- Geographical Ecology Group, Department of Biology, University of Oklahoma, Norman, OK, United States
Urbanization is a major cause of biotic homogenization and habitat fragmentation for native communities. However, the role of urbanization on the success of biological invasions on a continental scale has yet to be explored. Urbanization may facilitate the establishment success of invasive species by minimizing niche differentiation between native and invaded ranges. In such cases, we might expect anthropogenic variables to have stronger influence on the geographic distribution of invasive compared to native populations. In this study, we use ecological niche modeling to define the distribution of non-native brown widow spider (Latrodectus geometricus) and three native black widows (L. hespersus, L. mactans, L. variolus) in North America and gauge the importance of urbanization on the geographic ranges of widows at a continental scale. We also quantify the geographic overlap of L. geometricus with each native widow to assess potential species and regions at risk of ecological impact. Consistent with our hypothesis, we find that the distribution of L. geometricus is strongly constrained to urban environments, while native widow distributions are more strongly driven by climatic factors. These results show that urbanization plays a significant role in facilitating the success of invasion, weakening the significance of climate on the realized niche in its invaded range.
Introduction
The Anthropocene is characterized by human impacts driving shifts in ecological processes, with biological invasions ranking as one of the major threats to biodiversity (Sala et al., 2000; Simberloff et al., 2013; Pievani, 2014; Russell and Kueffer, 2019; Pyšek et al., 2020). Global trade is responsible for the movement of species from their native range into novel habitats, leaving native communities vulnerable to ecological impacts (Hastings et al., 2004; Olden, 2006; von der Lippe and Kowarik, 2008; Aronson et al., 2014). Geographic distributions of invasive species can be used to characterize the ecological niche and predict the risks of future invasions across spatial scales (Graham et al., 2004; Broennimann et al., 2007; Warren et al., 2014; Atwater, 2018). The ecological niche is generally a set of biotic and abiotic conditions that allow a species to maintain a viable population within some environmental or geographic limit (Peterson, 2011). A fundamental assumption in predictive modeling of ecological niches is that these sets of conditions are conserved over space and time (Pearman et al., 2008). Recent studies have found that invasive species are likely to conserve their climatic niche (i.e., climatic requirements for survival) during invasion (Petitpierre et al., 2012; Liu et al., 2020). However, previous studies have described the possibility of climatic niches shifting when exposed to novel conditions, reducing the predictability of invasion risk (Broennimann et al., 2007; Pearman et al., 2008; Bowler et al., 2020). A key, and likely confounding, aspect that is lacking in our understanding of biological invasions is the influence of human disturbance on the establishment and spread of species in their introduced range. Recent surges in availability of large-scale datasets of anthropogenic disturbance provide the opportunity to study the role of humans in aiding this process (Balk et al., 2018). Insights from such studies could have widespread implications for predicting invasion risks and impacts, especially of invasive synanthropic species that live in close association with humans.
Generalist arthropod predators have rapid and complex interactions with native communities when introduced into novel environments relative to specialist counterparts (Snyder and Evans, 2006; Crowder and Snyder, 2010). They link trophic levels by feeding on herbivores, detritivores, other predators, plant products, and as prey themselves. Previous studies have shown harmful, beneficial, and mixed impacts of these predators within the same native communities. For example, the introduction of Chinese mantids (Tenodera sinensis) in Delaware, United States, induced fleeing responses in other predators, leading to the displacement of native wolf spiders from the community, which should have directly benefited grazing insects by removing a prominent predator, promoting over-grazing of the plant community (Moran et al., 1996). However, mantids consume more than the predators they displaced, significantly reducing biomass of herbivores and indirectly increasing biomass of plants and altering top-down control (Moran et al., 1996). Introduced arthropod predators can also affect communities by introducing new pathogens, disrupting mating systems, inducing behavioral responses in native species, and further cascading impacts (Snyder and Evans, 2006). Given the complex and potentially adverse effects of invasive arthropod predators, there is a pressing need for approaches to monitor impacts of ongoing invasions. Studying the geographic extent of invasions at large spatial scales provides an opportunity to understand these processes that could have crucial implications for predicting invasion risk and, ultimately, protecting native communities.
Widow spiders of the genus Latrodectus (Araneae: Theridiidae) have historically been studied for their cannibalistic sexual behavior, but they are also vital native predators of many arthropods in their natural ecosystems, including the invasive and venomous fire ant, Solenopsis invicta (Nyffeler et al., 1988; Andrade, 1996; Segoli et al., 2006, 2008a,b; Vetter and Isbister, 2008; Vassilevski et al., 2009; Yan and Wang, 2015). They are regarded as pests due to their potent venom and because several species are considered synanthropic, constructing webs on human-made structures. They are widely feared by the public, in part due to the media sharing false and sensationalistic information about widow spiders, as was noted for the Mediterranean black widow, L. tredecimguttatus (Mammola et al., 2019). Synanthropic widow species are commonly transported and introduced to novel regions, but brown widows (L. geometricus) have had the clearest success in range expansion and establishment globally (Garb et al., 2004). The mechanisms responsible for their success at establishing a cosmopolitan distribution remain poorly understood.
Latrodectus geometricus is native to southern Africa and have drastically expanded its range, colonizing every continent other than Antarctica (Garb et al., 2004). Their spread in North America has been documented for decades, beginning with the first record in Florida in 1935 (Pearson, 1936; Brown et al., 2008), although they were not commonly found in the state until the 1960s, when they became abundant in coastal cities (Levi, 1959; McCrone and Stone, 1965; Vincent et al., 2008). Their presence was restricted to peninsular Florida for decades before expanding their range throughout southeastern United States beginning in the late 1990s, with confirmed occurrence records now reaching as far north as Connecticut (Vincent et al., 2008; Vetter et al., 2012). By 2008, several populations had also established in southern California, with museum specimens verifying their first record in the state in 2003. As a relatively well-documented introduced species in North America that relies on human development for shelter and dispersal, L. geometricus serve as a model for understanding the ecological impact of invasive generalist predators on native communities and the role of urbanization on their establishment and spread.
North America is also home to four native widow species: western (L. hesperus), southern (L. mactans), northern (L. variolus), and red (L. bishopi) widows. Black and brown widows are large-range, generalist predators, and generally prefer low, dark corners of low-traffic areas of residences to build their distinctive tangle-web shelters (Vetter et al., 2016). Latrodectus geometricus almost exclusively reside in the presence of humans, including in their native range, whereas black widows opportunistically use urban structures for shelter while maintaining populations in their native habitats (Lamoral, 1968; Johnson et al., 2012; Schraft et al., 2021). Previous experimental studies have demonstrated that L. geometricus show significant preference for establishing tangle-web refugia on rough surfaces and tight spaces with acute, 30° angles that are more frequently observed in urban architecture than in nature (Gutiérrez-Fonseca and Ortiz-Rivas, 2014; Vetter et al., 2016). Unlike their relatives, red widows (L. bishopi) do not live with humans at all, and are endemic to sand pine scrub habitats in Florida where they build retreats almost exclusively on saw palmetto (Serenoa repens) (Carrel, 2001; Carrel and Deyrup, 2014). Native black widows are potentially at risk of ecological impact from L. geometricus invasion because similarities in niche requirements increases the chance of competitive biotic encounters. For example, urban populations of L. hesperus in southern Californian (Vetter et al., 2012) and L. mactans in Jamaica (Baerg, 1954) show evidence of displacement, but little is known about the magnitude or extent of future impact as L. geometricus expand their geographic range.
Here we use species distribution models (SDMs) to extrapolate the geographic range of invasive L. geometricus and native black widows with similar range size (L. hesperus, L. mactans, and L. variolus) to compare the impact of urbanization on their distributions on a continental scale. To test this, we compared the species’ distributions using a climatic SDM model, which includes climate variables alone, and an anthropogenic model, which incorporates human population density as an urban variable, in addition to climate. If the geographic extent of any given species shows limitation to urban centers in the anthropogenic model, then its range is assumed to be limited by human activity. We refer to this as the “synanthropic invasion hypothesis”. This hypothesis predicts two fundamental patterns, first that L. geometricus’ geographic range will be strongly limited by human population density, and second that black widow distributions to be more strongly influenced by climate variables and will therefore show weak constraint to urban centers under the anthropogenic model. We propose three possible mechanisms to explain this pattern: urban heat island effect, homogenization of landscapes, and microhabitat selection. We expect urban microhabitat preferences provide a sheltering effect for L. geometricus, thereby reducing the significance of climate on their geographic distribution compared to native species and facilitating their ability to establish and expand in novel environments. We also quantify the extent of geographic overlap of L. geometricus with each native widow to identify potential species and regions at risk of ecological impact. For invasive species, time of residence has a positive correlation with range size (Wilson et al., 2007; Williamson et al., 2009; Schmidt et al., 2017). Because brown widows have been established for a longer period in the southeastern extent of the continent, they have had time to grow viable populations and locally expand their range, so we expect more extensive ranges for them in these regions. We therefore hypothesize that L. geometricus will have the strongest overlap with L. mactans.
