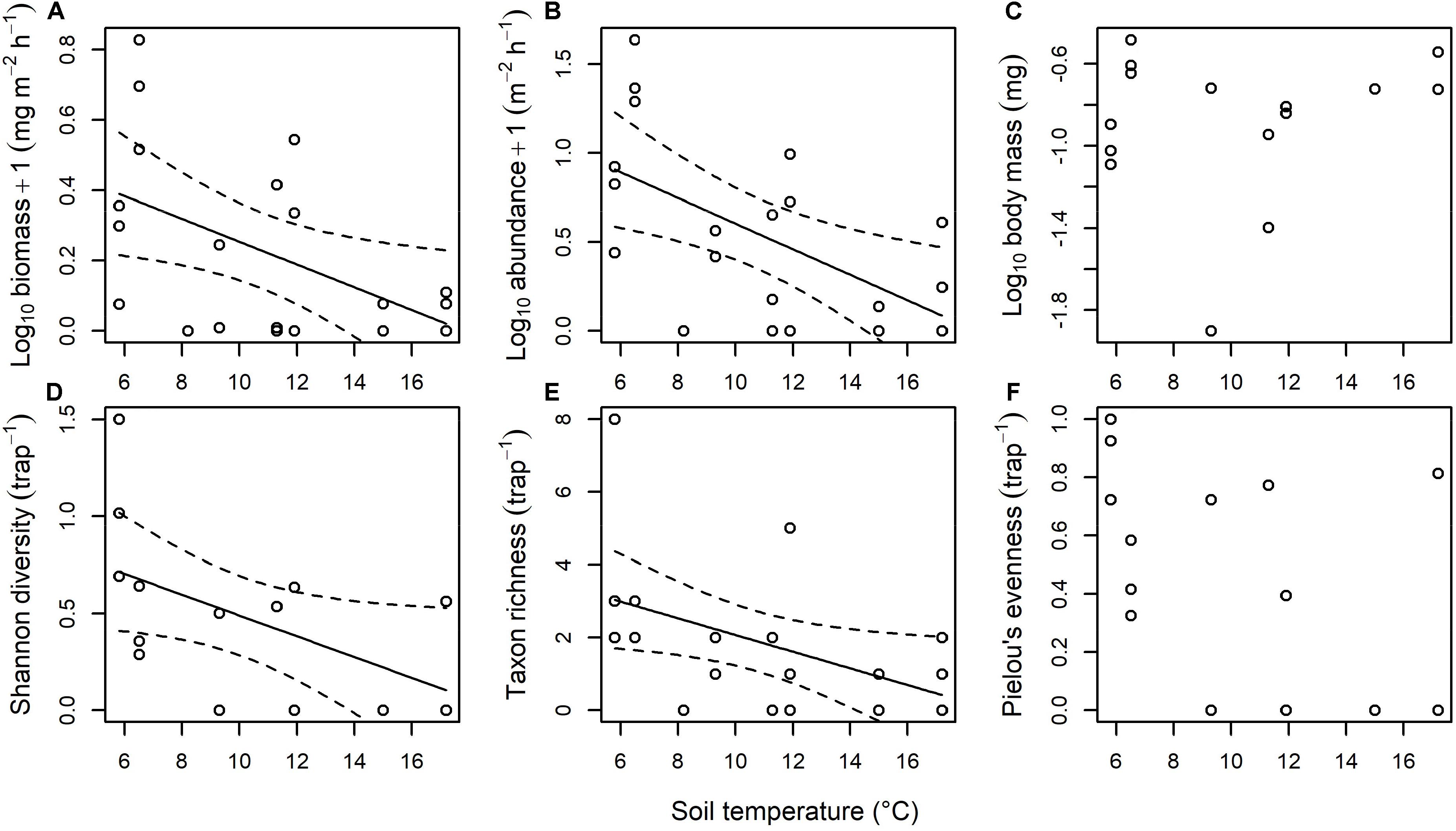
Figure 6. Effect of stream temperature on the terrestrial subsidy of invertebrates drifting through the streams. There was a significant effect of temperature on (A) biomass (y = 0.578 – 0.032x, p = 0.024, r2 = 0.21), (B) abundance (y = 1.325 – 0.072x, p = 0.008, r2 = 0.29), (D) Shannon diversity (y = 1.026 – 0.054x, p = 0.045, r2 = 0.22), and (E) taxon richness (y = 4.366 – 0.230x, p = 0.035, r2 = 0.18), with no significant effect of temperature on (C) body mass or (F) Pielou’s evenness.
