- 1Department of Animal and Biomedical Sciences, School of Life Sciences, Lanzhou University, Lanzhou, China
- 2State Key Laboratory of Grassland and Agro-Ecosystems, School of Life Sciences, Lanzhou University, Lanzhou, China
Investigating how highland amphibians respond to changes in ambient temperature may be of great significance for their fate prediction and effective conservation in the background of global warming. Here, using field individuals as the control group, we investigated the influence of high temperatures (20.5 and 25.5°C) and heat wave (15–26.6°C) on the thermal preference, critical thermal limits, locomotor performance, oxidative stress, and antioxidant enzyme activities in high-altitude frog Nanorana pleskei (3,490 m) endemic to the Qinghai-Tibet Plateau (QTP). After 2 weeks of acclimation to high temperatures and heat wave, the thermal preference (Tpref), critical thermal maximum (CTmax), and range of tolerable temperature significantly increased, while the critical thermal minimum (CTmin) was significantly decreased. The total time of jump to exhaustion significantly decreased, and burst swimming speed significantly increased in frogs acclimated in the high temperature and heat wave groups compared with the field group. In the high temperature group, the level of H2O2 and lipid peroxide (malondialdehyde, MDA), as well as the activities of glutathione peroxidase (GPX), glutathione reductase (GR), catalase (CAT), superoxide dismutase (SOD), and total antioxidant capacity (T-AOC) significantly increased in the liver or muscle. However, in the heat wave group, the MDA content significantly decreased in the liver, and antioxidants activities decreased in the liver and muscle except for CAT activities that were significantly increased in the liver. These results indicated that N. pleskei could respond to the oxidative stress caused by high temperatures by enhancing the activity of antioxidant enzymes. The heat wave did not appear to cause oxidative damage in N. pleskei, which may be attributed to the fact that they have successfully adapted to the dramatic temperature fluctuations on the QTP.
Introduction
In the background of global warming, the annual average air temperature in Qinghai Tibet Plateau (QTP) is increasing, with increase in frequency and intensity of extreme temperature events (Stocker et al., 2014; Zhao et al., 2014; Yi et al., 2018). Poikilothermic vertebrates, such as amphibians and non-avian reptiles, although have behavioral and weak physiological thermoregulatory mechanisms, their growth, reproduction, foraging, immunity, and competitiveness are easily affected by environmental temperatures (Panov and Mcqueen, 1998; Cherkasov et al., 2006; Pörtner and Farrell, 2008; Peng et al., 2020). Since 1980, 122 species have gone extinct, and more than 32% of amphibian species are vulnerable, endangered, or critically endangered because of climate change, habitat loss and so on (Collins and Storfer, 2003; Stuart et al., 2004; Mendelson et al., 2006; Wake and Vredenburg, 2008). Han et al. (2016) have demonstrated that temperature significantly affects the daily food intake, sprint speed, and length of continuous locomotion in Qinghai Plateau toad-headed lizard Phrynocephalus vlangalii. Peng et al. (2020) found that high temperature accelerates the development of tadpoles and reduces their body size during metamorphosis in Tibetan brown frog Rana kukunoris. The body mass of the corn snake Pantherophis guttatus is decreased significantly in a simulated heat wave (Stahlschmidt et al., 2017). Therefore, understanding how highland amphibians respond to climate warming and heat wave events may have great significance for their effective conservation.
The thermal biology of ectotherms comprise preferred body temperature (Tpref) and thermal tolerance (critical thermal maximum CTmax, and critical thermal minimum, CTmin) (Angilletta et al., 2010b). The Tpref, CTmax, and CTmin of ectotherms are affected by many factors, such as thermal acclimation trials (Avalos et al., 2020), light cycle (Lapwong et al., 2020), and ontogeny (Wollmuth et al., 1987). For example, Tpref is higher in the warm/wet season than in the cold/dry season in the terrestrial toad Rhinella icterica (César et al., 2018). Furthermore, the common coqui frog Eleutherodactylus coqui, acclimated to a cold temperature (16°C, 30 days), has CTmin lower than that of those acclimated to a high temperature (25°C) (Haggerty, 2016). The plasticity of thermal biology of amphibians can help them to adapt to the warming ecological environment and meet the needs of different physiology (Hernandez-Sandoval et al., 2018). The locomotion performance of individuals largely determine their adaptability, such as foraging, reproduction, and avoiding predators (Clusella-Trullas et al., 2010), while the locomotion performance of ectotherms is closely related to ambient temperature (Chown and Nicolson, 2004; Stillwell and Fox, 2005). The individual locomotion ability gradually decreases once the ambient temperature goes beyond optimal temperature range (Hertz et al., 1983; Bennett, 1990). However, some locomotion properties, i.e., stamina and burst performance, show plasticity after acclimation to different temperatures (Clusella-Trullas et al., 2010). For instance, the tropical clawed frog Xenopus tropicalis, acclimated to lower temperature (24°C, 2 months), has stronger stamina than individuals acclimated to high temperature (29°C, 2 months) (Padilla et al., 2019). The soft-shelled turtle Pelodiscus sinensis, acclimated to higher temperature (30°C, 4 weeks), has faster swimming speed than those acclimated to a low temperature (10 and 20°C, 4 weeks) (Wu et al., 2013). Nevertheless, there are limited studies that reported whether high-altitude amphibians also exhibit plasticity in thermal biology and locomotion performance after acclimation to high temperatures and heat wave.
The oxidative stress and antioxidant defense ability of organism are closely related to the ambient temperature (Zhang et al., 2021). A high-temperature environment can increase metabolism and accelerate oxygen consumption, which will increase the production of reactive oxygen species. For example, hydrogen peroxide (H2O2) content significantly increases in the liver of the giant spiny frog (Quasipaa spinosa) under heat stresses (Liu et al., 2018). The antioxidant defense system includes enzymatic antioxidants, such as catalase (CAT), glutathione peroxidase (GPX), glutathione reductase (GR), superoxide dismutase (SOD), and some non-enzymatic antioxidants, such as glutathione (GSH; Cheng et al., 2015; Paital et al., 2016). Liu et al. (2018) found that the antioxidant enzyme system in the liver of giant spiny frogs (Q. spinosa) was enhanced to cope with oxidative stress when they were exposed at 30°C for 48 h. Furthermore, the antioxidant system does not change in corn snake (P. guttatus) when acclimated to a simulated heat wave, although its oxidative damage level significantly decreases (Stahlschmidt et al., 2017). The telomere length of desert lizard (P. przewalskii) shortens while SOD activity did not change in a stimulated heat wave (Zhang et al., 2018). These research studies suggest that high temperature and heat wave may have a different impact on individuals.
Nanorana pleskei is endemic to the Qinghai Tibet Plateau and belongs to the Dicroglossinae family. It is distributed at 2,800 to 5,100 m above sea level and is considered to be one of the amphibians with highest altitude distribution in the world (Zhang et al., 2012). Niu et al. (2018) investigated oxidative stress and antioxidant defense in hibernating N. parkeri, and the results showed that the level of oxidative stress and oxidative damage was significantly increased, but that the total antioxidant capacity was significantly decreased compared with the summer frogs. Zhang et al. (2021) found that long-term cold acclimation would inhibit the antioxidant defense system of N. pleskei. However, there is little know about how N. pleskei responds to the warming environment at the organism’s performance and physiological and molecular levels.
Herein, to investigate the influence of high temperatures and heat wave on the high-altitude N. pleskei in the background of global and plateau warming, we measured the thermal biology, locomotor performance, oxidative stress, and antioxidant defense system in liver and muscle of frogs acclimated to high temperatures and heat waves, and compared the results with that of the field individuals. Our results provide a reference for studies on the physiological and biochemical mechanisms of high-altitude amphibians adapted to high temperatures, and for survival prediction and protection of high-altitude amphibians.
Materials and Methods
Ethics Statement
This study was carried out under the approval of the Ethics Committee of Animal Experiments at Lanzhou University and on the basis of principles from the China Council on Animal Care. Every effort was made to minimize the numbers used and any suffering experienced by the animals in the experiment.
Animal Acclimation
Adult N. pleskei frogs (n = 84) were captured in Maqu County (33.77°N, 101.74°E, 3,490 m asl), Gansu province, China, at the end of July, 2020. They were transported to the research station of alpine meadow and wetland ecosystems of Lanzhou University in Maqu, Azi Branch Station (33.67°N, 101.87°E, 3,512 m asl). The frogs used in the experiment were captured on post-breeding period, and the sex ratio was 1:1 in the measurement of biochemical indexes. Acclimation to high temperatures and heat wave, and the measurement of thermal biology and locomotor performance were all performed in the research station 15 km from the sampling site. The frogs were randomly separated into four groups (n = 21 for each group). The thermal trials included three treatments consisting of two high-temperature treatments and one heat wave treatment. The frogs captured directly from the field were assigned to the control group. We set two high temperature groups in this study, ETem20 (20.5 ± 0.5°C) and ETem25 (25 ± 0.5°C), according to the preferred body temperature of field frogs (21 ± 0.5°C) and the monthly maximum (22.5 ± 0.5°C) in August in Maqu County from 2005 to 2017, respectively (Figure 1A). To further simulate the temperature fluctuation experienced by frogs during the active period, the heat wave group (HWG) was set based on the monthly maximum (12.1 ± 0.5°C) and minimum (1.2 ± 0.5°C) average temperatures in August in Maqu County from 2005 to 2017 (Figure 1A). The temperatures of the ETem20, ETem25, and heat wave groups were controlled 2 2 weeks by an automatic temperature control system, and three temperature data loggers (iButton DS1922G; CA, United States) were fixed in the bottom of each aquarium to record temperature changes in real-time (Figure 1B). According to our records of temperature data loggers during the acclimations, the average temperature of the heat wave group was 19.3°C, and the range of diurnal temperature fluctuation was 15–26.6°C (Figure 1B).