Materials and Methods
Occurrence Data
We compiled a dataset of 6,703 georeferenced occurrences for all four species (Table 1). We collected presence-only data from GBIF (Global Biodiversity Information Facility, GBIF.org, 2020), which compiles occurrence records from museum collections and online community science repositories: iNaturalist and BugGuide. Data quality from these repositories have been criticized for misleading identifications of species. To moderate this concern, occurrences from these sources that were far outside of previously established ranges (Wang et al., 2018; Schraft et al., 2021) were visually verified by one of the authors (MS) on the original online post and eliminated were if they could not be confirmed. We also compiled data directly from community submissions from Facebook groups (“Spider/Bug Questions with TheBugGirl” and “Spider and Insect Enthusiast”) and Reddit (“r/whatsthisbug” and “r/spiderbro”). We included all occurrences with sufficient collection data that we were able to positively identify based on the photograph. The rest of our samples were generously provided by contacts at extension offices in Oklahoma and Texas, and opportunistic field collections in Oklahoma, Florida, and Arizona. Museum records with adequate locality information but no coordinates were georeferenced using the GEOLocate Web Application.
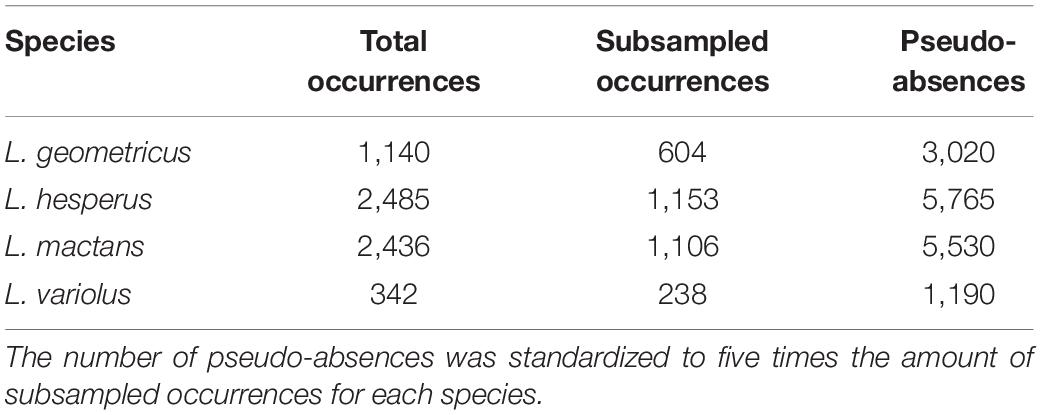
Table 1. Counts for total occurrence points collected, systematically subsampled points, and pseudo-absences retained for model evaluation for each species.
Clustered occurrences were systematically subsampled to a single point per cell on a 10 × 10 km grid to reduce the effects of sampling bias on model performance (Fourcade et al., 2014). Following systematic subsampling, we retained 3,101 total points for climatic model evaluations (Figure 1 and Table 1).
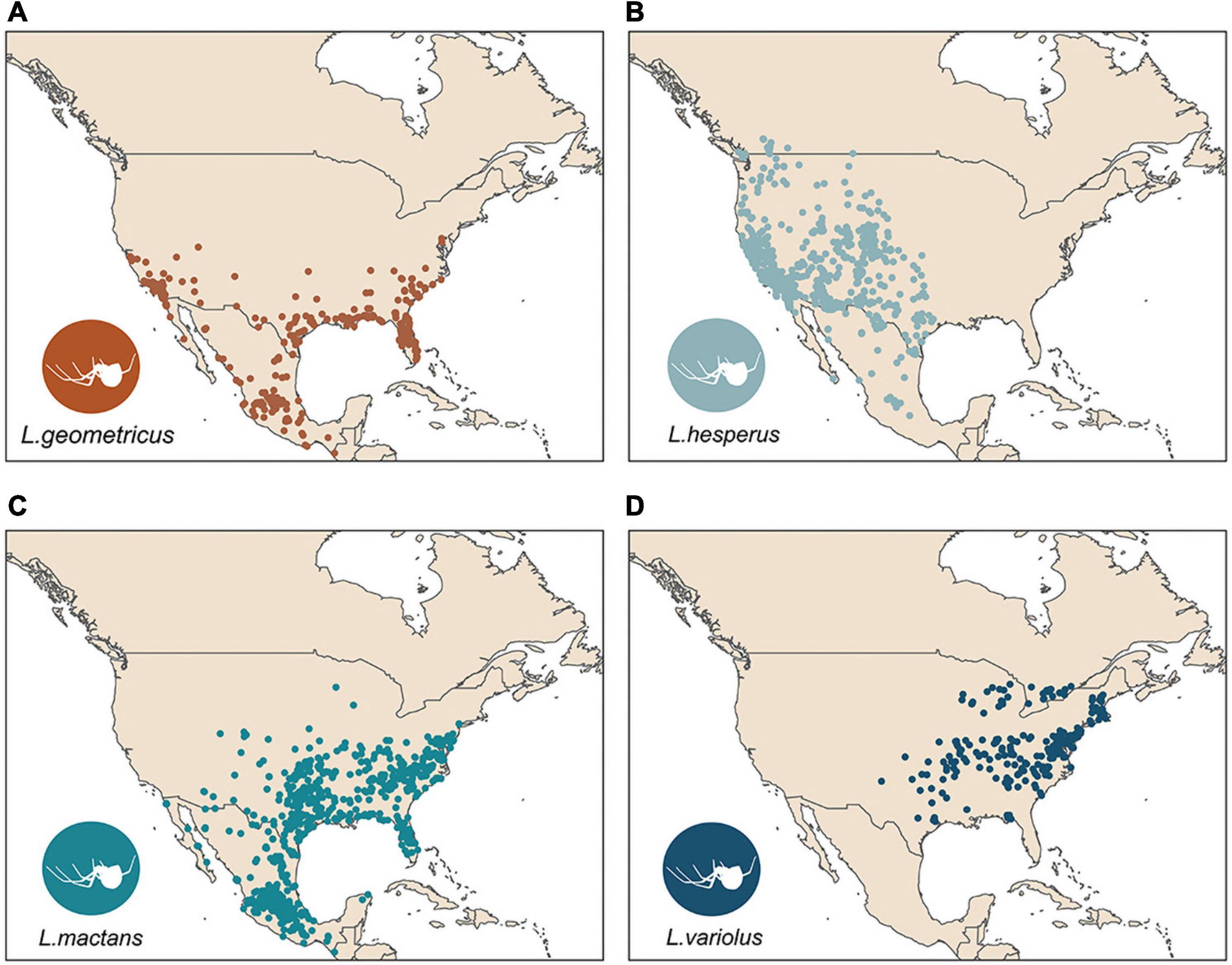
Figure 1. Subsampled occurrence points in North America used for SDMs for L. geometricus (A), L. hesperus (B), L. mactans (C), L. variolus (D).
Environmental Variables
We obtained CHELSA Bioclim variables, averaged from 1973 to 2013, from Paleoclim.org (Karger et al., 2017; Brown et al., 2018), where they had been rescaled to 2.5 arc-min resolution. We initially selected the most biologically significant variables a priori based on previous niche studies on spiders (Saupe et al., 2011; Taucare-Ríos et al., 2016; Wang et al., 2018). To minimize variable collinearity, we ran a principal component analysis (PCA) to reduce the pool of factors. Our final selection of bioclimatic variables include: isothermality (Bio3), temperature seasonality (Bio4), maximum temperature of warmest month (Bio5), minimum temperature of coldest month (Bio6), annual precipitation (Bio12), precipitation of the warmest quarter (Bio16), and precipitation of the coldest quarter (Bio17).
To assess the importance of humans on the distribution of widows, we further included human population density variables as proxies for urbanization. Using combined census and satellite-derived rasters of human demographics is a consistent way to estimate urbanization across space and time (Balk et al., 2018). We downloaded human population density data for the years 2000 and 2020 at 2.5 arc-min resolution from the NASA GPW v4, Gridded Population of the World, dataset built using remote-sensing data in conjunction with census data from the U.S. Bureau of the Census and other global intelligence agencies (Center for International Earth Science Information Network - CIESIN - Columbia University, 2016). We used the 2020 NASA population density raster as a contemporary urban factor and created a raster for the change in human population between 2000 and 2020 to include population growth as a factor in widow range limitations.
