- 1Honey Bee Health Research Group, School of Molecular Sciences, The University of Western Australia, Perth, WA, Australia
- 2The Cooperative Research Centre for Honey Bee Products Limited, Perth, WA, Australia
- 3Institute of Integrative Biology, ETH Zürich, Zurich, Switzerland
- 4Center for Integrative Bee Research (CIBER), Department of Entomology, University of California Riverside, Riverside, CA, United States
Honey bees can host a remarkably large number of different parasites and pathogens, and some are known drivers of recent declines in wild and managed bee populations. Here, we studied the interactions between the fungal pathogen Nosema apis and seminal fluid of the Western honey bee (Apis mellifera). Honey bee seminal fluid contains multiple antimicrobial molecules that kill N. apis spores and we therefore hypothesized that antimicrobial activities of seminal fluid are genetically driven by interactions between honey bee genotype and different N. apis strains/ecotypes, with the virulence of a strain depending on the genotype of their honey bee hosts. Among the antimicrobials, chitinases have been found in honey bee seminal fluid and have the predicted N. apis killing capabilities. We measured chitinase activity in the seminal fluid of eight different colonies. Our results indicate that multiple chitinases are present in seminal fluid, with activity significantly differing between genotypes. We therefore pooled equal numbers of N. apis spores from eight different colonies and exposed subsamples to seminal fluid samples from each of the colonies. We infected males from each colony with seminal fluid exposed spore samples and quantified N. apis infections after 6 days. We found that host colony had a stronger effect compared to seminal fluid treatment, and significantly affected host mortality, infection intensity and parasite prevalence. We also found a significant effect of treatment, as well as a treatment × colony interaction when our data were analyzed ignoring cage as a blocking factor. Our findings provide evidence that N. apis-honey bee interactions are driven by genotypic effects, which could be used in the future for breeding purposes of disease resistant or tolerant honey bee stock.
Introduction
Honey bees are important pollinators of both natural and agricultural ecosystems (Biesmeijer et al., 2006; Klein et al., 2007; Potts et al., 2010; Ollerton et al., 2011). However, an increase in colony loss rates over the last two decades has raised concerns (vanEngelsdorp et al., 2010; van der Zee et al., 2012; Brodschneider et al., 2018; Gray et al., 2019, 2020). Research into the causes of high colony losses has identified multiple factors as well as interactions between them (Potts et al., 2010; Goulson et al., 2015), including pesticides (van der Sluijs et al., 2013; Woodcock et al., 2017); inferior beekeeping practices (Jacques et al., 2017); and environmental degradation (Thimmegowda et al., 2020), such as habitat loss and fragmentation (Brown and Paxton, 2009), and climate change (Memmott et al., 2007; Le Conte and Navajas, 2008). Additionally, parasites and pathogens are generally accepted as a main driver of colony losses (Genersch, 2010; Genersch et al., 2010; Neumann and Carreck, 2010; Ratnieks and Carreck, 2010). Honey bees host a large variety of parasites, some having little or mild effects on individuals or hives while others are highly damaging, potentially triggering the collapse of the entire colony (Bailey, 1968; Schmid-Hempel, 1998; Higes et al., 2006, 2008; Cox-Foster et al., 2007; Chen and Siede, 2007; Genersch, 2010; Rosenkranz et al., 2010). The globally widespread fungal gut parasites Nosema apis and Nosema ceranae (Microsporidia) have been intensively studied over recent years (Fries et al., 2013; Goblirsch, 2018). Although they typically have low levels of virulence, they have been linked to major bee losses, particularly N. ceranae, which has been noted as a possible causal trigger of Colony Collapse Disorder (CCD) (Higes et al., 2008, 2009). Although recent analyses reported different effects on honey bees when being exposed to multiple environmental stressors including parasites (Bird et al., 2021; Siviter et al., 2021), Nosema infections become more detrimental to honey bees when they coincide with the presence of other environmental stressors such as pesticides (Aufauvre et al., 2012; Grassl et al., 2018; Al Naggar and Baer, 2019).
Honey bees are not defenseless, however, and possess an innate immune system, consisting of a cellular response, e.g., encapsulation and phagocytosis, as well as a humoral response, for example, hemolymph coagulation, or the production of antimicrobial peptides (Hoffmann, 1995; Hoffmann et al., 1996). The innate immune systems of invertebrates are often believed to consist of rather generic antimicrobial molecules that provide their hosts with broader but non-specific protection against parasites (Medzhitov and Janeway, 2000; Hoffmann, 2003; Lemaitre and Hoffmann, 2007). However, more recent research indicates that invertebrate innate immune responses, including insects like the honey bee, are more complex than initially thought, with evidence for some level of specificity and some form of immune memory being present (Kurtz, 2005; Cooper and Eleftherianos, 2017).
