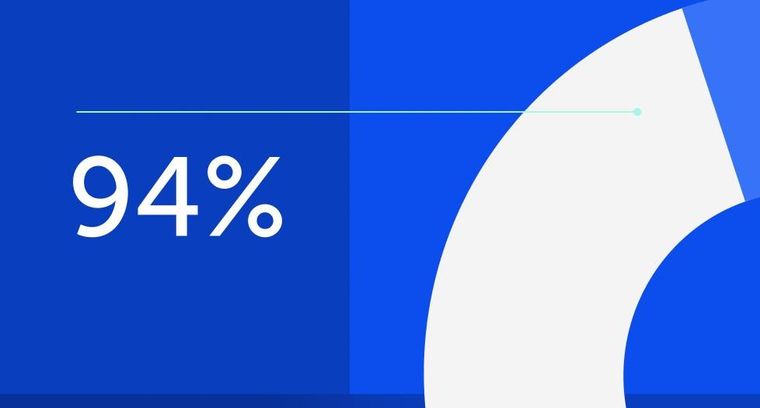
94% of researchers rate our articles as excellent or good
Learn more about the work of our research integrity team to safeguard the quality of each article we publish.
Find out more
ORIGINAL RESEARCH article
Front. Ecol. Evol., 23 December 2021
Sec. Evolutionary Developmental Biology
Volume 9 - 2021 | https://doi.org/10.3389/fevo.2021.786037
This article is part of the Research TopicEmerging Research Organisms in Regenerative BiologyView all 10 articles
The anthozoan sea anemone Nematostella vectensis belongs to the phylum of cnidarians which also includes jellyfish and corals. Nematostella are native to United States East Coast marsh lands, where they constantly adapt to changes in salinity, temperature, oxygen concentration and pH. Its natural ability to continually acclimate to changing environments coupled with its genetic tractability render Nematostella a powerful model organism in which to study the effects of common pollutants on the natural development of these animals. Potassium nitrate, commonly used in fertilizers, and Phthalates, a component of plastics are frequent environmental stressors found in coastal and marsh waters. Here we present data showing how early exposure to these pollutants lead to dramatic defects in development of the embryos and eventual mortality possibly due to defects in feeding ability. Additionally, we examined the microbiome of the animals and identified shifts in the microbial community that correlated with the type of water that was used to grow the animals, and with their exposure to pollutants.
Nematostella vectensis is a sea anemone that belongs to the class Anthozoa in the phylum Cnidaria. This species inhabits marsh habitats on the East Coast of the United States, where they constantly adapt to changes in salinity, temperature, oxygen concentration, and pH (Darling et al., 2005; Reitzel et al., 2013; Elran et al., 2014; Tarrant et al., 2018). Studies of embryonic development in Nematostella have provided new insights into how tissue layers differentiate in diploblastic animals (Wikramanayake et al., 2003; Kraus and Technau, 2006; Röttinger et al., 2012; Schwaiger et al., 2014; Amiel et al., 2017; Steinmetz et al., 2017; Wijesena et al., 2017; Kirillova et al., 2018; Technau, 2020). Like most cnidarians, Nematostella have unique specialized cells called cnidocytes which facilitate capture of prey and serve as inherent defense mechanism (Marlow et al., 2012; Babonis and Martindale, 2014; Babonis et al., 2016; Sebe-Pedros et al., 2018). The ease of culturing in laboratory conditions combined with genetic tractability render Nematostella a valuable system for investigating the evolution and molecular mechanisms of specialized cell types. More recently Nematostella has attracted attention in regenerative biology because of to their genetic tractability, rapid regeneration time and ability to easily compare development and regeneration (Trevino et al., 2011; Passamaneck and Martindale, 2012; Bossert et al., 2013; DuBuc et al., 2014; Amiel et al., 2015; Layden et al., 2016; Schaffer et al., 2016; Bossert and Thomsen, 2017; Warner et al., 2018; Amiel et al., 2021; Amiel and Röttinger, 2021; van der Burg and Prentis, 2021).
Many studies on cnidarians have shown that their genetic complexity and microbiome diversity rivals that of humans despite their having diverged from a common metazoan ancestor more than 1 billion years ago (Daniel et al., 1999; Darling et al., 2005; Fraune and Bosch, 2010; Fraune et al., 2010; Essock-Burns et al., 2020). The microbiome of several species of Nematostella from diverse geographical location has been mapped and it has been clearly shown that there is a distinct correlation between differences in the biogeography and microbiome (Mortzfeld et al., 2016). Several studies have shown the mutualistic association between host and microbes that lead to optimal fitness of the host (Thompson et al., 2014; Heath-Heckman et al., 2016; Rook et al., 2017; Essock-Burns et al., 2020; Bosch and McFall-Ngai, 2021). Species which live in coastal areas, especially marshes, exhibit residual plasticity in their physiology in response to continuous exposure to changing temperature and salinity. Agricultural and industrial pollutants have profound effects on marine ecosystems, however, our limited ability to make accurate predictions about the response, stability and resilience of the affected ecosystems and their inhabitants reflects our general lack of understanding of the complex interplay between genetic and environmental factors that influence acclimation and adaptation to environmental stressors.
This study focused on the environmental contaminants phthalates, specifically phthalic acid esters (PAEs) and nitrate, because they are common pollutants of salt marsh ecosystems in developed areas. PAEs are used in plasticizers and are found in a variety of plastic products, which can subsequently leach PAEs into the environment from landfills and sewage (Hu et al., 2021). Nitrate is a common pollutant in coastal ecosystems derived from agriculture and wastewater (McClelland and Valiela, 1998). We used both pollutants at concentrations between 1–20 μM, a range that is realistic for both compounds reflecting concentrations found in coastal and estuarine ecosystems (Gugliandolo et al., 2020; Valiela et al., 2021). Previous studies have demonstrated the detrimental effects of phthalates on the growth and development of a variety of vertebrates and invertebrates, including zebrafish, humans, and Daphnia sp. (Philippat et al., 2012; Kinch et al., 2016; Jergensen et al., 2019; Qian et al., 2020). Although this pollutant is prevalent in the Nematostella vectensis habitat, little is known about its effects on cnidarian growth and development. Similarly, elevated concentrations of nitrate are known to be toxic to many fish and invertebrates and are predicted to have similar detrimental effects on Nematostella vectensis development (Camargo et al., 2005).
Investigations that focus on altered gene expression patterns have commonly described adaptation to environmental shifts. Dysbiosis of an organism’s microbiome can also substantially influence the phenotype of an organism. The microbiome plays an important role in different aspects of an organism’s life cycle ranging from embryological development to nutrition, immune response, and development of disease (Zheng et al., 2020). Changes in the microbiome can correlate with numerous short-, mid- and long-term changes in the host, some of which promote adaption to new environmental conditions (Pita et al., 2018). Until recently, gene expression, epigenetics and microbiomes have all been studied separately and little is known about their interactions in terms of marine organisms and environmental pollution. In this study, we take advantage of the amenability of sea anemone Nematostella vectensis to culturing in the lab and use this organism to study more closely the effect of early exposure to environmental pollutants on its embryonic development and associated microbiome. We hypothesized that exposure to elevated levels of phthalates and nitrate would lead to increased relative abundances of taxa capable of metabolizing these environmental stressors.
We maintained adult Nematostella vectensis at 17 to 20°C in a flow through aquatic system or in Pyrex glass bowls kept in the dark in 15 parts per thousand (ppt) instant ocean, referred to here as “Nematostella water.” We fed adult animals 48-h old artemia, three times a week. The water quality of the system was monitored weekly. Animals kept in bowls were cleaned a few hours after feeding. Spawning of animals was induced by exposure to light and increase in temperature to 23–25°C. We collected embryos immediately after spawning, usually around 12 h after light exposure.
Freshly laid embryos were transferred to multi-well dishes. Embryos were incubated in Nematostella water containing 1, 10, or 20 μM of potassium nitrate (KNO3) (Sigma P8291) or containing 1, 10, or 20 μM of dioctyl phthalate (Sigma D201154). Control animals were incubated in multi-well plates in Nematostella water at 17–20°C. Solutions on all animals were changed every 3 days. We fed the developing animals’ rotifers or 24-hour old artemia, starting at the four-tentacle stage. In experiments testing the seawater of the local Sippewissett Salt Marsh, we diluted the seawater to 15 ppt with deionized water to obtain the same salinity as the standard Nematostella water the animals are usually maintained in. The environmental stressors were added directly into the diluted seawater and control embryos were incubated in the diluted 15 ppt Sippewissett seawater.
