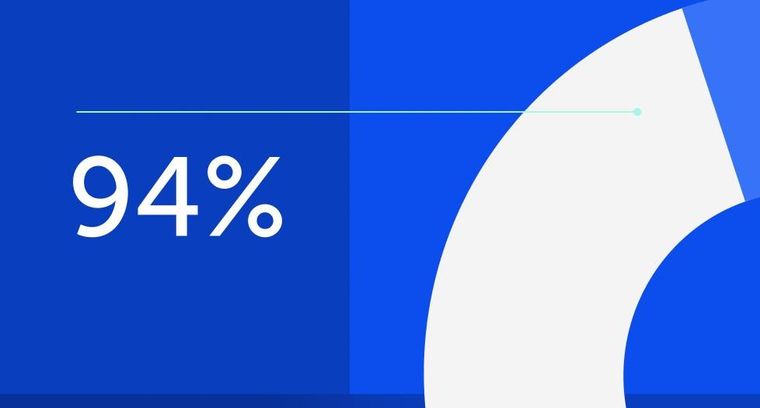
94% of researchers rate our articles as excellent or good
Learn more about the work of our research integrity team to safeguard the quality of each article we publish.
Find out more
ORIGINAL RESEARCH article
Front. Ecol. Evol., 24 December 2021
Sec. Population, Community, and Ecosystem Dynamics
Volume 9 - 2021 | https://doi.org/10.3389/fevo.2021.780706
Species- and population-specific responses to their environment may depend to a large extent on the spatial variation in life-history traits and in demographic processes of local population dynamics. Yet, those parameters and their variability remain largely unknown for many cold-adapted species, which are exposed to particularly rapid rates of environmental change. Here, we compared the demographic traits and dynamics for an emblematic bird species of European mountain ecosystems, the ring ouzel (Turdus torquatus). Using integrated population models fitted in a Bayesian framework, we estimated the survival probability, productivity and immigration of two populations from the Western European Alps, in France (over 11 years) and Switzerland (over 6 years). Juvenile apparent survival was lower and immigration rate higher in the Swiss compared to the French population, with the temporal variation in population growth rate driven by different demographic processes. Yet, when compared to populations in the northwestern part of the range, in Scotland, these two Alpine populations both showed a much lower productivity and higher adult survival, indicating a slower life-history strategy. Our results suggest that demographic characteristics can substantially vary across the discontinuous range of this passerine species, essentially due to contrasted, possibly locally evolved life-history strategies. This study therefore raises the question of whether flexibility in life-history traits is widespread among boreo-alpine species and if it might provide adaptive potential for coping with current environmental change.
The evolution of diversity in life-history strategies is a central topic in evolutionary ecology (Partridge and Harvey, 1988). It has become increasingly important from a conservation biology perspective, as understanding the type and flexibility of life-history strategies contributes to the estimation of the adaptive potential (Forcada et al., 2008), which will be decisive for accurately predicting the responses of biodiversity to environmental change (Reed et al., 2011; Swab et al., 2015). Life-history traits have already been recognized as good indicators of a species’ sensitivity to global change and extinction risk (Jiguet et al., 2007; Pearson et al., 2014). Yet, there is growing evidence that not only interspecific variation, but also intraspecific variation is widespread across a variety of taxonomic groups (Morrison and Hero, 2003; Blanck and Lamouroux, 2007; Nilsen et al., 2009; Albert et al., 2010). The lack of knowledge of demographic characteristics across the distribution range of animal populations thus still limits our ability to assess their vulnerability to global change.
In the Western Palearctic, many taxa present a discontinuous distribution where they occupy mountain ranges at medium to high elevations at lower latitudes (i.e., central and western Europe), as well as taiga and tundra ecosystems at lower elevations at higher latitudes (i.e., Fennoscandia and Northwest Russia), thus covering a large latitudinal and elevational gradient. These species are referred to as boreo-alpine (or arctic-alpine) and are considered to be particularly vulnerable to climate change (Scridel et al., 2018) as the latter disproportionately impacts the aforementioned regions (Ernakovich et al., 2014). Yet, population trends of several boreo-alpine species differ markedly across their range (Lehikoinen et al., 2019) and it remains unclear if this results from contrasting rates of environmental change or from population-specific responses. Indeed, we might expect that intraspecific differences in life-history traits contribute to spatially heterogeneous sensitivity (Jiguet et al., 2007; Swab et al., 2015), which would have important implications for conservation management (Caswell, 2000; Reed et al., 2011; Schwartz et al., 2021). This calls for more local studies to understand which ecological and demographic processes matter at the population level across latitudinal and elevational gradients (Chamberlain et al., 2012; Lehikoinen et al., 2019; Lundblad and Conway, 2020; Schwartz et al., 2021). Finally, this knowledge would help to improve predictions of population resilience or resistance to new environmental circumstances (Pearson et al., 2014; Swab et al., 2015; Boyle et al., 2016).
Birds have historically served as models to understand variation in life-histories (Lack, 1947; Martin, 2004). Indeed, despite a very consistent life cycle among species (Bennett and Owens, 2002), avian life-history strategies cover a large range of the well-known fast-slow continuum, i.e., contrasting long-lived species reproducing slowly to those with short lives and fast reproduction (Sæther and Bakke, 2000; Dobson, 2007). In addition, broad geographical differences in life-history traits have been extensively documented in birds, first along a latitudinal gradient (Lack, 1947), but also in relation to elevational gradients (Cody, 1966). While, on average, faster life-histories are generally reported toward northern latitudes, there is evidence of the opposite pattern toward higher elevations (Hille and Cooper, 2015; Boyle et al., 2016), at least in temperate regions (Balasubramaniam and Rotenberry, 2016). Such shifts have been evidenced both between and within species but are often limited to few life-history traits, such as clutch or egg size (Lack, 1947; Cody, 1966; Balasubramaniam and Rotenberry, 2016). Several authors have stressed that a suite of demographic parameters should be included in intra- and interspecific comparisons across geographical gradients for better appraisals (Bennett and Owens, 2002; Sandercock et al., 2005; Bears et al., 2009; Lundblad and Conway, 2020). Information on the demographic characteristics of bird species living at high elevations or latitudes should thus advance our knowledge of the ultimate and proximate drivers of life-history strategies (Bennett and Owens, 2002; Martin, 2004; Sandercock et al., 2005; Dobson, 2007; Balasubramaniam and Rotenberry, 2016), but research in this area is still excessively scarce (Chamberlain et al., 2012; Scridel et al., 2018).
The development of integrated population models (IPMs) has opened a range of opportunities to better assess the role of demographic processes in population dynamics (Besbeas et al., 2002; Schaub and Abadi, 2011; Schaub and Kéry, 2022). First, IPMs enable the simultaneous use of different types of demographic data in a combined model, leading to more precise estimates of demographic parameters, especially when sample sizes are small (Besbeas et al., 2002; Schaub et al., 2012). Second, a hierarchical formulation enables the separation of sampling from process variance, which translates into more reliable estimates of temporal variation in parameters (Kéry and Schaub, 2012). Third, it is possible to estimate parameters about which explicit information is lacking, such as immigration or productivity (Abadi et al., 2010; Schaub and Fletcher, 2015), albeit this requires some caution (Riecke et al., 2019; Paquet et al., 2021; Schaub and Kéry, 2022). All these advantages can be decisive when relationships between demographic parameters and variation in population growth rate or environmental variables need to be assessed (Besbeas et al., 2002; Schaub and Abadi, 2011).
