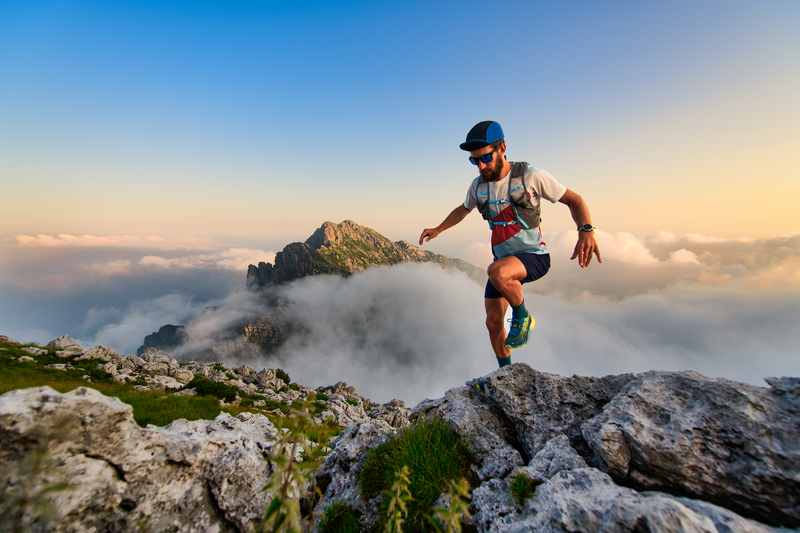
95% of researchers rate our articles as excellent or good
Learn more about the work of our research integrity team to safeguard the quality of each article we publish.
Find out more
ORIGINAL RESEARCH article
Front. Ecol. Evol. , 19 November 2021
Sec. Ecophysiology
Volume 9 - 2021 | https://doi.org/10.3389/fevo.2021.756027
This article is part of the Research Topic Insect Fertility in a Changing Environment View all 7 articles
Pressures from multiple, sometimes interacting, stressors can have negative consequences to important ecosystem-service providing species like the western honey bee (Apis mellifera). The introduced parasite Varroa destructor and the neonicotinoid class of insecticides each represent important, nearly ubiquitous biotic and abiotic stressors to honey bees, respectively. Previous research demonstrated that they can synergistically interact to negatively affect non-reproductive honey bee female workers, but no data exist on how concurrent exposure may affect reproductive honey bee males (drones). This is important, given that the health of reproductive females (queens), possibly because of poor mating, is frequently cited as a major driver of honey bee colony loss. To address this, known age cohorts of drones were obtained from 12 honey bee colonies—seven were exposed to field-relevant concentrations of two neonicotinoids (4.5 ppb thiamethoxam and 1.5 ppb clothianidin) during development via supplementary pollen patties; five colonies received patties not spiked with neonicotinoids. Artificially emerged drones were assessed for natural V. destructor infestation, weighed, and then allocated to the following treatment groups: 1. Control, 2. V. destructor only, 3. Neonicotinoid only, and 4. Combined (both mites and neonicotinoid). Adult drones were maintained in laboratory cages alongside attendant workers (1 drone: 2 worker ratio) until they have reached sexual maturity after 14 days so sperm concentration and viability could be assessed. The data suggest that V. destructor and neonicotinoids interacted synergistically to negatively affect adult drone survival, but that they interacted antagonistically on emergence mass. Although sample sizes were too low to assess the effects of V. destructor and combined exposure on sperm quality, we observed no influence of neonicotinoids on sperm concentration or viability. Our findings highlight the diverse effects of concurrent exposure to stressors on honey bees, and suggest that V. destructor and neonicotinoids can severely affect the number of sexually mature adult drones available for mating.
Stressors like habitat loss, climate change, pollution, and invasive species have resulted in widespread anthropogenic effects on ecosystems (Butchart et al., 2010; Geldmann et al., 2014). Research on how multiple stressors interact to pressure important ecosystem-service providing species have revealed complex effects, ranging from additive, whereby the effects of two stressors equal their combined individual effects, to synergism for which the combined effect of two stressors is greater than the predicted additive effects, to antagonism, when the combined effect of two stressors is less than the predicted additive effects (Côté et al., 2016). Mechanisms responsible for each type of interaction vary, but they may be influenced by exploitative competition for limited resources (Poulin, 2007) or host stress as a result of tissue pathology or immune suppression (Alaux et al., 2010; Pettis et al., 2013). Understanding and predicting the circumstances surrounding each type of interaction is currently difficult, but is expected to significantly improve as laboratory, field, and meta-analysis investigations on model or important ecosystem-service providing species continue (Boyd and Brown, 2015; Piggott et al., 2015; Kaunisto et al., 2016).
In recent years, it is believed that pressures caused by multiple, possibly interacting, stressors are responsible for consistently high losses of managed western honey bee (Apis mellifera) colonies across the northern hemisphere (Kulhanek et al., 2017; Bruckner et al., 2019; Gray et al., 2019, 2020). Both biotic and abiotic stressors, such as poor nutrition, introduced parasites, heavy metals, and pesticides are blamed (Steinhauer et al., 2018). Individually, they can elicit a range of negative consequences on honey bees, ranging from sub-lethal physiological and behavioral effects to lethal ones that result in reduced survival (Havard et al., 2020). When acting in concert, diverse effects are observed, ranging from antagonistic parasitic-pesticide interactions to synergistic pesticide-pesticide ones (Straub et al., 2020; Bird et al., 2021; Siviter et al., 2021).
Arguably one of the most important biotic stressors for honey bees is the ectoparasitic mite Varroa destructor (Rosenkranz et al., 2010; Traynor et al., 2020). Its life cycle is tightly linked to its honey bee host, and consists of two distinct stages—dispersal and reproduction (Traynor et al., 2020). Mature V. destructor foundress mites produce several offspring that subsequently feed on tissues of developing honey bees (hereafter called brood) until they emerge from brood cells alongside their honey bee hosts (Rosenkranz et al., 2010; Ramsey et al., 2019). Mature female V. destructor mites prefer brood of honey bee males, also called drones, because of specific characteristics such as an extended reproduction period as well as the chemical signals elicited by developing drones (Conte et al., 1989; Fuchs, 1992; Rosenkranz et al., 2010). Mite infestation can negatively affect drone body mass and mating efficiency through increased mortality, reduced flight activity, and low sperm quality (Collins and Pettis, 2001; Duay et al., 2002; Bubalo et al., 2005).
Insecticides like systemic neonicotinoids are important abiotic risk factors (Simon-Delso et al., 2015; Wood and Goulson, 2017). Neonicotinoids are among the most widely applied insecticides globally (Simon-Delso et al., 2015), and are predominantly employed as seed coating treatments translocating throughout the entire plant, including pollen and nectar (Bonmatin et al., 2007; Botías et al., 2015; Pang et al., 2020). Neonicotinoids have been detected in honey bee collected pollen from multiple sources, such as maize (Pilling et al., 2013) and squash (Stoner and Eitzer, 2012), herbaceous plants (Wood et al., 2019), and wild flowers (Botías et al., 2015), as well as in beebread, which is processed pollen stored within the colony (Bonmatin et al., 2015; Mogren and Lundgren, 2016; Tong et al., 2018). Furthermore, these compounds are readily released into the soil and water where they can persist and translocate to adjacent vegetation, posing a risk to non-target organisms (Sur et al., 2003; Bonmatin et al., 2015). The risk of exposure extends from individual honey bee foragers to the entire colony when contaminated resources are shared with other adults and developing individuals (Sanchez-Bayo and Goka, 2014). There is clear evidence that field-relevant concentrations of neonicotinoids have sub-lethal effects on honey bees, eliciting behavioral, physiological, and anatomical changes (Singla et al., 2021). For example, drones experienced reduced development stability and produced fewer living sperm under neonicotinoid exposure, which can ultimately affect colony reproductive potential and overall performance (Straub et al., 2016; Friedli et al., 2020).