Discussion
Reciprocal subsidies between aquatic and terrestrial environments often play a vital role in maintaining the biomass of consumer species (Nakano and Murakami, 2001). Such subsidies are likely to become increasingly important in a warming world (O’Gorman, 2016), as consumers require even more resources to meet their higher metabolic demands (Brown et al., 2004). Here, we show that rising temperatures may lead to asynchronous responses in the reciprocal flow of energy between aquatic and terrestrial ecosystems, with no effect of temperature on the biomass or diversity of aquatic larvae emerging as flies into the terrestrial landscape, but an increase in the biomass and diversity of terrestrial invertebrates entering warmer streams. This could lead to disproportionately larger effects of warming on riparian consumers, who may subsequently decrease in biomass due to their greater energy requirements at higher temperatures. The generality of this asynchronous response to warming across neighboring ecosystems requires further exploration given the likely consequences for consumer species in these interdependent habitats.
Emergence Traps
In contrast to our first hypothesis, there was no significant effect of temperature on the biomass, abundance, or body size of emerging insects (Figures 3A–C). This is in opposition to some previous research on the topic (Greig et al., 2012), and studies showing that the proportion of large, predatory taxa emerging as adults increase with warming in freshwaters (Wesner, 2012; Jonsson et al., 2015), although overall biomass was unchanged in the latter due to an increase in the abundance of small, emerging chironomids. Both the biomass and production of benthic invertebrates increases with temperature in the Hengill streams (O’Gorman et al., 2016, 2017), thus the lack of temperature effects on emergence may indicate that a declining proportion of aquatic resources are being exported to the terrestrial environment as stream temperature increases. This may be due to higher feeding rates on aquatic larval prey by invertebrate predators, as demonstrated in response to their greater metabolic demands in the warmer streams (Archer et al., 2019). The strongest top-down control on aquatic larvae is likely to be exerted by large fish predators in warmer environments though (Epanchin et al., 2010; Wesner, 2010). Previous research in the Hengill system showed that brown trout selectively feed on bigger predatory fly larvae as stream temperature increases (O’Gorman et al., 2016). Thus, trophic structure in the donor ecosystem could play a key role in moderating the impact of warming on subsidies to the recipient ecosystem. A fish exclusion experiment would be needed to definitively assess whether aquatic predators are affecting insect emergence, with a long enough duration to detect the time lag between suppression of larval biomass and the consequences for emerging adult flies (Wesner, 2013).
In contrast to our second hypothesis, there was no significant effect of temperature on the diversity, richness, or evenness of emerging insects (Figures 3D–F). This lack of response could be due to cold-adapted taxa simply being replaced by warm-adapted taxa as stream temperature increases. For example, chironomids are especially abundant in the colder streams in Hengill, but blackfly larvae and snails dominate in the warmer streams, helping to preserve macroinvertebrate species richness across the temperature gradient (Friberg et al., 2009). Nevertheless, the evenness of aquatic invertebrates declines with increasing temperature (Friberg et al., 2009), highlighting the surprising lack of temperature effects on the evenness of emerging insects. Whilst the sampling period coincided with peak emergence, the 1-month duration may simply have been insufficient to capture the full dynamics of insect emergence over the summer period, or the multiple emergence events associated with the warmer streams (Hannesdóttir et al., 2013). The chosen sampling duration was a logistical constraint of access to the field site, but future research should aim to quantify temperature effects on emergence on an annual time-scale. More frequent sampling of emergence traps (e.g., weekly throughout the chosen study period) could also help to capture fine-scale differences in the timing and distribution of peaks in emergent biomass across taxa and study streams.
Pan Traps
In support of our first hypothesis, there was an increase in the biomass of adult flies landing on the surface of streams as temperature increased (Figure 4A). Similar effects were demonstrated from a pan trapping study conducted in August 2012 in the Hengill system, illustrating consistent effects across seasons and years (O’Gorman et al., 2016). This result is interesting given that air temperature is unaffected by geothermal heating in the region and must be indirectly mediated by effects of temperature on the streams. For example, macrophyte cover and in-stream primary and secondary production all increase with temperature in the Hengill system (Hannesdóttir et al., 2013; O’Gorman et al., 2016), which may create greater foraging opportunities for herbivorous, saprophagous, or predatory flies. Indeed, adult Ephydridae and Dolichopodidae (both found in this study) are known to aggregate around warmer waters to feed on cyanobacteria and aquatic larvae, respectively (Brock et al., 1969). Note that we only analyzed flies with a terrestrial, not aquatic, origin in the pan traps, indicating that it was a true terrestrial subsidy, and may thus help to supplement the higher metabolic demands of aquatic organisms in the warmer streams.