The present study focuses on the population dynamics of the ring ouzel (Turdus torquatus), a typical boreo-alpine thrush species breeding in mountain and upland ecosystems within the Western Palearctic (Keller et al., 2020). Although globally classified as “least concern” (Birdlife International, 2020), the species is nationally or regionally threatened following recent population declines. To the best of our knowledge, detailed demographic analyses have been performed only for a single declining population in Scotland, where juvenile and adult survival were identified as key parameters in population dynamics (Sim et al., 2011). Hence, the steady decline observed in the United Kingdom is probably caused by particularly low survival probabilities, but ecological drivers are still poorly understood (Sim et al., 2011). The succession of warm and dry summers associated with a decrease in territory occupancy over several sites in northern United Kingdom could negatively impact survival through reduced food availability (Beale et al., 2006).
Here, we used IPMs to describe the local dynamics of two populations of ring ouzels in the French and Swiss Alps. Our aims were (1) to reliably estimate demographic rates, namely adult and juvenile apparent survival, immigration and productivity; (2) to identify key demographic rates contributing to temporal variation in local population growth rates using perturbation analyses; (3) to assess the relationships between annual demographic rates and a set of potentially important weather variables; (4) to compare estimates of demographic rates from these two Alpine populations with those from Scotland, i.e., at the western margin of the species’ range, and discuss any discrepancies in the light of both their contrasting population trends and life-history theory; and (5) to provide a better base for predictions from a biological conservation perspective.
Besides a subspecies occurring in the Middle East (T. t. amicorum in the Caucasus and Turkey), two other subspecies of ring ouzel are distinguished across the distribution range (Keller et al., 2020). T. t. alpestris breeds in central and southern Europe in semi-open forests, mostly within coniferous stands in the subalpine zone (Glutz von Blotzheim and Bauer, 1988). In the Alps, most breeding pairs are single-brooded (65–75%; Glutz von Blotzheim and Bauer, 1988) and annual survival probabilities are unknown. T. t. torquatus occupies more open areas in the British Isles, typically steep slopes of heather moorland, while it prefers semi-open habitats with bushes and shrubs in Fennoscandia (Burfield, 2002). Double brooding is common in the United Kingdom (e.g., 63% in Scotland; Sim et al., 2012), whereas it is apparently rare in Fennoscandia (Burfield, 2002). Demographic parameters from a population in Scotland are presented in Table 1 (Sim et al., 2011). All three subspecies are short-distance migrants, with birds from western Europe sharing overwintering grounds in mountainous areas of southern Spain and North Africa (Glutz von Blotzheim and Bauer, 1988; Sim et al., 2015).
Table 1. Posterior means (with 95% credible intervals) of demographic rates, generation time and results from prospective (elasticities) and retrospective (transient LTRE) perturbation analyses as obtained from integrated population models for two ring ouzel (T. t. alpestris) populations in the Alps (Valais and Vercors).
We focused on two different breeding populations of alpestris ring ouzels in the Alps, one in the Vercors massif (Drôme, France; 44.781 N, 5.564 E) and one in Valais (Switzerland; 46.328 N, 7.428 E). The Vercors population is located at the western periphery of the Alpine range, approximately 225 km southwest of the Valais population, which has a more central position. Study sites cover 90 and 200 ha, respectively. In spite of weakly overlapping elevation ranges [Vercors: 1,560–1,760 m above sea level (m asl), Valais: 1,720–2,120 m asl], habitat configuration is similar in both sites and consists of summer cattle pastures interspersed with coniferous trees (Pinus mugo uncinata in Vercors, Larix decidua and Picea abies in Valais) close to the treeline. Both sites receive similar amounts of precipitation during the core of the breeding season (250–270 mm over April–June).
Three types of data were collected at each study site: capture-mark-recapture (CMR), productivity and population size data. In Vercors, data collection occurred over the period 1999–2009. Birds were captured with mistnets and clap traps and ringed with unique color-ring combinations on a yearly basis from March to July. Adults were defined as birds in their second calendar year or older and sexed based on plumage coloration (total number of ringed individuals: nmale = 94, nfemale = 81). Juveniles were ringed directly at the nest or in its surroundings as fledglings (njuv = 239). Specific sessions to re-sight ringed individuals were performed using binoculars or spotting scopes, with an even effort across the years. Productivity data were collected every year except in 1999, from an annual average of 10.5 nests (total number of nests = 105; range over years 3–24). Each nest was visited at least every sixth day and its content monitored from the ground with a mirror or camera fixed on a perch to determine fledging success. Population surveys were performed each year (except in 1999 and 2000) on a subzone of the study area (32 ha) and consisted of 15 visits in May–June starting at dawn and along a predefined transect, recording all observations of ring ouzels (visual and acoustic) on a map. To estimate the annual number of breeding pairs, the observations were aggregated into territories (assuming that each territory corresponded to a breeding pair) by overlapping the maps of the different visits at the end of the season, following Bibby et al. (2000).
In the Valais study site, the methods to capture and re-sight the birds were identical to those in Vercors (except that single and unique alphanumeric color rings were used instead of color ring combinations) and were applied annually from March to June in the years 2015–2020. While adult birds were captured on a yearly basis (total number of ringed individuals: nmale = 203, nfemale = 107), juveniles were only ringed at the nest in 2017 and 2019 (njuv = 80). Direct information about productivity was thus only available for those 2 years, as well as in 2020 (total number of nests = 45; range over years 12–20). Population surveys were based on the same sampling design as in Vercors, except that it consisted of three visits per year in May–June and the total study area of 200 ha was covered.
We used IPMs to estimate demographic parameters and population size (Besbeas et al., 2002; Schaub and Abadi, 2011). An IPM is based on a joint likelihood that is created by the likelihoods of each contributing dataset (Schaub and Abadi, 2011; Kéry and Schaub, 2012; Schaub and Kéry, 2022), in our case of the population survey, the productivity and the CMR data. We defined a multinomial likelihood to fit the Cormack-Jolly-Seber (CJS) model on the CMR dataset (Lebreton et al., 1992). This model separately estimates apparent survival, here with an age structure, and recapture probability. Juvenile apparent survival (ϕjuv,t) is defined as the probability that a juvenile ringed in year t survives and returns to the study area in year t+1. Apparent adult survival (ϕad,t) is defined similarly, but applies to birds that are at least 1-year old. The recapture probability is defined as the probability of re-sighting a marked individual that is present in the study population. The productivity data was modeled with the Poisson likelihood, but differently for the two populations. For Vercors, we assumed that
where Jt is the total number of fledglings raised in year t in Rt monitored broods and fVE,t is the mean productivity in year t. Due to missing productivity data in the Valais population for half of the study years, we modeled productivity as
where J is the total number of fledglings from R broods monitored over the years and fVA is the mean productivity, which is assumed to be constant over time.