When animals reached the four tentacle stage they were relaxed in 7.4% v/v MgCl2 and then fixed in 4% paraformaldehyde (PFA) overnight at 4°C. Animals were imaged on a Zeiss Discovery V8 Stereo microscope. From these images, tentacle number and pharynx length were quantified using the measure function in Fiji and analyzed using Prism GraphPad.
Regeneration experiments were performed on adult animals at least 12 months of age. Animals were relaxed in 7.4% v/v MgCl2 for about 15 min. Individual animals were transferred to a 60 mm × 15 mm plastic petri dish (Fisher) using a glass pipette. Using a sterile no. 10 disposable scalpel (World Precision Instruments) animals were amputated at the bottom of the pharynx. The animals were then isolated into separate wells of 12 well cell culture plates. The treatments used were 15 ppt instant ocean water; 1 μM KNO3, 10 μM KNO3, 20 μM KNO3; 1, 10, and 20 μM dioctyl phthalate. In all experiments, solutions were changed every 3 days. The animals were allowed to regenerate for 14 days then relaxed in 7.4% MgCl2 and fixed in 4% PFA overnight and stored at 4°C. Animals were imaged on a Zeiss Discovery V8 Stereo microscope.
Cnidocyte staining was carried out according to Wolenski et al. (2013). Animals were relaxed in 7.4% v/v MgCl2 and then fixed overnight in 4% PFA plus 10 mM EDTA and washed 3 × 5 min in Tris-EDTA wash buffer (10 mM Tris, 10 mM EDTA, 10 mM NaCl, pH 7.6). The animals were incubated in 200 μg/ml DAPI diluted in Tris-EDTA buffer for 30 min at room temperature followed by rinsing 3 × 5 min in Tris-EDTA buffer. Animals were imaged on a Leica DMI6000B inverted microscope.
For the microbiome analyses we used animals from cultures investigating the effects of pollutants on embryonic growth and development. We used embryos spawned from mixed populations of animals for each biological replicate, embryos were collected 10 days post fertilization for DNA extraction. We flash froze each cohort of about 200 animals per condition prior to extraction, at 10 days post fertilization the control animals had reached the 4 tentacle stage. Three replicas of each condition were separately frozen, each replica was a different well. Brief centrifugation at 6000 rpm pelleted the suspended Nematostella sp. embryos before transfer of 50 μl solutions to bead beating tubes for DNA extraction using the PowerLyzer PowerSoil DNA Isolation kit (Qiagen, Hilden, Germany). Two parallel DNA extraction batches for each sample included randomized samples, environmental controls, and two extraction controls per batch.
Triplicate PCR reactions for the V4-V5 region of the 16S rRNA gene employed fusion primers which consisted of Illumina-specific adaptors for sequencing, indexes and barcodes for multiplexing samples, and the primer set 515F (5′-CCAGCAGCYGCGGTAAN-3′) and 926R (8:1:1 mixture of 5′-CCGTCAATTCNTTTRAGT-3′, 5′-CCGTCAAT TTCTTTGAGT-3′, 5′-CCGTCTATTCCTTTGANT-3′). No-template negative controls were prepared for each sample by moving 25 μl of the 125 μl reaction to a separate well prior to final assembly of the sequencing reaction. The remaining 100 μl was split into triplicate 33 μl reactions following addition of 6 μl template DNA. Thermocycler conditions for amplification included initial denaturation at 94°C for 3 min, 30 cycles of denaturing at 94°C for 30 s, annealing at 57°C for 45 s, and extension at 72°C for 1 min, and a final extension of 72°C for 10 min. Visualization on a TapeStation 4200 using D1000 ScreenTapes and D1000 DNA ladder (Agilent Technologies, California, United States) confirmed amplification. Treatment with AMPure XP magnetic beads (Agencourt, Beckmann-Coulter, United States) according to the manufacturer’s instructions, purified and concentrated the amplicons libraries. Pooled amplicon libraries at equivalent amounts of DNA (determined on the TapeStation 4200) ensured equal coverage across samples during sequencing. 515F/926R primers may also amplify host 18S rRNA genes yielding fragments of ∼760 bp length. We thus size selected the target 16S amplicon within a size range of 425 and 625 bp with a BluePippin instrument using a 1.5% agarose cassette and R2 marker (Sage Science, Massachusetts, United States). The multiplexed amplicon pools were then sequenced on an Illumina MiSeq instrument using a V3 600 cycle kit according to the manufacturer’s protocol (Illumina, California, United States). Samples, accession numbers and associated contextual data are listed in Supplementary Table 1.
Raw sequences were analyzed using DADA2 (Callahan et al., 2016) following the DADA2 Pipeline Tutorial v1.161. In brief, forward and reverse reads were quality-trimmed to 275 bp and 205 bp, respectively, and primer sequences (17 bp forward, 21 bp reverse) were removed. Reads with more than two expected errors were discarded, paired reads were merged, and chimeric sequences were removed. Species level taxonomy was assigned with the silva_nr_v138_train_set and silva_species_assignment_v138 based on the Silva small subunit reference database SSURef v138 [release date: 16-Dec-2019 (Quast et al., 2013)]. After quality control and removal of blanks and controls we obtained 42 bacterial amplicon datasets comprising a total of 6.59 × 107 sequence reads belonging to 2024 unique ASVs. Each sample had on average 1.46 ± 0.41 × 105 reads (average ± standard deviation) and 352 ± 139 unique ASVs (Supplementary Table 1). The ASV-by-sample table was used to determine the number of observed ASV, absolute singletons, relative singletons, relative abundance, and composition. Alpha diversity (richness, Shannon entropy, Inverse Simpson Diversity and Chao1 estimated richness) was calculated from the ASV-by-sample table using a subsampling of 87327 randomly chosen sequences to account for unequal sampling effort (Supplementary Table 1). Differences in diversity between conditions were tested using the Wilcoxon signed rank-test (ggsignif) as implemented in ggplot2 (Wickham, 2009). Bray-Curtis dissimilarities (Bray and Curtis, 1957) between all samples were calculated and used for two-dimensional non-metric multidimensional scaling (NMDS) ordinations with 20 random starts (Kruskal, 1964). All analyses were carried out with VisuaR v022 a publicly available workflow based on the R statistical environment, custom R scripts and several R packages including vegan (Oksanen et al., 2012) and ggplot2.
We have investigated the effect of common environmental stressors on the development and regeneration of Nematostella vectensis. Nematostella have a relatively rapid development, reaching a young independent feeding stage with four tentacles in about 1 week (Genikhovich and Technau, 2009; Marlow et al., 2009; Layden et al., 2016). Embryos were collected directly after spawning and between 200–300 embryos were placed in different concentrations of the common pollutants, dioctyl phthalate, which is derived from plastics, or potassium nitrate (KNO3), a common component of widely used fertilizers, both of which are often found in coastal waters. Two weeks post exposure the overall body length of the animal was measured from the tip of the pharynx to the foot of the animals (Figure 1A). Embryos exposed to phthalates or nitrites exhibited a gross difference in overall body size as compared to the control animals (Figures 1A–D). Increasing the concentration of stressors did not correlate with a decrease in body size, and similar defects in body size were observed in increased concentration (Figures 1B,C). However, exposure to higher concentrations, 20 μM and above led to possible toxicity and high morbidity. When the morphology of the animals was carefully examined, the animals generally appeared to develop all the expected visible structures. However, defects were noted first in the tentacles. Animals treated with phthalates all had fewer tentacles and the tentacles that did grow were uneven in length and number (Figures 2, 3). We noted other defects in which some animals had bifurcated tentacles, while others had tentacles that permanently curled at the end (Figure 1). The Nematostella pharynx, where the food is taken in did not show a significant difference in size in low concentration of phthalates, which contrasts with a significant decrease in the overall length of the pharynx at 10 μM concentrations (Figure 2C). Finally, developing animals exposed to low and high concentrations of phthalates exhibited significantly shorter mesenteries compared to control animals (Figure 2D).
Figure 1. Environmental Stressors Lead to Growth defects in Nematostella embryos. (A) Nematostella embryos exposed to phthalate are significantly shorter when compared to control siblings. (B) Increasing concentration of phthalates does not cause greater defects in overall length of the developing animals (control n = 149, 1 μM: n = 162, 10 μM n = 161). (C) Exposure to Potassium nitrate also causes defects in overall embryonic growth in comparison to control. (D) Defects in embryo size do not scale with increasing doses of KNO3 (control n = 90, 1 μM: n = 78, 10 μM n = 65). *p ≤ 0.05, **p ≤ 0.01, ***p ≤ 0.001, N.S. is not significant. Scale bar = 100 μM.