In contrast to our second hypothesis, there was a significant increase in the diversity of adult flies landing on the surface of the streams up to 12.5°C (Figure 4D). This could be linked to the increased productivity of the warmer streams (Hannesdóttir et al., 2013; O’Gorman et al., 2016), which could be attracting a broader range of foraging flies due to the increased resource availability (Burdon and Harding, 2008). There was a greater abundance and taxon richness of adult flies landing on the surface of the warmer streams, although the relative dominance of taxa remained unaffected by stream temperature (Figures 4B,E,F). Terrestrial subsidies have been shown to play an important role in the diet of brown trout (Salmo trutta) across the temperature gradient in the Hengill system (O’Gorman et al., 2016). Trout also tend to prefer habitats with a higher diversity of prey taxa, which contributes to faster growth rates for this fish species (Giller and Greenberg, 2015). Thus, a greater diversity of terrestrial subsidies at higher temperatures could boost fish production and may help to explain the surprising success of trout in the warmest streams in the Hengill system (O’Gorman et al., 2016).
Gutter Traps
In support of our first hypothesis, there was an increase in the biomass of ground-dwelling invertebrates falling into the streams with increasing soil temperature (Figure 5A). This pattern was underpinned by a greater mean body mass of invertebrates falling into the streams from warmer soils (Figure 5C), which is in direct contrast to the community-level temperature-size rule (Daufresne et al., 2009). This surprising pattern was driven by increasing prominence at higher temperatures by one of the largest terrestrial arthropods in the system: the wolf spider, Pirata piraticus. This species also begins to dominate the terrestrial habitat as soil temperature increases, leading to a greater mean body mass of ground-dwelling arthropod communities (Robinson et al., 2018, 2021). Pirata piraticus is a free-foraging spider whose diet is often made up of 40% or more aquatic invertebrates (Stenroth et al., 2015). Therefore, they are often found hunting along the stream banks or even on the water surface, which in turn increases the possibility of them becoming a terrestrial subsidy to the aquatic system (Collier et al., 2002). Larger organisms also have relatively higher metabolic demands and thus activity levels with increasing temperature (Brown et al., 2004; Dell et al., 2011; Cloyed et al., 2019), raising their chances of falling from the stream banks or foraging near the stream edge.
In contrast to our second hypothesis, there was a U-shaped relationship between soil temperature and the diversity of ground-dwelling invertebrates falling into the streams (Figure 5D). This indicates that the diversity of terrestrial subsidy to the streams initially decreased with temperature as expected, but the greater activity levels associated with the warmest soils led to a surprising subsequent increase. This was most likely underpinned by the dominant contribution of P. piraticus to the in-fall from the terrestrial system, given that only evenness and not taxon richness of the subsidy was affected by temperature (Figures 5E,F). This highlights the importance of differential thermal responses among the taxa from the regional species pool for community-level responses to warming (Daufresne et al., 2004).
Drift Nets
In direct contrast to our first hypothesis, there was a significant decrease in the biomass of terrestrial invertebrates drifting through the streams as soil temperature increased (Figure 6A). This does suggest, however, that the greater biomass of terrestrial subsidies entering the streams at higher temperatures (Figures 4A, 5A) is being assimilated into the aquatic food web. For example, brown trout become bigger and more abundant as temperature increases in the Hengill system (O’Gorman et al., 2016), likely exerting a much stronger top-down control on resources in the warmer streams (O’Gorman et al., 2012). Terrestrial subsidies have also been shown to play an increasingly important role in the diet of brown trout as they increase in size (Dineen et al., 2007; Sánchez-Hernández and Cobo, 2016). These observed differences in the effects of temperature on the stock of terrestrial subsidy entering the streams and their subsequent flow through the system is analogous to observed patterns for autochthonous resources in the freshwater system. There, the standing stock biomass of algal biofilm decreases with temperature due to stronger consumption by invertebrate grazers in the warmer environment, but this lost biomass is rapidly replenished because primary production increases with stream temperature (O’Gorman et al., 2012). Thus, the impacts of warming on the provision and usage of cross-ecosystem subsidies should always be considered within the context of the recipient ecosystem’s food web.