Lastly, the likelihood of the population survey data was formulated as a state-space model (Besbeas et al., 2002), which consists of state and observation processes to disentangle true variations in population size from observation errors (Kéry and Schaub, 2012). The state process corresponds to a pre-breeding census, female-based matrix projection model. For a given year t, we distinguished three types of females, 1-year old birds (N1,t; i.e., females born in the study area in the year t-1 that have survived and are present again in year t), adults (Nad,t; i.e., females that were present in the study area in year t-1 and at least 1-year old, and which have survived and are present again in year t) and immigrants (Nimm,t; i.e., females that were born outside the study area, are at least 1-year old and appear for the first time in the study area in year t). The total number of females in year t+1 (Ntot,t+1) was defined as the sum of the number of females in these three stage classes:
The temporal change in the numbers of females in each stage class was modeled with Poisson and binomial distributions to explicitly include demographic stochasticity:
where ωt is the immigration rate, i.e., the proportion of the number of female immigrants in year t+1 to the total number of females in year t, and ft is the mean productivity at time t (fVE,t and fVA for Vercors and Valais populations, respectively), divided by two as we assumed an even fledgling sex ratio. The observation process, i.e., the relationship between observed (Ct) and true (Ntot,t) population size, was modeled using a Poisson distribution:
Each demographic parameter was modeled with random time effects to estimate an overall mean and temporal variance (Kéry and Schaub, 2012). Preliminary analyses revealed that the model fit was poor for both populations unless the immigration rate (ωt) was assumed constant over the years. Hence, we were not able to estimate temporal variation of immigration. The joint likelihood of the IPM was obtained by the product of all three different likelihoods under the assumption of independence of the datasets. Although this assumption may be violated in our datasets, several studies have shown that this has only a minor influence on parameter estimates (Schaub and Fletcher, 2015; Weegman et al., 2021; Schaub and Kéry, 2022).
All models were fitted in a Bayesian framework, meaning that prior distributions of all unknown parameters had to be defined. We formulated vague priors for all parameters and used the program JAGS (Plummer, 2003) to run our models using package jagsUI (Kellner, 2019) in program R (R Development Core Team, 2021). We ran three chains with 1,100,000 iterations including a 100,000 burn-in and a 10–2 thinning rate. R-hat diagnostics were used to assess convergence (R̂ ≤ 1.01 for all parameters) and we report posterior means with 95% credible intervals (CRI) of the estimated parameters. Regarding the model structure, we fitted time effects on apparent adult and juvenile survival as one goal of the study was the estimation of the temporal variability in demographic rates. Recapture probability was modeled with time effects too, as inter-annual variability in environmental conditions may have led to differences in re-sighting probability, despite the even observation effort. Given the relatively small sample size and the absence of obvious sex-related differences in the species ecology, we did not fit any sex effect on the parameters. To assess the fit of the models, notably to diagnose over- or underdispersion, we computed Bayesian p-values from the posterior predictive distributions of a goodness-of-fit (GOF) statistic for each dataset (see Supplementary Figure 1). The GOF of the CJS model alone was further evaluated by frequentist tests using the R package R2ucare (Gimenez et al., 2018).
To assess the impact of demographic rates on population dynamics, we performed prospective and retrospective perturbation analyses. Prospective analyses address the question of how much would the population growth rate (λ) change if a given demographic rate changes by a small amount (Caswell, 2000). We calculated elasticities as the proportional changes of λ when each demographic rate was changed by the same proportion in turn. Retrospective analyses address the question of how strongly the temporal variability of each demographic rate has contributed to the variability of the population growth rate (Caswell, 2000). We applied transient life table response experiments (LTRE) for this purpose, as they fully account for the interplay between vital rates and population structure (Koons et al., 2017).
In addition to perturbation analyses, we assessed the position of each population on the fast-slow continuum using generation time. We calculated generation time following Gaillard et al. (2005) and assuming constant productivity from onset of reproduction (at age = 1 year). As for demographic rates, all metrics are reported as posterior means along with 95% CRI.
In order to identify possible drivers of annual variation in demographic rates, we examined temporal relationships with environmental factors. We restricted our selection to weather variables collected in the direct surroundings of the study sites during the breeding season. Only few potentially important weather factors were included, based on known important relationships for mountain birds. Ambient temperature and precipitation are key drivers of variation in demographic rates (Novoa et al., 2008; Chiffard et al., 2019; Strinella et al., 2020) and both affect the provisioning activity of the ring ouzel in the Swiss Alps. Indeed, provisioning rates and delivered prey biomass peak in wet and fresh weather conditions, due to the higher availability of earthworms, the staple food source (Barras et al., 2021c). We thus assumed that spring precipitation would have a positive effect, and ambient temperature a negative effect on survival and productivity. In addition, we considered the number of snow free days (defined as snow cover < 10 cm over a given period) as a proxy for the onset of snowmelt, since the latter may play an important role in the breeding ecology of high-elevation bird species (Resano-Mayor et al., 2019). For ring ouzels, snowmelt timing defines a brief peak in food availability that imposes strong temporal constraints on breeding (Barras et al., 2020, 2021c). We hypothesized that in years with an early snowmelt, i.e., a larger number of snow free days, breeding birds could miss this food peak, with detrimental effects on survival and productivity.
In Vercors, data on precipitation (sum over April–June) and number of snow free days (sum over March–April) was provided by the closest meteorological station in Chichilianne (44.812 N, 5.571 E; 1,010 m asl, 3.3 km distance) while temperature information (mean over April–June) was extracted from the Safran surface model (Quintana-Seguí et al., 2008) over the corresponding grid cell (8 km resolution; center 44.748 N, 5.518 E). In Valais, data on precipitation (sum over April–June), ambient temperature (mean over April–June) and number of snow-free days (sum over June–July) were obtained from the nearest meteorological stations for which this data was available (Anzère: 46.305 N, 7.408 E; 1,614 m asl, 3.2 km distance — Montana: 46.299 N, 7.461 E; 1,422 m asl, 4.1 km distance — Donin du Jour: 46.321 N, 7.367 E; 2,390 m asl, 4.1 km distance, respectively). We recorded the number of snow-free days over different months for Vercors and Valais, in order to capture the period of snowmelt, which occurred much later at the high-elevation weather station in Valais compared to Vercors. The snow height threshold at 10 cm was selected as this measure was the most reliable and available from both study sites. Despite the distance and elevation differences between weather stations and study sites, we assume that the annual variations in weather factors were equivalent.
All weather variables were standardized prior to the analyses and they were not correlated (Pearson’s correlation coefficients |r| < 0.7). Fitting the explanatory weather variables directly as temporal covariates in the IPM led to convergence problems in the estimates of survival and productivity (R̂ > 1.1 for some parameters), most probably due to the relatively small sample size. For this reason, we ran bivariate correlation analyses (again using Pearson’s r) between each weather variable and annual estimates of demographic rates from the IPM. We calculated these correlations for each MCMC draw such that the full uncertainty in the parameter estimates was accounted for. This resulted in posterior distributions of the correlation coefficients, so that the probability of coefficients being positive or negative (depending on the sign of r) could be computed.
The GOF tests indicated no lack of fit (Bayesian p = 0.20–0.80; see Supplementary Figure 1) except for the CMR data in the Vercors model (Bayesian p = 0.85). Nevertheless, the more powerful frequentist tests on the CJS models indicated a good fit for either CMR dataset (Vercors: = 26.43, p = 0.70; Valais: = 15.78, p = 0.20). The mean recapture probability was 0.27 (0.14–0.44) in Vercors and 0.44 (0.30–0.62) in Valais; annual estimates are reported in Supplementary Figure 2.