Despite V. destructor and neonicotinoids considered to ubiquitous stressors to honey bees (Little et al., 2015; Wilfert et al., 2016; Colwell et al., 2017; Mitchell et al., 2017), little is known about their potential interactive effects in honey bees. A handful of studies on non-reproductive honey bee females, the workers, have yielded conflicting results, ranging from no interaction to synergism (Straub et al., 2016; Siede et al., 2018; Morfin et al., 2020; Bird et al., 2021); however, no such work has investigated possible effects on honey bee drones, despite their predicted greater susceptibility to environmental stressors as proposed by the haploid susceptibility hypothesis (O’Donnell and Beshers, 2004), which suggests that drones are likely less resilient to environmental stressors than their worker counterparts due to a lack in allelic variation at important immune related genes (Hamilton, 1964; O’Donnell and Beshers, 2004; Retschnig et al., 2014; Friedli et al., 2020). The availability of high quality drones to mate with reproductive honey bee females, the queens, is crucial for the fitness of those queens and their colonies (Koeniger and Koeniger, 2007), since genetic variation confers benefits such as increased resilience to biotic risk factors (Tarpy, 2003; Tarpy and Seeley, 2006; Delaplane et al., 2015; Simone-Finstrom et al., 2016).
Therefore, for the first time we assessed the effects of simultaneous exposure to neonicotinoid insecticides and the V. destructor mite on honey bee drone emergence mass, adult survival, and sperm quality. Based on previous studies that employed worker honey bees, as well as the haploid susceptibility hypothesis, we expected that both stressors individually would have strong negative effects on drones, and that simultaneous exposure would result in a synergistic negative effect (O’Donnell and Beshers, 2004; Blackmon et al., 2015; Straub et al., 2016, 2019; Maher et al., 2019; Morfin et al., 2020).
Twelve western honey bee (Apis mellifera) packages, each headed by a laying sister queen and 1.5 kg workers, were installed in ten-frame Langstroth hives in Auburn AL, United States on 18 March 2020. To promote growth, colonies were provided ad libitum with sucrose solution (50% weight/volume with water) for 2 weeks before being randomly assigned to either a control or neonicotinoid treatment.
Following an established method (Straub et al., 2019; Friedli et al., 2020), neonicotinoid treatments were administered ad libitum via pollen patties (60% corbicular pollen, 30% powdered sugar, 10% organic honey), as pollen is a common route of neonicotinoid exposure for honey bees (Wood et al., 2019). The pollen was sourced from corbicular pollen removed from honey bee foragers returning to colonies that were located in a low intensity agricultural region of Colorado; subsequent analysis using ultra-high-performance liquid chromatography-tandem mass spectrometry (UHPLC-MS/MS) by the United States Department of Agriculture (USDA) National Science Laboratory (Gastonia, North Carolina, United States) detected no traceable levels of agricultural chemicals (Supplementary Figure 1; AOAC International, 2007). As in previous experiments (Straub et al., 2019; Friedli et al., 2020), colonies allocated to the neonicotinoid treatment (n = 7) received pollen patties spiked with field-relevant concentrations of two neonicotinoids—thiamethoxam and clothianidin (4.5 and 1.5 ppb, both Sigma-Aldrich) (Stoner and Eitzer, 2012; Pilling et al., 2013; Botías et al., 2015; Wood et al., 2019). To create these spiked pollen patties, pure analytical standards of both neonicotinoids (purities of > 99%; Sigma-Aldrich®, Burlington, Massachusetts, United States) were dissolved in distilled water (1 mg/L). Aliquots of a single stock solution for each compound were then added to the organic honey, which was then thoroughly mixed by kneading the components of the patties in a large plastic container until a homogenous paste was made (Sandrock et al., 2014). Concentrations were confirmed by the USDA National Science Laboratory (Gastonia, North Carolina, United States) using ultra-high-performance liquid chromatography-tandem mass spectrometry (UHPLC-MS/MS) (AOAC International, 2007) 4 ppb for thiamethoxam and < 6 ppb for clothianidin; levels of detection were 1 and 6 ppb, respectively) (Supplementary Figure 2). The control treatment (n = 5) was fed non-neonicotinoid spiked pollen patties. Prior to feeding, each colony was equipped with a Sundance pollen trap (Rossman Apiaries, LLC., Moultrie, GA, United States) to promote in-hive patty consumption and prevent the influx of natural pollen (Sandrock et al., 2014; Williams et al., 2015). Following a previously employed feeding regime (Forfert et al., 2015; Williams et al., 2015; Straub et al., 2019), pollen patties were provided for 49 days to cover two entire brood cycles (Winston, 1991), and to mimic a realistic exposure period encountered by foraging honey bees. Earlier studies have demonstrated that foraging honey bees can be exposed to neonicotinoid residues for a similar period because of overlapping bloom periods of treated crops (Tsvetkov et al., 2017), contaminated planter dust that is exhausted to the environment (Krupke et al., 2012), and crops and neighboring non-agricultural foraging areas being contaminated as a result of leaching (Botías et al., 2015; Schaafsma et al., 2015; Long and Krupke, 2016; Mogren and Lundgren, 2016).
To obtain a known age cohort of drones for the experiment, at 42 days post initial neonicotinoid exposure the queen of each colony was caged for 2 days onto a drone brood frame previously drawn-out by her own colony during neonicotinoid exposure (Williams et al., 2013). These drone frames remained in their respective colonies for 20 days more. Subsequently, the frames were moved on day 22 to an incubator (34°C and 60% RH, DR-41NL, Percival Scientific, Inc., Perry, IW) (Williams et al., 2013). The next day, which was 1 day prior to expected natural emergence (Winston, 1991), drones were artificially emerged from the capped brood cells using forceps. Each brood cell and drone were visually inspected for V. destructor parasitism, defined as the observation of one or more adult female V. destructor. Each drone was also inspected for wing deformities, which are typically clinical symptoms of deformed wing virus (Dainat et al., 2012), weighed to the nearest 0.1 mg using an analytical scale (VWR B2-Series, VWR, Radnor, PA, United States), and then assigned to one of the four treatment groups based on their previous colony-level exposure to neonicotinoids and individual V. destructor parasitism status (Straub et al., 2019): (1) No neonicotinoids and No V. destructor (Control), (2) No neonicotinoids and Yes V. destructor parasitism (V. destructor only), (3) Yes neonicotinoids and No V. destructor parasitism (Neonicotinoid only), and (4) Yes neonicotinoids and Yes V. destructor parasitism (Combined).
For each colony, we established up to five hoarding cages per treatment group from each available colony which resulted in a total of 60 experimental cages (Williams et al., 2013). Cages were made of 250 cm3 plastic cups (Plastikbecher.de GmbH, Giengen, Germany) equipped with a round lid. A ventilation hole (6 cm in diameter) was cut out from the lid and covered with a felt cloth (Maier Haushaltspflege GmbH, Murg, Germany). Generally, each cage contained 10–12 adult drones and 20–24 adult workers from the same colony. Adult workers were collected from a brood frame and added to the cage to provide caretaking duties for the drones (Ruttner, 1966; Straub et al., 2016). If a colony did not yield 10 drones for each cage we nonetheless collected all available drones. Therefore, we did not obtain 10 drones for nine of the 60 cages. Regardless, the adult drone to worker ratio was maintained at 1:2 for all cages for the duration of the experiment. Hoarding cages were kept in the incubator (30°C and 60% RH, DR-41NL, Percival Scientific, Inc., Perry, IW) and equipped with a 5 ml syringe containing sucrose solution (50% w/v) to feed the honey bees (Williams et al., 2013). Additionally, a 1.5 ml polypropylene tube (Eppendorf, Enfield, CT, United States), modified to act as an in-cage feeder containing sucrose solution (50% w/v), and a 1.5 ml polypropylene tube, modified to act as an in-cage feeder containing pollen (60% corbicular pollen, 40% powdered sugar), were added to facilitate autonomous drone feeding and to promote development and maturation of male reproductive organs, respectively (Brodschneider and Crailsheim, 2010; Williams et al., 2013). All food resources were provided ad libitum; feeders were replaced every 4 days (Fryday et al., 2015; Minnameyer et al., 2021). An orientation flight was simulated 8 days post cage initiation by exposing cages to indirect sunlight for 24 h; this has been suggested to mark the completion of sperm transition from testis to the seminal vesicles and initiate full maturation of the ejaculate (Schlüns et al., 2005; Hayashi and Satoh, 2019). Adult drones were maintained in hoarding cages for 14 days post emergence, when all surviving individuals were expected to be sexually mature (Rhodes et al., 2011); dead drones and workers were removed daily from each cage.