In support of our second hypothesis, there was a significant decrease in the diversity of drifting invertebrates as soil temperature increased (Figure 6D). This is unlikely to be driven by the hypothesized decline in diversity of the surrounding terrestrial community with increasing temperature, however, given that there was a greater diversity of terrestrial resources entering the warmer streams (Figures 4D, 5D), and soil temperature effects on terrestrial invertebrate diversity have been shown to vary dramatically over the course of the growing season (Robinson et al., 2021). Instead, the decline in diversity may be due to aquatic consumers intercepting a broader range of terrestrial taxa drifting through the warmer streams. Territorially dominant trout have been shown to preferentially feed on a greater diversity of terrestrial prey (Giller and Greenberg, 2015), and the observed decrease in diversity of drifting invertebrates in the warmer streams may simply reflect their maximal usage of the available terrestrial subsidy. Fish exclusion experiments are now needed to tease apart the relative effects of temperature on top-down control and the stocks and flows of subsidies in the aquatic system.
Conclusion
This study contributes to our understanding of how global warming might disrupt the reciprocal subsidies between neighboring ecosystems. Experimental evidence from tropical regions also indicates the potential for asynchronous responses to warming among aquatic-terrestrial linkages (Nash et al., 2021), and further studies are needed to test the robustness of this finding across biogeographical contexts. Future research should focus on experimentally manipulating top-down control, which increases with temperature, and may thus mask the effects of warming on cross-ecosystem linkages through the consumption of subsidies to and from both ecosystems. Longer timescales of research are also needed, given the pulsed nature of subsidies (Sato et al., 2016) and the possibility of negative feedbacks, e.g., even if warming initially increases terrestrial subsidies, subsequent loss of terrestrial consumer biomass due to reduced outputs from aquatic environments may result in long-term reduction of terrestrial inputs to freshwaters. Model microbial systems (e.g., Nash et al., 2021) may offer valuable and logistically tractable insights into these multigenerational responses to warming across ecosystem boundaries.
Data Availability Statement
The raw data supporting the conclusions of this article will be made available by the authors, without undue reservation.
Author Contributions
EO’G secured funding. EO’G, ÓM, and RS designed the research. IC processed the samples. EO’G and IC analyzed the data and wrote the first draft. ÓM and RS commented on the text. All authors conducted the fieldwork.
Funding
We acknowledge funding from NERC (NE/L011840/1), the Royal Society (RG140601), the British Ecological Society (4009-4884, 7283/5350), and Imperial College London’s MSc in Ecology, Evolution and Conservation.
Conflict of Interest
The authors declare that the research was conducted in the absence of any commercial or financial relationships that could be construed as a potential conflict of interest.
Publisher’s Note
All claims expressed in this article are solely those of the authors and do not necessarily represent those of their affiliated organizations, or those of the publisher, the editors and the reviewers. Any product that may be evaluated in this article, or claim that may be made by its manufacturer, is not guaranteed or endorsed by the publisher.
Acknowledgments
We thank Gísli Már Gíslason and Jón S. Ólafsson for providing valuable advice, research support, and laboratory facilities.
References
Archer, L. C., Sohlström, E. H., Gallo, B., Jochum, M., Woodward, G., Kordas, R. L., et al. (2019). Consistent temperature dependence of functional response parameters and their use in predicting population abundance. J. Anim. Ecol. 88, 1670–1683. doi: 10.1111/1365-2656.13060
Bartels, P., Cucherousset, J., Steger, K., Eklöv, P., Tranvik, L. J., and Hillebrand, H. (2012). Reciprocal subsidies between freshwater and terrestrial ecosystems structure consumer resource dynamics. Ecology 93, 1173–1182. doi: 10.1890/11-1210.1
Bauer, S., and Hoye, B. (2014). Migratory animals couple biodiversity and ecosystem functioning worldwide. Science 344:1242552. doi: 10.1126/science.1242552
Baxter, C. V., Fausch, K. D., and Carl Saunders, W. (2005). Tangled webs: reciprocal flows of invertebrate prey link streams and riparian zones. Freshw. Biol. 50, 201–220.
Brock, M. L., Wiegert, R. G., and Brock, T. D. (1969). Feeding by Paracoenia and Ephydra (Diptera> Ephydridae) on the microorganisms of hot springs. Ecology 50, 192–200.
Brown, J. H., Gillooly, J. F., Allen, A. P., Savage, V. M., and West, G. B. (2004). Toward a metabolic theory of ecology. Ecology 85, 1771–1789.
Buchanan, G. M., Grant, M. C., Sanderson, R. A., and Pearce-Higgins, J. W. (2006). The contribution of invertebrate taxa to moorland bird diets and the potential implications of land-use management. IBIS 148, 615–628.