Species Distribution Modeling
SDMs are quantitative tools that combine species occurrence data with relevant environmental variables to predict species habitat suitability across geographic space (Elith and Leathwick, 2009; Merow et al., 2013). To assess the importance of urbanization on geographic range of native and invasive widows, we ran two models to compare per species: first using climatic variables alone, and then using climatic and human population density variables.
We modeled our species distributions using Maxent 3.4.1. There are many software that extrapolate SDMs, but we chose Maxent because its prediction accuracy has been shown to outperform other methods given presence-only data, even in the case of invasion modeling (Elith et al., 2006, 2011; Mothes et al., 2019). Maxent is a machine-learning method that estimates species ranges by finding the probability distribution of maximum entropy (Elith et al., 2006). We performed all analyses in R version 3.6.1, using the package “ENMeval” (Muscarella et al., 2014) to run and evaluate Maxent models. We partitioned training data using 10-fold cross-validation and ran models across multiple user-tuned parameters. We tuned our parameters by running multiple models across every combination of three feature classes, which determine the potential shape of the response curves (L—linear, LQ—linear-quadratic, and LQP— linear-quadratic-product), and a series of regularization multipliers (1, 1.5, 2, 2.5, 3) to smooth the model response and avoid overfitting of testing data (Elith et al., 2011; Muscarella et al., 2014).
Of the resulting 15 model outputs, we first selected the best-fit model, indicated by a deltaAIC score of 0. Use of AUC has been criticized as a means of comparing model performance, however, in this study we are only using this metric to evaluate whether selected best-fit models performed above an acceptable (0.7) threshold (Lobo et al., 2008; Fourcade et al., 2014). Furthermore, we used multiple performance metrics to evaluate our best-fit models: AUC, AIC, AICc, and Boyce Index (BI). BI was calculated using “ecospat” package on R, and values range from –1 to 1. Positive BI values suggest predictions are consistent with the distribution presence data, values close to zero suggest results are no different from a random model, and negative values suggests no predicted occurrence in areas with actual presences (Boyce et al., 2002; Hirzel et al., 2006). Habitat suitability scores for range maps were rescaled to a clog-log scale of 0–1, where 0 denotes unsuitable habitat and 1 indicates the complete habitat suitability.
We calculated the difference in area between climate and anthropogenic models for each species as a measure of magnitude of range restriction to urban regions. This metric was measured by first quantifying the area, in km2, of moderate-to-high habitat suitability (suitability threshold set to > 0.5) in both models. Next, we calculated the percent change in area between the climate-only and anthropogenic models of each species.
We evaluated the importance of each variable using jackknife analyses to generate permutation importance measurements. Permutation importance is calculated by randomly arranging values of an environmental variable among points used in the training model, reevaluating the model, and measuring difference in AUC between the training and random model. A greater decrease in AUC suggests a greater impact on model performance. Preliminary analyses showed minimal permutation importance of change in population density between 2000 and 2020 (Supplementary Table 1), so we did not include this variable in our models.
To ensure reproducibility (Zurell et al., 2020) we have included a standardized ODMAP report of SDM parameters and assumptions in Supplementary Material.
Geographic Overlap Analysis
To identify the geographic extent of ecological impact of L. geometricus on native widows, we quantified geographic overlap of L. geometricus with each native widow species using their projected distributions under the anthropogenic model. Here, areas of geographic overlap indicate regions of more probable co-occurrence, and thus a heightened opportunity for antagonistic encounters and competition. Overlap analyses use projections of two species’ niches, in the case of geographic overlap using SDM outputs, to count the number of shared cells, resulting in a niche similarity measurement. Geographic overlap analyses were performed using “ENMTools” package (Warren et al., 2021) to obtain two overlap metrics: Schoener’s D and similarity index I, proposed by Warren et al. (2008). Schoener’s D remains the most commonly used metric of geographic overlap and has been demonstrated to be a reliable measure of niche similarity in environmental space (Brown and Carnaval, 2019). Though results for these metrics rarely differ qualitatively, ecological implications of Schoener’s D, which is traditionally used to measure niche similarity of microhabitats and diet, can be misleading with SDMs (Warren et al., 2008). Because we are concerned with the geographic extent of overlap, rather than similarities in niche requirements, we also include Warren’s I, which does not assign biological meaning to the probability of distributions and is specific to geographic space (Warren et al., 2008). Warren’s I is a variation of the Hellinger (H) metric, which measures distance between two geographic extents, and is calculated as one minus H (Warren et al., 2008). Schoener’s D, on the other hand, is calculated by subtracting the total variation distance between two niches by one (Schoener, 1968). Both metrics range from 0 (no overlap) to 1 (complete overlap).
To narrow down regions of potential impact, as well as regions safe from impact, we also overlaid maps for each species with L. geometricus and regions of overlap with moderate-to-high (threshold set to > 0.5) habitat suitability (Supplementary Figure 1).
Results
Overall, we found that L. geometricus show strongest constraint to urban environments, consistent with our hypothesis of synanthropic invasion. Unexpectedly, L. hesperus show moderate constraint to urban habitats, suggesting they have the strongest synanthropic association to humans of the native species. We also found strong geographic overlap between these two species, which in conjunction with shared preferences for urban environments, leaves L. hesperus at greatest risk of ecological impact by L. geometricus invasion.
Species Distribution Models
Climate-Only Model
While habitat suitability varies across the study region for each species, all species ranges are characterized by large extents of high habitat suitability (>0.75) that correspond to expected distributions (Figure 2). Areas of high habitat suitability for L. geometricus primarily occur along coastlines, especially along the west coast (Figure 2). L. hesperus has high habitat suitability along the west coast and expanding west to Oklahoma and as far north as southern Alberta (Figure 2). L. mactans optimal habitat are in southcentral Mexico and reaching east to Florida, but they are restricted to the southern regions of North America (Figure 2). The northeast has the most suitable habitat for L. variolus (Figure 2). Models showed low suitable habitat in the Rockies for L. variolus.
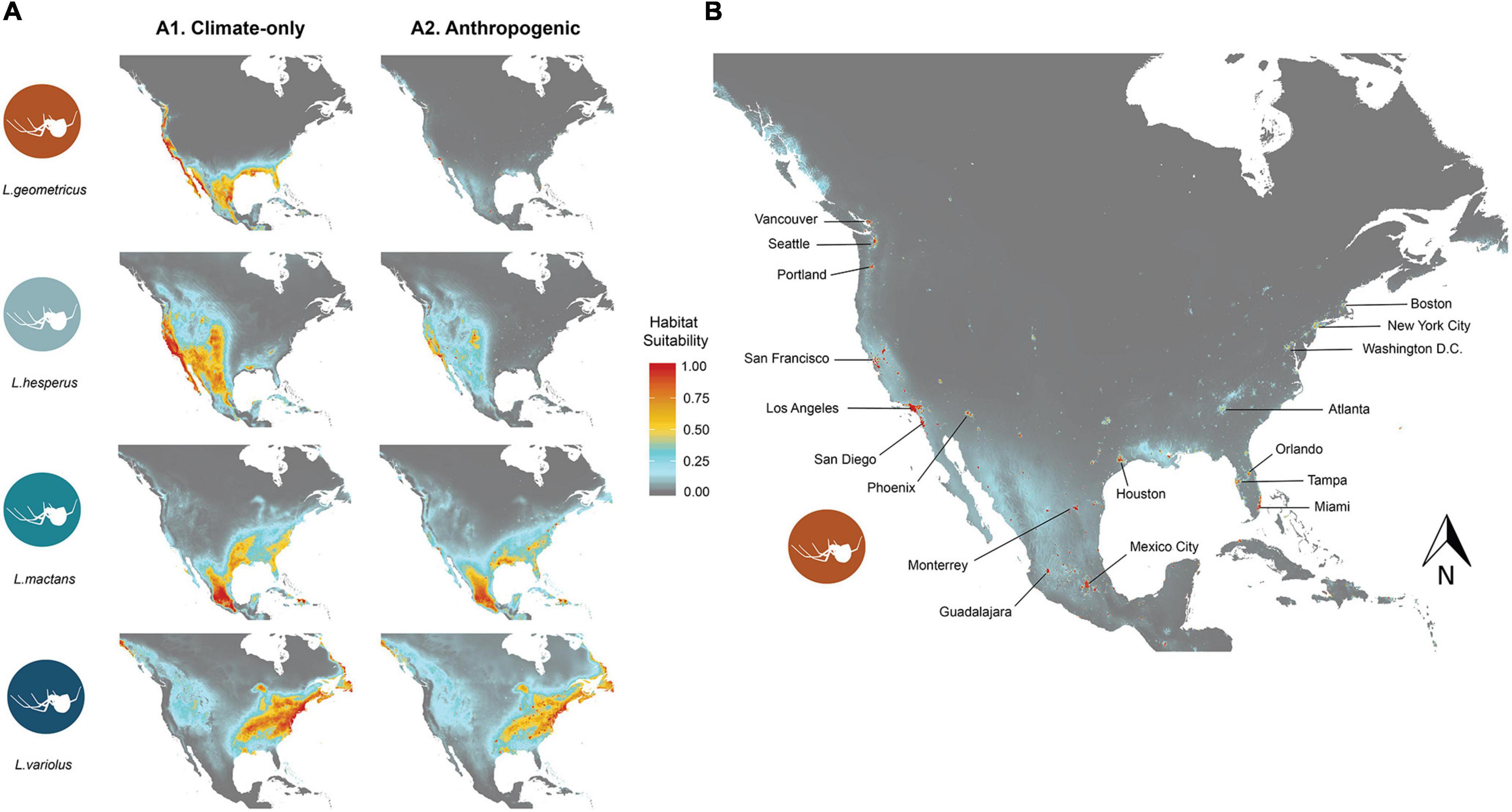
Figure 2. (A) Displays the distribution of habitat suitability under climate-only models (a) using climate variables alone, and anthropogenic models (b) using climate and human population density variables for L. geometricus (i), L. hesperus (ii), L. mactans (iii), and L. variolus (iv). A closer look at the distribution of L. geometricus under the anthropogenic model in (B) shows a restriction of habitat suitability to urban regions. Habitat suitability ranges from 0 (gray), which indicates no suitable habitat, to most suitable habitat 1 (red). Areas with low-to-moderate suitability are pictured in blue, and moderate-to-high are pictured in yellow.