All individuals surviving to day 14 post emergence were then sacrificed for subsequent in vivo sperm quality assessments to prevent sperm migration from seminal vesicles to the bulb (Straub et al., 2016). In brief, the abdomen of each drone was detached from its thorax using dissection scissors, then pinned onto a wax plate before removing ventral sternites so that the testes, mucus glands, and seminal vesicles could be removed using a forceps. For each individual, all structures were placed in a 1.5 ml polypropylene tube (Eppendorf, Enfield, CT, United States) containing 500 μl Kiev+ buffer and crushed to make a diluted sperm stock solution (SSS) (Carreck et al., 2013). Subsequently, sperm viability and concentration were assessed following Straub et al. (2016, 2021). For sperm viability, a 50 μl aliquot of the SSS was added to a 1.5 ml polypropylene tube containing 50 μl Kiev+ buffer (Company, City, State, United States). Then, 2 μl of Hoechst 33342 (0.5 mg ml–1) and 1 μl of propidium iodide (1 mg ml–1) (both Sigma-Aldrich®, Burlington, Massachusetts, United States) were added to label living (viable; green) and dead (non-viable; red) sperm. The suspension was then incubated for 20 min in complete darkness and then gently vortexed. Next, 10 μl of the solution were examined on a microscope slide at 400x magnification using a fluorescent light microscope (Leica, DM2500 LED, Morrisville, NC, United States). Ten arbitrary visual fields were selected to count the quantity of viable and non-viable sperm; an average value was then calculated from these fields. For sperm concentration, 20 μl SSS were diluted with 80 μl Kiev+ buffer (1:5 dilution) in a 1.5 ml polypropylene tube, then an aliquot was transferred to a cell counting chamber (Thermo Fisher Scientific, Waltham, MA, United States) to count sperm using the fluorescent light microscope. Total sperm concentration (in 500 μl SSS) was calculated by multiplying the average number of sperm counted in two chambers by the dilution factor (1:5) by the volume used for the counting chamber (10 μl) by the SSS volume (500 μl). Lastly, living sperm concentration was determined by multiplying average sperm viability by total sperm concentration.
Just prior to queen caging at 39 days post initial neonicotinoid exposure, adult bees and capped brood (bees developing under a wax capping) were visually assessed in each colony using the Liebefeld estimation method (Delaplane et al., 2013). For this, both colony strength parameters were first estimated for each frame in each colony as a percentage of frame coverage from 0 to 100. Then, percent coverage was converted to an absolute value of number of bees and area (cm2) for adults and capped brood, respectively. Colony-level V. destructor infestation was assessed at the same time using the alcohol wash method to determine the number of mites per 100 adult bees by sampling ∼300 adult bees from the brood nest (Dietemann et al., 2013).
All statistical analyses were performed in R (version 4.0.2., 11/2/20) using a significance level of α = 0.05. Colony-level neonicotinoid exposure and individual-level V. destructor infestation were always contained as fixed factors in the model, like (Straub et al., 2019). Employing a backward selection approach, we built linear Mixed Effect Models (lmm) for normally distributed data and Generalized Linear Mixed Effect Models (glmm) for data that was not normally distributed; data were tested for normality using the ggdensity and ggqqplot function from the R package ggpubr. All models started as a full model including all explanatory variables that could potentially affect observed variation in response variables (e.g., sperm viability). When a significant effect was detected, explanatory variables were included as random factors. The significance of individual explanatory variables was assessed using the Akaike Information Criterion (AIC function in R). Based on this approach, a lmm was built using the lmer function from R package lme4 to assess effects of neonicotinoids and V. destructor parasitism on emergence body mass of drones, while including colony and cage identification number as random factors.
The R package survival was used to fit a Cox proportional hazards regression model using the coxph function to assess effects of individual and combined neonicotinoid exposure and V. destructor infestation on drone survival. Furthermore, survival curves (Kaplan-Meier plots) were plotted using the ggsurvplot function and hazard rates for each treatment group and calculated using the tbl_regression function. Subsequently, cumulative survival was compared between treatment groups by using the pairwise_survdiff function from the R package survminer which allowed for pairwise comparisons with a Bonferroni correction [Survival Bonferroni Multiple Comparison Test (sbmct)].
For sperm concentration and living sperm concentration, a glmm including cage identification number as random factor to account for potential clustering effects was built. Data were not transformed. Therefore, a best fit distribution was incorporated (family = Poisson). For sperm viability data, a l mm was fitted to assess fixed factor effects, with cage and drone identification numbers as a random factors to account for potential clustering effects. For each model, post hoc pairwise comparisons of all treatment groups were performed by using the lsmeans function from the emmeans package in R and using a Bonferroni correction for multiple comparisons [Bonferroni Multiple Comparison Test (bmct)]. To identify potential interactions between neonicotinoid exposure and V. destructor infestation, an additive effects framework was employed (Folt et al., 1999). Interactions were considered synergistic or antagonistic if the effect of the combined stressor treatment group was greater or smaller than the sum resulting from individual stressors (V. destructor and neonicotinoid) (Hay, 1996). To assess this, the percent difference in treatment groups compared to the controls were calculated using mean survival [d], total sperm quantity [#], living sperm quantity [#], and sperm viability [%].
To assess the effects of the colony-level neonicotinoid treatment on number of adult bees, a glmm was built with a best fit distribution (family = negative binomial) to account for overdispersed count data. Similarly, a glmm with a best fit distribution (family = poisson) was fitted to assess effects on colony-level V. destructor infestation. Both glmm’s were followed by a Wilcoxon Rank Sum Test (wrt) using the wilcox.test function. For capped brood area, a lmm was fitted, followed by a Two-Samples t-test (t-test) using the t.test function.
No significant differences were observed between neonicotinoid treated colonies and control colonies for number of adult bees (wrt, W = 90, p = 0.42), capped brood area (t-test, t = 0.54, p = 0.59), or V. destructor mite infestation (wrt, W = 11, p = 0.19) (Supplementary Table 1). The V. destructor count per 100 bees for neonicotinoid treated colonies was 1.5 ± 1.6 (median ± SD) compared to 1 ± 0.5 (median ± SD) for control colonies. Wing deformities were not observed for any of the newly emerged drones.
Body mass of newly emerged drones (n = 792) was significantly affected by both neonicotinoid exposure (Linear Mixed Effect Model (l mm), t = 2.99, p = 0.003) and V. destructor infestation (lmm, t = −7.20, p < 0.001) (Figure 1 and Supplementary Table 1). Compared to drones from the Control (n = 247), V. destructor only (n = 91) and Combined stressor (n = 86) treatment groups, emergence body mass was significantly higher in Neonicotinoid only drones (n = 368, mean ± SE, 274.2 ± 3.9 mg; Bonferroni Multiple Comparison Test (bmct), all p < 0.05). The lowest body mass was recorded in V. destructor only drones, which was significantly different from the Control (mean ± SE, 245.9 ± 4.4 mg; bmct, p < 0.001) and Combined treatment groups (bmct, p = 0.02). Body mass of Control (mean ± SE, 261.8 ± 4.3 mg) and Combined drones (mean ± SE, 258.3 ± 4.3 mg) did not differ from each other (bmct, p > 0.05) (Supplementary Tables 2, 3). Exposure to the Combined stressors reduced worker body mass by 0% compared to the Controls. This was higher than the sum of the individual stressors, which was −2.1% because of a 4.0% increase for Neonicotinoid only and 6.1% reduction for V. destructor only treatment workers. This suggests an antagonistic interaction.