Burdon, F. J., and Harding, J. S. (2008). The linkage between riparian predators and aquatic insects across a stream-resource spectrum. Freshw. Biol. 53, 330–346.
Chen, I.-C., Hill, J. K., Ohlemüller, R., Roy, D. B., and Thomas, C. D. (2011). Rapid range shifts of species associated with high levels of climate warming. Science 333, 1024–1026. doi: 10.1126/science.1206432
Cloyed, C. S., Dell, A. I., Hayes, T., Kordas, R. L., and O’Gorman, E. J. (2019). Long-term exposure to higher temperature increases the thermal sensitivity of grazer metabolism and movement. J. Anim. Ecol. 88, 833–844. doi: 10.1111/1365-2656.12976
Collier, K. J., Bury, S., and Gibbs, M. (2002). A stable isotope study of linkages between stream and terrestrial food webs through spider predation. Freshw. Biol. 47, 1651–1659.
Currie, D. J., Mittelbach, G. G., Cornell, H. V., Field, R., Guégan, J. F., Hawkins, B. A., et al. (2004). Predictions and tests of climate-based hypotheses of broad-scale variation in taxonomic richness. Ecol. Lett. 7, 1121–1134.
Daufresne, M., Lengfellner, K., and Sommer, U. (2009). Global warming benefits the small in aquatic ecosystems. Proc. Natl. Acad. Sci. U.S.A. 106, 12788–12793.
Daufresne, M., Roger, M., Capra, H., and Lamouroux, N. (2004). Long-term changes within the invertebrate and fish communities of the Upper Rhône River: effects of climatic factors. Glob. Change Biol. 10, 124–140.
Dell, A. I., Pawar, S., and Savage, V. M. (2011). Systematic variation in the temperature dependence of physiological and ecological traits. Proc. Natl. Acad. Sci. U.S.A. 108, 10591–10596.
Dineen, G., Harrison, S., and Giller, P. (2007). Diet partitioning in sympatric Atlantic salmon and brown trout in streams with contrasting riparian vegetation. J. Fish Biol. 71, 17–38.
Eitzinger, B., Roslin, T., Vesterinen, E., Robinson, S. I., and O’Gorman, E. J. (2021). Temperature affects both the Grinnellian and Eltonian dimensions of ecological niches–a tale of two Arctic wolf spiders. Basic Appl. Ecol. 50, 132–143.
Epanchin, P. N., Knapp, R. A., and Lawler, S. P. (2010). Nonnative trout impact an alpine-nesting bird by altering aquatic-insect subsidies. Ecology 91, 2406–2415. doi: 10.1890/09-1974.1
Forster, J., Hirst, A. G., and Atkinson, D. (2012). Warming-induced reductions in body size are greater in aquatic than terrestrial species. Proc. Natl. Acad. Sci. U.S.A. 109, 19310–19314. doi: 10.1073/pnas.1210460109
Friberg, N., Dybkjaer, J. B., Ólafsson, J. S., Gíslason, G. M., Larsen, S. E., and Lauridsen, T. L. (2009). Relationships between structure and function in streams contrasting in temperature. Freshw. Biol. 54, 2051–2068. doi: 10.1111/j.1365-2427.2009.02234.x
Gardner, J. L., Peters, A., Kearney, M. R., Joseph, L., and Heinsohn, R. (2011). Declining body size: a third universal response to warming? Trends Ecol. Evol. 26, 285–291. doi: 10.1016/j.tree.2011.03.005
Giller, P., and Greenberg, L. (2015). The relationship between individual habitat use and diet in brown trout. Freshw. Biol. 60, 256–266. doi: 10.1111/fwb.12472
Gratton, C., Donaldson, J., and Vander Zanden, M. J. (2008). Ecosystem linkages between lakes and the surrounding terrestrial landscape in northeast Iceland. Ecosystems 11, 764–774. doi: 10.1007/s10021-008-9158-8
Greig, H. S., Kratina, P., Thompson, P. L., Palen, W. J., Richardson, J. S., and Shurin, J. B. (2012). Warming, eutrophication, and predator loss amplify subsidies between aquatic and terrestrial ecosystems. Glob. Change Biol. 18, 504–514. doi: 10.1111/j.1365-2486.2011.02540.x
Häder, D.-P., and Barnes, P. W. (2019). Comparing the impacts of climate change on the responses and linkages between terrestrial and aquatic ecosystems. Sci. Total Environ. 682, 239–246. doi: 10.1016/j.scitotenv.2019.05.024
Hannesdóttir, E. R., Gíslason, G. M., Ólafsson, J. S., Ólafsson, ÓP., and O’Gorman, E. J. (2013). Increased stream productivity with warming supports higher trophic levels. Adv. Ecol. Res. 48, 283–340.