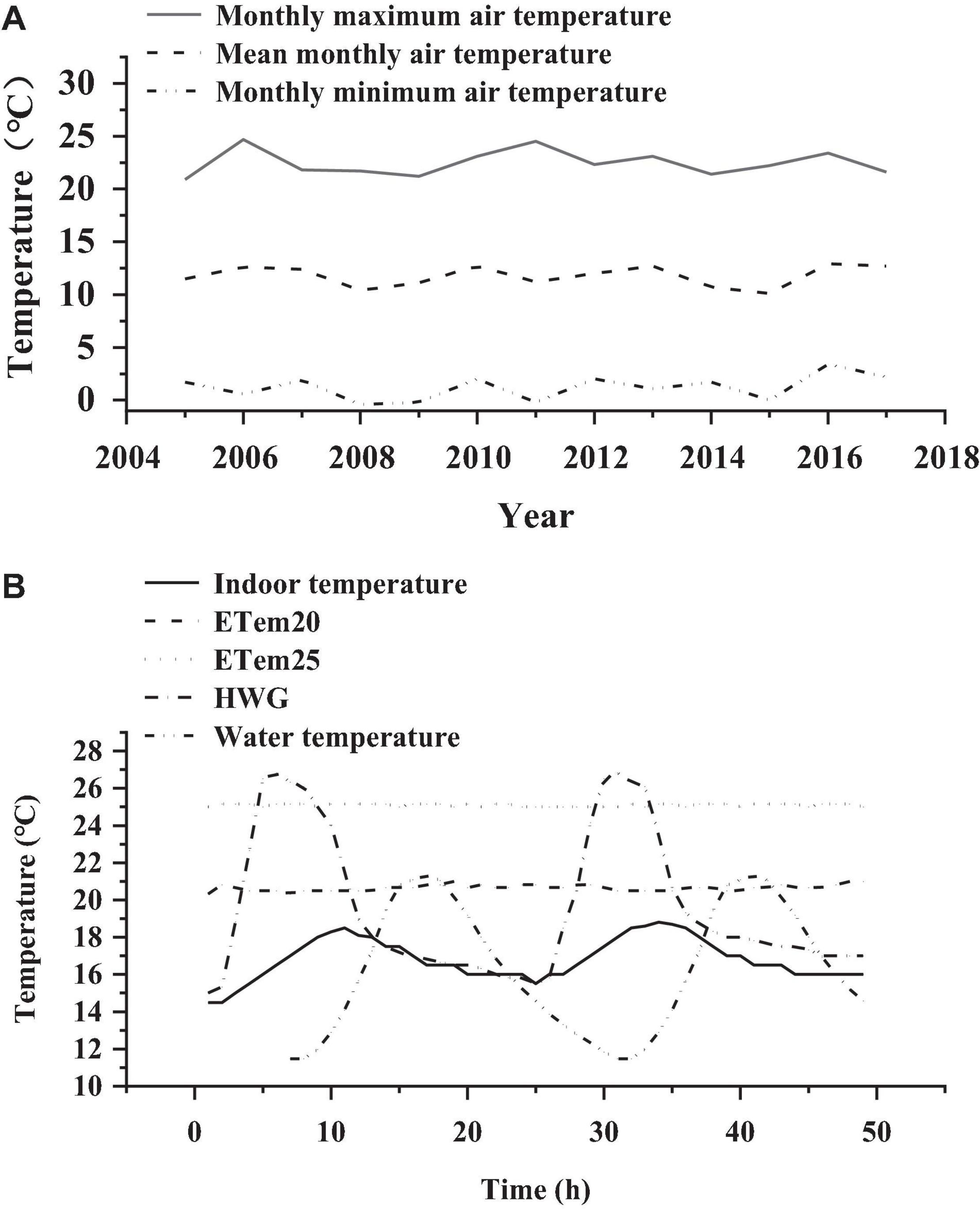
Figure 1. (A) Monthly maximum air temperature, mean monthly air temperature, and monthly minimum air temperature in August in Maqu County from 2005 to 2017. (B) Changes in temperature in ETem20, ETem25, and HWG, and changes in indoor temperature and water temperature of Nanorana pleskei for 2 consecutive days. ETem20, ETem25, HWG, and water temperature represent the group that elevated temperature to 20°C, the group that elevated temperature to 25°C, water temperature at sampling site heat, and wave group, respectively.
The frogs (n = 21 for each group) were placed in glass aquaria (48.7 cm × 35.5 cm × 28.5 cm) containing water and grass from the wild. The animals were fed with insects that were captured near the habitat of frogs, such as mosquitoes, screwworms, and locust larvae. The water and grass were replaced every 3 days. The photoperiod was 12 L: 12 D. Before the experiment, the cloacal temperature, snout-to-vent length (SVL), and body mass of all the frogs were measured. Body mass index (BMI, body mass × SVL–2 × 100%) was calculated before and after the acclimation treatments. Then, 13 frogs from each group were used to measure thermal biology and locomotor performance parameters, and 8 frogs from each group were sacrificed to collect the whole liver and hind leg muscle tissues. The hepatosomatic index (HSI, liver mass × body mass –1 × 100%) and muscle index (MI, muscle mass × body mass –1 × 100%) were also calculated. All tissues were quick-frozen in liquid nitrogen immediately and stored at -80°C until the measurement of biochemical parameters.
Thermal Biology
Preferred body temperature (Tpref) and ambient temperature (Tamb) were measured in a homemade cuboid-shaped glass tank (120 cm × 15 cm × 30 cm), which was covered up with a shading cloth in all the trials to create a completely dark environment (César et al., 2018). A layer of moist gauze was spread on the bottom of the tank to ensure an adequately humid environment in the trials. One side of the tank was warmed using a ceramic lamp placed inside, and the other side was cooled using ice packs placed outside. Three temperature data loggers were placed on both ends and the middle to record temperature changes in real time. Our results showed that the range of temperature gradient was 14.6 ± 0.5 to 39.1 ± 0.5°C, and that the temperature in the middle was 20.7 ± 0.5°C. Frogs (n = 13 for each group) were placed in the temperature gradient for about 1 h to adapt before each trial. After 1 h, the cloacal temperatures of the frogs were measured every 30 min using a handheld electronic thermometer (TM6801b, China). Each frog was tested three times, and the average was defined as Tpref. The ambient temperature (Tamb) in the location of each frog was also measured three times using the same thermometer, and the average was defined as Tamb.
The frogs used for measuring Tpref and Tamb were used further to measure thermal tolerances after resting for 24 h. The thermal tolerances of frogs comprise the non-lethal critical thermal maximum (CTmax) and minimum (CTmin), which were the cloacal temperatures at which frogs lose their ability of righting reflex at high and low temperatures (Lowe and Vance, 1955). The time interval between the measure of CTmax and CTmin was 48 h. Low-temperature thermostat bath (HMDC-2006, China) was used to control temperatures in the measurement of CTmax and CTmin. In the process of measuring CTmax and CTmin, water temperature was gradually increased or decreased at a rate of.33°C min–1. In the measurement of thermal tolerances, the frogs were checked every 5 min at the beginning, and then checked every 1 min when their movement slows down until individuals lose their ability of righting reflex, and then the cloacal temperature was recorded as the CTmax and CTmin. The measurement time of each group was not more than 30 min to avoid short-term adaptation.
Stamina and Burst Swimming Speed
After 48 h of measuring CTmax and CTmin, the stamina of frogs was measured in a 2.7-m homemade runway with a water temperature of about 16°C. An individual was chased by a glass stick until it was exhausted and lost the ability of righting reflex, and then the total distance and time of jumping to exhaustion were recorded to characterize the stamina of frogs. The burst swimming speed of frogs was further measured after 48 h of rest. The frogs were placed in a custom-made cuboid-shaped glass tank (120 cm × 15 cm × 30 cm) containing 10-cm deep water collected from the field, and a ruler with an accuracy of.1 cm was fixed at the bottom of the tank to record the distance of swimming. A digital camera (Canon A610; Canon, Japan) was fixed above the tank to videotape the swimming movements of the frogs when they were being stimulated with a glass stick on the tail. Then, the videos were analyzed to measure the swimming distance during the 1 s using the Adobe Premiere Pro 2020 frame-by-frame. Each video was analyzed 10 times, and the maximum distance during the 1 s was regarded as burst swimming speed (cm/s).
Measurement of Oxidative Damage and Antioxidant Activity Indices
The liver and muscle tissues from the eight frogs in each group were accurately weighed, and then we immediately added a quantitative pre-cooled.8% saline solution in a 1:4 ratio in 1.5-ml centrifugal tubes to thoroughly grind, and then the supernatant fluid was collected by centrifuging the tissue fluid at 3,000 rpm and 4°C for 10 min. Afterward, the supernatant fluid was diluted in proportion to 10, 1, 0.3, and.1% tissue solution with the pre-cooled.8% saline solution. Chemical assay kits were used to determine the total protein content, MDA, H2O2, GPX, SOD, CAT, GR, T-AOC, GSH, and GSSG (Nanjing Jiancheng Bioengineering Institute, Nanjing, China) according to the instructions. The GSH-eq was calculated as the sum of GSH and 2 × GSSG.
Statistical Analysis
All the data were represented as means ± standard error and analyzed on the SPSS 20.0 (IBM SPSS statistics 20.0). The data were checked by Kolmogorov–Smirnov test for normality. The statistical differences (P < 0.05) of each measured parameter among the high temperatures, heat wave, and field groups were analyzed by one-way ANOVA. The results of homogeneity variance were showed in the one-way ANOVA analysis, and LSD post hoc test for data in homogeneity and Tamhane’s T2 test for data in heterogeneity were used in the post hoc multiple comparisons to detect the differences between every two groups (P < 0.05). Furthermore, a paired t-test was performed to compare the significant difference in measured parameters before and after acclimation of each group. All graphs were generated with Origin 2018 64Bit (OriginLab, American).