Figure 1. Emergence body mass of experimental western honey bee (Apis mellifera) drones across four treatment groups: Control—drones without neonicotinoid exposure and without Varroa destructor parasitism (n = 247), Neonicotinoid only—drones exposed to neonicotinoids during development but free from V. destructor parasitism (n = 368), V. destructor only—drones infested by V. destructor during development, but not exposed to neonicotinoids (n = 91), and Combined—drones exposed to both neonicotinoids and V. destructor during development (n = 86). Boxplots show the inter-quartile range (box), the mean (black circles), the median (black line within box), data range (vertical black lines from box), and outliers (open circles). Different letters above boxplots indicate statistically significant differences (p ≤ 0.05).
Significant differences in adult drone survival were observed among treatment groups (Figure 2). Although no difference in survival was observed between V. destructor only and Control drones (sbmct, z = 1.96, p = 0.16), Neonicotinoids only (sbmct, z = 2.84, p = 0.03) and Combined drones (sbmct, z = 6.61, p < 0.001) experienced significantly reduced survival compared to Controls (Supplementary Tables 2, 3). Despite the lack of a statistical difference, hazard rate (HR) for V. destructor only drones was 135% (95% CI [100, 182%]) compared to Controls, whereas it was 136% (95% CI [110, 168%]) and 267% (95% CI [200, 358%]) for drones belonging to Neonicotinoid only and Combined treatment groups, respectively (Supplementary Table 4). Compared to Controls, the reduction in survival of adult drones that were exposed to Combined stressors (55%) was greater than the sum of individual stressor effects, which were 17 and 28% reductions for Neonicotinoid only and V. destructor only drones, respectively. This suggests a synergistic interaction between the two stressors. This is further supported by the increase in HR for the Combined stressors (167%) compared to Controls, which was also greater than the sum of increased HRs for individual stressors, which was 35% for V. destructor only and 36% for neonicotinoid only.
Figure 2. Kaplan-Meier survival curves of experimental western honey bee (Apis mellifera) drones during a 14-day laboratory cage assay. Summary of survival curves for all four treatment groups: Control—drones without neonicotinoid exposure and without Varroa destructor parasitism, Neonicotinoid only—drones exposed to neonicotinoids during development but free from V. destructor parasitism, V. destructor only—drones parasitized by V. destructor during development but not exposed to neonicotinoids, and Combined—drones exposed to both neonicotinoids and V. destructor during development. Different letters placed before treatment groups indicate statistically significant differences (p ≤ 0.05).
Compared to Controls, Neonicotinoid only drones did not experience a significant reduction in sperm concentration [Kruskal-Wallis rank sum test (KW), χ2 = 2.11, p = 0.15], sperm viability (t-test, t = 1.72, p = 0.09), or living sperm concentration (KW, χ2 = 0.69, p = 0.41) (Supplementary Tables 1, 3). Sample sizes of drones belonging to the V. destructor only and the Combined treatment group were lower than 15 for each sperm quality trait. Therefore, these two treatment groups were omitted from statistical analyses regarding sperm quality traits.
Pressure caused by stressor interactions is believed to be responsible for widespread negative effects on biodiversity (Butchart et al., 2010; Barnosky et al., 2011), including on the economically and ecologically important honey bee (Alaux et al., 2010; Straub et al., 2019). For the first time, we investigated the potential effects of simultaneous pressure from neonicotinoid insecticides and V. destructor mites on adult male honey bee (drone) emergence mass and survival. The data revealed that combined exposure to both stressors resulted in a negative synergistic effect on adult drone survival, but an antagonistic effect on emergence mass. Our results suggest that combined exposure to these two ubiquitous stressors can severely affect the availability of drones for mating, and further highlight the complexity of potential interactive effects of simultaneous stressor pressures on honey bees.
Effects of concurrent exposure from multiple stressors on honey bees have garnered considerable attention of late. This is especially the case considering negative synergistic interactions for which the effect of multiple concurrent factors are worse than the sum of individual effects (Maher et al., 2019). As hypothesized, we observed a synergistic effect of neonicotinoids and V. destructor on adult drone survival. Interestingly, this same interaction was not observed for adult worker survival by a similar experiment performed during the same time of year (Straub et al., 2019). Although that study was performed in a different location, comparative results between the two suggest that differences in observed combined stressor effects on adult drone and worker survival could be explained by the haploid susceptibility hypothesis. This hypothesis states that drones experience higher susceptibility to environmental stressors due to their hemizygosity at loci involved in immune response (O’Donnell and Beshers, 2004; Blackmon et al., 2015). Since both neonicotinoids and V. destructor are known to impair the immune response of honey bees (Claudianos et al., 2006; Prisco et al., 2013; Brandt et al., 2016), a reduction in allelic diversity due to hemizygosity may result in more severe consequences when exposed, although future studies should be performed under the same conditions to further explore this as a reason for differences observed between these two types of honey bees.
In contrast to the observed synergistic negative effect on adult drone survival, we found that the two stressors had an antagonistic effect on drone emergence mass. Similar, contradictory observations were observed by a recent meta-analysis of agrochemical pesticide interactions, whereby proxies of honey bee fitness like behavior and physiology revealed additive or antagonistic effects, whereas synergistic effects were common on honey bee mortality (Siviter et al., 2021). A possible explanation for our observation may be because neonicotinoids are known to affect honey bee carbohydrate and lipid metabolism (Derecka et al., 2013; Cook, 2019). For example, Cook (2019) found that honey bees exposed to low concentrations of clothianidin had high lipid contents compared to controls. Thus, feeding by V. destructor during development may possibly offset the gain in body mass resulting from metabolic dysfunction associated with exposure to neonicotinoids, since V. destructor parasitism alone reduced emergence body mass in our experiment. Unfortunately, due to low sample size as a result of synergistic effects on mortality, we could not sufficiently examine possible interactions of neonicotinoids and V. destructor on sperm traits. Although examining all stressor combinations on multiple proxies of honey bee fitness are not realistic (Côté et al., 2016), the synergistic interaction that we observed on drone mortality by the time of sexual maturity could justify further attempts to understand how these two ubiquitous stressors affect the reproductive health of drones at this important time period, as a reduction in queen health, possibly due to poor mating, is frequently reported as a primary reason for honey bee colony mortality (Pettis et al., 2016).
Both neonicotinoids and V. destructor have each been shown to negatively affect drone health and performance (Rangel and Fisher, 2019; Friedli et al., 2020; Straub et al., 2021). On its own, V. destructor parasitism reduced drone emergence body mass by 6% compared to controls during our experiment. This has been shown for both workers and drones (Bowen-Walker and Gunn, 2001; Duay et al., 2003; Blanken et al., 2015; Ramsey et al., 2019; Straub et al., 2019), and is likely the result of mites feeding on the honey bees’ fat bodies during development. In contrast, exposure to neonicotinoids alone resulted in increased emergence body mass of drones (+ 4%). Low concentrations of neonicotinoids are known to affect carbohydrate and lipid metabolism, both which could affect body mass (Derecka et al., 2013). Other examples suggest that hormesis, a biphasic dose response in which biological processes can be stimulated by low concentrations of normally harmful chemical (Calabrese, 2005), can result in increased body mass, possibly at the expense of fitness (Cutler, 2012; Cutler and Rix, 2015). Indeed, our results suggest a negative effect of neonicotinoids on drone survival until sexual maturity, 14 days post emergence. A possible explanation for this could be a reduction in detoxification-competence (Claudianos et al., 2006). Additionally, experimental drones might have been nourished by compromised worker nurses during development, as previous investigations demonstrated that neonicotinoid exposure negatively affected nurse food glands. Additionally, experimental drones might have been nourished by compromised worker nurses during development, as previous investigations demonstrated that neonicotinoid exposure negatively affected nurse food glands (Hatjina et al., 2013). In contrast, V. destructor parasitism had no effect on adult drone survival. This is unexpected, since mites prefer drone brood cells and have been shown to negatively affect adult worker survival (Straub et al., 2019). Surprisingly few efforts have investigated the effects of V. destructor on drone survival (Fuchs, 1992; Rinderer et al., 1999; Collins and Pettis, 2001). For example, Collins and Pettis (2001) found that survival of drones parasitized by more than one reproductive female mite was not impacted when only one female V. destructor was present. Thus, low levels of colony-level infestation in our study could explain why we also did not observe a negative effect of V. destructor alone on drone survival. Despite a lack of a significant reduction in drone survival, it is noteworthy that V. destructor parasitism during development still increased the hazard ratio for drones (35% compared to Controls, Supplementary Table 4); thus, parasitized drones still likely experience increased stress. We were not able to assess effects of V. destructor only on sperm quality traits due to low sample size at the end of the cage trial. Relative to the Control and Neonicotinoid only treatment groups, fewer drones were assigned to this treatment groups at the start of the trail, potentially because parasitized developing drones were removed from their cells prior to artificial emergence (Harbo and Harris, 2005). Low adult survival of drones exposed to V. destructor during development further limited sample size. However, we found that there was no effect of neonicotinoids on sperm quality traits when drones were exposed during development. These results align with Ciereszko et al. (2017), but they contrast Straub et al. (2016) who found negative effects on sperm viability and concentration of living sperm, but not total sperm concentration. Given that spermatogenesis begins during the larval stage and terminates at pupation (Yániz et al., 2020), drones were potentially only exposed to a low dose of insecticides for a portion of the total spermatogenesis process, during the larval stage. This highlights that testing arena and experimental design likely contribute to the complexity of comparing observations among multiple studies, and demonstrates the importance of controlled ring tests performed in multiple laboratories when investigating stressor effects (Medrzycki et al., 2013; van der Sluijs et al., 2015).