As expected, because of its large range but smaller sample size in this study, L. variolus had the lowest performing model (0.77 AUC). To ensure accurate evaluation, we also report the difference in training and test AUC (AUCdiff), as well as the corrected Akaike information criteria (AICc). These performance metrics also indicated good model performance across species (Table 2). We selected best-fit models with performance metrics deltaAIC of 0 (Muscarella et al., 2014). The estimated optimal climate-only model feature class and regularization multiplier for each species are shown in Table 2. All selected models had fair to excellent performance (0.70 > AUC < 0.90; 0.85 > BI) (Araújo et al., 2005).
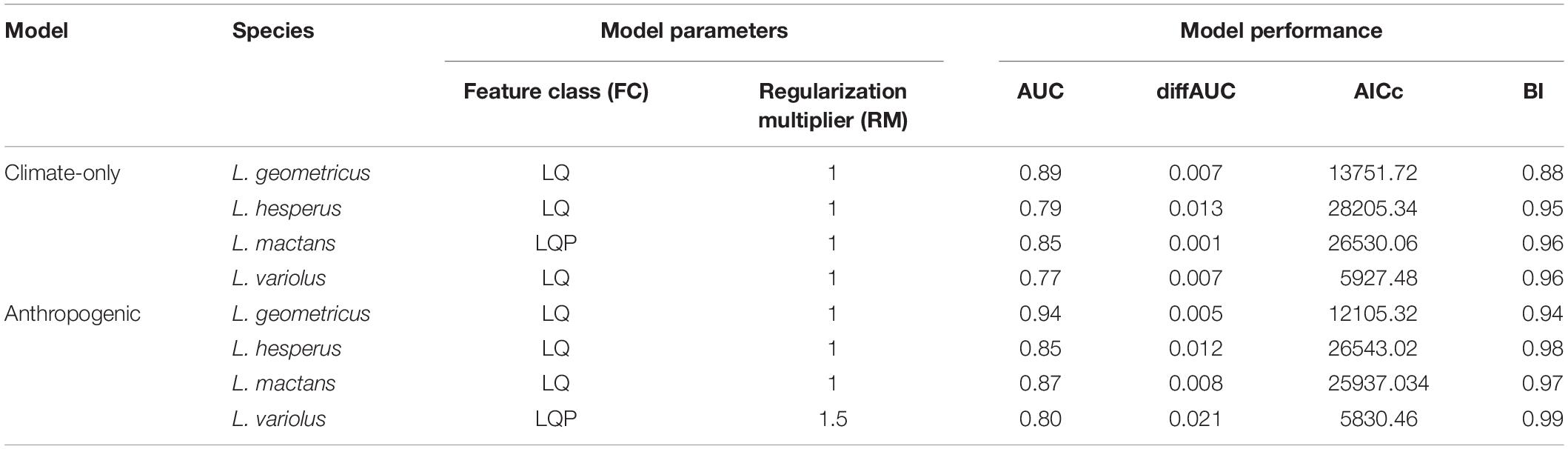
Table 2. Optimal model parameters (feature class, regularization multiplier) and performance metrics (AUC, diffAUC, AICc, BI) for climate-only and anthropogenic models.
Anthropogenic Model
Climate + anthropogenic SDMs (hereafter, anthropogenic models) showed significantly less highly suitable areas (habitat suitability > 0.5) for L. geometricus (94%) and L. hesperus (87%) than those calibrated with climate only. For L. mactans and L. variolus, adding human population density resulted in much less change, with L. mactans and L. variolus showing only a 21 and 19% reduction in range size, respectively. Areas of high habitat suitability for L. geometricus are highly restricted to regions with high human population density, corresponding to major urban centers: Los Angeles, San Francisco, Sacramento, San Diego, Miami-Dade, Tampa, Orlando, Mexico City, Guadalajara, and Monterey, with intervening non-urban areas showing a reduction in suitability relative to the climate-only models (Figure 2). Latrodectus hesperus had the greatest reduction of suitable area of the native species. Similar to L. geometricus, their range contracted toward urban centers in the anthropogenic model. Areas of habitat suitability for L. mactans and L. variolus remained like their projected ranges in the climate-only models (Figure 2).
All selected models had fair to excellent performance (0.70 > AUC < 0.90; 0.85 > BI) (Araújo et al., 2005). The estimated optimal anthropogenic model parameters and performance metrics for each species are shown in Table 2.
Variable Importance
Jackknife analyses of permutation importance show that, consistent with our hypotheses, human population density is the most important variable in defining the range of the invasive widow, while native species distributions are primarily predicted by climatic variables. Human population density explained over 40% of the distribution of L. geometricus, but less than 20% for L. hesperus and L. variolus, and less than 5% for L. mactans (Table 3). The highest contributing climatic variables for each native species in the anthropogenic model were: isothermality (30.4%) for L. hesperus, temperature seasonality (40.7%) for L. mactans, and precipitation of the driest quarter (54.0%) for L. variolus (Table 3).
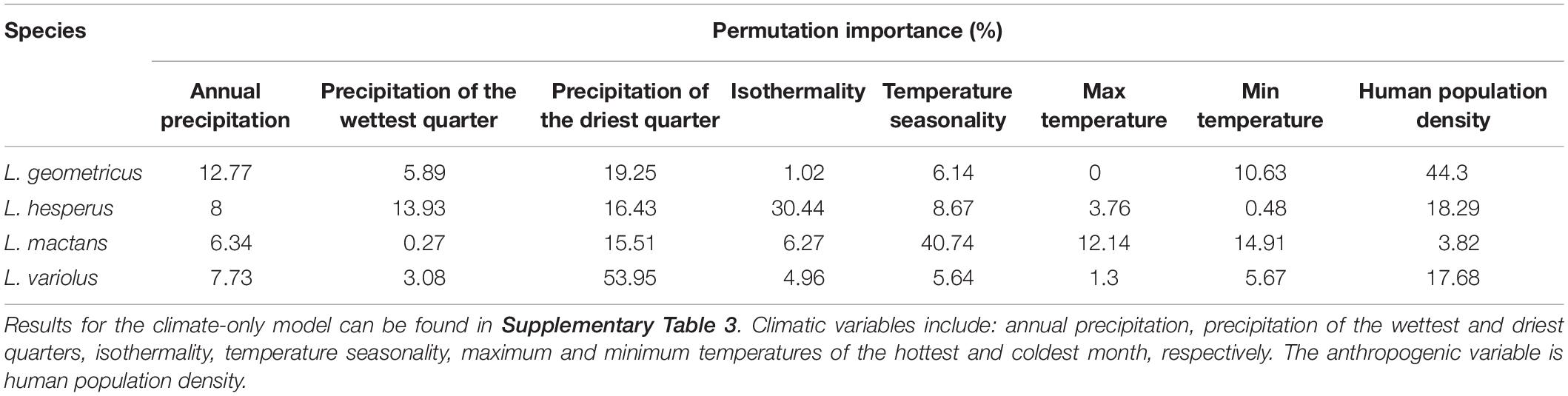
Table 3. Percent permutation importance of climatic and anthropogenic predictors used in the anthropogenic model for each species.