Results
Morphological Characteristics
There was no significant difference in body mass (BM) and body size (SVL) of the frogs among the field groups, ETem20, ETem25, and HWG (BM: F3,82 = 0.271, P < 0.05; SVL: F3,77 = 0.914, <0.05), at the beginning of the acclimation trial (Table 1). After 2 weeks of acclimation to high temperatures and heat wave, the body mass in ETem25 (t21 = 1.926, P < 0.05) and the BMI in ETem20 (t21 = 2.31, P < 0.05) and ETem25 (t21 = 3.342, P < 0.01) was significantly decreased compared with before acclimation, but there was no significant difference in HWG (Table 1). In addition, the hepatosomatic index was significantly decreased in ETem25 (F3,28 = 2.441, P < 0.05) compared with the field groups, ETem20 and HWG. The muscle index was remarkably increased in ETem20 compared with field group, ETem25, and HWG (F3,28 = 5.035, P < 0.01).
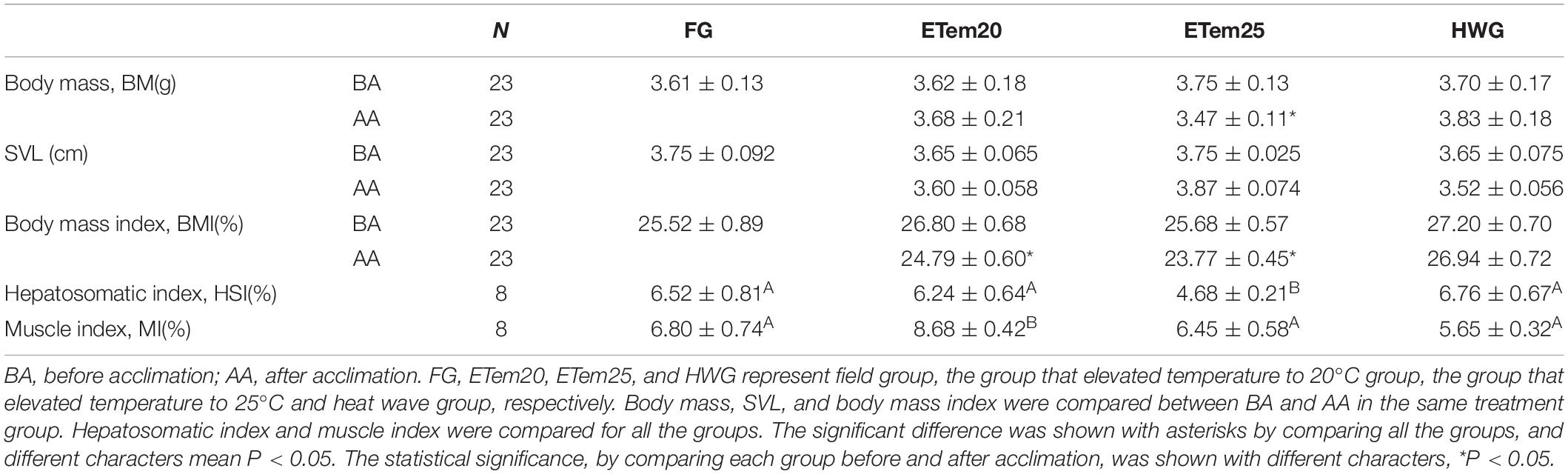
Table 1. Morphological comparison of Nanorana pleskei in different temperature acclimation groups and field group.
Thermal Biology
Thermal preference was significantly increased in ETem20 (23.2 ± 0.61°C, F3,41 = 19.007, P < 0.01) and ETem25 (23.57 ± 0.21°C, F3,41 = 19.007, P < 0.01) compared to the that in field group (21.73 ± 0.69°C) (Figure 2). Moreover, Tpref was decreased in HWG compared with ETem25 (F3,41 = 19.007, P < 0.001). Tamb was also significantly increased in ETem20 (24.84 ± 1.14°C, F3,44 = 3.412, P < 0.01), ETem25 (23.39 ± 0.53°C, F3,44 = 3.412, P < 0.01), and HWG (23.85 ± 0.59°C, F3,44 = 3.412, P < 0.01) compared to the field group (21.23 ± 0.78°C). Tamb did not have a significant difference among the high temperature groups and heat wave group (Figure 2).
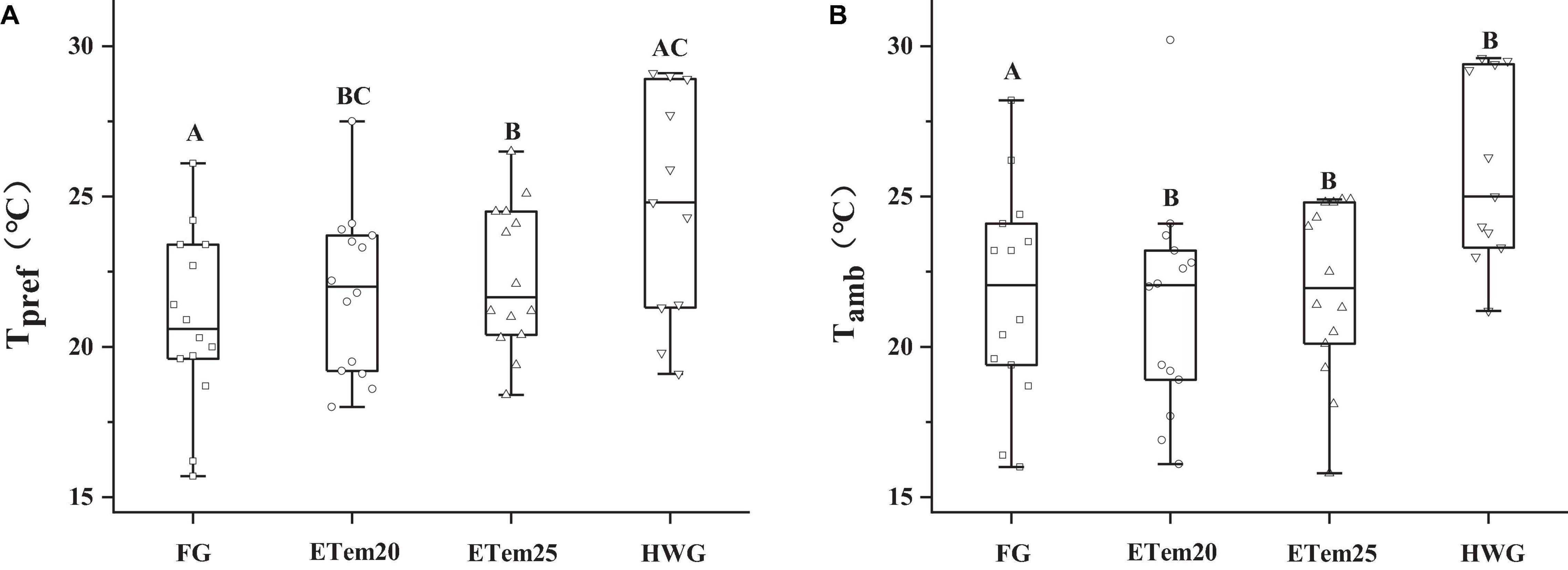
Figure 2. Comparison of (A) thermal preference (Tpref) and (B) ambient temperature (Tamb) of N. pleskei (n = 13) in different temperature acclimation groups and field group. The line inside the box plot denotes median, the borders of the box depict 25th and 75th quartiles, and the whiskers represent the 5th and 95th percentiles. The dots indicate the outliers and data points. The significant difference was shown by comparing all the groups. Different characters mean P < 0.05.
The CTmax, CTmin, and thermal tolerance range of N. pleskei are illustrated in Figure 3. Compared with the field group (CTmax = 31.62 ± 0.33°C, CTmin = 3.17 ± 0.34°C), CTmax in ETem20 (33.39 ± 0.3°C, F3,44 = 8.707, P < 0.001), ETem25 (32.63 ± 0.14°C, F3,44 = 8.707, P < 0.001), and HWG (32.56 ± 0.16°C, F3,44 = 8.707, P < 0.01) were markedly elevated, and CTmin was significantly reduced in ETem20 (2.16 ± 0.25°C, F3,38 = 11.652, P < 0.01), ETem25 (1.74 ± 0.06°C, F3,38 = 11.652, P < 0.001), and HWG (1.47 ± 0.11°C, F3,38 = 11.652, P < 0.001) (Figures 3A,B). Besides, compared with ETem25 and HWG, CTmax significantly increased in ETem20 (F3,44 = 8.707, P < 0.05). The thermal tolerance range (ΔCT, the value of CTmax minus CTmin) was significantly increased in ETem20 (31.74 ± 0.42°C, F3,42 = 18.499, P < 0.001), ETem25 (30.93 ± 0.23°C, F3,42 = 18.499, P < 0.001), and HWG (31.13 ± 0.22°C, F3,42 = 18.499, P < 0.001) compared with the field group (28.25 ± 0.57°C) (Figure 3C). There was no significant difference in CTmin and thermal tolerances range among the high temperatures group and heat wave group (Figures 3B,C).