Figure 2. Early exposure to phthalates leads to defects in tissue development. Two weeks post fertilization Nematostella have grown at least four tentacles and are independently feeding. Animals exposed to 1 or 10 μM phthalate have much shorter tentacles (A) and developed fewer tentacles when compared to control animals (B). The length of pharynx was also measured but no significant difference was found in overall length in comparison to sibling control animals at 1 μM but at 10 μM the pharynx was significantly shorter (C). The overall length of the mesenteries was also found to be shorter in animals exposed to phthalates (D). (control n = 76, 1 μM n = 113, 10 μM n = 82) *p ≤ 0.05, **p ≤ 0.01, ***p ≤ 0.001, N.S. is not significant.
Figure 3. Exposure to Potassium Nitrate leads to defects in tissue development. Animals exposed to 1 or 10 μM KNO3 have much shorter tentacles (A) and developed fewer tentacles when compared to control animals (B). The length of pharynx was also measured but no significant difference was found in overall length in comparison to sibling control animals (C). The overall length of the mesenteries was also found to be shorter in animals exposed to phthalates (D). Control n = 72, 1 μM n = 66, 10 μM n = 68, *p ≤ 0.05, **p ≤ 0.01, ***p ≤ 0.001, N.S. is not significant.
The same quantifications were carried out on animals exposed to KNO3 during early development. In these animals, we observed the same decrease in overall tentacle length and decrease in tentacle number (Figures 3A,B). Interestingly, embryos exposed to KNO3 did not have any significant difference in the length of their pharynx in comparison to controls (Figure 3C). However, these animals had much smaller mesenteries in comparison to control animals that were growing in KNO3 concentrations greater than 10 μM but not at lower concentrations. This observation suggests that growth of the mesenteries tolerates low levels of KNO3 (Figure 3D). The dramatic difference in overall size of the animals, and in most cases aberrant development of mesenteries and tentacles, prompted questions about the impact of tested environmental stressors on specialized cell types. Cnidocytes are an ectodermal derived cell types used for defense, prey capture and environmental sensing. Overall, we found that animals exposed to Phthalates or KNO3 have cnidocytes on the ectodermal layer but fewer than in controls (Figures 4A–C). We also examined if earlier in development cnidocytes develop was effected, we carried out in situs on embryos 72 h post fertilization using the gene minicollagen which is expressed in all developing cnidocytes (Babonis and Martindale, 2017) and found decreased expression in phthalate of nitrite treated embryos (Supplementary Figure 1), suggesting that that these pollutants may affect early differentiation of these specialized cell types. We more closely examined the cnidocytes on the tentacles, which the animals use to capture their food and found far fewer cnidocytes on the tentacles of animals exposed to the environmental stressors (E, F). In particular, animals incubated in KNO3 had very few cnidocytes of the normal elongated shape, suggesting that these pollutants may affect differentiation of these specialized cell types.
Figure 4. Common environmental stressors cause defects in cnidocytes. (A) DAPI staining showing cnidocytes all over the outer body wall (A) and on the tentacle (D). Embryos exposed to phthalates appear to have fewer cnidocytes all over the body (B), especially on the tentacles (D). Similarly, embryos exposed to KNO3 have slightly less cnidocyte on the body (C). Higher magnification images of the tentacles show fewer cnidocytes (D,F). (A–C), 10 × Scale bar = 500 μm (D–E), 20 ×, Scale bar = 50 μm.
Since Nematostella has the robust ability to regenerate (Amiel et al., 2015; Schaffer et al., 2016; Warner et al., 2018), we examined whether the presence of these environmental stressors influences regrowth of the tentacles. Adult animals were amputated through the bottom of the pharynx and then incubated in the presence of 20 μM Phthalate or KNO3 for 2 weeks. At the end of the time period, the animals were relaxed and imaged. Animals exposed to phthalates regenerated the pharynx and partial tentacles. In all animals’ defects in tentacle regeneration were observed, in most cases different numbers and lengths of tentacles were regenerated (Figure 5B) and often the tentacles were fused or bifurcated (data not shown). Animals exposed to KNO3 mainly failed to regrow the tentacles or in some cases 1 tentacle regrew, even in lower concentrations of KNO3 the oral portion of the animal failed to regrow (Figure 5C). We also examined if the cnidocytes are regenerated on the limited tentacles that are regenerated. Like in embryonic development, we observed a decrease in number of cnidocytes per tentacle in comparison to the control animal (Figures 5D–F), and the cnidocytes in animals exposed to the stressors were shorter and less elongated than in control animals.
Figure 5. Common environmental stressors inhibit oral regeneration. Nematostella can regenerate throughout life. Adult animals were amputated through the pharynx and assessed for completion of oral regeneration 2 weeks post injury. (A) Control animals regenerated all tentacles (n = 85). (B) Animals exposed to phthalates at 20 μM failed to regenerate tentacles of the correct length (n = 90). In contrast animals exposed to KNO3 during regeneration mainly failed to regenerate tentacles, occasionally one tiny tentacle was regenerated (C) (n = 95). Staining of cnidocytes revealed that control animals fully regenerate the cnidocytes within 2 weeks (D), while animals exposed to phthalates or KNO3 have very few cnidocytes on the limited tentacles that are regenerated (E,F). (D–F) Scale bar = 50 μm.
Taken together, these data suggest that early exposure to two common environmental stressors has a major impact on developmental growth, possibly due to a failure of the animals to feed due to lack of normal tentacles and decreased numbers of cnidocytes which are used to capture their food. Additionally, we observed that exposure of adult Nematostella to these pollutants after amputation leads either to complete failure of tentacle regeneration or results in major defects in the number and length of the tentacles and of the cnidocytes regenerated.
To determine whether early exposure to environmental pollutants not only causes developmental defects but also leads to changes in the microbiome, we employed 16S rRNA gene sequencing to investigate the microbiome of Nematostella embryos after a 10-day exposure to different concentrations of KNO3 or phthalates. In addition, we tested each pollutant concentration on animals that were grown in either instant ocean medium or natural seawater, to test if a potential effect of the pollutants is similar under different environmental regimes. The exposure to pollutants caused shifts in the microbial community structure and composition. Within a set of experiments, e.g., exposing the animals to different concentrations of KNO3, these shifts were minor regarding the richness and evenness of the microbiomes (Figure 6), independent of the medium the animals were grown in. This suggests that the exposure to increasing pollutant concentrations did not change the richness of the animal-associated microbiome. Although no significant differences in alpha diversity were observed between pollutant treatments and their controls, alpha diversity was significantly different between incubation media (Figure 6). Animals that were grown in instant ocean artificial seawater had a significantly lower diversity than those grown in seawater from the nearby Sippewissett salt marsh, a native habitat of Nematostella (Supplementary Figure 2 NMDS) which is rich in natural seawater microbiota. Yet the overall lack of significant changes in microbial richness and evenness with exposure to different concentrations of KNO3 or different concentrations of phthalates was similar in both media. Despite minor differences in alpha diversity, we saw substantial change in the community structure. This trend was independent of the basal medium used to incubate the animals. The communities that have been exposed to the two different pollutants KNO3 and phthalates were well separated in an NMDS ordination (Figure 7). Especially in the case of nitrate, it seems as if there was a clear pattern of increasing community dissimilarity with increasing concentration. The clear separation based on pollutants and concentration is similar in animals that were raised in the two different sources of salt water, despite the overall large differences caused by the two types of salt water used for growth of the embryos (Supplementary Figure 2 NMDS). The three biological replicates generally cluster tightly in the NMDS, indicating that each pollutant and each concentration caused very similar deterministic community shifts.
Figure 6. Alpha diversity indices of different groupings of samples. Richness is shown as the number of observed bacterial amplicon sequence variants (ASV). Evenness is represented by the Inverse Simpson Diversity, Shannon entropy takes into account both richness and evenness. The number (n) of included samples per group is shown.
Figure 7. Non-metric multidimensional scaling ordination based on a distance matrix of all available amplicon sequence variants (ASVs). The microbial communities exposed to different pollutants and concentrations are significantly different from each other. Each dot represents the total bacterial community of a sample, the closer the dots are the more similar are the underlying communities. The dots of each condition are connected to the weighted average mean of within group distances (centroid), ellipses represent one standard deviation of the centroid. Ellipses that do not overlap generally show substantial differences between groups.