Geographic Overlap
Using both I and D metrics, our results show strongest pairwise comparison of range overlap in the anthropogenic model to be between L. geometricus and L. mactans, whose ranges overlap throughout the gulf coast and Mexico (Supplementary Figure 1). Although they can both be found throughout these regions, L. geometricus have a patchy distribution restricted to cities, while L. mactans that have a more continuous range widely distributed outside of regions of high human population density (Figure 2 and Supplementary Figure 1). Ranges of both L. hesperus and L. geometricus show constraint to urban centers in California, where they strongly overlap (Figure 2 and Supplementary Figure 1). L. variolus shows limited overlap with L. geometricus in southeastern United States, excluding Florida (Table 4).
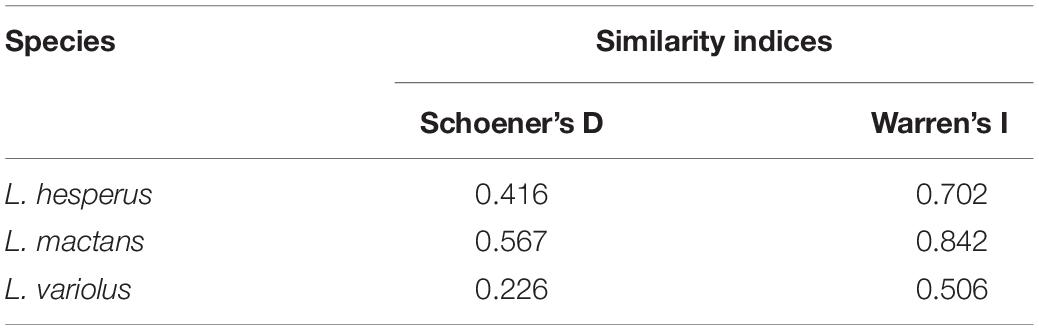
Table 4. Similarity indices, Schoener’s D and Warren’s I, ranging from 0 (no overlap) to 1 (complete overlap) of species geographic overlap with L. geometricus.
Discussion
Widow spiders in North America provide unique insights for addressing the role of humans on invasion success of an invasive generalist predator with close relatives throughout its introduced range. Invasion success for species with close associations to people is aided by repeated introductions from global trade routes lessening dispersal limitations and increasing propagule pressure in urban ports of entry. Still, humans can also aid in establishment success by sheltering species from climatic conditions that might lie beyond their climatic niche. In this study, we considered the role of urban environments, in addition to climate, in shaping the distribution of non-native compared to native widows and compared geographic overlap of L. geometricus with each black widow species to assess potential areas and species at risk of ecological impact. Consistent with our “synanthropic invasion hypothesis,” we found that the geographic distribution of L. geometricus is patchy and more strongly constrained to urban environments, while native widows have more continuous distributions that are limited by climatic factors.
Using geographic overlap as a proxy for negative interactions between species, these findings suggest reduced risk of ecological impact on native widows overall because areas of overlap are more highly constrained to urban environments. This leaves populations of black widows in their native habitats outside of urban areas at low risk of co-occurrence. Therefore, we also considered areas where they do not overlap as regions of sanctuary from impact in our risk assessment. We expected that there would be the strongest overlap with L. mactans because of the longer establishment period of L. geometricus within their range in southern North America beginning in the 1940s. Overlap maps, in conjunction with D and I metrics, confirmed geographic overlap of L. geometricus was strongest with L. mactans. However, their range also has large, continuously distributed areas of highly suitable habitat outside of major urban centers to maintain viable populations, buffering the risk of impact.
Unexpectedly, we found that L. hesperus showed strong overlap as well (Table 4). In addition, their patchy distribution in the Anthropogenic model suggests they find urban habitats highly suitable (Figure 2). This increases the possibility of co-occurrence with L. geometricus given reduced areas in their native habitat for maintaining viable populations safe from antagonistic encounters. Empirical studies demonstrating impact are lacking, however, L. geometricus were found to significantly outnumber and produce more egg sacs than L. hesperus at sampled sites in urban Southern California, showing evidence for risk of displacement (Vetter et al., 2012). Furthermore, L. geometricus may also experience competitive release within their shared range with L. hesperus. While they are impacted by parasitoid wasps of L. mactans in their southern range, they are not fed upon by Pseudogaurax signatus, a fly that commonly infests L. hesperus egg sacs (Vetter et al., 2012). Because of the apparent constraint to urban environments, the strong signal of overlap, and higher potential for competitive release, L. hesperus are at greatest risk of ecological impact from the invasion of L. geometricus in North America. Still, range restriction of L. geometricus to urban habitats suggests impact on native species is likely to be reserved to urban populations.
The strength of human population density as a predictor of L. geometricus presence in their invaded range indicates urbanization is a potential driver for invasion success of the species. Although the mechanism remains unclear, we identified three possible mechanisms that are not mutually exclusive: the urban heat island effect, which reflects locally favorable climatic conditions in urban areas; homogenization of landscapes, which decreases biotic resistance; and microhabitat selection, which limits species to specific local conditions for shelter and may advantage some species over others. Urban heat islands are cities that have more elevated temperatures than surrounding areas (Oke, 1982; Kim, 1992), potentially allowing species to inhabit regions that are colder than predicted by their climatic niche, sheltered by the warming effects of urban heat (Murakami et al., 2007; Sołtysiak, 2020). Although urban heat island effect may influence establishment success of L. geometricus, particularly in Denver, New York City, and up to Boston, it does not explain why they are not occupying non-urban areas in southern North America that fall within their climatic needs.
The two remaining mechanisms both relate to habitat. The second potential mechanism explaining this restricted range pattern may be that urbanization homogenizes landscapes, decreasing biotic resistance and making these environments more easily invadable by introduced species (McKinney, 2006; La Sorte et al., 2007; Shochat et al., 2010; Aronson et al., 2014). Availability of similar habitats globally enables range expansion by facilitating establishment once the species is introduced to a novel region. Under this mechanism, L. geometricus would be restricted to urban areas because they fail to establish outside of urban areas where biotic resistance is higher. Within these homogenized landscapes, micro-habitat selection is a likely mechanism that addresses range-restriction for these spiders via positive selection of urban habitats. One of the many ways spiders use silk is to create complex web structures that are used as temporary or permanent shelters. The characteristic tangle-web of female widow spiders is used for both shelter and prey capture. As generalist predators that feed on a variety of invertebrates and occasional small vertebrates, their webs are structured with different silk lines used to capture both aerial and ground-dwelling prey. Web-building is energetically costly, especially for widow spiders that build semi-permanent webs. Preferences for web-site features thus have a strong effect on widow spider fitness and are heritable (Salomon et al., 2010; Gutiérrez-Fonseca and Ortiz-Rivas, 2014). Selection of web-site microhabitats is therefore likely an important predictor of their niche that would restrict habitat suitability to regions that support conditions suitable for shelter.
An experimental study found that L. geometricus prefer web-sites with deep cavities and 30° or 90° angles, a prominent feature of urban architecture, because these angles fit the conical retreats of L. geometricus web structures (Vetter et al., 2016). Unlike black widows, they also prefer web-sites that have been previously occupied by conspecifics (Vetter et al., 2016). In the field, brown widows are often found under objects with minimal but strong material cover that contain 30°/90 angles, such as between fencing, in gaps of objects like trash bin handles, and under tables and chairs with solid tops (Vetter et al., 2012). In their native range, L. geometricus are also highly synanthropic. There, they are distinguished from another widow species native to South Africa, L. indistinctus, by their predilection for residing on garden furniture over grass and shrubbery in veld habitats (Muller, 1993). Synanthropic adaptation such as this in their native range shows a tendency for using human-made objects for web-site construction that could predispose the species to fare well under urban conditions in an introduced environment (Simberloff et al., 2012). Microhabitat selection also shapes the restricted range of some conspecifics, including the red widow, which builds its webs exclusively on the endemic saw palmetto (S. repens) of the Florida sand pine scrubs (Carrel, 2001; Carrel and Deyrup, 2014). Given strong enough preferences, web-site selection may be an important range-limiting factor for some spiders, including L geometricus. Although the actual mechanisms behind this pattern were not uncovered in this study, invasion success of L. geometricus appears to be facilitated by urbanization, minimizing the importance of the climatic niche on survival and facilitating spread and establishment of the species in North America.