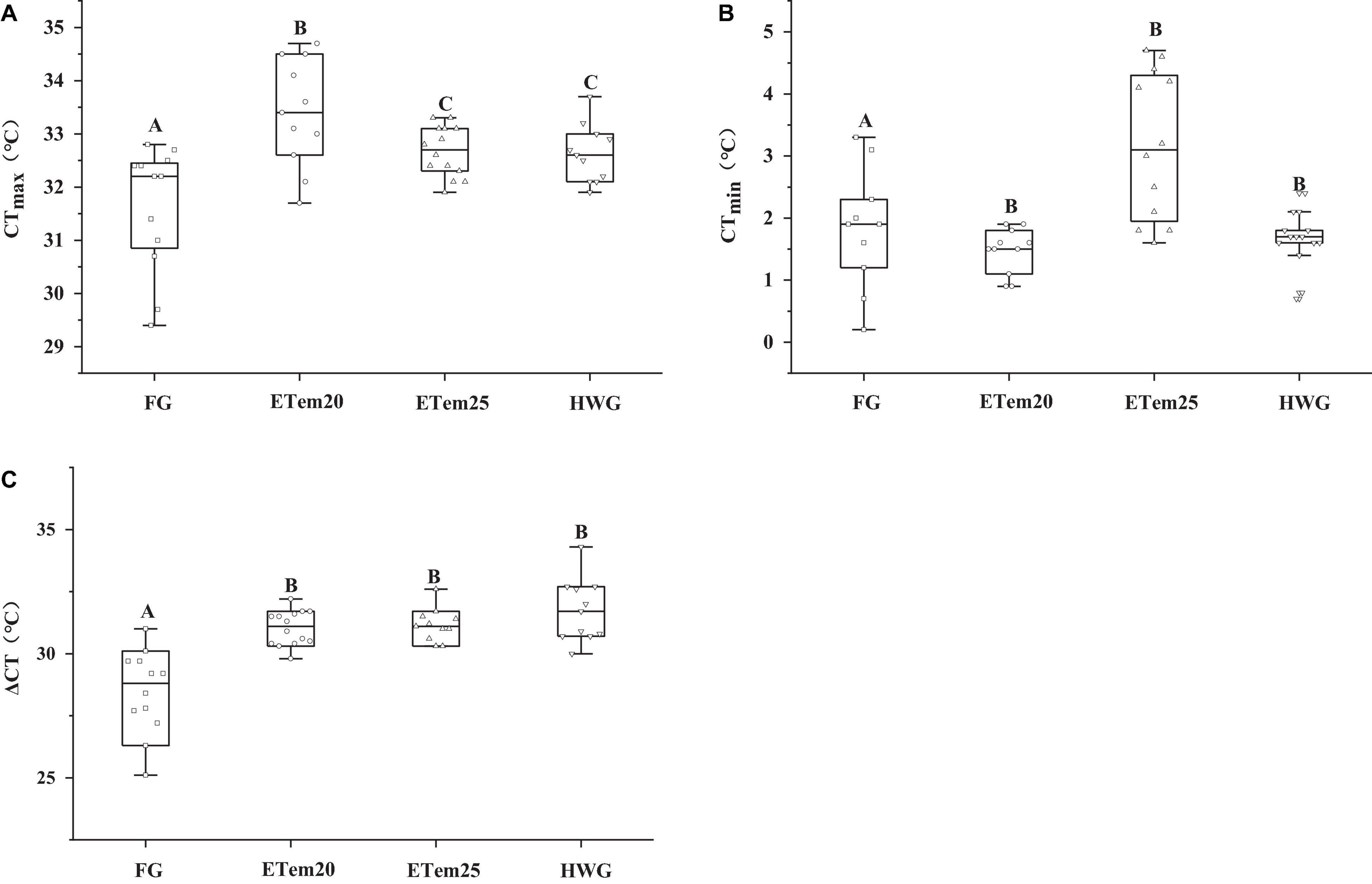
Figure 3. Comparison of (A) critical thermal maximum (CTmax), (B) critical thermal minimum (CTmin), and (C) thermal tolerance range (ΔCT) of N. pleskei (n = 13) in different temperature acclimation groups and field group. The significant difference was shown by comparing all the groups. Different characters mean P < 0.05.
Stamina and Burst Swimming Speed
The total time of jumping to exhaustion was significantly influenced by acclimation temperatures, but there was no significant difference in the total distance to exhaustion among the ETem20, ETem25, and heat wave groups (F3,38 = 0.66, P > 0.05) (Table 2). Compared with the field group, the total time to exhaustion was markedly shortened (F3,38 = 3.337, P < 0.001; F3,38 = 3.337, P < 0.001; F3,38 = 3.337, P < 0.001), and the burst swimming speed (F3,38 = 12.32, P < 0.01; F3,38 = 12.32, P < 0.001; F3,38 = 12.32, P < 0.001) was significantly increased in ETem20, ETem25, and HWG, respectively (Table 2). The total time to exhaustion was significantly decreased in ETem20 compared with HWG (F3,38 = 3.337, P < 0.01), and the burst swimming speed was significantly decreased in ETem20 compared with ETem25 (F3,38 = 12.32, P < 0.05).

Table 2. Stamina and burst swimming performance comparison of N. pleskei in different temperature acclimation groups and field group.
Oxidative Stress and Oxidative Damage in Liver and Muscle
The results of post hoc test showed that the level of MDA in the liver was significantly increased in ETem20 (F3,22 = 21.361, P < 0.01) but significantly decreased in ETem25 (F3,22 = 21.361, P < 0.001) and HWG (F3,22 = 21.361, P < 0.05) compared with the field group, and that it was remarkably enhanced in ETem20 compared with ETem25 and HWG (F3,22 = 21.361, P < 0.001). Furthermore, the level of MDA in the muscle was significantly decreased in ETem20 (F3,23 = 363.323, P < 0.001) and increased in ETem25 (F3,23 = 363.323, P < 0.001) compared with the field group, and significantly increased in the ETem25 (F3,23 = 363.323, P < 0.001) compared with ETem20 and HWG (Figure 4A). The level of H2O2 in the liver was significantly elevated in ETem20 (F3,23 = 13.785, P < 0.001) and ETem25 (F3,23 = 13.785, P < 0.001) compared with the field group and HWG. It was also significantly increased in the ETem25 (F3,23 = 75.799, P < 0.001) compared with the field group, ETem20, and HWG in the muscle (Figure 4B).
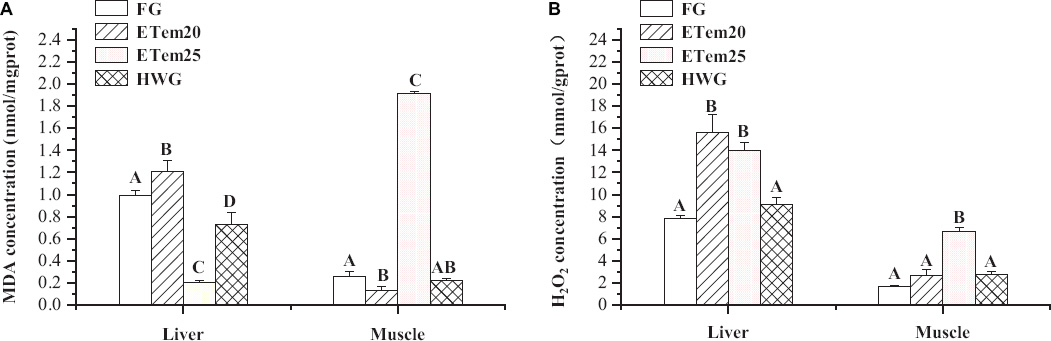
Figure 4. Changes in panel (A) malondialdehyde (MDA) and (B) H2O2 level in the liver and muscle of N. pleskei (n = 8) in different temperature acclimation groups and field group. The sample size of each group was eight. The significant difference was shown by comparing all the groups. Different characters mean P < 0.05.
The level of GSH, an effective antioxidant, in the liver (F3,26 = 23.92, P < 0.001; F3,26 = 23.92, P < 0.001; F3,26 = 23.92, P < 0.001) and muscle (F3,20 = 153.889, P < 0.001; F3,20 = 153.889, P < 0.001; F3,20 = 153.889, P < 0.001) was remarkably decreased in ETem20, ETem25, and HWG compared with the field group, respectively. In addition, it was significantly increased in HWG (F3,26 = 23.92, P < 0.05) compared with ETem20 and ETem25 in the liver, but significantly decreased in ETem20 (F3,20 = 153.889, P < 0.001) compared with ETem25 and HWG in the muscle (Table 3). The ratio of GSH/GSSG, an oxidative stress index, was significantly decreased in the liver (F3,25 = 24.089, P < 0.001; F3,25 = 24.089, P < 0.001; F3,25 = 24.089, P < 0.001) and muscle (F3,21 = 60.417, P < 0.001; F3,21 = 60.417, P < 0.001; F3,21 = 60.417, P < 0.001) of ETem20, ETem25, and HWG compared with the field group. Furthermore, it was significantly increased in HWG (F3,25 = 24.089, P < 0.05) compared with ETem20 and ETem25 in the liver, and was significantly decreased in ETem20 (F3,21 = 60.417, P < 0.001) compared with ETem25 and HWG in the muscle (Table 3).
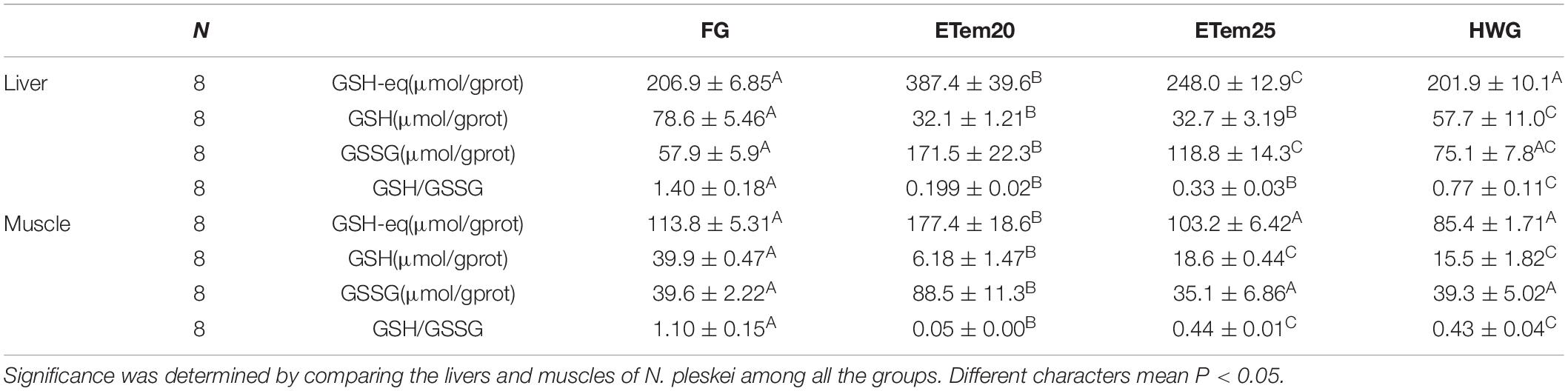
Table 3. Glutathione status in the liver and muscle of N. pleskei in different temperature acclimation groups and field group.