The relative sequence abundance of an uncultured population within the genus Flavobacterium was elevated in the KNO3 treatments compared to the unamended controls and phthalates treatments, and relative abundance of this group increased with increasing nitrate concentrations (Figure 8). In contrast, a population affiliating with the genus Mariniflexile, which was abundant in the unamended conditions, decreased at higher KNO3 concentrations. The animals that were grown in natural seawater, and were thus exposed to a diverse marine microbiome, were colonized by very different genera than those cultured in instant ocean. Here, the KNO3 and phthalates exposed animals showed a very different microbiome. In the KNO3 condition, the most sequence abundant population belonged to Pseudomonas and the phthalate cultures showed high sequence abundances of an unknown genus within the family Saprospiraceae (Figure 7). Overall, the majority of reads in any given condition belonged to organisms within the Bacteroidia (marked with an asterisk in Figure 8), including six of the seven most abundant lineages on species level, which accounted for more than 50% of the reads on average per sample.
Figure 8. Bacterial community composition of populations at species level. Each bar represents one sample and shows the 20 species-level lineages with the highest relative sequence abundance averaged across all datasets. All other lineages are summed up as “Other.” The average composition across all samples is shown in the last column. An unclassified population within the genus Flavobacterium (yellow) substantially increases with increasing nitrate concentrations. “Asterisk” denotes lineages that belong to the phylum Bacteroidia. Lineages that were not classified to genus level represent species in unclassified families (e.g., Saprospiraceae – red) or unclassified orders (e.g., Kordiimonadales – sky blue).
In summary, our results indicate that embryonic exposure to two common environmental pollutants leads to severe defects in embryonic development. In addition, we found that the source of the water in which embryos are grown influences the complexity of an animal’s microbiome.
Nematostella vectensis undergo rapid development when cultured in lab conditions, fertilized embryos emerge from the egg mass at around 48 h post fertilization and quickly developed into ciliated planula, with an apical cilium by 3 days. The free-swimming planula progressively changes shape, becoming more elongated and by 5–7 days have 4 tentacle buds (Hand and Uhlinger, 1992; Layden et al., 2016). We investigated the effect of early exposure to two common environmental stressors found in marsh waters on the early development of Nematostella. Phthalates and potassium nitrate were used as environmental stressors in this experiment because they are some of the most frequently found toxins in heavily populated marsh areas. These impurities in the water come mainly from plastics, which leach phthalic acid esters from plasticizers, and from freshwater run-off containing nitrates from fertilizers into the march areas. We observed very significant overall defects in the size of the embryos by two-week post-fertilization, all embryos incubated even in low concentrations of the pollutants were overall much shorter than control embryos (Figure 1). Furthermore, we observed clear defects in the number and length of the tentacles and in size of the pharynx and mesenteries (Figures 2, 3). We also looked more closely at the composition of the tentacles, Nematostella have an ectodermal derived specialized cell type known as the cnidocyte which it uses as a defense mechanism and to capture its food. In all cases we found a reduction in number of the cnidocytes especially in the tentacles (Figure 4). The lack of cnidocytes especially on the tentacles is suggestive of an inability of the animals to capture their food. When the first tentacles are observed around 7 days post-fertilization, we started to feed the animals rotifers, as we noted that at this timepoint no significant size difference was observed between animal, however by 10 days we could already see clear size difference. Additionally, when we observed feeding behavior under the microscope, we could see the animals in the pollutants had no rotifers or fewer rotifers in their abdomen. Work of Ikmi et al. (2020) has identified that tentacle growth and increase in number occurs in a feeding dependent manner, this would suggest that the failure in growth we see in these stressed embryos is partially due to an inability to obtain enough food to drive tentacle growth. Far fewer cnidocytes, the specialized cell type that the animals use to capture its food were seen in the animals exposed to the environmental pollutants (Figure 4). We used a high concentration of DAPI staining method that label the poly-Y-glutamate in the matrix of mature cnidocytes to identify these cells (Szczepanek et al., 2002; Babonis and Martindale, 2014; Babonis and Martindale, 2017), our images suggest a lack of mature cnidocytes, however from this data we cannot distinguish whether or not this is due to apoptosis of these mature cells due to exposure to the toxins or if there is a defect in the early specification and differentiation of these cells types. We also tested the impact of these environmental pollutants on the adult animal’s ability to regenerate its tentacles. Here we again saw strong phenotypes with a failure to regenerate the correct number and length of tentacles (phthalates) or in many cases exposure to KNO3 resulted in a lack of regeneration or 1 or 2 tiny tenacles. Imaging of the specialized cnidocytes on the regenerated tentacles again showed a lack of these specialized cell types in the regenerates in comparison to controls (Figure 5). The magnitude of the defects in adult regeneration suggests in the case of exposure to KNO3 a failure to deploy the “regeneration program,” while the phthalate phenotype suggests more a fault in the execution of the “regeneration program” leading to incomplete regeneration and differentiation of the required amount of tissue and differentiated cell types.
Interestingly, other studies of the effect of phthalates on development in several species including zebrafish and frogs has also identified defects in body growth and spinal defects (Philippat et al., 2012; Kinch et al., 2016; Jergensen et al., 2019; Qian et al., 2020) similar to what we see here with the marine invertebrate Nematostella, suggesting a very common negative side effect of exposure to phthalates during embryonic development is slower body growth and defects in cells of the ectodermal lineage. Similar defects were seen when embryos were incubated in potassium nitrate and this has been observed in other species like newts, frogs and zebrafish (Fan and Steinberg, 1996; Ortiz et al., 2004; Orton et al., 2006; Ortiz-Santaliestra et al., 2007; Ortiz-Santaliestra and Sparling, 2007; Kinch et al., 2016; Conlin et al., 2018). Additionally in these species negative impacts on the endocrine system and on fertility have been documented (Fan and Steinberg, 1996; Fisher, 2004; Orton et al., 2006; Krishnamurthy and Smith, 2011; Jannat et al., 2014; Conlin et al., 2018; Lv et al., 2020; Sharma et al., 2020; Tang et al., 2020).
We also explored how these environmental pollutants may affect the host’s microbiome. Prior reports describe shifts in the microbiome that might serve as indicators for changes in host health in marine organisms, including corals (Glasl et al., 2016) and vertebrates (Sehnal et al., 2021). In the case of Nematostella previous research has shown that the host microbiome is affected by changes in temperature and light conditions (Leach et al., 2019). Here, we studied potential connections between the Nematostella microbiome and host during exposure to environmental pollutants. In the samples of animals that were grown in Instant Ocean artificial seawater without pollutants we found high sequence abundances of populations affiliating with the genera Tenacibaculum, Flavobacterium and Mariniflexile (Figure 8). In the datasets from animals grown in unamended seawater Pseudomonas and Saprospiraceae were most abundant, indicating that the medium that was used to culture the embryos had a large effect on which microbiota colonized the animals, supporting previous studies showing substantial variability of the Nematostella microbiome with environment, season and biogeography (Har et al., 2015; Mortzfeld et al., 2016). It was also shown that stochastic community assembly processes can play a major role and result in different host-associated microbiomes independent of the traits of the host or the microbiota (Douglas, 2019). Such stochastic assembly processes during colonization may explain that not all microbiomes of animals grown in unamended seawater had a similar community structure after 10 days of incubation. The differences between these controls indicate that substantial variation exists in the microbiomes of groups of embryos grown separately, and that the separation of embryos into different wells early on may drive changes in the development of an organism’s microbiome and lead to different community trajectories.
Despite the phylogenetic differences on genus-level, the microbiome of all conditions featured sequence abundant populations affiliating with the phylum Bacteroidia, which were shown to be of particular importance in the microbiome at early stages of the animals’ development (Mortzfeld et al., 2016). The different genera that were enriched in the microbiome under certain conditions often belonged to the same family within the Bacteroidia and may thus be functionally redundant indicating that microbial function played a role in microbiome assembly. Overall, the composition of the Nematostella microbiome was similar to that reported in previous studies where Bacteroidia and Proteobacteria sequences represented the most abundant taxa (Har et al., 2015; Mortzfeld et al., 2016; Baldassarre et al., 2021). We found Spirochetes in low abundance in the host microbiomes as well, which agrees with a previous study showing that Spirochetes colonize the capitulum (Bonacolta et al., 2021).