Widow spiders are one of the most distinguishable spiders, yet, like many other invertebrates, sampling efforts from online data repositories present a challenge in ensuring representation. Most occurrence data for this study were acquired from GBIF, an online database that compiles museum and community science data acquired iNaturalist and BugGuide. Though these data repositories are the best we have for large-scale studies of many invertebrates, and are valuable for the breadth of area sampled, they are still limited by species misidentifications, and sampling that is unstandardized and geographically biased toward sites that are more accessible to people (e.g., roads, trails, backyards). Sampling efforts made by natural history collections tend to be biased to the geographic scope of individual collections, so we incorporated specimens from multiple museum collections to improve accuracy of our models (Ferro and Flick, 2015). Community science efforts are especially controversial for acquiring quality data due to the lack of representativeness across species distributions and misidentifications of species (Ferro and Flick, 2015; Zhang and Zhu, 2018). To moderate this concern, we performed data quality checks by manually verifying identifications from photographs and systematically subsampling occurrence data on a 10 × 10 km grid to reduce spatial bias around more populated areas. We note that although community sampling efforts are patchy and ad hoc, they show a relatively robust sampling across the entire distribution of each species because widows are widespread, abundant spiders of particular interest to the community due to their venomous bite.
With rates of urbanization and trade of goods increasing globally, introduction and establishment of invasive species are becoming a greater risk to biodiversity and are a growing threat to local economies (Diagne et al., 2021). Descriptions of the distributions of invasive species have historically focused on the climatic niche to predict geographic ranges (Fourcade et al., 2017). This is in part due to limited availability of biotic and non-climatic abiotic data at appropriate resolutions for continental and global-scale modeling. In recent years, advances in technologies in remote sensing and statistical methods have led to an increase in available human activity data. In this study, we observed drastic differences in the implications of invasion risk when incorporating anthropogenic predictors. When modeling using climatic variables alone, signals for invasion risk are much stronger, but insights on L. geometricus’ urban range restriction reveal minimal impact as long as native habitats remain intact. Looking forward, anthropogenic activity data provide an opportunity to estimate disturbance and expand beyond climatic predictors for modeling distributions, proving to be an invaluable tool for predictive modeling of invasive spread to forecast regions and species at risk of impact.
Data Availability Statement
The original contributions presented in the study are included in the article/Supplementary Material, further inquiries can be directed to the corresponding author/s.
Author Contributions
MS collected data and ran analyses. MS led the writing with input and revisions by KM. Both authors conceived the study.
Funding
This research was supported by funding from the OU Graduate Adams Research Scholarship.
Conflict of Interest
The authors declare that the research was conducted in the absence of any commercial or financial relationships that could be construed as a potential conflict of interest.
Publisher’s Note
All claims expressed in this article are solely those of the authors and do not necessarily represent those of their affiliated organizations, or those of the publisher, the editors and the reviewers. Any product that may be evaluated in this article, or claim that may be made by its manufacturer, is not guaranteed or endorsed by the publisher.
Acknowledgments
We thankfully acknowledge the valuable insights and support from Drs. Lara Souza and Jeffrey Kelly. We also thank Dr. Claire Curry and Elyse Ellsworth for analytical assistance. We thank to Andrea Haberkern for admission to collect community submissions from “Spider/Bug Questions with TheBugGirl” Facebook community. We also thank Cayley Buckner, Madison Heisey, and Laura Gatch for specimen donations.
Supplementary Material
The Supplementary Material for this article can be found online at: https://www.frontiersin.org/articles/10.3389/fevo.2021.757902/full#supplementary-material
References
Andrade, M. C. B. (1996). Sexual selection for male sacrifice in the australian redback spider. Science 271, 70–72. doi: 10.1126/science.271.5245.70
Araújo, M. B., Pearson, R. G., Thuiller, W., and Erhard, M. (2005). Validation of species-climate impact models under climate change. Glob. Change Biol. 11, 1504–1513. doi: 10.1111/j.1365-2486.2005.01000.x
Aronson, M. F. J., La Sorte, F. A., Nilon, C. H., Katti, M., Goddard, M. A., Lepczyk, C. A., et al. (2014). A global analysis of the impacts of urbanization on bird and plant diversity reveals key anthropogenic drivers. Proc. R. Soc. B. 281:20133330. doi: 10.1098/rspb.2013.3330
Atwater, D. Z. (2018). Climatic niche shifts are common in introduced plants. Nat. Ecol. Evol. 2, 34–43. doi: 10.1038/s41559-017-0396-z
Baerg, W. J. (1954). The brown widow and the black widow spiders in jamaica (Araneae, Theridiidae). Ann. Entomol. Soc. Am. 47, 52–60. doi: 10.1093/aesa/47.1.52
Balk, D., Leyk, S., Jones, B., Montgomery, M. R., and Clark, A. (2018). Understanding urbanization: a study of census and satellite-derived urban classes in the United States, 1990-2010. PLoS One 13:e0208487. doi: 10.1371/journal.pone.0208487
Bowler, D. E., Bjorkman, A. D., Dornelas, M., Myers-Smith, I. H., Navarro, L. M., Niamir, A., et al. (2020). Mapping human pressures on biodiversity across the planet uncovers anthropogenic threat complexes. People Nat. 2, 380–394. doi: 10.1002/pan3.10071
Boyce, M. S., Vernier, P. R., Nielsen, S. E., and Schmiegelow, F. K. A. (2002). Evaluating resource selection functions. Ecol. Model. 157, 281–300. doi: 10.1016/S0304-3800(02)00200-4
Broennimann, O., Treier, U. A., Müller-Schärer, H., Thuiller, W., Peterson, A. T., and Guisan, A. (2007). Evidence of climatic niche shift during biological invasion. Ecol. Lett. 10, 701–709. doi: 10.1111/j.1461-0248.2007.01060.x
Brown, J. L., and Carnaval, A. C. (2019). A tale of two niches: methods, concepts, and evolution. Front. Biogeogr. 11:e44158. doi: 10.21425/F5FBG44158
Brown, J. L., Hill, D. J., Dolan, A. M., Carnaval, A. C., and Haywood, A. M. (2018). PaleoClim, high spatial resolution paleoclimate surfaces for global land areas. Sci. Data 5:180254. doi: 10.1038/sdata.2018.254
Brown, K. S., Necaise, J. S., and Goddard, J. (2008). Additions to the Known U.S. Distribution of Latrodectus geometricus (Araneae: Theridiidae). J. Med. Entomol. 45, 959–962. doi: 10.1603/0022-2585(2008)45[959:ATTKUD]2.0.CO;2
Carrel, J. E. (2001). Population dynamics of the red widow spider (Araneae: Theridiidae). Fla. Entomol. 84, 385–390. doi: 10.2307/3496497
Carrel, J. E., and Deyrup, M. (2014). Red widow spiders (Araneae: Theridiidae) prey extensively on scarab beetles endemic in Florida Scrub. Fla. Entomol. 97, 130–137. doi: 10.1653/024.097.0155
Center for International Earth Science Information Network - CIESIN - Columbia University (2016). Gridded Population of the World, Version 4 (GPWv4): Population Density. Palisades, NY: NASA, doi: 10.7927/H4NP22DQ