There were apparent differences between the two populations as revealed by the estimates of the demographic parameters, despite their large uncertainties (Table 1). Productivity was higher on average in the Valais (2.80, 2.33–3.31) compared to the Vercors population (1.92, 0.87–3.41). On the contrary, juvenile apparent survival was almost null in Valais (0.003, 0.00–0.02) and clearly lower than in Vercors (0.13, 0.07–0.22). Adult apparent survival was also higher in Vercors than in Valais, but only slightly (0.69, 0.59–0.81 vs. 0.61, 0.39–0.86). The extremely low apparent survival of juveniles in the Valais population means that local recruitment was almost non-existent. Immigration was nearly four times as strong in the Valais (0.43, 0.30–0.57) compared to the Vercors population (0.11, 0.01–0.26). The estimated mean population growth rate indicated a declining population in Vercors [λ = 0.93, 0.87–1.01; p(λ < 1) = 0.95], while the Valais population was increasing [λ = 1.04, 0.98–1.10; p(λ > 1) = 0.91]. Estimated population sizes were close to counts from the surveys (Supplementary Figure 3). Annual estimates of population growth rate, adult survival and juvenile survival showed no clear temporal trend for either population, whereas productivity in Vercors appeared to be declining over the years (Figure 1).
Figure 1. Annual population growth rate, adult and juvenile apparent survival probabilities for two ring ouzel populations in the Alps. Annual productivity parameters were also obtained for the Vercors population, whereas only the average productivity was estimated in the Valais population. Error bars represent 95% credible intervals (CRI).
The calculated elasticities show that the growth rate of both populations is most sensitive to variation in adult survival. Moreover, the Valais population is potentially more impacted by a change in immigration than by the same relative change in local recruitment (in terms of both productivity and juvenile survival), while the opposite was found for the Vercors population (Table 1). Stability of the Vercors population (λ = 1) would necessitate an average increase in either mean adult survival to 0.77 (0.64–0.90), productivity to 3.36 (1.58–6.37), juvenile survival to 0.25 (0.10–0.54) or immigration rate to 0.20 (0.05–0.32). Transient LTRE, i.e., retrospective analyses, revealed that growth rate fluctuations in the Vercors population were primarily due to the variation in productivity, while survival parameters contributed less (Table 1). By contrast, the growth rate of the Valais population was essentially driven by the variation in adult survival (Table 1). Lastly, mean generation time appeared higher in Vercors (4.31, 2.67–7.78) than in Valais (2.68, 1.59–5.53; Table 1).
Best supported correlations were found with parameters that varied most in the population with the longest monitoring period, i.e., in Vercors (Table 2). There was a high posterior probability (p = 0.92) for productivity to be positively correlated with the amount of precipitation, but also with the number of snow free days (p = 0.93). It appears that the extremely low productivity in 2006, a dry year with a late snowmelt, was partly responsible for this pattern, while early snowmelt years resulted in higher productivity overall (Figure 2). We could not assess if these relationships were similar in the Valais population, as annual estimates of productivity were not computed due to missing data for some years. No strong correlation was detected for other parameters, however, most probably due to the low number of annual estimates (Table 2).
Table 2. Mean Pearson’s correlation coefficients r (with 95% credible intervals) between annual demographic rates and weather variables during the breeding season in two different populations of ring ouzels in the Alps, along with the probability of the correlation coefficient to be positive or negative, depending on the sign of r.
Figure 2. Relationships between demographic rates and weather variables for which the probability of a positive correlation coefficient was high (p > 0.90) in the Vercors population. Error bars represent 95% CRI. Given are posterior means, 95% CRI of Pearson’s correlation coefficients (r) and the probability of r being positive.
Intraspecific variation in life history across the distribution range is increasingly documented and can have large implications on population-specific responses to environmental change (Reed et al., 2011). This may apply to many cold-adapted, high-elevation species, given their discontinuous ranges and vulnerability to climate shifts, but demographic information is generally lacking (Chamberlain et al., 2012). Here, we highlight differences between two geographically distinct populations of the ring ouzel in the Alps, in terms of both the mechanisms underpinning their respective trends and in a suite of demographic traits. Compared to a population from the northern part of the range, however, our two study populations evidence characteristics of a slower life-history strategy, with a lower productivity and higher adult survival leading to a longer generation time. This study establishes that demographic characteristics can substantially vary across the range of a boreo-alpine songbird and raises the question of whether flexibility in life-history strategies might provide adaptive potential for coping with current environmental change.
First of all, we would like to stress that several aspects in the structure of the collected data complicate an extensive comparison between populations. On the one hand, given the non-overlapping periods of population monitoring, we cannot rule out that these differences within the distribution range result from period-specific effects rather than a population response to local conditions. On the other hand, the relatively brief time series and thus small sample sizes (1) precluded the estimation of annual estimates for some parameters and led to a high level of uncertainty in others, making comparisons between populations more difficult and (2) hindered fitting environmental variables directly as covariates in the IPM, so that relationships were only explored with bivariate correlations. With these limitations in mind, we discuss and interpret a few pronounced differences in demographic parameters that were highlighted across populations.
Concerning demographic trajectories first, the recent population growth rate in Valais (Switzerland) indicated a stable or even slightly increasing population size, while the Vercors (France) population declined before that, in the first decade of this century. Independent data collected via a “constant effort site” ringing scheme at the same Vercors study site in 2002–2020 (i.e., in the late phase and in prolongation of the period of the present CMR dataset) revealed a linear decrease in the number of annual captures of adult ring ouzels (β = −0.16, p = 0.04; Renous N. and Blache S., unpubl. data). This strongly suggests that the decline continued unabated beyond the last year of systematic CMR data collection at the French site in 2009, and thus during the period of monitoring of the Swiss population (2015–2020). Besides population growth rate, other demographic parameters differed between the two study sites. The Valais population was characterized by a higher immigration rate, lower juvenile apparent survival and, to a lesser extent, lower adult survival than the Vercors population. This indicates frequent permanent immigration and emigration across age classes, which is typical in core populations (Reichert et al., 2021), whereas the higher local recruitment and site fidelity in Vercors suggests a geographically and demographically more isolated population. A lower habitat suitability in Vercors, as typically observed at the distribution margin (Hampe and Petit, 2005), could explain a lower attractivity for immigrants, in addition to the lower average reproductive output achieved by breeding pairs compared to Valais. Yet, estimated immigration rates should be interpreted with great caution. First, the size of a study site directly influences estimates of immigration (a smaller area leads to higher apparent immigration; Millon et al., 2019), although this should render our results even more conservative since the Vercors site is smaller than the Valais one. Another caveat is reduced reliability of immigration estimates from IPMs, in particular of their temporal variation, when not informed directly by data (Riecke et al., 2019; Paquet et al., 2021).