When comparing the unamended animal microbiomes to those exposed to different concentrations of pollutants, we observed that the detrimental effects caused by each pollutant on the animals’ development apparently did not have a similar impact on the animal-associated microbiome. We did not find significant changes in the richness and evenness of the microbial communities when comparing animals that were grown without pollutant with those grown under different concentrations of each pollutant. The slightly higher variability in microbial richness especially between the phthalate treatments as compared to the unamended cultures (Figure 6) may be due to increased stress, as stressors can impact alpha diversity (Rocca et al., 2019). The effect of pollutants apparently manifested in shifts of community structure (Figure 7) and composition (Figure 8) rather than richness. These shifts indicate that taxa in the N. vectensis microbiome were replaced rather than completely eliminated. In contrast to certain unamended cultures that featured relatively strong differences in community composition, potentially caused by stochastic processes during colonization, deterministic processes may have played an important role in the pollutant treated animals., In most cases the microbiomes of the different treatments formed well separated clusters in the NMDS ordination with low beta diversity within replicates of a given treatment. Such patterns can be caused by stressors that select for certain taxa and increase their abundance leading to deterministic community changes (Zaneveld et al., 2017).
The high relative sequence abundance of Tenacibaculum sp. across the Instant Ocean artificial seawater incubations, but not in those using Sippewissett salt marsh water growth medium, is likely due to initial differences in microbial community composition between the growth media. However, Tenacibaculum spp. are capable of thriving on polysaccharides and proteins (Pérez-Pascual et al., 2017) and many are pathogenic or associated with diseased fish and anemones (Wang et al., 2008) which might explain the slight increase in relative sequence abundance for this organism in the 10 μM phthalate treatment when the health of Nematostella was impaired. Flavobacterium increased in relative abundance and a member of the genus Mariniflexile decreased in relative abundance with increasing nitrate concentrations. All three genera belong to the family Flavobacteriaceae and are known to include marine species that can degrade polysaccharides (Barbeyron et al., 2008; Nedashkovskaya et al., 2014), yet only the genus Flavobacterium contains organisms that can reduce nitrate (Nupur et al., 2013). It is likely that they have similar niches concerning carbon sources but can differently utilize nitrate. For example, nitrate-reducing Flavobacterium columnare are associated with disease in fish experiencing environmental stress (Abdelhamed et al., 2021). In this situation, nitrate can be used by opportunist pathogenic Flavobacterium sp. as an alternative electron acceptor in oxygen-limited microenvironments, such as in biofilms or during infection of tissue (Abdelhamed et al., 2021).
The phthalate exposed microbiomes in the Sippewissett salt marsh seawater cultures were enriched with a lineage affiliating with Saprospiraceae. These organisms also affiliate with Bacteroidetes and are not only related to the most abundant clades in the instant ocean cultures but have a similar metabolic capabilities degrading complex organic matter such as polysaccharides (McIlroy and Nielsen, 2014). It is thus very likely that functionally redundant, yet taxonomically different clades were recruited from the communities of the initial culture medium. Organisms belonging to the genus Pseudomonas were present all animal microbiomes (Figure 8), yet sporadically appeared in higher relative sequence abundance in animals cultivated in Sippewissett salt marsh seawater. This genus is known to contain organisms able to degrade phthalates (Vamsee-Krishna and Phale, 2008), however, the highest relative sequence abundances of this organism were found mainly in KNO3 -treated samples and unamended controls, and the observed pattern does not indicate that the presence of phthalate or KNO3 selected for Pseudomonas in either growth media. The activity of the microbiome determines potential physiological feedbacks between the microbiome and host and is thus an important factor for the examination of holobiont health. Due to the limitations of taxonomy-based analyses future studies would benefit from analyses of the functional capabilities and activity of the microbiome to understand feedbacks between the microbiome and host health.
In this study, the Nematostella vectensis microbiome was significantly influenced by the growth medium (Supplementary Figure 2 NMDS). The reported deterministic changes in the microbial community structure caused by the pollutants can therefore be easily missed. From the findings of this study, we conclude that the detrimental effects of pollutants on the development of marine invertebrates are not always mirrored to the same degree in the animals’ microbiome. However, as the embryos were exposed to the pollutants for 10 days only this may not be enough time to result in a substantial change in the microbiome. Moreover, the source of seawater, biogeography and the environmental variability of Nematostella itself (Darling et al., 2004) can apparently have large effects on the outcome of such cultivation experiments potentially masking the underlying positive or negative trends.
In summary, our results demonstrate that common pollutants found in salt marshes adversely affect the development of Nematostella embryos, ultimately leading to death. This is an important finding as globally populations of Nematostella are decreasing and as their natural habitats is the marsh lands, they are very susceptible to pollution. This study looks mainly at the effect on embryos, in the future it will be interesting to determine if exposure of adult animals to these pollutants also causes changes in the microbiome and ultimate fitness of the offspring.
The 16S rRNA gene sequence datasets are publicly archived at NCBI under BioProject PRJNA767880.
SK carried out development and regeneration assays, took all measurements and prepared the graphs using PRISM. TR, ED-J, and EL contributed to the testing of stressors on embryos and regenerating animals. VF carried out the DNA isolation and library preparation and sequencing. MS helped design and oversee the microbial sequencing. ER analyzed and visualized nucleic acid sequence data. KE and ER conceived the project, oversaw the design and execution of the experiments and wrote the manuscript. All authors read and contributed to the manuscript.
This work was funded by a Pilot Program award to ER and KE from the Microbiome Center at the University of Chicago. The microbiome sequencing was funded by a grant from the McDonnell Initiative to ER. KE was supported by a grant from NICHD R01 HD092451, start-up funds from the MBL and funding from the Owens Family Foundation. ER was supported by start-up funds from the MBL and MLS receives support from the Unger G. Vetlesen Foundation.
The authors declare that the research was conducted in the absence of any commercial or financial relationships that could be construed as a potential conflict of interest.
All claims expressed in this article are solely those of the authors and do not necessarily represent those of their affiliated organizations, or those of the publisher, the editors and the reviewers. Any product that may be evaluated in this article, or claim that may be made by its manufacturer, is not guaranteed or endorsed by the publisher.
We thank Sherlynette Pérez Castro and Keith Sabin who supervised high school students Melissa Ham and Alley Rivera to first work on the effect of environmental stressors on Nematostella regeneration as a science fair project which led to the conception of this project.