Antioxidant Activity in Liver and Muscle
The acclimation to high temperatures had a significant effect on antioxidant enzymes activities and total antioxidant capacity (T-AOC; Figure 5). The activity of CAT increased by 2-fold in ETem20 (F3,27 = 16.714, P < 0.001) and HWG (F3,27 = 16.714, P < 0.001) in the liver compared with the field group and ETem25, but was decreased by 1.9-fold in ETem20 (F3,26 = 8.121, P < 0.001), 2.3-fold in ETem25 (F3,26 = 8.121, P < 0.001), and 2.6-fold in HWG (F3,26 = 8.121, P < 0.001) in the muscle compared with the field group (Figure 5A). The activity of GR in the liver increased by 1.7-fold in ETem20 (F3,25 = 386.082, P < 0.001), but decreased by 3.2-fold in ETem25 (F3,25 = 386.082, P < 0.001) and 1.6-fold in HWG (F3,25 = 386.082, P < 0.001), whereas in the muscle, it decreased by 1.8-fold in ETem20 (F3,26 = 133.269, P < 0.001), 2-fold in HWG (F3,26 = 133.269, P < 0.001), and increased by 2.7-fold in ETem25 (F(1,13) = 20.049, P < 0.001) compared with the field group (Figure 5B). In addition, it significantly increased in ETem20 (F3,25 = 386.082, P < 0.001) compared with ETem25 and HWG in the liver, and increased in ETem25 (F3,26 = 133.269, P < 0.001) compared with ETem20 and HWG in the muscle. Compared with the field group, the activity of GPX increased by 2.7-fold in ETem20 (F3,25 = 33.278, P < 0.001), 2.3-fold in ETem25 (F3,25 = 33.278, P < 0.01) and decreased by 1.9-fold in HWG (F3,25 = 33.278, P < 0.05) in the liver, and it decreased by 2.6-fold in ETem20 (F3,25 = 67.267, P < 0.01), 4.1-fold in HWG (F3,25 = 67.267, P < 0.001) and increased by 1.3-fold in ETem25 (F3,25 = 67.267, P < 0.001) in the muscle (Figure 5C). Moreover, it increased in the ETem20 and ETem25 compared with the HWG (F3,25 = 33.278, P < 0.001) in the liver, and in the ETem25 (F3,25 = 67.267, P < 0.001) compared with ETem20 and HWG in the muscle (Figure 5C). The activity of SOD increased by 2.2-fold in ETem20 (F3,27 = 24.413, P < 0.001), 1.2-fold in ETem25 (F3,27 = 24.413, P < 0.01) and decreased by 1.3-fold in HWG (F3,27 = 24.413, P < 0.05) in the liver compared with the field group, but it decreased by 2-fold in ETem20 (F3,26 = 18.291, P < 0.01) and 1.8-fold in HWG (F3,26 = 18.291, P < 0.01) in the muscle compared with the field group and ETem25 (Figure 5D). In addition, it increased in the ETem20 (F3,27 = 24.413, P < 0.001) in the liver compared with the ETem25 and HWG (Figure 5D). The T-AOC in the liver increased by 2.7-fold in ETem20 (F3,20 = 47.238, P < 0.001), 1.6-fold in ETem25 (F3,20 = 47.238, P < 0.01), and decreased by 1.1-fold in HWG (F3,20 = 47.238, P < 0.05) compared with the field group. Furthermore, it increased in ETem20 (F3,20 = 47.238, P < 0.01) in the liver compared with ETem25 and HWG (Figure 5E). There was no significant difference in the muscle among all the groups (Figure 5E).
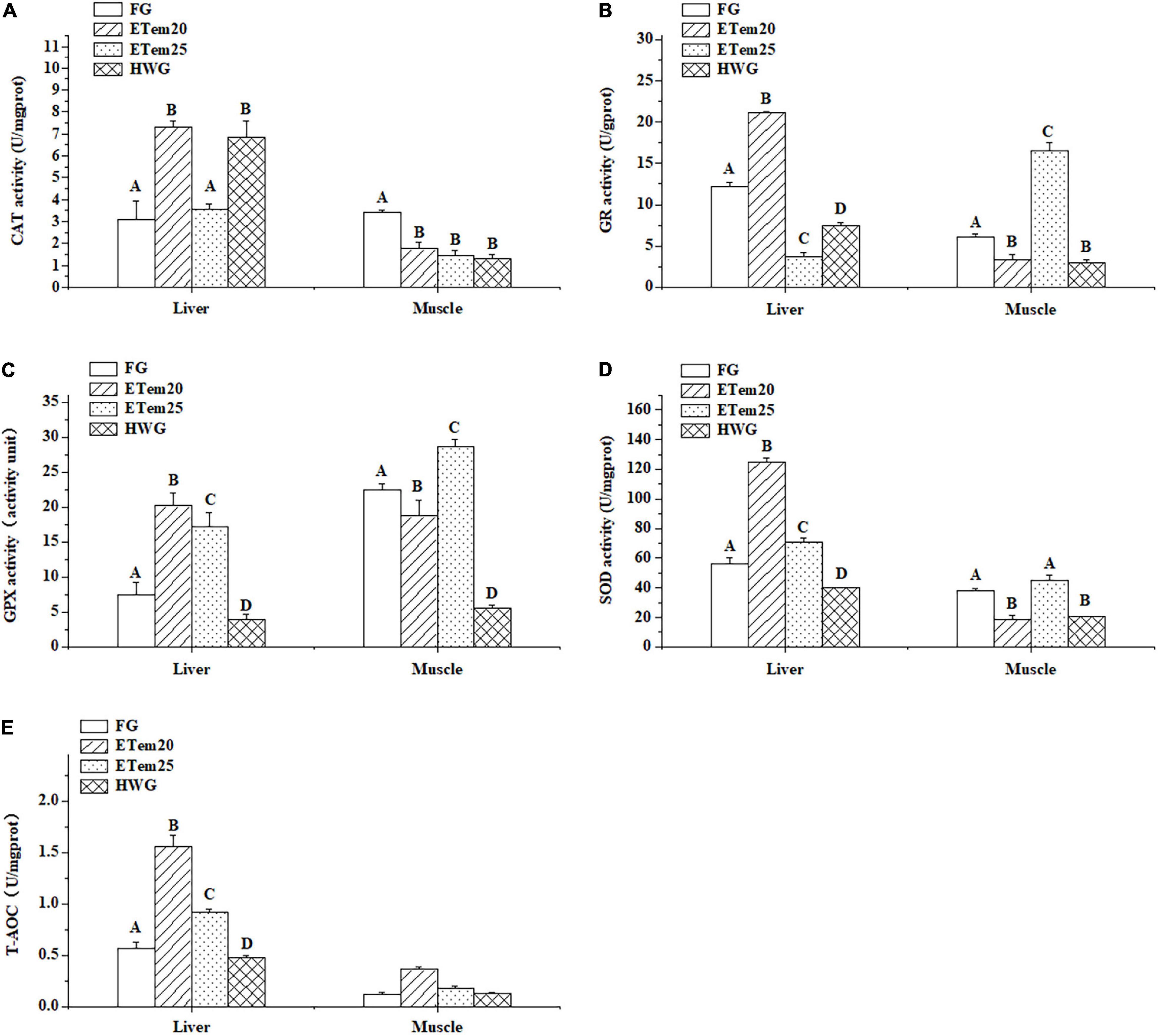
Figure 5. Changes in panel (A) catalase (CAT), (B) glutathione reductase (GR), (C) glutathione peroxidase (GPX), and (D) superoxide dismutase (SOD) activity, and (E) total antioxidant capacity (T-AOC) in the liver and muscle of N. pleskei (n = 8) in different temperature acclimation groups and field group. The sample size of each group was 8. The significant difference was shown by comparing all the groups. Different characters mean P < 0.05.
Discussion
This study investigated the effect of high temperatures and heat wave on thermal biology, locomotion performance, oxidative stress, and antioxidant defense systems in the high-altitude frog N. pleskei. The results indicated that thermal biology and locomotion performance showed plasticity for N. pleskei acclimated for 2 weeks at high temperatures and heat wave. The level of oxidative stress, oxidative damage, and antioxidant activity were remarkably enhanced in frogs acclimated at high temperatures. However, heat wave nearly has no significant influence on the oxidative stress of N. pleskei and even decreased the oxidative damage and activities of mostly antioxidant enzymes.