Previous studies on the effects of N. apis on honey bees found that the parasite can contaminate ejaculates (Peng et al., 2015) and trigger new infections if transferred to queens during mating (Roberts et al., 2015). Interestingly, seminal fluid contains a substantial number of immune proteins and metabolites and is indeed capable of neutralizing N. apis spores with high efficiency, but has no measurable effects on a non-parasitic fungi (Saccharomyces cerevisiae) or bacteria (Arthrobacter globiformis and Escherichia coli) (Grassl et al., 2016). The immune system of male bees can not only recognize N. apis infections but raise an immune response that seems specifically tailored toward these parasites (Grassl et al., 2016). As a result, seminal fluid is remarkably efficient in killing N. apis, although some spores survive the exposure to seminal fluid and are able to successfully trigger new infections (Peng et al., 2016). One likely candidate involved in neutralizing N. apis spores are chitinases. These enzymes degrade chitin, a major component of insect exoskeletons and the cell wall of fungal spores (Vega and Kalkum, 2012; Hamid et al., 2013). The chitinase 5 protein (GB53565) has previously been identified in honey bee seminal fluid (Grassl et al., 2016). Chitinase could weaken and potentially rupture the chitin-based cell wall of N. apis spores, triggering premature germination, which has been previously reported for N. apis spores exposed to seminal fluid (Peng et al., 2016).
Based on previous research (Grassl et al., 2016; Peng et al., 2016) we here challenge the idea that innate immune responses offer broad and non-specific protection and conducted an experiment to further our understanding about the antimicrobial activity of honey bee seminal fluid. Our main aim was to find empirical support that the previously documented reduction of spore viability in response to seminal fluid exposure is resulting from more complex interactions, driven by genetic diversity in immune competence on the host side, as well as strain driven resistance to host defense molecules on the parasite side. If the antimicrobial activity of seminal fluid is determined by such genetic N. apis × honey bee interactions, then we expect firstly, that the seminal fluid from different bee genotypes acts as a “filter” for N. apis strains, targeting and neutralizing some strains but not being able to successfully recognize and combat resistant strains. Secondly, host bees infected with individual strains are expected to vary in their levels of resistance, with a specific host’s susceptibility being dependent on the parasite strain/isolate (Figure 1). The presence of innate immunity proteins in seminal fluid (Grassl et al., 2016) provides a possible genomic driver of these interactions and therefore offers the possibility to empirically test these predicted outcomes.
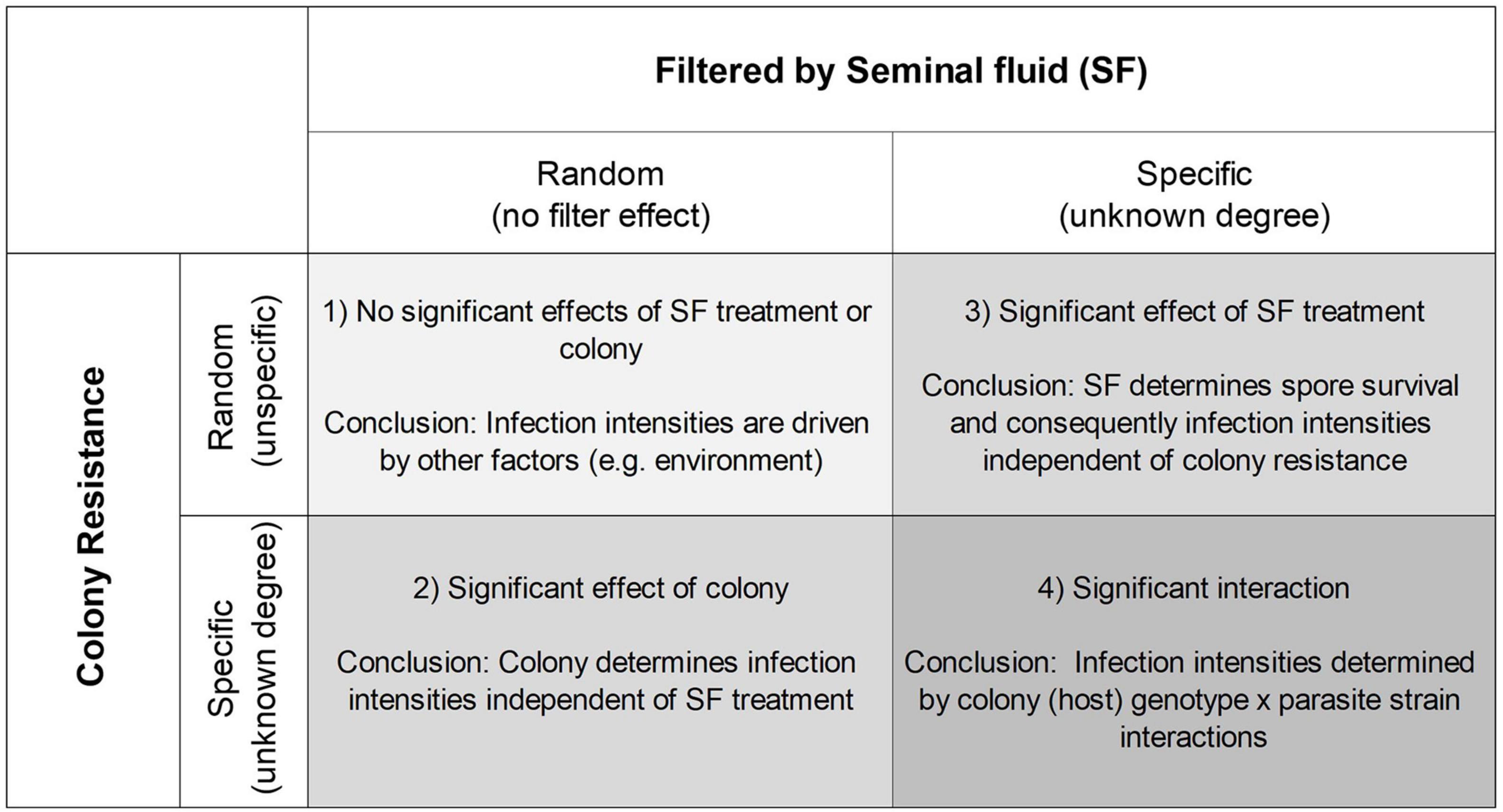