The Supplementary Material for this article can be found online at: https://www.frontiersin.org/articles/10.3389/fevo.2021.786037/full#supplementary-material
Abdelhamed, H., Nho, S. W., Karsi, A., and Lawrence, M. L. (2021). The role of denitrification genes in anaerobic growth and virulence of Flavobacterium columnare. J. Appl. Microbiol. 130, 1062–1074. doi: 10.1111/jam.14855
Amiel, A. R., Johnston, H., Chock, T., Dahlin, P., Iglesias, M., Layden, M., et al. (2017). A bipolar role of the transcription factor ERG for cnidarian germ layer formation and apical domain patterning. Dev. Biol. 430, 346–361. doi: 10.1016/j.ydbio.2017.08.015
Amiel, A. R., Johnston, H. T., Nedoncelle, K., Warner, J. F., Ferreira, S., and Rottinger, E. (2015). Characterization of Morphological and Cellular Events Underlying Oral Regeneration in the Sea Anemone, Nematostella vectensis. Int. J. Mol. Sci. 16, 28449–28471. doi: 10.3390/ijms161226100
Amiel, A. R., Michel, V., Carvalho, J. E., Shkreli, M., Petit, C., and Röttinger, E. (2021). [The sea anemone Nematostella vectensis, an emerging model for biomedical research: mechano-sensitivity, extreme regeneration and longevity]. Med. Sci. 37, 167–177. doi: 10.1051/medsci/2020282
Amiel, A. R., and Röttinger, E. (2021). Experimental Tools to Study Regeneration in the Sea Anemone Nematostella vectensis. Methods Mol. Biol. 2219, 69–80. doi: 10.1007/978-1-0716-0974-3_4
Babonis, L. S., and Martindale, M. Q. (2014). Old cell, new trick? Cnidocytes as a model for the evolution of novelty. Integr. Comp. Biol. 54, 714–722. doi: 10.1093/icb/icu027
Babonis, L. S., and Martindale, M. Q. (2017). PaxA, but not PaxC, is required for cnidocyte development in the sea anemone Nematostella vectensis. Evodevo 8:14. doi: 10.1186/s13227-017-0077-7
Babonis, L. S., Martindale, M. Q., and Ryan, J. F. (2016). Do novel genes drive morphological novelty? An investigation of the nematosomes in the sea anemone Nematostella vectensis. BMC Evol. Biol. 16:114. doi: 10.1186/s12862-016-0683-3
Baldassarre, L., Levy, S., Bar-Shalom, R., Steindler, L., Lotan, T., and Fraune, S. (2021). Contribution of Maternal and Paternal Transmission to Bacterial Colonization in Nematostella vectensis. Front. Microbiol. 12:726795. doi: 10.3389/fmicb.2021.726795
Barbeyron, T., L’haridon, S., Michel, G., and Czjzek, M. (2008). Mariniflexile fucanivorans sp. nov., a marine member of the Flavobacteriaceae that degrades sulphated fucans from brown algae. Int. J. Syst. Evol. Microbiol. 58, 2107–2113. doi: 10.1099/ijs.0.65674-0
Bonacolta, A. M., Connelly, M. T., Rosales, S. M., del Campo, J., and Traylor-Knowles, N. (2021). The starlet sea anemone, Nematostella vectensis, possesses body region-specific bacterial associations with spirochetes dominating the capitulum. FEMS Microbiol. Lett. 368:fnab002. doi: 10.1093/femsle/fnab002
Bosch, T. C. G., and McFall-Ngai, M. (2021). Animal development in the microbial world: re-thinking the conceptual framework. Curr. Top. Dev. Biol. 141, 399–427. doi: 10.1016/bs.ctdb.2020.11.007
Bossert, P., and Thomsen, G. H. (2017). Inducing Complete Polyp Regeneration from the Aboral Physa of the Starlet Sea Anemone Nematostella vectensis. J. Vis. Exp. 119:54626. doi: 10.3791/54626
Bossert, P. E., Dunn, M. P., and Thomsen, G. H. (2013). A staging system for the regeneration of a polyp from the aboral physa of the anthozoan Cnidarian Nematostella vectensis. Dev. Dyn. 242, 1320–1331. doi: 10.1002/dvdy.24021
Bray, J. R., and Curtis, J. T. (1957). An ordination of the upland forest communities of Southern Wisconsin. Ecol. Monogr. 27, 325–349. doi: 10.2307/1942268
Callahan, B. J., McMurdie, P. J., Rosen, M. J., Han, A. W., Johnson, A. J. A., and Holmes, S. P. (2016). DADA2: high-resolution sample inference from Illumina amplicon data. Nat. Methods 13, 581–583. doi: 10.1038/nmeth.3869
Camargo, J. A., Alonso, A., and Salamanca, A. (2005). Nitrate toxicity to aquatic animals: a review with new data for freshwater invertebrates. Chemosphere 58, 1255–1267. doi: 10.1016/j.chemosphere.2004.10.044
Conlin, S. M., Tudor, M. S., Shim, J., Gosse, J. A., Neilson, A., and Hamlin, H. J. (2018). Elevated nitrate alters the metabolic activity of embryonic zebrafish. Environ. Pollut. 235, 180–185. doi: 10.1016/j.envpol.2017.12.069
Daniel, Y. C. W., Kumar, S., and Hedges, S. B. (1999). Divergence Time Estimates for the Early History of Animal Phyla and the Origin of Plants, Animals and Fungi. Proc. Biol. Sci. 266, 163–171. doi: 10.1098/rspb.1999.0617
Darling, J. A., Reitzel, A. M., and Finnerty, J. R. (2004). Regional population structure of a widely introduced estuarine invertebrate: nematostella vectensis Stephenson in New England. Mol. Ecol. 13, 2969–2981. doi: 10.1111/j.1365-294X.2004.02313.x
Darling, J. A., Reitzel, A. R., Burton, P. M., Mazza, M. E., Ryan, J. F., Sullivan, J. C., et al. (2005). Rising starlet: the starlet sea anemone, Nematostella vectensis. Bioessays 27, 211–221. doi: 10.1002/bies.20181
Douglas, A. E. (2019). Simple animal models for microbiome research. Nat. Rev. Microbiol. 17, 764–775. doi: 10.1038/s41579-019-0242-1
DuBuc, T. Q., Traylor-Knowles, N., and Martindale, M. Q. (2014). Initiating a regenerative response; cellular and molecular features of wound healing in the cnidarian Nematostella vectensis. BMC Biol. 12:24. doi: 10.1186/1741-7007-12-24
Elran, R., Raam, M., Kraus, R., Brekhman, V., Sher, N., Plaschkes, I., et al. (2014). Early and late response of Nematostella vectensis transcriptome to heavy metals. Mol. Ecol. 23, 4722–4736. doi: 10.1111/mec.12891
Essock-Burns, T., Bongrand, C., Goldman, W. E., Ruby, E. G., and McFall-Ngai, M. J. (2020). Interactions of Symbiotic Partners Drive the Development of a Complex Biogeography in the Squid-Vibrio Symbiosis. mBio 11, e00853–20. doi: 10.1128/mBio.00853-20
Fan, A. M., and Steinberg, V. E. (1996). Health implications of nitrate and nitrite in drinking water: an update on methemoglobinemia occurrence and reproductive and developmental toxicity. Regul. Toxicol. Pharmacol. 23, 35–43. doi: 10.1006/rtph.1996.0006
Fisher, J. S. (2004). Environmental anti-androgens and male reproductive health: focus on phthalates and testicular dysgenesis syndrome. Reproduction 127, 305–315. doi: 10.1530/rep.1.00025
Fraune, S., Augustin, R., Anton-Erxleben, F., Wittlieb, J., Gelhaus, C., Klimovich, V. B., et al. (2010). In an early branching metazoan, bacterial colonization of the embryo is controlled by maternal antimicrobial peptides. Proc. Natl. Acad. Sci. U. S. A. 107, 18067–18072. doi: 10.1073/pnas.1008573107
Fraune, S., and Bosch, T. C. (2010). Why bacteria matter in animal development and evolution. Bioessays 32, 571–580. doi: 10.1002/bies.200900192
Genikhovich, G., and Technau, U. (2009). The starlet sea anemone Nematostella vectensis: an anthozoan model organism for studies in comparative genomics and functional evolutionary developmental biology. Cold Spring Harb. Protoc. 2009:pdb.emo129. doi: 10.1101/pdb.emo129
Glasl, B., Herndl, G. J., and Frade, P. R. (2016). The microbiome of coral surface mucus has a key role in mediating holobiont health and survival upon disturbance. ISME J. 10, 2280–2292. doi: 10.1038/ismej.2016.9
Gugliandolo, E., Licata, P., Crupi, R., Albergamo, A., Jebara, A., Lo Turco, V., et al. (2020). Plasticizers as Microplastics Tracers in Tunisian Marine Environment. Front. Mar. Sci. 7:589398. doi: 10.3389/fmars.2020.589398
Hand, C., and Uhlinger, K. R. (1992). The Culture, Sexual and Asexual Reproduction, and Growth of the Sea Anemone Nematostella vectensis. Biol. Bull. 182, 169–176. doi: 10.2307/1542110