Preferred Body Temperature and Thermal Tolerances
The increasing ambient temperature may reduce the activity time of poikilotherms, and further affect their fitness-related activities, such as foraging and mating, which will directly lead to the decline in population size and even lead to species extinction (Gvozdík, 2012). Although behavioral thermoregulation enables them to maintain their body temperature within an optimal range, it also comes at the cost of increased energy consumption and predation risk (Sartorius et al., 2002). Tpref is closely related to the optimal temperature range of biochemical and physiological activities of organisms (Angilletta et al., 2010a). In this study, the Tpref of N. pleskei was significantly increased after acclimation to high temperatures and heat wave, compared with field frogs. The significant effect of thermal acclimation on Tpref has been confirmed in other ectotherms, such as terrestrial toad (R. icterica) (César et al., 2018), fresh-water prawn (Macrobrachium tenellum) (Hernandez-Sandoval et al., 2018), river shrimp (Cryphiops caementarius) (Avalos et al., 2020), and soft-shelled turtle (P. sinensis) (Wu et al., 2013). The great ability of thermal acclimation makes them remain active at high temperature, and expand the time window for activity, so as to partially or completely compensate for the impact of climate change (Gvozdík, 2012).
CTmax and CTmin are usually defined as the upper limit and lower limit of individual survival, and animals will experience movement disorder, spasm, and loss of righting reflex or balance when the ambient temperature reaches or exceeds the high-temperature and low-temperature limits (Lowe and Vance, 1955). We found that both the CTmax (31.62°C) and CTmin(3.17°C) of unacclimated frog N. pleskei were higher than the annual maximum (24.7°C) and minimum(−24.4°C) temperatures in their distribution range. Ectothermic animals have thermal tolerance after a short period of sublethal temperature, which is an acute response, enabling them to cope with short-term fluctuations in ambient temperature (Hong et al., 2009). That was aligned with the results, 2 weeks of acclimation to high temperatures significantly increased the temperature tolerance range of N. pleskei (CTmax was significantly increased, and CTmin was significantly decreased). The plasticity of thermal tolerance has also been demonstrated in other exothermic animals, such as Galaxias zebratus (Olsen et al., 2021), Argyrosomus regius (Kır et al., 2017), and Eleutherodactylus coqui (Haggerty, 2016).
The Tpref, CTmax, and CTmin of frogs acclimated in heat wave also showed plasticity. The Tamb and CTmax increased and CTmin decreased significantly, although there was no significant difference in the Tpref compared with field frogs. Brusch et al. (2016) investigated the effects of microhabitat type, species, and SVL on thermal tolerances of 10 lizards from Costa Rica and revealed that microhabitat type is an important factor to predict the maximum tolerated temperature regardless of size or evolutionary relationship. The climate conditions in Tibetan plateau are complex and changeable, such as windy and dry in spring and winter, rainy in summer and autumn, and great circadian temperature difference (Zhang et al., 2006). In addition, Zhao et al. (2014) found that the summer days and daily maximum and minimum air temperature have all risen, while the freezing and frost days have all declined in the QTP from 1963 to 2012. The warming trend of lowest temperature was more striking than that of highest temperature. Chen et al. (2020) predicted that the number of heat wave days in the Qinghai Tibet Plateau will significantly increase in the future. Therefore, we suggested that the plasticity of thermal biology in frogs acclimated to high temperatures and heat wave may be closely related to the climate of microhabitat of N. pleskei on the plateau, and that it would show great adaptability to the variability of habitat climate. The thermal tolerance of high-altitude N. pleskei may be due to their adaptation mechanism to the changing thermal environment, enabling them to survive in the short-term exposure at high temperatures. A similar phenomenon has also been found in the anuran species that have transformed from aquatic to terrestrial or semi-terrestrial during ontogenesis, and their increased thermal tolerance is also important for their survival, as they may be exposed to a wide range of diurnal temperature fluctuations (Sherman, 1980; Rome et al., 1992).
Stamina and Burst Swimming Speed
As found in most frogs and toads (Knowles and Weigl, 1990; Wilson and Franklin, 1999; Wilson et al., 2000; Padilla et al., 2019), the locomotor performance of highland N. pleskei was also affected by the acclimation temperature. Compared with the field group, the total time of jumping to exhaustion significantly decreased while the burst swimming speed of the frogs was significantly increased after the acclimation to high temperatures and heat wave. The locomotor performance of the animals, especially the burst performance, is important for avoiding predators and catching prey. However, stamina may be more important in finding and copulating with mates. Probably there is a trade-off between these two locomotor properties, as they depend on different underlying physiological mechanisms (Padilla et al., 2019). Our results demonstrated that N. pleskei was able to jump faster until exhaustion and swim faster after the acclimation to high temperature and heat wave, and that this may be more likely to contribute to their foraging and avoiding predators successfully to maintain normal physical condition (Preest and Pough, 2003). Accordingly, we speculated that the strengthening of burst ability and the attenuation of endurance may be beneficial to the survival of frogs in a short time, but with the continuous rising of global temperatures, further research is needed to assess the impact of extreme high temperature on the survival of plateau amphibians.
Oxidative Stress and Oxidative Damage
The oxygen consumption and metabolism of animals increase with rise in temperature within a certain range, resulting in increased generation of reactive oxygen species (ROS; Paital et al., 2016). The overproduction of ROS will damage DNA, proteins, lipids, and other biomolecules (Cheng et al., 2015). The thermal acclimation significantly increased the level of H2O2 (Figure 4B), and significantly decreased GSH content and GSH/GSSG ratio in the liver and muscle in N. pleskei (Table 3). This suggests that high-temperature-acclimated N. pleskei is in a state of oxidative stress compared to the field frogs. The body mass index (BMI) and muscle index (MI) values significantly decreased in the frogs after acclimation in high temperatures compared with those before acclimation, and the hepatosomatic index (HSI) was lowest in ETem25 among all the groups (Table 1). These may be the result of the energy budget among antioxidation, growth, and development for N. pleskei in response to high temperatures (Ferral et al., 2020). Niu et al. (2018) and Zhang et al. (2021) have demonstrated that the content of GSH-eq and GSSG in N. parkeri was markedly decreased in winter and long-term cold acclimation. On the contrary, the content of GSH-eq and GSSG obviously increased in the liver and muscle in N. pleskei acclimated at high temperatures and heat wave. This inconsistency may be related to food intake. The frogs were unfed during winter and cold acclimation, but food was plenty during thermal acclimation in our experiment. Therefore, it is possible that the de novo synthesis of glutathione increased in the frogs during our thermal acclimation, so the GSH-eq content in the liver and muscle significantly increased (Zhang et al., 2012; Niu et al., 2018). GSH is one of the important non-enzymatic antioxidants in cells to inhibit the production of ROS (Meister and Anderson, 1983). In our study, GSH is greatly oxidized to GSSG by the catalysis of GPX to inhibit the further production of ROS, because the activity of GPX was significantly enhanced in N. pleskei acclimated at high temperatures and heat wave (Figure 5C). This maintains the redox status in hepatocytes and muscle cells of frogs under thermal acclimation, which may be the main reason for the increase in GSSG content and decrease in GSH/GSSG ratio in the liver and muscle.
The acclimation to heat wave did not significantly influence the level of H2O2 in the liver and muscle, but it remarkably reduced the lipid oxidative damage in the liver (Figure 4). This may be due to the increase in CAT activity (Figure 5A), because it decomposes H2O2 into H2O and oxygen (Lledías et al., 1998). The GSH content in the liver and muscle, and GSH/GSSG ratio in the muscle significantly decreased after the acclimation to heat wave. These results correspond with the study on corn snake (P. guttatus), in which the acclimation to heat wave reduced its oxidative stress (Stahlschmidt et al., 2017). However, the telomere length and overwinter survival of desert toad-headed agama (Phrynocephalus przewalskii) were shortened after exposure to a stimulated heat wave (Zhang et al., 2018). In Asian yellow pond turtle (Mauremys mutica), heat wave does not significantly affect the level of MDA in the liver and muscle (Li et al., 2021). Therefore, heat wave has different physiological effects on different species, positive or negative. Our study indicated that simulated heat wave does not induce oxidative stress, and even significantly decreased oxidative damage in frogs. In addition, the BMI did not significantly change in the frogs acclimated in heat wave compared with that before the acclimation to heat wave. Therefore, we suggested that heat wave may have positive effects on N. pleskei. The possible reason for the non-significant or positive effects of heat wave is that the thermal regimes of the heat wave group may be too mild for frogs and they can recover from heat stress in the low-temperature period.
Antioxidant Defense System
Under normal conditions, the production and elimination of ROS maintain a dynamic balance in cell metabolism. Thermal stress could impair the balance between oxidant and antioxidant defense mechanisms, causing oxidative stress and oxidative damage in tissues of organisms (Maud et al., 2006; Tu et al., 2012). As we have mentioned above, higher temperature acclimation induced oxidative stress and oxidative damage in N. pleskei (Figure 4). To offset the adverse effects of peroxides, the organism usually activates the antioxidant enzymes and some non-enzymatic antioxidants to resist oxidative stress and prevent or repair oxidative damage (Tu et al., 2012; Duan et al., 2016). In this study, compared to the field group, the activities of GPX, GR, CAT, SOD, and T-AOC in the liver were significantly increased in ETem20 (Figure 5). In addition, the activities of GPX and SOD and T-AOC in the liver, and GPX and GR activity in the muscle were significantly increased in ETem25 (Figure 5). These results indicated that the overall antioxidant capacity of N. pleskei is enhanced after high-temperature acclimation. Similar inference was found in giant spiny frog (Quasipaa spinosa) (Liu et al., 2018), puffer fish (Takifugu obscurus) (Cheng et al., 2015), and ark shell (Scapharca broughtonii) (An and Choi, 2010).
In terms of the effect of heat wave on antioxidant defense, our results showed that the activities of GR, GPX, and SOD decreased in the liver and muscle, but that the activity of CAT in the liver increased in high-altitude frog, N. pleskei (Figure 5). This may be the reason for the significant decrease in lipid peroxide level. Similar results were found in P. guttatus in the acclimation to simulated heat wave, in which the antioxidative capacity did not change but the oxidative damage level obviously decreased (Stahlschmidt et al., 2017). On the contrary, although the activity of T-SOD and CAT, and T-AOC are not affected by heat wave, the GPX activity in the muscle in M. mutica significantly increased (Li et al., 2021). The activities of antioxidant enzymes were attenuated for N. pleskei in the acclimation to simulated heat wave, but its oxidative damage level dramatically decreased. In the process of long-term adaptation to the plateau environment, N. pleskei may have evolved a unique mechanism to deal with temperature fluctuations. Therefore, their oxidative damage and antioxidant enzyme activity did not significantly change and even attenuated with simulated heat wave treatment.