Our results highlight that interaction effects between two important stressors, like V. destructor mites and neonicotinoid insecticides for honey bees, can range from synergism to antagonism depending on the variable measured. According to our findings, neonicotinoids and V. destructor interacted synergistically to induce a severe lethal effect on adult honey bee drones, but interacted antagonistically on drone emergence body mass. The complexity of stressor interactions in the honey bee warrants future work elucidating outcomes of simultaneous pressure from multiple stressors, especially for risk assessment schemes that may otherwise not accurately estimate the interactive effects of stressors. Additionally, extrapolating effects observed under laboratory conditions to the field remain a major challenge and additional field-studies are required to confirm our findings.
Data are available via the Dryad Digital Repository at https://doi.org/10.5061/dryad.gmsbcc2p8.
SB, GW, and PN developed the experimental design. SB performed the experiment and collected the data. SB and LS performed statistical analysis. All authors wrote the manuscript and approved its final submission.
This work was supported by the USDA National Institute of Food and Agriculture Multi-state Hatch project NC1173, the Alabama Agricultural Experiment Station, the Foundation for Food and Agriculture Research Pollinator Health Fund grant 549003, the USDA ARS Cooperative Agreement 6066-21000-001-02-S, the Swiss National Science Foundation Project 31003A_169751, and the California State Beekeepers’ Association. Additional support was provided by the Bundesamt für Umwelt (BAFU) to LS and PN (16.0091.PJ/R102- 1664), by Agroscope to LS and PN, and by the Vinetum Foundation to LS and PN.
The authors declare that the research was conducted in the absence of any commercial or financial relationships that could be construed as a potential conflict of interest.
All claims expressed in this article are solely those of the authors and do not necessarily represent those of their affiliated organizations, or those of the publisher, the editors and the reviewers. Any product that may be evaluated in this article, or claim that may be made by its manufacturer, is not guaranteed or endorsed by the publisher.
We thank the Auburn Bee Lab master students and technicians Christian Baker, Suleyman Alparslan, Adler Salem, and Rachel Jacobson for their assistance in setting up the experimental colonies and administering the neonicotinoid treatment.
The Supplementary Material for this article can be found online at: https://www.frontiersin.org/articles/10.3389/fevo.2021.756027/full#supplementary-material
Alaux, C., Brunet, J.-L., Dussaubat, C., Mondet, F., Tchamitchan, S., Cousin, M., et al. (2010). Interactions between Nosema microspores and a neonicotinoid weaken honeybees (Apis mellifera). Environ. Microbiol. 12, 774–782. doi: 10.1111/j.1462-2920.2009.02123.x
AOAC International (2007). Official Methods of Analysis. Available online at: http://www.eoma.aoac.org/methods/info.asp?ID=48938 (accessed August 3, 2021).
Barnosky, A. D., Matzke, N., Tomiya, S., Wogan, G. O. U., Swartz, B., Quental, T. B., et al. (2011). Has the Earth’s sixth mass extinction already arrived? Nature 471, 51–57. doi: 10.1038/nature09678
Bird, G., Wilson, A. E., Williams, G. R., and Hardy, N. B. (2021). Parasites and pesticides act antagonistically on honey bee health. J. Appl. Ecol. 58, 997–1005. doi: 10.1111/1365-2664.13811
Blackmon, H., Hardy, N. B., and Ross, L. (2015). The evolutionary dynamics of haplodiploidy: genome architecture and haploid viability. Evolution 69, 2971–2978. doi: 10.1111/evo.12792
Blanken, L. J., van Langevelde, F., and van Dooremalen, C. (2015). Interaction between Varroa destructor and imidacloprid reduces flight capacity of honeybees. Proc. R. Soc. B 282:20151738. doi: 10.1098/rspb.2015.1738
Bonmatin, J.-M., Giorio, C., Girolami, V., Goulson, D., Kreutzweiser, D. P., Krupke, C., et al. (2015). Environmental fate and exposure; neonicotinoids and fipronil. Environ. Sci. Pollut. Res. 22, 35–67. doi: 10.1007/s11356-014-3332-7
Bonmatin, J.-M., Marchand, P., Cotte, J.-F., Aajoud, A., Casabianca, H., Goutailler, G., et al. (2007). “Bees and systemic insecticides (imidacloprid, fipronil) in pollen: subnano-quantification by HPLC/MS/MS and GC/MS,” in Environmental Fate and Ecological Effects of Pesticide, eds A. A. M. Del Re, E. Capri, and T. M. Fragoulis (Pavia: La Goliardica Pavese), 827.
Botías, C., David, A., Horwood, J., Abdul-Sada, A., Nicholls, E., Hill, E., et al. (2015). Neonicotinoid residues in wildflowers, a potential route of chronic exposure for bees. Environ. Sci. Technol. 49, 12731–12740. doi: 10.1021/acs.est.5b03459
Bowen-Walker, P. L., and Gunn, A. (2001). The effect of the ectoparasitic mite, Varroa destructor on adult worker honeybee (Apis mellifera) emergence weights, water, protein, carbohydrate, and lipid levels. Entomol. Exp. Appl. 101, 207–217. doi: 10.1046/j.1570-7458.2001.00905.x
Boyd, P. W., and Brown, C. J. (2015). Modes of interactions between environmental drivers and marine biota. Front. Mar. Sci. 2:7. doi: 10.3389/fmars.2015.00009
Brandt, A., Gorenflo, A., Siede, R., Meixner, M., and Büchler, R. (2016). The neonicotinoids thiacloprid, imidacloprid, and clothianidin affect the immunocompetence of honey bees (Apis mellifera L.). J. Insect Physiol. 86, 40–47. doi: 10.1016/j.jinsphys.2016.01.001
Brodschneider, R., and Crailsheim, K. (2010). Nutrition and health in honey bees. Apidologie 41, 278–294. doi: 10.1051/apido/2010012
Bruckner, S., Steinhauer, N., Aurell, D., Caron, D. M., Ellis, J. D., Fauvel, A.-M., et al. (2019). 2018-2019 Honey Bee Colony Losses in the United States: Preliminary Results. Available online at: https://beeinformed.org/wp-content/uploads/2019/11/2018_2019-Abstract.pdf (accessed September 08, 2021).
Bubalo, D., Pechhacker, H., Licek, E., and Kexic, N. (2005). The effect of Varroa destructor infestation on flight activity and mating efficiency of drones (Apis mellifera L.). Wien. Tierarztl. Monatsschr. 92, 11–15.