Har, J. Y., Helbig, T., Lim, J. H., Fernando, S. C., Reitzel, A. M., Penn, K., et al. (2015). Microbial diversity and activity in the Nematostella vectensis holobiont: insights from 16S rRNA gene sequencing, isolate genomes, and a pilot-scale survey of gene expression. Front. Microbiol. 6:818. doi: 10.3389/fmicb.2015.00818
Heath-Heckman, E. A., Foster, J., Apicella, M. A., Goldman, W. E., and McFall-Ngai, M. (2016). Environmental cues and symbiont microbe-associated molecular patterns function in concert to drive the daily remodelling of the crypt-cell brush border of the Euprymna scolopes light organ. Cell. Microbiol. 18, 1642–1652. doi: 10.1111/cmi.12602
Hu, R., Zhao, H., Xu, X., Wang, Z., Yu, K., Shu, L., et al. (2021). Bacteria-driven phthalic acid ester biodegradation: current status and emerging opportunities. Environ. Int. 154:106560. doi: 10.1016/j.envint.2021.106560
Ikmi, A., Steenbergen, P. J., Anzo, M., Mcmullen, M. R., Stokkermans, A., Ellington, L. R., et al. (2020). Feeding-dependent tentacle development in the sea anemone Nematostella vectensis. Nat. Commun. 11:4399. doi: 10.1038/s41467-020-18133-0
Jannat, M., Fatimah, R., and Kishida, M. (2014). Nitrate (NO3(-)) and nitrite (NO2(-)) are endocrine disruptors to downregulate expression of tyrosine hydroxylase and motor behavior through conversion to nitric oxide in early development of zebrafish. Biochem. Biophys. Res. Commun. 452, 608–613. doi: 10.1016/j.bbrc.2014.08.114
Jergensen, T., Cusmano, D., and Roy, N. M. (2019). Di-butyl phthalate (DBP) induces craniofacial defects during embryonic development in zebrafish. Ecotoxicology 28, 995–1002. doi: 10.1007/s10646-019-02100-7
Kinch, C. D., Kurrasch, D. M., and Habibi, H. R. (2016). Adverse morphological development in embryonic zebrafish exposed to environmental concentrations of contaminants individually and in mixture. Aquat. Toxicol. 175, 286–298. doi: 10.1016/j.aquatox.2016.03.021
Kirillova, A., Genikhovich, G., Pukhlyakova, E., Demilly, A., Kraus, Y., and Technau, U. (2018). Germ-layer commitment and axis formation in sea anemone embryonic cell aggregates. Proc. Natl. Acad. Sci. U. S. A. 115, 1813–1818. doi: 10.1073/pnas.1711516115
Kraus, Y., and Technau, U. (2006). Gastrulation in the sea anemone Nematostella vectensis occurs by invagination and immigration: an ultrastructural study. Dev. Genes Evol. 216, 119–132. doi: 10.1007/s00427-005-0038-3
Krishnamurthy, S. V., and Smith, G. R. (2011). Combined effects of malathion and nitrate on early growth, abnormalities, and mortality of wood frog (Rana sylvatica) tadpoles. Ecotoxicology 20, 1361–1367. doi: 10.1007/s10646-011-0692-3
Kruskal, J. B. (1964). Nonmetric multidimensional scaling: a numerical method. Psychometrika 29, 115–129. doi: 10.1007/BF02289694
Layden, M. J., Rentzsch, F., and Rottinger, E. (2016). The rise of the starlet sea anemone Nematostella vectensis as a model system to investigate development and regeneration. Wiley Interdiscip. Rev. Dev. Biol. 5, 408–428. doi: 10.1002/wdev.222
Leach, W. B., Carrier, T. J., and Reitzel, A. M. (2019). Diel patterning in the bacterial community associated with the sea anemone Nematostella vectensis. Ecol. Evol. 9, 9935–9947. doi: 10.1002/ece3.5534
Lv, H., Liu, B., and Qin, Y. (2020). Isosorbide mononitrate promotes angiogenesis in embryonic development of zebrafish. Genet. Mol. Biol. 43:20190233. doi: 10.1590/1678-4685-gmb-2019-0233
Marlow, H., Roettinger, E., Boekhout, M., and Martindale, M. Q. (2012). Functional roles of Notch signaling in the cnidarian Nematostella vectensis. Dev. Biol. 362, 295–308. doi: 10.1016/j.ydbio.2011.11.012
Marlow, H. Q., Srivastava, M., Matus, D. Q., Rokhsar, D., and Martindale, M. Q. (2009). Anatomy and development of the nervous system of Nematostella vectensis, an anthozoan cnidarian. Dev. Neurobiol. 69, 235–254. doi: 10.1002/dneu.20698
McClelland, J. W., and Valiela, I. (1998). Linking nitrogen in estuarine producers to land-derived sources. Limnol. Oceanogr. 43, 577–585. doi: 10.4319/lo.1998.43.4.0577
McIlroy, S. J., and Nielsen, P. H. (2014). “The Family Saprospiraceae,” in The Prokaryotes: other Major Lineages of Bacteria and The Archaea, eds E. Rosenberg, E. F. Delong, S. Lory, E. Stackebrandt, and F. Thompson (Berlin, Heidelberg: Springer Berlin Heidelberg).
Mortzfeld, B. M., Urbanski, S., Reitzel, A. M., Künzel, S., Technau, U., and Fraune, S. (2016). Response of bacterial colonization in Nematostella vectensis to development, environment and biogeography. Environ. Microbiol. 18, 1764–1781. doi: 10.1111/1462-2920.12926
Nedashkovskaya, O. I., Balabanova, L. A., Zhukova, N. V., Kim, S.-J., Bakunina, I. Y., and Rhee, S.-K. (2014). Flavobacterium ahnfeltiae sp. nov., a new marine polysaccharide-degrading bacterium isolated from a Pacific red alga. Arch. Microbiol. 196, 745–752. doi: 10.1007/s00203-014-1010-2
Nupur, Bhumika, V., Srinivas, T. N. R., and Kumar, P. A. (2013). Flavobacterium nitratireducens sp. nov., an amylolytic bacterium of the family Flavobacteriaceae isolated from coastal surface seawater. Int. J. Syst. Evol. Microbiol. 63, 2490–2496. doi: 10.1099/ijs.0.046524-0
Oksanen, J., Blanchet, F. G., Kindt, R., Legendre, P., Minchin, P. R., O’Hara, R. B., et al. (2012). vegan: Community Ecology Package. Available online at: https://cran.r-project.org/web/packages/vegan/index.html
Ortiz, M. E., Marco, A., Saiz, N., and Lizana, M. (2004). Impact of ammonium nitrate on growth and survival of six European amphibians. Arch. Environ. Contam. Toxicol. 47, 234–239. doi: 10.1007/s00244-004-2296-x
Ortiz-Santaliestra, M. E., Marco, A., Fernández-Benéitez, M. J., and Lizana, M. (2007). Effects of ammonium nitrate exposure and water acidification on the dwarf newt: the protective effect of oviposition behaviour on embryonic survival. Aquat. Toxicol. 85, 251–257. doi: 10.1016/j.aquatox.2007.09.008
Ortiz-Santaliestra, M. E., and Sparling, D. W. (2007). Alteration of larval development and metamorphosis by nitrate and perchlorate in southern leopard frogs (Rana sphenocephala). Arch. Environ. Contam. Toxicol. 53, 639–646. doi: 10.1007/s00244-006-0277-y
Orton, F., Carr, J. A., and Handy, R. D. (2006). Effects of nitrate and atrazine on larval development and sexual differentiation in the northern leopard frog Rana pipiens. Environ. Toxicol. Chem. 25, 65–71. doi: 10.1897/05-136R.1
Passamaneck, Y. J., and Martindale, M. Q. (2012). Cell proliferation is necessary for the regeneration of oral structures in the anthozoan cnidarian Nematostella vectensis. BMC Dev. Biol. 12:34. doi: 10.1186/1471-213X-12-34
Pérez-Pascual, D., Lunazzi, A., Magdelenat, G., Rouy, Z., Roulet, A., Lopez-Roques, C., et al. (2017). The Complete Genome Sequence of the Fish Pathogen Tenacibaculum maritimum Provides Insights into Virulence Mechanisms. Front. Microbiol. 8:1542. doi: 10.3389/fmicb.2017.01542
Philippat, C., Mortamais, M., Chevrier, C., Petit, C., Calafat, A. M., Ye, X., et al. (2012). Exposure to phthalates and phenols during pregnancy and offspring size at birth. Environ. Health Perspect. 120, 464–470. doi: 10.1289/ehp.1103634
Pita, L., Rix, L., Slaby, B. M., Franke, A., and Hentschel, U. (2018). The sponge holobiont in a changing ocean: from microbes to ecosystems. Microbiome 6:46. doi: 10.1186/s40168-018-0428-1
Qian, L., Liu, J., Lin, Z., Chen, X., Yuan, L., Shen, G., et al. (2020). Evaluation of the spinal effects of phthalates in a zebrafish embryo assay. Chemosphere 249:126144. doi: 10.1016/j.chemosphere.2020.126144
Quast, C., Pruesse, E., Yilmaz, P., Gerken, J., Schweer, T., Yarza, P., et al. (2013). The SILVA ribosomal RNA gene database project: improved data processing and web-based tools. Nucleic Acids Res. 41, D590–D596. doi: 10.1093/nar/gks1219
Reitzel, A. M., Herrera, S., Layden, M. J., Martindale, M. Q., and Shank, T. M. (2013). Going where traditional markers have not gone before: utility of and promise for RAD sequencing in marine invertebrate phylogeography and population genomics. Mol. Ecol. 22, 2953–2970. doi: 10.1111/mec.12228