Conclusion
In conclusion, thermal preference, thermal tolerance, stamina, and burst performance showed plasticity under thermal acclimation and simulated heat wave. In addition, thermal acclimation disturbs the redox state, which is manifested as oxidative stress and oxidative damage in the liver and muscle of the high-altitude frog N. pleskei. The antioxidant capacity was enhanced significantly after high-temperatures acclimation. Unexpectedly, the heat wave did not cause oxidative stress and oxidative damage, and even decreased the activity of antioxidant enzymes. In summary, the impact of heat wave on amphibians is not as great as we expected, while the short-term extreme high temperatures have a greater impact on them. These results can provide a reference for studies on the adaptation of other high-altitude amphibians and protection of the QTP ecosystem.
Data Availability Statement
The original contributions presented in the study are included in the article/supplementary material, further inquiries can be directed to the corresponding author/s.
Ethics Statement
The animal study was reviewed and approved by the Ethics Committee of Animal Experiments at Lanzhou University.
Author Contributions
QC and JH: conceptualization. QC, JH, PP, MM, and ZN: methodology. JH: formal analysis and writing – original draft. QC, JH, TZ, FM, MM, and LX: investigation. QC, JH, and XT: data curation. PP, XT, and QC: writing – review and editing. JH and PP: visualization. QC, MM, and XT: supervision. All the authors read and approved the submitted version.
Funding
This research was supported by grants from the National Natural Science Foundation of China (3197141 to QC) and the Fundamental Research Funds for the Central Universities (lzujbky-2021-it23 to ZN).
Conflict of Interest
The authors declare that the research was conducted in the absence of any commercial or financial relationships that could be construed as a potential conflict of interest.
Publisher’s Note
All claims expressed in this article are solely those of the authors and do not necessarily represent those of their affiliated organizations, or those of the publisher, the editors and the reviewers. Any product that may be evaluated in this article, or claim that may be made by its manufacturer, is not guaranteed or endorsed by the publisher.
Acknowledgments
We would like to thank the research station of alpine meadow and wetland ecosystems of Lanzhou University in Maqu, Azi Branch Station for the assistance with animal acclimation and the Core Facility of School of Life Sciences, Lanzhou University for the technical assistance. We would also like to thank associate professor Chen Hou of Missouri University of Science and Technology, America for correcting the grammar.
References
An, M. I., and Choi, C. Y. (2010). Activity of antioxidant enzymes and physiological responses in ark shell, Scapharca broughtonii, exposed to thermal and osmotic stress: effects on hemolymph and biochemical parameters. Comp. Biochem. Physiol. Part B Biochem. Mol. Biol. 155, 34–42. doi: 10.1016/j.cbpb.2009.09.008
Angilletta, M. J., Huey, R. B., and Frazier, M. R. (2010b). Thermodynamic effects on organismal performance: is hotter better? Physiol. Biochem. Zool. 83, 197–206. doi: 10.1086/648567
Angilletta, M. J., Cooper, B. S., Schuler, M. S., and Boyles, J. G. (2010a). The evolution of thermal physiology in endotherms. Front Biosci. (Elite Ed) 2:861–881. doi: 10.2741/e148
Avalos, W. R., Vigo, L. C., Chujutalli, K. F., and Jacinto, J. S. (2020). Thermal preference of postlarvae of the river shrimp Cryphiops caementarius previously acclimated to different temperatures. Ecosistemas 29:1802. doi: 10.7818/ECOS.1802
Bennett, A. F. (1990). Thermal dependence of locomotor capacity. Am. J. Physiol. 259, 253–258. doi: 10.1111/j.1748-1716.1990.tb08968.x
Brusch, G. A., Taylor, E. N., and Whitfield, S. M. (2016). Turn up the heat: thermal tolerances of lizards at La Selva, Costa Rica. Oecologia 180, 325–334.
César, D. O. A. R., Bovo, R. P., and Andrade, D. V. (2018). Seasonal variation in the thermal biology of a terrestrial toad, Rhinella icterica (Bufonidae), from the Brazilian Atlantic forest. J. Therm. Biol. 74, 77–83. doi: 10.1016/j.jtherbio.2018.03.011
Chen, X., Li, N., Huang, C., Liu, J., and Zhang, Z. (2020). Projection of heatwaves by the combined impact of humidity and temperature in China. Prog. Geogr. 39, 36–44. doi: 10.18306/dlkxjz.2020.01.004
Cheng, C., Yang, F., Liao, S., Miao, Y., Ye, C., Wang, A., et al. (2015). High temperature induces apoptosis and oxidative stress in pufferfish (Takifugu obscurus) blood cells. J. Therm. Biol. 53, 172–179. doi: 10.1016/j.jtherbio.2015.08.002
Cherkasov, A. S., Biswas, P. K., Ridings, D. M., Ringwood, A. H., and Sokolova, I. M. (2006). Effects of acclimation temperature and cadmium exposure on cellular energy budgets in the marine mollusk Crassostrea virginica: linking cellular and mitochondrial responses. J. Exp. Biol. 209, 1274–1284. doi: 10.1242/jeb.02093
Chown, S. L., and Nicolson, S. W. (2004). Insect Physiological Ecology: Mechanisms and Patterns. New York, NY: Oxford University Press.
Clusella-Trullas, S., Terblanche, J. S., and Chown, S. L. (2010). Phenotypic plasticity of locomotion performance in the seed harvester Messor capensis (Formicidae). Physiol. Biochem. Zool. 83, 519–530. doi: 10.1086/651387
Collins, J. P., and Storfer, A. (2003). Global amphibian declines: sorting the hypotheses. Divers. Distrib. 9, 89–98. doi: 10.1046/j.1472-4642.2003.00012.x
Duan, Y., Zhang, Y., Dong, H., and Zhang, J. (2016). Effect of desiccation on oxidative stress and antioxidant response of the black tiger shrimp Penaeus monodon. Fish Shellfish Immunol. 58, 10–17. doi: 10.1016/j.fsi.2016.09.004
Ferral, N., Gomez, N., Holloway, K., Neeter, H., Fairfield, M., Pollman, K., et al. (2020). The extremely low energy cost of biosynthesis in holometabolous insect larvae. J. Insect Physiol. 120, 103988. doi: 10.1016/j.jinsphys.2019.103988
Gvozdík, L. (2012). Plasticity of preferred body temperatures as means of coping with climate change? Biol. Lett. 8, 262–265. doi: 10.1098/rsbl.2011.0960
Haggerty, J. (2016). Thermal Tolerance of the Common Coqui Frog (Eleutherodactylus coqui) in East Hawaii Along an Elevation Gradient. Master’s thesis. Hawaii: University of Hawaii.
Han, J., Guo, R., Li, J., Guan, C., Chen, Y., and Zhao, W. (2016). Organ mass variation in a toad headed lizard Phrynocephalus vlangalii in response to hypoxia and low temperature in the Qinghai-Tibet Plateau, China. PLoS One 11:e0162572. doi: 10.1371/journal.pone.0162572
Hernandez-Sandoval, P., Díaz, F., Re-Araujo, A., López-Sánchez, J., Martínez-Valenzuela, M., García-Guerrero, M., et al. (2018). Thermal preference, critical thermal limits, oxygen routine consumption and active metabolic scope of Macrobrachium tenellum (Smith, 1871) maintained at different acclimation temperatures. Lat. Am. J. Aquat. Res. 46, 558–569. doi: 10.3856/vol46-issue3-fulltext-9
Hertz, P. E., Huey, R. B., and Nevo, E. (1983). Homage to Santa Anita: thermal sensitivity of sprint speed in agamid lizards. Evolution 37, 1075–1084. doi: 10.1111/j.1558-5646.1983.tb05634.x
Hong, L., Zheng, W., Wenbin, M., and Xiang, J. (2009). Temperature acclimation affects thermal preference and tolerance in three Eremias lizards (Lacertidae). Curr. Zool. 55, 258–265. doi: 10.1360/972009-1142
Kır, M., Sunar, M. C., and Altındaǧ, B. C. (2017). Thermal tolerance and preferred temperature range of juvenile meagre acclimated to four temperatures. J. Therm. Biol. 65, 125–129. doi: 10.1016/j.jtherbio.2017.02.018
Knowles, T. W., and Weigl, P. D. (1990). Thermal dependence of anuran burst locomotor performance. Copeia 1990, 796–802. doi: 10.2307/1446445
Lapwong, Y., Dejtaradol, A., and Webb, J. K. (2020). Shifts in thermal preference of introduced Asian house geckos (Hemidactylus frenatus) in temperate regions of southeastern Australia. J. Therm. Biol. 91:102625. doi: 10.1016/j.jtherbio.2020.102625
Li, S., Li, J., Chen, W., Xu, Z., Xie, L., and Zhang, Y. (2021). Effects of simulated heat wave on oxidative physiology and immunity in Asian yellow pond turtle (Mauremys mutica). Front. Ecol. Evol. 9:704105. doi: 10.3389/fevo.2021.704105
Liu, Z., Gu, W., Tu, D., Zhu, Q., Zhou, Y., Wang, C., et al. (2018). Effects of both cold and heat stresses on the liver of giant spiny frog Quasipaa spinosa : stress response and histological changes. J. Exp. Biol. 221:jeb186379. doi: 10.1242/jeb.186379
Lledías, F., Rangel, P., and Hansberg, W. (1998). Oxidation of catalase by singlet oxygen. J. Biol. Chem. 273, 10630–10637. doi: 10.1074/jbc.273.17.10630
Lowe, C. H., and Vance, V. J. (1955). Acclimation of the critical thermal maximum of the reptile Urosaurus ornatus. Science 122, 73–74. doi: 10.1126/science.122.3158.73
Maud, A. J., Gonzalez, P., Marie, V., Baudrimont, M., and Bourdineaud, J. (2006). Cytochrome c oxydase subunit I gene is up-regulated by cadmium in freshwater and marine bivalves. Biometals 19, 237–244. doi: 10.1007/s10534-005-5671-9
Meister, A., and Anderson, M. E. (1983). Glutathione. Annu. Rev. Bioche. 52, 711–760. doi: 10.1146/annurev.bi.52.070183.003431
Mendelson, J. R., Lips, K. R., Gagliardo, R. W., Rabb, G. B., Collins, J. P., Diffendorfer, J. E., et al. (2006). Biodiversity. Confronting amphibian declines and extinctions. Science 313:48. doi: 10.1126/science.1128396
Niu, Y., Cao, W., Zhao, Y., Zhai, H., Zhao, Y., Tang, X., et al. (2018). The levels of oxidative stress and antioxidant capacity in hibernating Nanorana parkeri. Comp. Biochem. Physiol. Part A Mol. Integr. Physiol 219-220, 19–27. doi: 10.1016/j.cbpa.2018.02.003
Olsen, T., Shelton, J. M., and Dallas, H. F. (2021). Does thermal history influence thermal tolerance of the freshwater fish Galaxias zebratus in a global biodiversity hotspot? J. Thermal Biol. 97:102890.