Crowder, D. W., and Snyder, W. E. (2010). Eating their way to the top? Mechanisms underlying the success of invasive insect generalist predators. Biol. Invasions. 12, 2857–2876. doi: 10.1007/s10530-010-9733-8
Diagne, C., Leroy, B., Vaissière, A.-C., Gozlan, R. E., Roiz, D., Jarić, I., et al. (2021). High and rising economic costs of biological invasions worldwide. Nature 592, 571–576. doi: 10.1038/s41586-021-03405-6
Elith, J., Graham, C. H., Anderson, R. P., Dudík, M., Ferrier, S., Guisan, A., et al. (2006). Novel methods improve prediction of species’ distributions from occurrence data. Ecography 29, 129–151. doi: 10.1111/j.2006.0906-7590.04596.x
Elith, J., and Leathwick, J. R. (2009). Species distribution models: ecological explanation and prediction across space and time. Annu. Rev. Ecol. Evol. Syst. 40, 677–697. doi: 10.1146/annurev.ecolsys.110308.120159
Elith, J., Phillips, S. J., Hastie, T., Dudík, M., Chee, Y. E., and Yates, C. J. (2011). A statistical explanation of MaxEnt for ecologists: statistical explanation of MaxEnt. Divers. Distribut. 17, 43–57. doi: 10.1111/j.1472-4642.2010.00725.x
Ferro, M. L., and Flick, A. J. (2015). “Collection Bias” and the importance of natural history collections in species habitat modeling: a case study using Thoracophorus costalis Erichson (Coleoptera: Staphylinidae: Osoriinae), with a Critique of GBIF.org. Coleopt. Bull. 69, 415–425. doi: 10.1649/0010-065X-69.3.415
Fourcade, Y., Besnard, A. G., and Secondi, J. (2017). Paintings predict the distribution of species, or the challenge of selecting environmental predictors and evaluation statistics. Glob. Ecol. Biogeogr. 27, 245–256. doi: 10.1111/geb.12684
Fourcade, Y., Engler, J. O., Rödder, D., and Secondi, J. (2014). Mapping species distributions with MAXENT using a geographically biased sample of presence data: a performance assessment of methods for correcting sampling bias. PLoS One 9:e97122. doi: 10.1371/journal.pone.0097122
Garb, J. E., González, A., and Gillespie, R. G. (2004). The black widow spider genus Latrodectus (Araneae: Theridiidae): phylogeny, biogeography, and invasion history. Mol. Phylogenet. Evol. 31, 1127–1142. doi: 10.1016/j.ympev.2003.10.012
Graham, C., Ferrier, S., Huettman, F., Moritz, C., and Peterson, A. (2004). New developments in museum-based informatics and applications in biodiversity analysis. Trends Ecol. Evol. 19, 497–503. doi: 10.1016/j.tree.2004.07.006
Gutiérrez-Fonseca, P. E., and Ortiz-Rivas, L. (2014). substrate preferences for attaching gumfoot lines in Latrodectus geometricus (Araneae: Theridiidae). Entomol. News 123, 371–379. doi: 10.3157/021.123.0508
Hastings, A., Cuddington, K., Davies, K. F., Dugaw, C. J., Elmendorf, S., Freestone, A., et al. (2004). The spatial spread of invasions: new developments in theory and evidence: spatial spread of invasions. Ecol. Lett. 8, 91–101. doi: 10.1111/j.1461-0248.2004.00687.x
Hirzel, A. H., Le Lay, G., Helfer, V., Randin, C., and Guisan, A. (2006). Evaluating the ability of habitat suitability models to predict species presences. Ecol. Model. 199, 142–152. doi: 10.1016/j.ecolmodel.2006.05.017
Johnson, J. C., Trubl, P. J., and Miles, L. S. (2012). Black widows in an urban desert: city-living compromises spider fecundity and egg investment despite urban prey abundance. Am. Midl. Nat. 168, 333–340. doi: 10.1674/0003-0031-168.2.333
Karger, D. N., Conrad, O., Foppolo, S., Bruban, V., Etienne-Selloum, N., Jung, A. C., et al. (2017). Climatologies at high resolution for the earth’s land surface areas. Sci. Data 4:170122. doi: 10.1038/sdata.2017.122
Kim, H. H. (1992). Urban heat island. Int. J. Remote Sens. 13, 2319–2336. doi: 10.1080/01431169208904271
La Sorte, F. A., McKINNEY, M. L., and Pyšek, P. (2007). Compositional similarity among urban floras within and across continents: biogeographical consequences of human-mediated biotic interchange: intercontinental compositional similarity. Glob. Change Biol. 13, 913–921. doi: 10.1111/j.1365-2486.2007.01329.x
Lamoral, B. H. (1968). On the nest and web structure of Latrodectus in South Africa, and some observations on body colouration of L. geometricus (Araneae: Theridiidae). Anna. Nat. Museum 20, 1–14. doi: 10.1007/s10530-014-0679-0
Levi, H. W. (1959). The spider genus latrodectus (Araneae, Theridiidae). Trans. Am. Microsc. Soc. 78, 7–43. doi: 10.2307/3223799
Liu, C., Wolter, C., Xian, W., and Jeschke, J. M. (2020). Most invasive species largely conserve their climatic niche. Proc. Natl. Acad. Sci. U.S.A. 117, 23643–23651. doi: 10.1073/pnas.2004289117
Lobo, J. M., Jiménez-Valverde, A., and Real, R. (2008). AUC: a misleading measure of the performance of predictive distribution models. Glob. Ecol. Biogeogr. 17, 145–151. doi: 10.1111/j.1466-8238.2007.00358.x
Mammola, S., Milano, F., Vignal, M., Andrieu, J., and Isaia, M. (2019). Associations between habitat quality, body size and reproductive fitness in the alpine endemic spider Vesubia jugorum. Glob. Ecol. Biogeogr. 28, 1325–1335. doi: 10.1111/geb.12935
McCrone, J. D., and Stone, K. J. (1965). The Widow Spiders of Florida. Arthropods of Florida and Neighboring Land Areas. Gainesville, FL: Florida State Collection of Arthropods.
McKinney, M. L. (2006). Urbanization as a major cause of biotic homogenization. Biol. Conserv. 127, 247–260. doi: 10.1016/j.biocon.2005.09.005
Merow, C., Smith, M. J., and Silander, J. A. (2013). A practical guide to MaxEnt for modeling species’ distributions: what it does, and why inputs and settings matter. Ecography 36, 1058–1069. doi: 10.1111/j.1600-0587.2013.07872.x
Moran, M. D., Rooney, T. P., and Hurd, L. E. (1996). Top-down cascade from a bitrophic predator in an old-field community. Ecology 77, 2219–2227. doi: 10.2307/2265715
Mothes, C. C., Stroud, J. T., Clements, S. L., and Searcy, C. A. (2019). Evaluating ecological niche model accuracy in predicting biotic invasions using South Florida’s exotic lizard community. J. Biogeogr. 46, 432–441. doi: 10.1111/jbi.13511
Muller, G. J. (1993). Black and brown widow spider bites in South Africa. A series of 45 cases. S. Afr. Med. J. 83, 399–405.
Murakami, K., Matsui, R., and Morimoto, Y. (2007). Northward invasion and range expansion of the invasive fern Thelypteris dentata (Forssk.) St. John into the Urban Matrix of Three Prefectures in Kinki District, Japan. Am. Fern J. 97, 186–198. doi: 10.1640/0002-8444(2007)97[186:niareo]2.0.co;2
Muscarella, R., Galante, P. J., Soley-Guardia, M., Boria, R. A., and Kass, J. M. (2014). ENMeval: an R package for conducting spatially independent evaluations and estimating optimal model complexity for Maxent ecological niche models. Methods Ecol. Evol. 5, 1198–1205. doi: 10.1111/2041-210X.12261
Nyffeler, M., Dean, D. A., and Sterling, W. L. (1988). The southern black widow spider, Latrodectus mactans (Araneae, Theridiidae), as a predator of the red imported fire ant, Solenopsis invicta (Hymenoptera, Formicidae), in Texas cotton fields. J. Appl. Entomol. 106, 52–57. doi: 10.1111/j.1439-0418.1988.tb00563.x
Oke, T. R. (1982). The energetic basis of the urban heat island. Q. J. R. Met. Soc. 108, 1–24. doi: 10.1002/qj.49710845502
Olden, J. D. (2006). Biotic homogenization: a new research agenda for conservation biogeography. J. Biogeogr. 33, 2027–2039. doi: 10.1111/j.1365-2699.2006.01572.x
Pearman, P. B., Guisan, A., Broennimann, O., and Randin, C. F. (2008). Niche dynamics in space and time. Trends Ecol. Evol. 23, 149–158. doi: 10.1016/j.tree.2007.11.005
Pearson, J. F. W. (1936). Latrodectus geometricus Koch in Southern Florida. Science 83, 522–523. doi: 10.1126/science.83.2161.522-b
Peterson, A. T. (ed.) (2011). Ecological Niches and Geographic Distributions. Princeton, N.J: Princeton University Press.