Rocca, J. D., Simonin, M., Blaszczak, J. R., Ernakovich, J. G., Gibbons, S. M., Midani, F. S., et al. (2019). The Microbiome Stress Project: toward a Global Meta-Analysis of Environmental Stressors and Their Effects on Microbial Communities. Front. Microbiol. 9:3272. doi: 10.3389/fmicb.2018.03272
Rook, G., Bäckhed, F., Levin, B. R., McFall-Ngai, M. J., and Mclean, A. R. (2017). Evolution, human-microbe interactions, and life history plasticity. Lancet 390, 521–530. doi: 10.1016/S0140-6736(17)30566-4
Röttinger, E., Dahlin, P., and Martindale, M. Q. (2012). A framework for the establishment of a cnidarian gene regulatory network for “endomesoderm” specification: the inputs of ß-catenin/TCF signaling. PLoS Genet. 8:e1003164. doi: 10.1371/journal.pgen.1003164
Schaffer, A. A., Bazarsky, M., Levy, K., Chalifa-Caspi, V., and Gat, U. (2016). A transcriptional time-course analysis of oral vs. aboral whole-body regeneration in the Sea anemone Nematostella vectensis. BMC Genomics 17:718. doi: 10.1186/s12864-016-3027-1
Schwaiger, M., Schönauer, A., Rendeiro, A. F., Pribitzer, C., Schauer, A., Gilles, A. F., et al. (2014). Evolutionary conservation of the eumetazoan gene regulatory landscape. Genome Res. 24, 639–650. doi: 10.1101/gr.162529.113
Sebe-Pedros, A., Saudemont, B., Chomsky, E., Plessier, F., Mailhe, M. P., Renno, J., et al. (2018). Cnidarian Cell Type Diversity and Regulation Revealed by Whole-Organism Single-Cell RNA-Seq. Cell 173, 1520–1534.e20. doi: 10.1016/j.cell.2018.05.019
Sehnal, L., Brammer-Robbins, E., Wormington, A. M., Blaha, L., Bisesi, J., Larkin, I., et al. (2021). Microbiome Composition and Function in Aquatic Vertebrates: small Organisms Making Big Impacts on Aquatic Animal Health. Front. Microbiol. 12:567408. doi: 10.3389/fmicb.2021.567408
Sharma, A., Mollier, J., Brocklesby, R. W. K., Caves, C., Jayasena, C. N., and Minhas, S. (2020). Endocrine-disrupting chemicals and male reproductive health. Reprod. Med. Biol. 19, 243–253. doi: 10.1002/rmb2.12326
Steinmetz, P. R. H., Aman, A., Kraus, J. E. M., and Technau, U. (2017). Gut-like ectodermal tissue in a sea anemone challenges germ layer homology. Nat. Ecol. Evol. 1, 1535–1542. doi: 10.1038/s41559-017-0285-5
Szczepanek, S., Cikala, M., and David, C. N. (2002). Poly-gamma-glutamate synthesis during formation of nematocyst capsules in Hydra. J. Cell Sci. 115, 745–751. doi: 10.1242/jcs.115.4.745
Tang, Z. R., Xu, X. L., Deng, S. L., Lian, Z. X., and Yu, K. (2020). Oestrogenic Endocrine Disruptors in the Placenta and the Fetus. Int. J. Mol. Sci. 21:1519. doi: 10.3390/ijms21041519
Tarrant, A. M., Payton, S. L., Reitzel, A. M., Porter, D. T., and Jenny, M. J. (2018). Ultraviolet radiation significantly enhances the molecular response to dispersant and sweet crude oil exposure in Nematostella vectensis. Mar. Environ. Res. 134, 96–108. doi: 10.1016/j.marenvres.2018.01.002
Technau, U. (2020). Gastrulation and germ layer formation in the sea anemone Nematostella vectensis and other cnidarians. Mech. Dev. 163:103628. doi: 10.1016/j.mod.2020.103628
Thompson, J. R., Rivera, H. E., Closek, C. J., and Medina, M. (2014). Microbes in the coral holobiont: partners through evolution, development, and ecological interactions. Front. Cell. Infect. Microbiol. 4:176. doi: 10.3389/fcimb.2014.00176
Trevino, M., Stefanik, D. J., Rodriguez, R., Harmon, S., and Burton, P. M. (2011). Induction of canonical Wnt signaling by alsterpaullone is sufficient for oral tissue fate during regeneration and embryogenesis in Nematostella vectensis. Dev. Dyn. 240, 2673–2679. doi: 10.1002/dvdy.22774
Valiela, I., Lloret, J., Chenoweth, K., Elmstrom, E., and Hanacek, D. (2021). Control of N Concentrations in Cape Cod Estuaries by Nitrogen Loads, Season, and Down-Estuary Transit: assessment by Conventional and Effect-Size Statistics. Estuaries Coasts 44, 1294–1309. doi: 10.1007/s12237-020-00869-z
Vamsee-Krishna, C., and Phale, P. S. (2008). Bacterial degradation of phthalate isomers and their esters. Indian J. Microbiol. 48, 19–34. doi: 10.1007/s12088-008-0003-8
van der Burg, C. A., and Prentis, P. J. (2021). The Tentacular Spectacular: evolution of Regeneration in Sea Anemones. Genes 12:1072. doi: 10.3390/genes12071072
Wang, J.-T., Chou, Y.-J., Chou, J.-H., Chen, C. A., and Chen, W.-M. (2008). Tenacibaculum aiptasiae sp. nov., isolated from a sea anemone Aiptasia pulchella. Int. J. Syst. Evol. Microbiol. 58, 761–766. doi: 10.1099/ijs.0.65437-0
Warner, J. F., Guerlais, V., Amiel, A. R., Johnston, H., Nedoncelle, K., and Rottinger, E. (2018). NvERTx: a gene expression database to compare embryogenesis and regeneration in the sea anemone Nematostella vectensis. Development 145:dev162867. doi: 10.1242/dev.162867
Wickham, H. (2009). ggplot2: Elegant Graphics for Data Analysis. New York, NY: Springer-Verlag New York. Available online at: https://ggplot2-book.org/
Wijesena, N., Simmons, D. K., and Martindale, M. Q. (2017). Antagonistic BMP-cWNT signaling in the cnidarian Nematostella vectensis reveals insight into the evolution of mesoderm. Proc. Natl. Acad. Sci. U. S. A. 114, E5608–E5615. doi: 10.1073/pnas.1701607114
Wikramanayake, A. H., Hong, M., Lee, P. N., Pang, K., Byrum, C. A., Bince, J. M., et al. (2003). An ancient role for nuclear beta-catenin in the evolution of axial polarity and germ layer segregation. Nature 426, 446–450. doi: 10.1038/nature02113
Wolenski, F. S., Bradham, C. A., Finnerty, J. R., and Gilmore, T. D. (2013). NF-κB is required for cnidocyte development in the sea anemone Nematostella vectensis. Dev. Biol. 373, 205–215. doi: 10.1016/j.ydbio.2012.10.004
Zaneveld, J. R., Mcminds, R., and Vega Thurber, R. (2017). Stress and stability: applying the Anna Karenina principle to animal microbiomes. Nat. Microbiol. 2:17121. doi: 10.1038/nmicrobiol.2017.121
Keywords: Nematostella, growth, microbiome, stressors, development
Citation: Klein S, Frazier V, Readdean T, Lucas E, Diaz-Jimenez EP, Sogin M, Ruff ES and Echeverri K (2021) Common Environmental Pollutants Negatively Affect Development and Regeneration in the Sea Anemone Nematostella vectensis Holobiont. Front. Ecol. Evol. 9:786037. doi: 10.3389/fevo.2021.786037
Received: 29 September 2021; Accepted: 15 November 2021;
Published: 23 December 2021.
Edited by:
Igor Schneider, Federal University of Pará, BrazilReviewed by:
Adam Michael Reitzel, The University of North Carolina at Charlotte, United StatesCopyright © 2021 Klein, Frazier, Readdean, Lucas, Diaz-Jimenez, Sogin, Ruff and Echeverri. This is an open-access article distributed under the terms of the Creative Commons Attribution License (CC BY). The use, distribution or reproduction in other forums is permitted, provided the original author(s) and the copyright owner(s) are credited and that the original publication in this journal is cited, in accordance with accepted academic practice. No use, distribution or reproduction is permitted which does not comply with these terms.
*Correspondence: Emil S. Ruff, ZXJ1ZmZAbWJsLmVkdQ==; Karen Echeverri, a2VjaGV2ZXJyaUBtYmwuZWR1
†Present address: Sylvia Klein, University of Minnesota, Minneapolis, MN, United States
Disclaimer: All claims expressed in this article are solely those of the authors and do not necessarily represent those of their affiliated organizations, or those of the publisher, the editors and the reviewers. Any product that may be evaluated in this article or claim that may be made by its manufacturer is not guaranteed or endorsed by the publisher.
Research integrity at Frontiers
Learn more about the work of our research integrity team to safeguard the quality of each article we publish.