We could detect only weak correlations of demographic rates with weather conditions during reproduction. In the case of Valais, this is inherent to the brevity of the time series, which is also reflected in the high level of uncertainty in the estimates of the correlation coefficients. Furthermore, demographic parameters of altricial bird species inhabiting temperate biomes are often driven by weather circumstances during the non-breeding season (Sæther et al., 2004), with time-lagged effects upon reproduction. We had to restrain our analysis to weather conditions during the breeding season because of limited knowledge about the whereabouts of wintering Alpine ring ouzels. Notwithstanding these issues, some interesting patterns emerged. Firstly, productivity was positively associated with precipitation in Vercors, as expected, which contrasts with the usually negative impact of precipitation on avian productivity (Novoa et al., 2008; Arlettaz et al., 2010). This is likely explained by the reliance of ring ouzel on earthworms for chick provisioning (Barras et al., 2021c), the availability of which increases with rainfall. Secondly, but contrary to our prediction, productivity was enhanced in years with an early onset of the snowmelt. This pattern is known from other mountain birds (Novoa et al., 2008; Saracco et al., 2019), suggesting that some species may cope better with earlier phenological events than when the breeding season is delayed.
Results from the perturbation analyses also highlighted some discrepancies in the contribution of demographic rates to the dynamics of the two populations. Retrospective analyses revealed that productivity contributed most to growth rate annual variation in Vercors, while it was adult survival in Valais. Because productivity in Valais was modeled as a constant due to limited data, we call for caution when interpreting these results. It is anyway very unlikely that productivity impacts population growth rate when one considers the extremely low local juvenile apparent survival in Valais, given that the number of juvenile recruits depends on both parameters (as the product of productivity and apparent survival). In the end, the most prominent drivers of dynamics were those showing marked temporal variability in both populations (Figure 1), in line with numerous other demographic studies (Caswell, 2000; Sæther and Bakke, 2000). However, the demographic parameters that are considered as important in retrospective analyses usually differ from those evidenced in prospective analyses. This is due to a process called “demographic buffering,” where natural selection reduces variation in demographic traits exhibiting a high elasticity (i.e., those contributing much to population growth rate), because variation of the latter has negative fitness consequences (Pfister, 1998; Sæther and Bakke, 2000; Hilde et al., 2020). This was not obvious in our results since adult survival was important according to both prospective and retrospective appraisals. Yet, the short time series covered by our datasets and other confounding effects certainly question our ability to evidence such a pattern (see Hilde et al., 2020). All in all, this suggests that the Valais population would be particularly vulnerable to variations in adult survival, while the Vercors population decline probably results from a series of years with low productivity.
To our knowledge, estimates of a suite of demographic rates exist for only one other ring ouzel population, which is located in Scotland (Sim et al., 2011). Scottish birds (subspecies torquatus) appear to have a fairly different life-history strategy than Alpine birds. Compared to Vercors and Valais populations, they exhibit a higher productivity but lower adult annual survival (Sim et al., 2011), resulting in a shorter generation time, i.e., ranking lower on the fast-slow continuum (Table 1). This difference in productivity between the British uplands and the Alps has most likely its source in the number of broods achieved per reproductive season. While double brooding has never been observed in our two focal Alpine populations, it concerns more than half of the breeding females in the Scottish highlands, with even triple-brooding observed in rare cases (Sim et al., 2012). Yet, an increased reproductive effort probably entails higher intrinsic costs (Bennett and Owens, 2002; Martin, 2004; Dobson, 2007) that are paid back in the form of reduced adult survival in Scottish ring ouzels. Single brooding in the Alps is probably imposed by the shorter time window with suitable breeding conditions at higher elevations, mediated through prey availability (Boyle et al., 2016; Lundblad and Conway, 2020). In effect, the deep snowpack in the Alps impedes any access to the traditional foraging grounds when birds return from their winter quarters (Barras et al., 2021b), whilst snow is most of the time absent at the same period in the British uplands. Later in the season, the suitability of the Alpine foraging habitat and thereby prey availability decreases rapidly (Barras et al., 2020). Other factors could also play a role, such as a greater predation pressure on ground-nesting females in British uplands, which might select for higher productivity (Sandercock et al., 2005; Boyle et al., 2016), or the slightly longer migration route of northern populations to non-breeding grounds, which could result in lower survival (Martin, 2004; Sim et al., 2011).
Regardless of the underlying mechanism, these apparent differences in life-history strategies across the distribution range of the ring ouzel are in line with intraspecific differences evidenced across a wide range of taxa along eco-geographical gradients (Morrison and Hero, 2003; Martin, 2004; Blanck and Lamouroux, 2007; Nilsen et al., 2009; Boyle et al., 2016). In birds, the populations that inhabit high latitudes usually show higher fecundity and lower adult survival than those closer to the tropics (Lack, 1947; Martin, 2004), which is what we report for the ring ouzel. Second, bird populations at higher elevations tend to adopt slower life-history strategies (Sandercock et al., 2005; Hille and Cooper, 2015; Boyle et al., 2016), which is also corroborated by the present results. In effect, our two Alpine populations occur at much higher elevations (1,650–2,100 m asl) than the Scottish population (350–850 m asl). Third, the geographic position of a population within its species range can also affect life-history traits: populations occurring at range margins should theoretically exhibit a slower pace of life than those in the core zones (Hampe and Petit, 2005; Canonne et al., 2020). The difference reported here between Scotland and the Alps does not support this third theoretical prediction, but the latter could still explain the differences between Vercors, which is peripheral, and Valais, which is more central.
Our study highlights different demographic trends across the range of the ring ouzel: population declines at the range margin (Vercors and Scotland) and stable or slightly increasing numbers closer to the core of its range in the Alps (Valais). This view is corroborated by recent observations and predictions of the species’ demographic trajectories across the Swiss mountain massifs, where peripheral populations suffer more than central ones (Knaus et al., 2018; Barras et al., 2021a). Our findings of a very low local juvenile recruitment and a rather high immigration rate in the Valais population might indicate that we are in the presence of a complex metapopulation system, with numerous exchanges of individuals between subpopulations. In this case, conservation action should be envisioned at a larger spatial scale than the population under study (Schaub et al., 2012; Schaub and Ullrich, 2021). A second series of lessons can be drawn from the local demographic population specificities. Low productivity is driving the negative demographic trajectory of the French population (this study), whereas reduced survival was identified as the reason for the decline in the United Kingdom (Sim et al., 2011). Local conservation interventions should thus focus on measures contributing to an amelioration of these respective demographic traits (e.g., habitat restoration to enhance reproductive output), in addition to keeping an eye on the underlying metapopulational issue.
As an emblematic mountain species with a typical, complex boreo-alpine distribution, the ring ouzel shows a pronounced geographic variation in the realization of its life-history tactics. This may indicate a high level of intrinsic potential for adjustments to environmental change in this species (Forcada et al., 2008; Reed et al., 2011). For instance, an increase in the duration of the available temporal window for breeding, notably following modifications of snowfall regimes and an earlier snowmelt in spring at high elevations (Saracco et al., 2019), could provide room for adapting to even fairly rapid changes in environmental conditions. The question thus remains whether a similar variability is shared by other cold-adapted species (Morrison and Hero, 2003; Forcada et al., 2008) and whether it may suffice to achieve viable life history trade-offs in response to the novel challenges that future environments will impose on biodiversity (Albert et al., 2010; Reed et al., 2011). What is certain is that predictive models of species’ future distribution ranges would certainly gain in accuracy by accounting for this variability in life-history traits.