Harper, M. P., and Peckarsky, B. L. (2006). Emergence cues of a mayfly in a high-altitude stream ecosystem: potential response to climate change. Ecol. Appl. 16, 612–621. doi: 10.1890/1051-0761(2006)016[0612:ecoami]2.0.co;2
Henschel, J. R., Mahsberg, D., and Stumpf, H. (2001). Allochthonous aquatic insects increase predation and decrease herbivory in river shore food webs. Oikos 93, 429–438. doi: 10.1034/j.1600-0706.2001.930308.x
Hering, D., and Plachter, H. (1997). Riparian ground beetles (Coeloptera, Carabidae) preying on aquatic invertebrates: a feeding strategy in alpine floodplains. Oecologia 111, 261–270. doi: 10.1007/s004420050234
Hickling, R., Roy, D. B., Hill, J. K., Fox, R., and Thomas, C. D. (2006). The distributions of a wide range of taxonomic groups are expanding polewards. Glob. Change Biol. 12, 450–455. doi: 10.1111/j.1365-2486.2006.01116.x
Hoekman, D., Dreyer, J., Jackson, R. D., Townsend, P. A., and Gratton, C. (2011). Lake to land subsidies: experimental addition of aquatic insects increases terrestrial arthropod densities. Ecology 92, 2063–2072. doi: 10.1890/11-0160.1
Hogg, I. D., and Williams, D. D. (1996). Response of stream invertebrates to a global-warming thermal regime: an ecosystem-level manipulation. Ecology 77, 395–407. doi: 10.2307/2265617
IPBES (2019). Summary For Policymakers of The Global Assessment Report on Biodiversity And Ecosystem Services. Bonn: Intergovernmental Science-Policy Platform on Biodiversity and Ecosystem Services.
IPCC (2021). Climate Change 2021: The Physical Science Basis. Contribution of Working Group I to the Sixth Assessment Report of the Intergovernmental Panel on Climate Change, eds V. Masson-Delmotte, P. Zhai, A. Pirani, S. L. Connors, C. Péan, S. Berger, et al. (Cambridge: Cambridge University Press).
Johnson, C. N., Balmford, A., Brook, B. W., Buettel, J. C., Galetti, M., Guangchun, L., et al. (2017). Biodiversity losses and conservation responses in the Anthropocene. Science 356, 270–275. doi: 10.1126/science.aam9317
Jonsson, M., Hedström, P., Stenroth, K., Hotchkiss, E. R., Vasconcelos, F. R., Karlsson, J., et al. (2015). Climate change modifies the size structure of assemblages of emerging aquatic insects. Freshw. Biol. 60, 78–88. doi: 10.1111/fwb.12468
Kato, C., Iwata, T., and Wada, E. (2004). Prey use by web-building spiders: stable isotope analyses of trophic flow at a forest-stream ecotone. Ecol. Res. 19, 633–643. doi: 10.1111/j.1440-1703.2004.00678.x
Knight, T. M., McCoy, M. W., Chase, J. M., McCoy, K. A., and Holt, R. D. (2005). Trophic cascades across ecosystems. Nature 437, 880–883.
Larsen, S., Muehlbauer, J. D., and Marti, E. (2016). Resource subsidies between stream and terrestrial ecosystems under global change. Glob. Change Biol. 22, 2489–2504. doi: 10.1111/gcb.13182
Leroux, S. J., and Loreau, M. (2012). Dynamics of reciprocal pulsed subsidies in local and meta-ecosystems. Ecosystems 15, 48–59. doi: 10.1007/s10021-011-9492-0
Lurgi, M., López, B. C., and Montoya, J. M. (2012). Novel communities from climate change. Philos. Trans. R. Soc. B 367, 2913–2922.