Butchart, S. H. M., Walpole, M., Collen, B., van Strien, A., Scharlemann, J. P. W., Almond, R. E. A., et al. (2010). Global biodiversity: indicators of recent declines. Science 328, 1164–1168. doi: 10.1126/science.1187512
Calabrese, E. J. (2005). Paradigm lost, paradigm found: the re-emergence of hormesis as a fundamental dose response model in the toxicological sciences. Environ. Pollut. 138, 378–411. doi: 10.1016/j.envpol.2004.10.001
Carreck, N. L., Andree, M., Brent, C. S., Cox-Foster, D., Dade, H. A., Ellis, J. D., et al. (2013). Standard methods for Apis mellifera anatomy and dissection. J. Apic. Res. 52, 1–40. doi: 10.3896/IBRA.1.52.4.03
Ciereszko, A., Wilde, J., Dietrich, G. J., Siuda, M., Ba̧k, B., Judycka, S., et al. (2017). Sperm parameters of honeybee drones exposed to imidacloprid. Apidologie 48, 211–222. doi: 10.1007/s13592-016-0466-2
Claudianos, C., Ranson, H., Johnson, R. M., Biswas, S., Schuler, M. A., Berenbaum, M. R., et al. (2006). A deficit of detoxification enzymes: pesticide sensitivity and environmental response in the honeybee. Insect Mol. Biol. 15, 615–636. doi: 10.1111/j.1365-2583.2006.00672.x
Collins, A. M., and Pettis, J. S. (2001). Effect of Varroa infestation on semen quality. Am. Bee J. 141, 590–593.
Colwell, M. J., Williams, G. R., Evans, R. C., and Shutler, D. (2017). Honey bee-collected pollen in agro-ecosystems reveals diet diversity, diet quality, and pesticide exposure. Ecol. Evol. 7, 7243–7253. doi: 10.1002/ece3.3178
Conte, Y. L., Arnold, G., Trouiller, J., Masson, C., Chappe, B., and Ourisson, G. (1989). Attraction of the parasitic mite Varroa to the drone larvae of honey bees by simple aliphatic esters. Science 245, 638–639. doi: 10.1126/science.245.4918.638
Cook, S. C. (2019). Compound and dose-dependent effects of two neonicotinoid pesticides on honey bee (Apis mellifera) metabolic physiology. Insects 10:18. doi: 10.3390/insects10010018
Côté, I. M., Darling, E. S., and Brown, C. J. (2016). Interactions among ecosystem stressors and their importance in conservation. Proc. R. Soc. B Biol. Sci. 283:9. doi: 10.1098/rspb.2015.2592
Cutler, G. C. (2012). Insects, insecticides and hormesis: evidence and considerations for study. Dose Response 11, 154–177. doi: 10.2203/dose-response.12-008.Cutler
Cutler, G. C., and Rix, R. R. (2015). Can poisons stimulate bees? Appreciating the potential of hormesis in bee–pesticide research. Pest Manag. Sci. 71, 1368–1370. doi: 10.1002/ps.4042
Dainat, B., Evans, J. D., Chen, Y. P., Gauthier, L., and Neumann, P. (2012). Predictive markers of honey bee colony collapse. PLoS One 7:e32151. doi: 10.1371/journal.pone.0032151
Delaplane, K. S., Pietravalle, S., Brown, M. A., and Budge, G. E. (2015). Honey bee colonies headed by hyperpolyandrous queens have improved brood rearing efficiency and lower infestation rates of parasitic Varroa mites. PLoS One 10:e0142985. doi: 10.1371/journal.pone.0142985
Delaplane, K. S., van der Steen, J., and Guzman-Novoa, E. (2013). Standard methods for estimating strength parameters of Apis mellifera colonies. J. Apic. Res. 52, 1–12. doi: 10.3896/IBRA/1.52.1.03
Derecka, K., Blythe, M. J., Malla, S., Genereux, D. P., Guffanti, A., Pavan, P., et al. (2013). Transient exposure to low levels of insecticide affects metabolic networks of honeybee larvae. PLoS One 8:e68191. doi: 10.1371/journal.pone.0068191
Dietemann, V., Nazzi, F., Martin, S. J., Anderson, D. L., Locke, B., Delaplane, K. S., et al. (2013). Standard methods for Varroa research. J. Apic. Res. 52, 1–54. doi: 10.3896/IBRA.1.52.1.09
Duay, P., Jong, D. D., and Engels, W. (2002). Decreased flight performance and sperm production in drones of the honey bee (Apis mellifera) slightly infested by Varroa destructor mites during pupal development. Genet. Mol. Res. 1, 227–232.
Duay, P., Jong, D. D., and Engels, W. (2003). Weight loss in drone pupae (Apis mellifera) multiply infested by Varroa destructor mites. Apidologie 34, 61–65. doi: 10.1051/apido:2002052
Folt, C. L., Chen, C. Y., Moore, M. V., and Burnaford, J. (1999). Synergism and antagonism among multiple stressors. Limnol. Oceanogr. 44, 864–877.
Forfert, N., Natsopoulou, M. E., Frey, E., Rosenkranz, P., Paxton, R. J., and Moritz, R. F. A. (2015). Parasites and pathogens of the honeybee (Apis mellifera) and their influence on inter-colonial transmission. PLoS One 10:e0140337. doi: 10.1371/journal.pone.0140337
Friedli, A., Williams, G. R., Bruckner, S., Neumann, P., and Straub, L. (2020). The weakest link: haploid honey bees are more susceptible to neonicotinoid insecticides. Chemosphere 242:125145. doi: 10.1016/j.chemosphere.2019.125145
Fryday, S., Tiede, K., and Stein, J. (2015). Scientific services to support EFSA systematic reviews: lot 5 systematic literature review on the neonicotinoids (namely active substances clothianidin, thiamethoxam and imidacloprid) and the risks to bees (Tender specifications RC/EFSA/PRAS/2013/03). EFSA Support. Publ. 12:756E. doi: 10.2903/sp.efsa.2015.EN-756
Fuchs, S. (1992). Choice in Varroa jacobsoni Oud. between honey bee drone or worker brood cells for reproduction. Behav. Ecol. Sociobiol. 31, 429–435.