The datasets presented in this study can be found in online repositories. The names of the repository/repositories and accession number(s) can be found below: https://doi.org/10.6084/m9.figshare.14724531.v1.
The animal study was reviewed and approved by the responsible Cantonal Ethics Committee for animal experimentation.
AB and SB collected the data. AB analyzed the data and led the writing of the manuscript. All authors conceived the ideas, designed the methodology, contributed critically to the drafts, performed thorough editing, and gave final approval for publication.
The authors declare that the research was conducted in the absence of any commercial or financial relationships that could be construed as a potential conflict of interest.
All claims expressed in this article are solely those of the authors and do not necessarily represent those of their affiliated organizations, or those of the publisher, the editors and the reviewers. Any product that may be evaluated in this article, or claim that may be made by its manufacturer, is not guaranteed or endorsed by the publisher.
We would like to thank I. Candolfi, A. Fenestraz, C. Jourdan, S. Marti, J. Resano-Mayor, N. Renous, Y. Rime, S. Vignali, and many others for their help in carrying out fieldwork activities in the Swiss and French sites. We would also like to thank the nature reserve “Hauts Plateaux du Vercors” and its rangers for initiating the ringing project in Vercors and providing financial and logistic support. Moreover, we are grateful to the CRBPO of the National Museum of Natural History, in particular F. Jiguet and R. Julliard, for granting the research project in Vercors, to Météo-France and MeteoSwiss for access to weather data, and to E. Ritschard for drawing the illustration in Figure 1. We would also further like to thank the reviewers who provided valuable comments on previous versions of this manuscript.
The Supplementary Material for this article can be found online at: https://www.frontiersin.org/articles/10.3389/fevo.2021.780706/full#supplementary-material
Abadi, F., Gimenez, O., Ullrich, B., Arlettaz, R., and Schaub, M. (2010). Estimation of immigration rate using integrated population models. J. Appl. Ecol. 47, 393–400. doi: 10.1111/j.1365-2664.2010.01789.x
Albert, C. H., Thuiller, W., Yoccoz, N. G., Soudant, A., Boucher, F., Saccone, P., et al. (2010). Intraspecific functional variability: extent, structure and sources of variation. J. Ecol. 98, 604–613. doi: 10.1111/j.1365-2745.2010.01651.x
Arlettaz, R., Schaad, M., Reichlin, T. S., and Schaub, M. (2010). Impact of weather and climate variation on hoopoe reproductive ecology and population growth. J. Ornithol. 151, 889–899. doi: 10.1007/s10336-010-0527-7
Balasubramaniam, P., and Rotenberry, J. T. (2016). Elevation and latitude interact to drive life-history variation in precocial birds: a comparative analysis using galliformes. J. Anim. Ecol. 85, 1528–1539. doi: 10.1111/1365-2656.12570
Barras, A. G., Marti, S., Ettlin, S., Vignali, S., Resano-Mayor, J., Braunisch, V., et al. (2020). The importance of seasonal environmental factors in the foraging habitat selection of Alpine Ring Ouzels Turdus torquatus alpestris. Ibis 162, 505–519. doi: 10.1111/ibi.12764
Barras, A. G., Braunisch, V., and Arlettaz, R. (2021a). Predictive models of distribution and abundance of a threatened mountain species show that impacts of climate change overrule those of land use change. Divers. Distrib/ 27, 989–1004. doi: 10.1111/ddi.13247
Barras, A. G., Liechti, F., and Arlettaz, R. (2021b). Seasonal and daily movement patterns of an alpine passerine suggest high flexibility in relation to environmental conditions. J. Avian Biol. e02860. doi: 10.1111/jav.02860
Barras, A. G., Niffenegger, C. A., Candolfi, I., Hunziker, Y. A., and Arlettaz, R. (2021c). Nestling diet and parental food provisioning in a declining mountain passerine reveal high sensitivity to climate change. J. Avian Biol. 52:e02649. doi: 10.1111/jav.02649
Bastianelli, G., Tavecchia, G., Meléndez, L., Seoane, J., Obeso, J. R., and Laiolo, P. (2017). Surviving at high elevations: an inter- and intra-specific analysis in a mountain bird community. Oecologia 184, 293–303. doi: 10.1007/s00442-017-3852-1
Beale, C. M., Burfield, I. J., Sim, I. M. W., Rebecca, G. W., Pearce-Higgins, J. W., and Grant, M. C. (2006). Climate change may account for the decline in British ring ouzels Turdus torquatus. J. Anim. Ecol. 75, 826–835. doi: 10.1111/j.1365-2656.2006.01102.x
Bears, H., Martin, K., and White, G. C. (2009). Breeding in high-elevation habitat results in shift to slower life-history strategy within a single species. J. Anim. Ecol. 78, 365–375. doi: 10.1111/j.1365-2656.2008.01491.x
Bennett, P. M., and Owens, I. P. F. (2002). Evolutionary Ecology of Birds: Life Histories, Mating Systems, and Extinction. New York, NY: Oxford University Press.
Besbeas, P., Freeman, S. N., Morgan, B. J. T., and Catchpole, E. A. (2002). Integrating mark–recapture–recovery and census data to estimate animal abundance and demographic parameters. Biometrics 58, 540–547. doi: 10.1111/j.0006-341X.2002.00540.x
Bibby, C. J., Burgess, N. D., Hill, D. A., and Mustoe, S. (2000). Bird Census Techniques. London: Academic Press.
Birdlife International (2020). Species Factsheet: Turdus torquatus. Cambridge: Birdlife International.
Blanck, A., and Lamouroux, N. (2007). Large-scale intraspecific variation in life-history traits of European freshwater fish. J. Biogeogr. 34, 862–875. doi: 10.1111/j.1365-2699.2006.01654.x
Boyle, A. W., Sandercock, B. K., and Martin, K. (2016). Patterns and drivers of intraspecific variation in avian life history along elevational gradients: a meta-analysis. Biol. Rev. 91, 469–482. doi: 10.1111/brv.12180
Burfield, I. J. (2002). The Breeding Ecology and Conservation of the Ring Ouzel Turdus torquatus in Britain. Ph.D. dissertation. Cambridge: University of Cambridge.