Marczak, L. B., and Richardson, J. S. (2008). Growth and development rates in a riparian spider are altered by asynchrony between the timing and amount of a resource subsidy. Oecologia 156, 249–258. doi: 10.1007/s00442-008-0989-y
McCauley, S. J., Hammond, J. I., and Mabry, K. E. (2018). Simulated climate change increases larval mortality, alters phenology, and affects flight morphology of a dragonfly. Ecosphere 9:e02151. doi: 10.1002/ecs2.2151
McLaughlin, O. B., Jonsson, T. and Emmerson, M. C. (2010). Temporal variability in predator−prey relationships of a forest floor food web. Adv. Ecol. Res., 42, 171–264. doi: 10.1016/B978-0-12-381363-3.00004-6
Menzel, A., Sparks, T. H., Estrella, N., Koch, E., Aasa, A., Ahas, R., et al. (2006). European phenological response to climate change matches the warming pattern. Glob. Change Biol. 12, 1969–1976. doi: 10.1111/gcb.15000
Nakano, S., and Murakami, M. (2001). Reciprocal subsidies: dynamic interdependence between terrestrial and aquatic food webs. Proc. Natl. Acad. Sci. U.S.A. 98, 166–170. doi: 10.1073/pnas.98.1.166
Nakano, S., Miyasaka, H., and Kuhara, N. (1999). Terrestrial-aquatic linkages: riparian arthropod inputs alter trophic cascades in a stream food web. Ecology 80, 2435–2441.
Nash, L. N., Antiqueira, P. A., Romero, G. Q., de Omena, P. M., and Kratina, P. (2021). Warming of aquatic ecosystems disrupts aquatic–terrestrial linkages in the tropics. J. Anim. Ecol. 90, 1623–1634. doi: 10.1111/1365-2656.13505
O’Gorman, E. J., Petchey, O. L., Faulkner, K. J., Gallo, B., Gordon, T. A., Neto-Cerejeira, J., et al. (2019). A simple model predicts how warming simplifies wild food webs. Nat. Clim. Change 9, 611–616.
O’Gorman, E. J., Pichler, D. E., Adams, G., Benstead, J. P., Cohen, H., Craig, N., et al. (2012). Impacts of warming on the structure and functioning of aquatic communities: individual- to ecosystem-level responses. Adv. Ecol. Res. 47, 81–176.
O’Gorman, E. J. (2016). It’s only a matter of time: the altered role of subsidies in a warming world. J. Anim. Ecol. 85, 1133–1135. doi: 10.1111/1365-2656.12560
O’Gorman, E. J., Benstead, J. P., Cross, W. F., Friberg, N., Hood, J. M., Johnson, P. W., et al. (2014). Climate change and geothermal ecosystems: natural laboratories, sentinel systems, and future refugia. Glob. Change Biol. 20, 3291–3299. doi: 10.1111/gcb.12602
O’Gorman, E. J., Ólafsson, ÓP., Demars, B. O. L., Friberg, N., Guðbergsson, G., Hannesdóttir, E. R., et al. (2016). Temperature effects on fish production across a natural thermal gradient. Glob. Change Biol. 22, 3206–3220. doi: 10.1111/gcb.13233
O’Gorman, E. J., Zhao, L., Pichler, D. E., Adams, G., Friberg, N., Rall, B. C., et al. (2017). Unexpected changes in community size structure in a natural warming experiment. Nat. Clim. Change 7, 659–666.
Ottesen, P. S. (1985). Diel activity patterns of South Scandinavian high mountain ground beetles (Coleoptera, Carabidae). Ecography 8, 191–203.
Paetzold, A., Schubert, C. J., and Tockner, K. (2005). Aquatic terrestrial linkages along a braided-river: riparian arthropods feeding on aquatic insects. Ecosystems 8, 748–759.
Pilotto, F., Kühn, I., Adrian, R., Alber, R., Alignier, A., Andrews, C., et al. (2020). Meta-analysis of multidecadal biodiversity trends in Europe. Nat. Commun. 11, 1–11. doi: 10.1038/s41467-020-17171-y
Robinson, S. I., McLaughlin, Ó. B., Marteinsdóttir, B., and O’Gorman, E. J. (2018). Soil temperature effects on the structure and diversity of plant and invertebrate communities in a natural warming experiment. J. Anim. Ecol. 87, 634–646. doi: 10.1111/1365-2656.12798
Robinson, S. I., Mikola, J., Ovaskainen, O., and O’Gorman, E. J. (2021). Temperature effects on the temporal dynamics of a subarctic invertebrate community. J. Anim. Ecol. 90, 1217–1227.
Rueger, T., and Sommer, U. (2012). Warming does not always benefit the small – results from a plankton experiment. Aquat. Bot. 97, 64–68.
Sánchez-Hernández, J., and Cobo, F. (2016). Ontogenetic shifts in terrestrial reliance of stream-dwelling brown trout. J. Limnol. 14:75.