Geldmann, J., Joppa, L. N., and Burgess, N. D. (2014). Mapping change in human pressure globally on land and within protected areas. Conserv. Biol. 28, 1604–1616. doi: 10.1111/cobi.12332
Gray, A., Adjlane, N., Arab, A., Ballis, A., Brusbardis, V., Charrière, J.-D., et al. (2020). Honey bee colony winter loss rates for 35 countries participating in the COLOSS survey for winter 2018–2019, and the effects of a new queen on the risk of colony winter loss. J. Apic. Res. 59, 744–751. doi: 10.1080/00218839.2020.1797272
Gray, A., Brodschneider, R., Adjlane, N., Ballis, A., Brusbardis, V., Charrière, J.-D., et al. (2019). Loss rates of honey bee colonies during winter 2017/18 in 36 countries participating in the COLOSS survey, including effects of forage sources. J. Apic. Res. 58, 479–485. doi: 10.1080/00218839.2019.1615661
Hamilton, W. D. (1964). The genetical evolution of social behaviour. II. J. Theor. Biol. 7, 17–52. doi: 10.1016/0022-5193(64)90039-6
Harbo, J. R., and Harris, J. W. (2005). Suppressed mite reproduction explained by the behaviour of adult bees. J. Apic. Res. 44, 21–23. doi: 10.1080/00218839.2005.11101141
Hatjina, F., Papaefthimiou, C., Charistos, L., Dogaroglu, T., Bouga, M., Emmanouil, C., et al. (2013). Sublethal doses of imidacloprid decreased size of hypopharyngeal glands and respiratory rhythm of honey bees in vivo. Apidologie 44, 467–480. doi: 10.1007/s13592-013-0199-4
Havard, T., Laurent, M., and Chauzat, M.-P. (2020). Impact of stressors on honey bees (Apis mellifera; Hymenoptera: Apidae): some guidance for research emerge from a meta-analysis. Diversity 12:7. doi: 10.3390/d12010007
Hayashi, S., and Satoh, T. (2019). Sperm maturation process occurs in the seminal vesicle following sperm transition from testis in honey bee males. Apidologie 50, 369–378. doi: 10.1007/s13592-019-00652-5
Kaunisto, S., Ferguson, L. V., and Sinclair, B. J. (2016). Can we predict the effects of multiple stressors on insects in a changing climate? Curr. Opin. Insect Sci. 17, 55–61. doi: 10.1016/j.cois.2016.07.001
Koeniger, N., and Koeniger, G. (2007). Mating flight duration of Apis mellifera queens: as short as possible, as long as necessary. Apidologie 38, 606–611. doi: 10.1051/apido:2007060
Krupke, C. H., Hunt, G. J., Eitzer, B. D., Andino, G., and Given, K. (2012). Multiple routes of pesticide exposure for honey bees living near agricultural fields. PLoS One 7:e29268. doi: 10.1371/journal.pone.0029268
Kulhanek, K., Steinhauer, N., Rennich, K., Caron, D. M., Sagili, R. R., Pettis, J. S., et al. (2017). A national survey of managed honey bee 2015–2016 annual colony losses in the USA. J. Apic. Res. 56, 328–340. doi: 10.1080/00218839.2017.1344496
Little, C. M., Shutler, D., and Williams, G. R. (2015). Associations among Nosema spp. fungi, Varroa destructor mites, and chemical treatments in honey bees, Apis mellifera. J. Apic. Res. 54, 378–385. doi: 10.1080/00218839.2016.1159068
Long, E. Y., and Krupke, C. H. (2016). Non-cultivated plants present a season-long route of pesticide exposure for honey bees. Nat. Commun. 7:11629. doi: 10.1038/ncomms11629
Maher, R. L., Rice, M. M., McMinds, R., Burkepile, D. E., and Thurber, R. V. (2019). Multiple stressors interact primarily through antagonism to drive changes in the coral microbiome. Sci. Rep. 9:6834. doi: 10.1038/s41598-019-43274-8
Medrzycki, P., Giffard, H., Aupinel, P., Belzunces, L. P., Chauzat, M.-P., Claßen, C., et al. (2013). Standard methods for toxicology research in Apis mellifera. J. Apic. Res. 52, 1–60. doi: 10.3896/IBRA.1.52.4.14
Minnameyer, A., Strobl, V., Bruckner, S., Camenzind, D. W., Van Oystaeyen, A., Wäckers, F., et al. (2021). Eusocial insect declines: insecticide impairs sperm and feeding glands in bumblebees. Sci. Total Environ. 785:146955. doi: 10.1016/j.scitotenv.2021.146955
Mitchell, E. A. D., Mulhauser, B., Mulot, M., Mutabazi, A., Glauser, G., and Aebi, A. (2017). A worldwide survey of neonicotinoids in honey. Science 358, 109–111. doi: 10.1126/science.aan3684
Mogren, C. L., and Lundgren, J. G. (2016). Neonicotinoid-contaminated pollinator strips adjacent to cropland reduce honey bee nutritional status. Sci. Rep. 6:29608. doi: 10.1038/srep29608
Morfin, N., Goodwin, P. H., and Guzman-Novoa, E. (2020). Interaction of field realistic doses of clothianidin and Varroa destructor parasitism on adult honey bee (Apis mellifera L.) health and neural gene expression, and antagonistic effects on differentially expressed genes. PLoS One 15:e0229030. doi: 10.1371/journal.pone.0229030
O’Donnell, S., and Beshers, S. N. (2004). The role of male disease susceptibility in the evolution of haplodiploid insect societies. Proc. R. Soc. Lond. B Biol. Sci. 271, 979–983. doi: 10.1098/rspb.2004.2685
Pang, S., Lin, Z., Zhang, W., Mishra, S., Bhatt, P., and Chen, S. (2020). Insights into the microbial degradation and biochemical mechanisms of neonicotinoids. Front. Microbiol. 11:868. doi: 10.3389/fmicb.2020.00868
Pettis, J. S., Lichtenberg, E. M., Andree, M., Stitzinger, J., Rose, R., vanEngelsdorp, D., et al. (2013). Crop pollination exposes honey bees to pesticides which alters their susceptibility to the gut pathogen Nosema ceranae. PLoS One 8:e70182. doi: 10.1371/journal.pone.0070182
Pettis, J. S., Rice, N., Joselow, K., vanEngelsdorp, D., and Chaimanee, V. (2016). Colony failure linked to low sperm viability in honey bee (Apis mellifera) queens and an exploration of potential causative factors. PLoS One 11:e0147220. doi: 10.1371/journal.pone.0147220
Piggott, J. J., Townsend, C. R., and Matthaei, C. D. (2015). Reconceptualizing synergism and antagonism among multiple stressors. Ecol. Evol. 5, 1538–1547. doi: 10.1002/ece3.1465
Pilling, E., Campbell, P., Coulson, M., Ruddle, N., and Tornier, I. (2013). A four-year field program investigating long-term effects of repeated exposure of honey bee colonies to flowering crops treated with thiamethoxam. PLoS One 8:e77193. doi: 10.1371/journal.pone.0077193
Poulin, R. (2007). Evolutionary Ecology of Parasites, 2nd Edn. Princeton, NJ: Princeton University Press.
Prisco, G. D., Cavaliere, V., Annoscia, D., Varricchio, P., Caprio, E., Nazzi, F., et al. (2013). Neonicotinoid clothianidin adversely affects insect immunity and promotes replication of a viral pathogen in honey bees. Proc. Natl. Acad. Sci. U.S.A. 110, 18466–18471. doi: 10.1073/pnas.1314923110
Ramsey, S. D., Ochoa, R., Bauchan, G., Gulbronson, C., Mowery, J. D., Cohen, A., et al. (2019). Varroa destructor feeds primarily on honey bee fat body tissue and not hemolymph. Proc. Natl. Acad. Sci. U.S.A. 116, 1792–1801. doi: 10.1073/pnas.1818371116
Rangel, J., and Fisher, A. (2019). Factors affecting the reproductive health of honey bee (Apis mellifera) drones—a review. Apidologie 50, 759–778. doi: 10.1007/s13592-019-00684-x
Retschnig, G., Williams, G. R., Mehmann, M. M., Yañez, O., de Miranda, J. R., and Neumann, P. (2014). Sex-specific differences in pathogen susceptibility in honey bees (Apis mellifera). PLoS One 9:e85261. doi: 10.1371/journal.pone.0085261
Rhodes, J. W., Harden, S., Spooner-Hart, R., Anderson, D. L., and Wheen, G. (2011). Effects of age, season and genetics on semen and sperm production in Apis mellifera drones. Apidologie 42, 29–38. doi: 10.1051/apido/2010026
Rinderer, T. E., De Guzman, L., Lancaster, V., Delatte, G. T., and Stelzer, J. A. (1999). Varroa in the mating yard: I. The effects of Varroa jacobsoni and Apistan§on drone honey bees. Am. Bee J. 139, 134–139.