Canonne, C., Novoa, C., Muffat-Joly, B., Resseguier, J., Desmet, J.-F., Casadesus, J. B., et al. (2020). Life on the edge: common slow pace of life but contrasted trajectories of alpine rock ptarmigan populations at their southern margin. Wildlife Biol. 2020:wlb.00628. doi: 10.2981/wlb.00628
Caswell, H. (2000). Prospective and retrospective perturbation analyses: their roles in conservation biology. Ecology 81, 619–627. doi: 10.2307/177364
Chamberlain, D., Arlettaz, R., Caprio, E., Maggini, R., Pedrini, P., Rolando, A., et al. (2012). The altitudinal frontier in avian climate impact research. Ibis 154, 205–209. doi: 10.1111/j.1474-919X.2011.01196.x
Chiffard, J., Delestrade, A., Yoccoz, N. G., Loison, A., and Besnard, A. (2019). Warm temperatures during cold season can negatively affect adult survival in an alpine bird. Ecol. Evol. 9, 12531–12543. doi: 10.1002/ece3.5715
Dobson, F. S. (2007). A lifestyle view of life-history evolution. Proc. Natl. Acad.Sci. U.S.A. 104, 17565–17566. doi: 10.1073/pnas.0708868104
Ernakovich, J. G., Hopping, K. A., Berdanier, A. B., Simpson, R. T., Kachergis, E. J., Steltzer, H., et al. (2014). Predicted responses of arctic and alpine ecosystems to altered seasonality under climate change. Glob. Chang. Biol. 20, 3256–3269. doi: 10.1111/gcb.12568
Forcada, J., Trathan, P. N., and Murphy, E. J. (2008). Life history buffering in Antarctic mammals and birds against changing patterns of climate and environmental variation. Glob. Chang. Biol. 14, 2473–2488. doi: 10.1111/j.1365-2486.2008.01678.x
Gaillard, J. M., Yoccoz, N. G., Lebreton, J.-D., Bonenfant, C., Devillard, S., Loison, A., et al. (2005). Generation time: a reliable metric to measure life-history variation among mammalian populations. Am. Natural. 166, 119–123. doi: 10.1086/430330
Gimenez, O., Lebreton, J.-D., Choquet, R., and Pradel, R. (2018). R2ucare: an r package to perform goodness-of-fit tests for capture–recapture models. Methods Ecol. Evol. 9, 1749–1754. doi: 10.1111/2041-210x.13014
Glutz von Blotzheim, U. N., and Bauer, K. M. (1988). “Turdus torquatus Linnaeus 1758 – Ringdrossel, Ringamsel,” in Handbuch der Vögel Mitteleuropas, ed. U. N. Glutz Von Blotzheim (Wiesbaden: AULA-Verlag), 801–838.
Hampe, A., and Petit, R. J. (2005). Conserving biodiversity under climate change: the rear edge matters. Ecol. Lett. 8, 461–467. doi: 10.1111/j.1461-0248.2005.00739.x
Hilde, C. H., Gamelon, M., Sæther, B. E., Gaillard, J. M., Yoccoz, N. G., and Pélabon, C. (2020). The demographic buffering hypothesis: evidence and challenges. Trends Ecol. Evol. 35, 523–538. doi: 10.1016/j.tree.2020.02.004
Hille, S. M., and Cooper, C. B. (2015). Elevational trends in life histories: revising the pace-of-life framework. Biol. Rev. 90, 204–213. doi: 10.1111/brv.12106
Jiguet, F., Gadot, A.-S., Julliard, R., Newson, S. E., and Couvet, D. (2007). Climate envelope, life history traits and the resilience of birds facing global change. Glob. Chang. Biol. 13, 1672–1684. doi: 10.1111/j.1365-2486.2007.01386.x
Keller, V., Herrando, S., Vorisek, P., Franch, M., Kipson, M., Milanesi, P., et al. (2020). European Breeding Bird Atlas 2: Distribution, Abundance and Change. Barcelona: European Bird Census Council & Lynx Edicions.
Kellner, K. (2019). jagsUI: A wrapper around ‘rjags’ to streamline ‘JAGS’ analyses. ver. 1.5.1. Available online at: https://CRAN.R-project.org/package=jagsUI (accessed June 18, 2021).
Kéry, M., and Schaub, M. (2012). Bayesian Population Analysis Using WinBUGS: A Hierarchical Perspective. Waltham, MA: Academic Press.
Knaus, P., Antoniazza, S., Wechsler, S., Guélat, J., Kéry, M., Strebel, N., et al. (2018). Swiss Breeding Bird Atlas 2013-2016. Distribution and Population Trends of Birds in Switzerland and Liechtenstein. Sempach: Swiss Ornithological Institute.
Koons, D. N., Arnold, T. W., and Schaub, M. (2017). Understanding the demographic drivers of realized population growth rates. Ecol. Appl. 27, 2102–2115. doi: 10.1002/eap.1594
Lack, D. (1947). The significance of clutch-size. Ibis 89, 302–352. doi: 10.1111/j.1474-919X.1947.tb04155.x
Lebreton, J.-D., Burnham, K. P., Clobert, J., and Anderson, D. R. (1992). Modeling survival and testing biological hypotheses using marked animals: a unified approach with case studies. Ecol. Monogr. 62, 67–118. doi: 10.2307/2937171
Lehikoinen, A., Brotons, L., Calladine, J., Campedelli, T., Escandell, V., Flousek, J., et al. (2019). Declining population trends of European mountain birds. Glob. Chang. Biol. 25, 577–588. doi: 10.1111/gcb.14522
Lundblad, C. G., and Conway, C. J. (2020). Variation in selective regimes drives intraspecific variation in life-history traits and migratory behaviour along an elevational gradient. J. Anim. Ecol. 89, 397–411. doi: 10.1111/1365-2656.13134
Martin, T. E. (2004). Avian life-history evolution has an eminent past: does it have a bright future? Auk 121, 289–301. doi: 10.1093/auk/121.2.289
Masoero, G., Maurino, L., Rolando, A., and Chamberlain, D. (2016). The effect of treeline proximity on predation pressure: an experiment with artificial nests along elevational gradients in the European Alps. Bird Study 63, 395–405. doi: 10.1080/00063657.2016.1214106
Millon, A., Lambin, X., Devillard, S., and Schaub, M. (2019). Quantifying the contribution of immigration to population dynamics: a review of methods, evidence and perspectives in birds and mammals. Biol. Rev. 94, 2049–2067. doi: 10.1111/brv.12549
Morrison, C., and Hero, J.-M. (2003). Geographic variation in life-history characteristics of amphibians: a review. J. Anim. Ecol. 72, 270–279. doi: 10.1046/j.1365-2656.2003.00696.x
Nilsen, E. B., Gaillard, J.-M., Andersen, R., Odden, J., Delorme, D., Van Laere, G., et al. (2009). A slow life in hell or a fast life in heaven: demographic analyses of contrasting roe deer populations. J. Anim. Ecol. 78, 585–594. doi: 10.1111/j.1365-2656.2009.01523.x
Novoa, C., Besnard, A., Brenot, J.-F., and Ellison, L. N. (2008). Effect of weather on the reproductive rate of Rock Ptarmigan Lagopus muta in the eastern Pyrenees. Ibis 150, 270–278. doi: 10.1111/j.1474-919X.2007.00771.x
Paquet, M., Knape, J., Arlt, D., Forslund, P., Pärt, T., Flagstad, Ø, et al. (2021). Integrated population models poorly estimate the demographic contribution of immigration. Methods Ecol. Evol. 12, 1899–1910. doi: 10.1111/2041-210X.13667
Partridge, L., and Harvey, P. H. (1988). The ecological context of life history evolution. Science 241, 1449–1455. doi: 10.1126/science.241.4872.1449
Pearson, R. G., Stanton, J. C., Shoemaker, K. T., Aiello-Lammens, M. E., Ersts, P. J., Horning, N., et al. (2014). Life history and spatial traits predict extinction risk due to climate change. Nat. Clim. Chang. 4, 217–221. doi: 10.1038/nclimate2113
Pfister, C. A. (1998). Patterns of variance in stage-structured populations: evolutionary predictions and ecological implications. Proc. Nal. Acad. Sci. U.S.A. 95, 213–218. doi: 10.1073/pnas.95.1.213
Plummer, M. (2003). “JAGS: a program for analysis of Bayesian graphical models using Gibbs sampling,” in Proceedings of the 3rd International Workshop on Distributed Statistical Computing (DSC). Vienna. 1–10.