Padilla, P., Ducret, V., Bonneaud, C., Courant, J., and Herrel, A. (2019). Acclimation temperature effects on locomotor traits in adult aquatic anurans (X. tropicalis and X. laevis) from different latitudes: possible implications for climate change. Conserv. Physiol. 7:coz019. doi: 10.1093/conphys/coz019
Paital, B., Panda, S. K., Hati, A. K., Mohanty, B., Mohapatra, M. K., Kanungo, S., et al. (2016). Longevity of animals under reactive oxygen species stress and disease susceptibility due to global warming. World J. Biol. Chem. 7, 118–135. doi: 10.4331/wjbc.v7.i1.110
Panov, V. E., and Mcqueen, D. J. (1998). Effects of temperature on individual growth rate and body size of a freshwater amphipod. Can. J. Zool. 76, 1107–1116. doi: 10.1139/cjz-76-6-1107
Peng, L., Tang, M., Liao, J., Liang, S., Gan, L., Hua, K., et al. (2020). Effects of temperature on growth and development of amphibian larvae across an altitudinal gradient in the Tibetan Plateau. Anim. Biol. 70, 239–250.
Pörtner, H. O., and Farrell, A. P. (2008). Physiology and climate change. Science 322, 690–692. doi: 10.1126/science.1163156
Preest, M. R., and Pough, F. H. (2003). Effects of body temperature and hydration state on organismal performance of toads, Bufo americanus. Physiol. Biochem. Zool. 76, 229–239.
Rome, L. C., Stevens, E. D., and John-Alder, H. B. (1992). “The influence of temperature and thermal acclimation on physiological function,” in Environmental Physiology of the Amphibians, eds M. E. Feder and W. W. Burggren (Chicago, IL: University of Chicago Press).
Sartorius, S. S., Amaral, J. D., Durtsche, R. D., Deen, C. M., and Lutterschmidt, W. I. (2002). Thermoregulatory accuracy, precision, and effectiveness in two sand-dwelling lizards under mild environmental conditions. Can. J. Zool. 80, 1966–1976. doi: 10.1139/z02-191
Sherman, E. (1980). Ontogenetic change in thermal tolerance of the toad Bufo woodhousii fowleri. Comp. Biochem. Physiol. Part A Physiol. 65, 227–230. doi: 10.1016/0300-9629(80)90229-7
Stahlschmidt, Z. R., French, S. S., Ahn, A., Webb, A., and Butler, M. W. (2017). A simulated heat wave has diverse effects on immune function and oxidative physiology in the corn snake (Pantherophis guttatus). Physiol. Biochem. Zool. 90, 434–444. doi: 10.1086/691315
Stillwell, R. C., and Fox, C. W. (2005). Complex patterns of phenotypic plasticity: pnteractive effects of temperature during rearing and oviposition. Ecology 86, 924–934. doi: 10.1890/04-0547
Stocker, T. F., Qin, D., Plattner, G. K., Tignor, M. M. B., Allen, S. K., Boschung, J., et al. (2014). “Climate change 2013: the physical science basis,” in Proceedings of the Contribution of Working Group I to the Fifth Assessment Report of IPCC the Intergovernmental Panel on Climate Change (Cambridge: Cambridge University Press).
Stuart, S. N., Chanson, J. S., Cox, N. A., Young, B. E., Rodrigues, A. S., Fischman, D. L., et al. (2004). Status and trends of amphibian declines and extinctions worldwide. Science 306, 1783–1786. doi: 10.1126/science.1103538
Tu, H. T., Silvestrea, F., Meulder, B. D., Thome, J. P., Phuong, N. T., and Kestemont, P. (2012). Combined effects of deltamethrin, temperature and salinity on oxidative stress biomarkers and acetylcholinesterase activity in the black tiger shrimp (Penaeus monodon). Chemosphere 86, 83–91. doi: 10.1016/j.chemosphere.2011.09.022
Wake, D. B., and Vredenburg, V. T. (2008). Are we in the midst of the sixth mass extinction? A view from the world of amphibians. Proc. Natl. Acad. Sci. U.S.A. 105(Suppl. 1), 11466–11473. doi: 10.1073/pnas.0801921105
Wilson, R. S., and Franklin, C. E. (1999). Thermal acclimation of locomotor performance in tadpoles of the frog Limnodynastes peronii. J. Comp. Physiol. B 169, 445–451. doi: 10.1007/s003600050241
Wilson, R. S., James, R. S., and Johnston, I. A. (2000). Thermal acclimation of locomotor performance in tadpoles and adults of the aquatic frog Xenopus laevis. J. Comp. Physiol. B 170, 117–124. doi: 10.1007/s003600050266
Wollmuth, L. P., Crawshaw, L. I., Forbes, R. B., and Grahn, D. A. (1987). Temperature selection during development in a montane anuran species, Rana cascadae. Physiol. Zool. 60, 472–480.
Wu, M., Hu, L., Dang, W., Lu, H., and Du, W. (2013). Effect of thermal acclimation on thermal preference, resistance and locomotor performance of hatchling soft-shelled turtle. Curr. Zool. 59, 718–724. doi: 10.1093/czoolo/59.6.718
Yi, Z., Fan, G., Wei, H., and Wang, Q. (2018). Analysis of the temporal and spatial variation in land surface temperature over the Qinghai-Tibet Plateau from 1981 to 2015. J. Southwest Univ. 40, 128–140. doi: 10.13718/j.cnki.xdzk.2018.11.018
Zhang, L., Ma, X., Jiang, J., and Lu, X. (2012). Stronger condition dependence in female size explains altitudinal variation in sexual size dimorphism of a Tibetan frog. Biol. J. Linn. Soc. 107, 558–565. doi: 10.1111/j.1095-8312.2012.01953.x
Zhang, Q., Han, X., Hao, X., Ma, L., Li, S., Wang, Y., et al. (2018). A simulated heat wave shortens the telomere length and lifespan of a desert lizard. J. Therm. Biol. 72, 94–100. doi: 10.1016/j.jtherbio.2018.01.004
Zhang, Q., Kang, S., and Yan, Y. (2006). Characteristics of spatial and temporal variations of monthly mean surface air temperature over Qinghai-Tibet Plateau. Chin. Geogr. Sci. 16, 351–358. doi: 10.1007/s11769-006-0351-4
Zhang, X., Niu, Y., Zhang, H., Xu, T., Zeng, Q., Storey, K. B., et al. (2021). The effect of long-term cold acclimation on redox state and antioxidant defense in the high-altitude frog, Nanorana pleskei. J. Therm. Biol. 99:103008. doi: 10.1016/j.jtherbio.2021.103008
Keywords: thermal biology, locomotor performance, oxidative stress, antioxidant defense, high-altitude amphibian
Citation: He J, Tang X, Pu P, Zhang T, Niu Z, Meng F, Xi L, Ma M, Wu J, Ma M and Chen Q (2021) Influence of High Temperatures and Heat Wave on Thermal Biology, Locomotor Performance, and Antioxidant System of High-Altitude Frog Nanorana pleskei Endemic to Qinghai-Tibet Plateau. Front. Ecol. Evol. 9:763191. doi: 10.3389/fevo.2021.763191
Received: 23 August 2021; Accepted: 28 October 2021;
Published: 02 December 2021.
Edited by:
Jigang Xia, Chongqing Normal University, ChinaReviewed by:
Yongpu Zhang, Wenzhou University, ChinaFernando Diaz, Center for Scientific Research and Higher Education in Ensenada (CICESE), Mexico
Copyright © 2021 He, Tang, Pu, Zhang, Niu, Meng, Xi, Ma, Wu, Ma and Chen. This is an open-access article distributed under the terms of the Creative Commons Attribution License (CC BY). The use, distribution or reproduction in other forums is permitted, provided the original author(s) and the copyright owner(s) are credited and that the original publication in this journal is cited, in accordance with accepted academic practice. No use, distribution or reproduction is permitted which does not comply with these terms.
*Correspondence: Xiaolong Tang, dGFuZ3hsQGx6dS5lZHUuY24=; Qiang Chen, Y2hlbnFAbHp1LmVkdS5jbg==
†These authors have contributed equally to this work