Rosenkranz, P., Aumeier, P., and Ziegelmann, B. (2010). Biology and control of Varroa destructor. J. Invertebr. Pathol. 103(Suppl. 1), S96–S119. doi: 10.1016/j.jip.2009.07.016
Ruttner, F. (1966). The life and flight activity of drones. Bee World 47, 93–100. doi: 10.1080/0005772X.1966.11097111
Sanchez-Bayo, F., and Goka, K. (2014). Pesticide residues and bees–a risk assessment. PLoS One 9:e94482. doi: 10.1371/journal.pone.0094482
Sandrock, C., Tanadini, M., Tanadini, L. G., Fauser-Misslin, A., Potts, S. G., and Neumann, P. (2014). Impact of chronic neonicotinoid exposure on honey bee colony performance and queen supersedure. PLoS One 9:e103592. doi: 10.1371/journal.pone.0103592
Schaafsma, A., Limay-Rios, V., Baute, T., Smith, J., and Xue, Y. (2015). Neonicotinoid insecticide residues in surface water and soil associated with commercial maize (Corn) fields in Southwestern Ontario. PLoS One 10:e0118139. doi: 10.1371/journal.pone.0118139
Schlüns, H., Moritz, R. F. A., Neumann, P., Kryger, P., and Koeniger, G. (2005). Multiple nuptial flights, sperm transfer and the evolution of extreme polyandry in honeybee queens. Anim. Behav. 70, 125–131. doi: 10.1016/j.anbehav.2004.11.005
Siede, R., Meixner, M. D., Almanza, M. T., Schoening, R., Maus, C., and Buechler, R. (2018). A long-term field study on the effects of dietary exposure of clothianidin to varroosis-weakened honey bee colonies. Ecotoxicology 27, 772–783. doi: 10.1007/s10646-018-1937-1
Simon-Delso, N., Amaral-Rogers, V., Belzunces, L. P., Bonmatin, J. M., Chagnon, M., Downs, C., et al. (2015). Systemic insecticides (neonicotinoids and fipronil): trends, uses, mode of action and metabolites. Environ. Sci. Pollut. Res. 22, 5–34. doi: 10.1007/s11356-014-3470-y
Simone-Finstrom, M., Walz, M., and Tarpy, D. R. (2016). Genetic diversity confers colony-level benefits due to individual immunity. Biol. Lett. 12:20151007. doi: 10.1098/rsbl.2015.1007
Singla, A., Barmota, H., Sahoo, S. K., and Kang, B. K. (2021). Influence of neonicotinoids on pollinators: a review. J. Apic. Res. 60, 19–32. doi: 10.1080/00218839.2020.1825044
Siviter, H., Bailes, E. J., Martin, C. D., Oliver, T. R., Koricheva, J., Leadbeater, E., et al. (2021). Agrochemicals interact synergistically to increase bee mortality. Nature 596:389–392. doi: 10.1038/s41586-021-03787-7
Steinhauer, N., Kulhanek, K., Antúnez, K., Human, H., Chantawannakul, P., Chauzat, M.-P., et al. (2018). Drivers of colony losses. Curr. Opin. Insect Sci. 26, 142–148. doi: 10.1016/j.cois.2018.02.004
Stoner, K. A., and Eitzer, B. D. (2012). Movement of soil-applied imidacloprid and thiamethoxam into nectar and pollen of squash (Cucurbita pepo). PLoS One 7:e39114. doi: 10.1371/journal.pone.0039114
Straub, L., Minnameyer, A., Strobl, V., Kolari, E., Friedli, A., Kalbermatten, I., et al. (2020). From antagonism to synergism: extreme differences in stressor interactions in one species. Sci. Rep. 10:4667. doi: 10.1038/s41598-020-61371-x
Straub, L., Villamar-Bouza, L., Bruckner, S., Chantawannakul, P., Gauthier, L., Khongphinitbunjong, K., et al. (2016). Neonicotinoid insecticides can serve as inadvertent insect contraceptives. Proc. R. Soc. B Biol. Sci. 283:20160506. doi: 10.1098/rspb.2016.0506
Straub, L., Villamar-Bouza, L., Bruckner, S., Chantawannakul, P., Kolari, E., Maitip, J., et al. (2021). Negative effects of neonicotinoids on male honeybee survival, behaviour and physiology in the field. J. Appl. Ecol. 1–14. doi: 10.1111/1365-2664.14000
Straub, L., Williams, G. R., Vidondo, B., Khongphinitbunjong, K., Retschnig, G., Schneeberger, A., et al. (2019). Neonicotinoids and ectoparasitic mites synergistically impact honeybees. Sci. Rep. 9:8159. doi: 10.1038/s41598-019-44207-1
Sur, R., Stork, A., and Ag, B. C. (2003). Uptake, translocation and metabolism of imidacloprid in plants. Bull. Insectol. 56, 35–40.
Tarpy, D. R. (2003). Genetic diversity within honeybee colonies prevents severe infections and promotes colony growth. Proc. R. Soc. Lond. B Biol. Sci. 270, 99–103. doi: 10.1098/rspb.2002.2199
Tarpy, D. R., and Seeley, T. D. (2006). Lower disease infections in honeybee (Apis mellifera) colonies headed by polyandrous vs monandrous queens. Naturwissenschaften 93, 195–199. doi: 10.1007/s00114-006-0091-4
Tong, Z., Duan, J., Wu, Y., Liu, Q., He, Q., Shi, Y., et al. (2018). A survey of multiple pesticide residues in pollen and beebread collected in China. Sci. Total Environ. 640–641, 1578–1586. doi: 10.1016/j.scitotenv.2018.04.424
Traynor, K. S., Mondet, F., de Miranda, J. R., Techer, M., Kowallik, V., Oddie, M. A. Y., et al. (2020). Varroa destructor: a complex parasite, crippling honey bees worldwide. Trends Parasitol. 36, 592–606. doi: 10.1016/j.pt.2020.04.004
Tsvetkov, N., Samson-Robert, O., Sood, K., Patel, H. S., Malena, D. A., Gajiwala, P. H., et al. (2017). Chronic exposure to neonicotinoids reduces honey bee health near corn crops. Science 356, 1395–1397. doi: 10.1126/science.aam7470
van der Sluijs, J. P., Amaral-Rogers, V., Belzunces, L. P., Bijleveld van Lexmond, M. F. I. J., Bonmatin, J.-M., Chagnon, M., et al. (2015). Conclusions of the worldwide integrated assessment on the risks of neonicotinoids and fipronil to biodiversity and ecosystem functioning. Environ. Sci. Pollut. Res. 22, 148–154. doi: 10.1007/s11356-014-3229-5
Wilfert, L., Long, G., Leggett, H. C., Schmid-Hempel, P., Butlin, R., Martin, S. J. M., et al. (2016). Deformed wing virus is a recent global epidemic in honeybees driven by Varroa mites. Science 351, 594–597. doi: 10.1126/science.aac9976
Williams, G. R., Alaux, C., Costa, C., Csáki, T., Doublet, V., Eisenhardt, D., et al. (2013). Standard methods for maintaining adult Apis mellifera in cages under in vitro laboratory conditions. J. Apic. Res. 52, 1–36. doi: 10.3896/IBRA.1.52.1.04
Williams, G. R., Troxler, A., Retschnig, G., Roth, K., Yañez, O., Shutler, D., et al. (2015). Neonicotinoid pesticides severely affect honey bee queens. Sci. Rep. 5:14621. doi: 10.1038/srep14621
Wood, T. J., and Goulson, D. (2017). The environmental risks of neonicotinoid pesticides: a review of the evidence post 2013. Environ. Sci. Pollut. Res. 24, 17285–17325. doi: 10.1007/s11356-017-9240-x
Wood, T. J., Kaplan, I., Zhang, Y., and Szendrei, Z. (2019). Honeybee dietary neonicotinoid exposure is associated with pollen collection from agricultural weeds. Proc. R. Soc. B Biol. Sci. 286:20190989. doi: 10.1098/rspb.2019.0989
Keywords: honey bee, drone, neonicotinoid, thiamethoxam, Varroa destructor, parasite, interaction
Citation: Bruckner S, Straub L, Neumann P and Williams GR (2021) Synergistic and Antagonistic Interactions Between Varroa destructor Mites and Neonicotinoid Insecticides in Male Apis mellifera Honey Bees. Front. Ecol. Evol. 9:756027. doi: 10.3389/fevo.2021.756027
Received: 09 August 2021; Accepted: 29 September 2021;
Published: 19 November 2021.
Edited by:
Alison McAfee, North Carolina State University, United StatesCopyright © 2021 Bruckner, Straub, Neumann and Williams. This is an open-access article distributed under the terms of the Creative Commons Attribution License (CC BY). The use, distribution or reproduction in other forums is permitted, provided the original author(s) and the copyright owner(s) are credited and that the original publication in this journal is cited, in accordance with accepted academic practice. No use, distribution or reproduction is permitted which does not comply with these terms.
*Correspondence: Selina Bruckner, c3piMDEzMEBhdWJ1cm4uZWR1
Disclaimer: All claims expressed in this article are solely those of the authors and do not necessarily represent those of their affiliated organizations, or those of the publisher, the editors and the reviewers. Any product that may be evaluated in this article or claim that may be made by its manufacturer is not guaranteed or endorsed by the publisher.
Research integrity at Frontiers
Learn more about the work of our research integrity team to safeguard the quality of each article we publish.