Quintana-Seguí, P., Le Moigne, P., Durand, Y., Martin, E., Habets, F., Baillon, M., et al. (2008). Analysis of near-surface atmospheric variables: validation of the SAFRAN analysis over france. J. Appl. Meteorol. Climatol. 47, 92–107. doi: 10.1175/2007jamc1636.1
R Development Core Team (2021). R: A Language and Environment for Statistical Computing. ver. 4.0.4. Vienna: R Foundation for Statistical Computing.
Reed, T. E., Schindler, D. E., and Waples, R. S. (2011). Interacting effects of phenotypic plasticity and evolution on population persistence in a changing climate. Conserv. Biol. 25, 56–63. doi: 10.1111/j.1523-1739.2010.01552.x
Reichert, B. E., Fletcher, R. J. J., and Kitchens, W. M. (2021). The demographic contributions of connectivity versus local dynamics to population growth of an endangered bird. J. Anim. Ecol. 90, 574–584. doi: 10.1111/1365-2656.13387
Resano-Mayor, J., Korner-Nievergelt, F., Vignali, S., Horrenberger, N., Barras, A. G., Braunisch, V., et al. (2019). Snow cover phenology is the main driver of foraging habitat selection for a high-alpine passerine during breeding: implications for species persistence in the face of climate change. Biodivers. Conserv. 28, 2669–2685. doi: 10.1007/s10531-019-01786-9
Riecke, T. V., Williams, P. J., Behnke, T. L., Gibson, D., Leach, A. G., Sedinger, B. S., et al. (2019). Integrated population models: model assumptions and inference. Methods Ecol. Evol. 10, 1072–1082. doi: 10.1111/2041-210X.13195
Sandercock, B. K., Martin, K., and Hannon, S. J. (2005). Life history strategies in extreme environments: comparative demography of arctic and alpine ptarmigan. Ecology 86, 2176–2186. doi: 10.1890/04-0563
Saracco, J. F., Siegel, R. B., Helton, L., Stock, S. L., and Desante, D. F. (2019). Phenology and productivity in a montane bird assemblage: trends and responses to elevation and climate variation. Glob. Chang. Biol. 25, 985–996. doi: 10.1111/gcb.14538
Sæther, B.-E., and Bakke, Ø (2000). Avian life history variation and contribution of demographic traits to the population growth rate. Ecology 81, 642–653.
Sæther, B.-E., Sutherland, W. J., and Engen, S. (2004). Climate influences on avian population dynamics. Adv. Ecol. Res. 35, 185–209. doi: 10.1016/S0065-2504(04)35009-9
Schaub, M., and Abadi, F. (2011). Integrated population models: a novel analysis framework for deeper insights into population dynamics. J. Ornithol. 152, 227–237. doi: 10.1007/s10336-010-0632-7
Schaub, M., and Fletcher, D. (2015). Estimating immigration using a Bayesian integrated population model: choice of parametrization and priors. Environ. Ecol. Stat. 22, 535–549. doi: 10.1007/s10651-015-0309-8
Schaub, M., and Kéry, M. (2022). Integrated Population Models: Theory and Ecological Applications With R and JAGS. Cambridge, MA: Academic Press.
Schaub, M., Reichlin, T. S., Abadi, F., Kéry, M., Jenni, L., and Arlettaz, R. (2012). The demographic drivers of local population dynamics in two rare migratory birds. Oecologia 168, 97–108. doi: 10.1007/s00442-011-2070-5
Schaub, M., and Ullrich, B. (2021). A drop in immigration results in the extinction of a local woodchat shrike population. Anim. Conserv. 24, 335–345. doi: 10.1111/acv.12639
Schwartz, T., Besnard, A., Avilés, J. M., Catry, T., Górski, A., Kiss, O., et al. (2021). Geographical variation in pace-of-life in a long-distance migratory bird: implications for population management. Oecologia 197, 167–178. doi: 10.1007/s00442-021-05012-8
Scridel, D., Brambilla, M., Martin, K., Lehikoinen, A., Iemma, A., Matteo, A., et al. (2018). A review and meta-analysis of the effects of climate change on Holarctic mountain and upland bird populations. Ibis 160, 489–515. doi: 10.1111/ibi.12585
Sim, I. M., Rebecca, G. W., Ludwig, S. C., Grant, M. C., and Reid, J. M. (2011). Characterizing demographic variation and contributions to population growth rate in a declining population. J. Anim. Ecol. 80, 159–170. doi: 10.1111/j.1365-2656.2010.01750.x
Sim, I. M. W., Green, M., Rebecca, G. W., and Burgess, M. D. (2015). Geolocators reveal new insights into Ring Ouzel Turdus torquatus migration routes and non-breeding areas. Bird Study 62, 561–565. doi: 10.1080/00063657.2015.1077779
Sim, I. M. W., Rebecca, G. W., and Wilkinson, N. I. (2012). Frequency of multiple brooding in Ring Ouzels, including first documented cases of triple brooding. Bird Study 59, 358–362. doi: 10.1080/00063657.2012.707637
Strinella, E., Scridel, D., Brambilla, M., Schano, C., and Korner-Nievergelt, F. (2020). Potential sex-dependent effects of weather on apparent survival of a high-elevation specialist. Sci. Rep. 10:8386. doi: 10.1038/s41598-020-65017-w
Swab, R. M., Regan, H. M., Matthies, D., Becker, U., and Bruun, H. H. (2015). The role of demography, intra-species variation, and species distribution models in species’ projections under climate change. Ecography 38, 221–230. doi: 10.1111/ecog.00585
Keywords: conservation, immigration, integrated population model, intraspecific variation, ring ouzel
Citation: Barras AG, Blache S, Schaub M and Arlettaz R (2021) Variation in Demography and Life-History Strategies Across the Range of a Declining Mountain Bird Species. Front. Ecol. Evol. 9:780706. doi: 10.3389/fevo.2021.780706
Received: 21 September 2021; Accepted: 25 November 2021;
Published: 24 December 2021.
Edited by:
Mauricio Lima, Pontificia Universidad Católica de Chile, ChileReviewed by:
Morten Frederiksen, Aarhus University, DenmarkCopyright © 2021 Barras, Blache, Schaub and Arlettaz. This is an open-access article distributed under the terms of the Creative Commons Attribution License (CC BY). The use, distribution or reproduction in other forums is permitted, provided the original author(s) and the copyright owner(s) are credited and that the original publication in this journal is cited, in accordance with accepted academic practice. No use, distribution or reproduction is permitted which does not comply with these terms.
*Correspondence: Arnaud G. Barras, YXJuYXVkLmJhcnJhc0B2b2dlbHdhcnRlLmNo
Disclaimer: All claims expressed in this article are solely those of the authors and do not necessarily represent those of their affiliated organizations, or those of the publisher, the editors and the reviewers. Any product that may be evaluated in this article or claim that may be made by its manufacturer is not guaranteed or endorsed by the publisher.
Research integrity at Frontiers
Learn more about the work of our research integrity team to safeguard the quality of each article we publish.