Sardina, P., Beardall, J., Beringer, J., Grace, M., and Thompson, R. M. (2017). Consequences of altered temperature regimes for emerging freshwater invertebrates. Aquat. Sci. 79, 265–276. doi: 10.1890/1051-0761(2006)016[1608:ceisrf]2.0.co;2
Sato, T., El-Sabaawi, R. W., Campbell, K., Ohta, T., and Richardson, J. S. (2016). A test of the effects of timing of a pulsed resource subsidy on stream ecosystems. J. Anim. Ecol. 85, 1136–1146.
Soininen, J., Bartels, P., Heino, J., Luoto, M., and Hillebrand, H. (2015). Toward more integrated ecosystem research in aquatic and terrestrial environments. BioScience 65:biu216.
Spiller, D. A., Piovia-Scott, J., Wright, A. N., Yang, L. H., Takimoto, G., Schoener, T. W., et al. (2010). Marine subsidies have multiple effects on coastal food webs. Ecology 91, 1424–1434. doi: 10.1890/09-0715.1
Stenroth, K., Polvi, L. E., Fältström, E., and Jonsson, M. (2015). Land-use effects on terrestrial consumers through changed size structure of aquatic insects. Freshw. Biol. 60, 136–149. doi: 10.1007/s00442-015-3462-8
Takimoto, G., Iwata, T., and Murakami, M. (2002). Seasonal subsidy stabilizes food web dynamics: balance in a heterogeneous landscape. Ecol. Res. 17, 433–439.
Trisos, C. H., Merow, C., and Pigot, A. L. (2020). The projected timing of abrupt ecological disruption from climate change. Nature 580, 496–501. doi: 10.1038/s41586-020-2189-9
Urban, M. C. (2015). Accelerating extinction risk from climate change. Science 348, 571–573. doi: 10.1126/science.aaa4984
Warner, E., Marteinsdóttir, B., Helmutsdóttir, V. F., Ehrlén, J., Robinson, S. I., and O’Gorman, E. J. (2021). Impacts of soil temperature, phenology, and plant community composition on invertebrate herbivory in a natural warming experiment. Oikos 130, 1572–1582. doi: 10.1111/oik.08046
Warren, R., Price, J., Graham, E., Forstenhaeusler, N., and VanDerWal, J. (2018). The projected effect on insects, vertebrates, and plants of limiting global warming to 1.5 C rather than 2 C. Science 360, 791–795. doi: 10.1126/science.aar3646
Wesner, J. S. (2010). Aquatic predation alters a terrestrial prey subsidy. Ecology 91, 1435–1444. doi: 10.1890/09-1532.1
Wesner, J. S. (2012). Emerging aquatic insects as predators in terrestrial systems across a gradient of stream temperature in North and South America. Freshw. Biol. 57, 2465–2474. doi: 10.1111/fwb.12013
Wesner, J. S. (2013). Fish predation alters benthic, but not emerging, insects across whole pools of an intermittent stream. Freshw. Sci. 32, 438–449. doi: 10.1899/12-124.1
Woodward, G., Dybkjaer, J. B., Ólafsson, J. S., Gíslason, G. M., Hannesdóttir, E. R., and Friberg, N. (2010). Sentinel systems on the razor’s edge: effects of warming on Arctic geothermal stream ecosystems. Glob. Change Biol. 16, 1979–1991. doi: 10.1111/j.1365-2486.2009.02052.x
Keywords: emergence, allochthonous, geothermal streams, climate change, decoupling, subarctic, drift
Citation: O’Gorman EJ, Chemshirova I, McLaughlin ÓB and Stewart RIA (2021) Impacts of Warming on Reciprocal Subsidies Between Aquatic and Terrestrial Ecosystems. Front. Ecol. Evol. 9:795603. doi: 10.3389/fevo.2021.795603
Received: 15 October 2021; Accepted: 12 November 2021;
Published: 02 December 2021.
Edited by:
Jeff Wesner, University of South Dakota, United StatesReviewed by:
Micael Jonsson, Umeå University, SwedenJustin Pomeranz, Colorado Mesa University, United States
Copyright © 2021 O’Gorman, Chemshirova, McLaughlin and Stewart. This is an open-access article distributed under the terms of the Creative Commons Attribution License (CC BY). The use, distribution or reproduction in other forums is permitted, provided the original author(s) and the copyright owner(s) are credited and that the original publication in this journal is cited, in accordance with accepted academic practice. No use, distribution or reproduction is permitted which does not comply with these terms.
*Correspondence: Eoin J. O’Gorman, ZS5vZ29ybWFuQGVzc2V4LmFjLnVr