Petitpierre, B., Kueffer, C., Broennimann, O., Randin, C., Daehler, C., and Guisan, A. (2012). Climatic niche shifts are rare among terrestrial plant invaders. Science 335, 1344–1348. doi: 10.1126/science.1215933
Pievani, T. (2014). The sixth mass extinction: anthropocene and the human impact on biodiversity. Rend. Fis. Acc. Lincei 25, 85–93. doi: 10.1007/s12210-013-0258-9
Pyšek, P., Hulme, P. E., Simberloff, D., Bacher, S., Blackburn, T. M., Carlton, J. T., et al. (2020). Scientists’ warning on invasive alien species. Biol. Rev. 95, 1511–1534. doi: 10.1111/brv.12627
Russell, J. C., and Kueffer, C. (2019). Island biodiversity in the anthropocene. Annu. Rev. Environ. Resour. 44, 31–60. doi: 10.1146/annurev-environ-101718-033245
Sala, O. E., Chapin, F. S. III, Armesto, J. J., Berlow, E., Bloomfield, J., Dirzo, R., et al. (2000). Global biodiversity scenarios for the year 2100. Science 287, 1770–1774. doi: 10.1126/science.287.5459.1770
Salomon, M., Vibert, S., and Bennett, R. G. (2010). Habitat use by western black widow spiders (Latrodectus hesperus) in coastal British Columbia: evidence of facultative group living. Can. J. Zool. 88, 334–346. doi: 10.1139/Z10-004
Saupe, E. E., Papes, M., Selden, P. A., and Vetter, R. S. (2011). Tracking a medically important spider: climate change, ecological niche modeling, and the brown recluse (Loxosceles reclusa). PLoS One 6:e17731. doi: 10.1371/journal.pone.0017731
Schmidt, J. P., Drake, J. M., and Stephens, P. (2017). Residence time, native range size, and genome size predict naturalization among angiosperms introduced to Australia. Ecol. Evol. 7, 10289–10300. doi: 10.1002/ece3.3505
Schoener, T. W. (1968). The anolis lizards of bimini: resource partitioning in a complex fauna. Ecology 49, 704–726. doi: 10.2307/1935534
Schraft, H., Jaham, M. D., Toupin, L.-P., and Montiglio, P.-O. (2021). North American widow spiders (Araneae: Theridiidae). Arachnology 18, 783–802. doi: 10.13156/arac.2020.18.7.783
Segoli, M., Arieli, R., Sierwald, P., Harari, A. R., and Lubin, Y. (2008a). Sexual cannibalism in the brown widow spider (Latrodectus geometricus). Ethology 114, 279–286. doi: 10.1111/j.1439-0310.2007.01462.x
Segoli, M., Lubin, Y., and Harari, A. R. (2008b). Frequency and consequences of damage to male copulatory organs in a widow spider. J. Arachnol. 36, 533–537. doi: 10.1636/St07-30.1
Segoli, M., Harari, A. R., and Lubin, Y. (2006). Limited mating opportunities and male monogamy: a field study of white widow spiders, Latrodectus pallidus (Theridiidae). Anim. Behav. 72, 635–642. doi: 10.1016/j.anbehav.2005.11.021
Shochat, E., Lerman, S. B., Anderies, J. M., Warren, P. S., Faeth, S. H., and Nilon, C. H. (2010). Invasion, competition, and biodiversity loss in Urban ecosystems. BioScience 60, 199–208. doi: 10.1525/bio.2010.60.3.6
Simberloff, D., Martin, J.-L., Genovesi, P., Maris, V., Wardle, D. A., Aronson, J., et al. (2013). Impacts of biological invasions: what’s what and the way forward. Trends Ecol. Evol. 28, 58–66. doi: 10.1016/j.tree.2012.07.013
Simberloff, D., Souza, L., Nuñez, M. A., Barrios-Garcia, M. N., and Bunn, W. (2012). The natives are restless, but not often and mostly when disturbed. Ecology 93, 598–607. doi: 10.1890/11-1232.1
Snyder, W. E., and Evans, E. W. (2006). Ecological effects of invasive arthropod generalist predators. Annu. Rev. Ecol. Evol. Syst. 37, 95–122. doi: 10.1146/annurev.ecolsys.37.091305.110107
Sołtysiak, J. (2020). Does the urban heat island determine the distribution of Fallopia taxa in cities? – preliminary study from Wrocław (Central Europe). EQ 31, 19–25. doi: 10.12775/EQ.2020.010
Taucare-Ríos, A., Bizama, G., and Bustamante, R. O. (2016). Using global and regional species distribution models (SDM) to infer the invasive stage of Latrodectus geometricus(Araneae: Theridiidae) in the Americas. Environ. Entomol. 45, 1379–1385. doi: 10.1093/ee/nvw118
Vassilevski, A. A., Kozlov, S. A., and Grishin, E. V. (2009). Molecular diversity of spider venom. Biochem. Moscow. 74, 1505–1534. doi: 10.1134/S0006297909130069
Vetter, R. S., and Isbister, G. K. (2008). Medical aspects of spider bites. Annu. Rev. Entomol. 53, 409–429. doi: 10.1146/annurev.ento.53.103106.093503
Vetter, R. S., Penas, L. M., and Hoddle, M. S. (2016). Laboratory refugia preferences of the brown widow spider, Latrodectus geometricus (Araneae: Theridiidae). J. Arachnol. 44, 52–57. doi: 10.1636/J15-58.1
Vetter, R. S., Vincent, L. S., Danielsen, D. W. R., Reinker, K. I., Clarke, D. E., Itnyre, A. A., et al. (2012). The prevalence of brown widow and black widow spiders (Araneae: Theridiidae) in Urban Southern California. J. Med. Entomol. 49, 947–951. doi: 10.1603/ME11285
Vincent, L. S., Vetter, R. S., Wrenn, W. J., Kempf, J. K., and Berrian, J. E. (2008). The brown widow spider Latrodectus geometricus C. L. Koch, 1841, in southern California. Pan Pacific Entomol. 84, 344–349. doi: 10.3956/2008-07.1
von der Lippe, M., and Kowarik, I. (2008). Do cities export biodiversity? Traffic as dispersal vector across urban-rural gradients: Traffic as dispersal vector across urban-rural gradients. Divers. Distrib. 14, 18–25. doi: 10.1111/j.1472-4642.2007.00401.x
Wang, Y., Casajus, N., Buddle, C., Berteaux, D., and Larrivée, M. (2018). Predicting the distribution of poorly-documented species, Northern black widow (Latrodectus variolus) and black purse-web spider (Sphodros niger), using museum specimens and citizen science data. PLoS One 13:e0201094. doi: 10.1371/journal.pone.0201094
Warren, D. L., Cardillo, M., Rosauer, D. F., and Bolnick, D. I. (2014). Mistaking geography for biology: inferring processes from species distributions. Trends Ecol. Evol. 29, 572–580. doi: 10.1016/j.tree.2014.08.003
Warren, D. L., Glor, R. E., and Turelli, M. (2008). Environmental niche equivalency versus conservatism: quatitative approaches to niche evolution. Evolution 62, 2868–2883. doi: 10.1111/j.1558-5646.2008.00482.x
Warren, D. L., Matzke, N. J., Cardillo, M., Baumgartner, J. B., Beaumont, L. J., Turelli, M., et al. (2021). ENMTools 1.0: an R package for comparative ecological biogeography. Ecography 44, 504–511. doi: 10.1111/ecog.05485
Williamson, M., Dehnen-Schmutz, K., Kühn, I., Hill, M., Klotz, S., Milbau, A., et al. (2009). The distribution of range sizes of native and alien plants in four European countries and the effects of residence time. Diver. Distrib. 15, 158–166. doi: 10.1111/j.1472-4642.2008.00528.x
Wilson, J. R. U., Richardson, D. M., Rouget, M., Proche,
., Amis, M. A., Henderson, L., et al. (2007). Residence time and potential range: crucial considerations in modelling plant invasions: range size of invasive plants. Diver. Distrib. 13, 11–22. doi: 10.1111/j.1366-9516.2006.00302.x
Yan, S., and Wang, X. (2015). Recent advances in research on widow spider venoms and toxins. Toxins 7, 5055–5067. doi: 10.3390/toxins7124862
Zhang, G., and Zhu, A.-X. (2018). The representativeness and spatial bias of volunteered geographic information: a review. Ann. GIS 24, 151–162. doi: 10.1080/19475683.2018.1501607
Keywords: species distribution models, anthropogenic activity, urbanization, biological invasion, spiders, North America, microhabitat selection
Citation: Sadir M and Marske KA (2021) Urban Environments Aid Invasion of Brown Widows (Theridiidae: Latrodectus geometricus) in North America, Constraining Regions of Overlap and Mitigating Potential Impact on Native Widows. Front. Ecol. Evol. 9:757902. doi: 10.3389/fevo.2021.757902
Received: 13 August 2021; Accepted: 16 November 2021;
Published: 07 December 2021.
Edited by:
Anouschka R. Hof, Wageningen University and Research, NetherlandsReviewed by:
Jiufeng Wei, Shanxi Agricultural University, ChinaAndrés Taucare-Ríos, Arturo Prat University, Chile
Stefano Mammola, National Research Council, Consiglio Nazionale delle Ricerche (CNR), Italy
Copyright © 2021 Sadir and Marske. This is an open-access article distributed under the terms of the Creative Commons Attribution License (CC BY). The use, distribution or reproduction in other forums is permitted, provided the original author(s) and the copyright owner(s) are credited and that the original publication in this journal is cited, in accordance with accepted academic practice. No use, distribution or reproduction is permitted which does not comply with these terms.
*Correspondence: Melissa Sadir, melissa.sadir@ou.edu