Figure 1. Predicted experimental outcomes depending on whether seminal fluid (SF) from different genotypes filters Nosema apis spores, creating different subsets of spores, and whether the hosts (colony) resistance is random or specific toward these spore isolates.
Disentangling these effects is challenging, and we used a common garden design to separate effects of seminal fluid exposure, host genetics and interactions between them on parasite infectivity and virulence. We exposed a large mixture of N. apis spores (strains) to the seminal fluid of eight genetically different bee colonies (genotypes), before using them to inoculate male bees from each of the eight source colonies and quantifying spore infectivity and virulence 6 days post infection. There are four possible outcomes being that spore virulence is (1) driven entirely by non-genetic factors, i.e., colony resistance is driven by other factors and/or seminal fluid does not have the predicted strain filtering effect, (2) driven by resistance that is defined by host colony with no or minimal filtering effects of seminal fluid, (3) driven by a highly specific filtering effect of seminal fluid with colony having little or no significant effects, and (4) parasite infectivity being determined by significant effects of both colony and seminal fluid identity (Figure 1). The latter outcome aligns with theoretical considerations of classical host parasite interactions (Schmid-Hempel, 1998). Additionally, we quantified chitinase activity in seminal fluid and hypothesized that activity levels would differ between bee genotypes.
Materials and Methods
All experiments were conducted between September 2016 and April 2017, using Western honey bee (Apis mellifera ligustica) stock kept in an apiary at The University of Western Australia in Perth. We used a total of eight genetically distinct colonies of comparable size that we inspected prior to any experimental work. This confirmed their general good health status as indicated by the presence of a single egg laying queen, worker and/or male brood, combs with stored honey and pollen as well as the absence of any pathological signs of disease that can be present in Western Australia, such as chalkbrood (Ascosphaera apis), sacbrood (Morator aetatulas), or American foulbrood (Paenibacillus larvae).
Drone Breeding and Seminal Fluid Collection
All experiments were conducted during the spring and summer season in Western Australia coinciding with the natural mating season of local honey bees. Following standardized procedures developed earlier (Williams et al., 2013), we bred males by restricting queens to only one male and two worker brood frames for 8 days to encourage queens to lay haploid male eggs. We removed frames containing capped male brood 21 days after the queen was first restricted to frames and transferred them to the lab where we kept them in an incubator at 32°C and ∼60% relative humidity (RH) for hatching. We collected newly hatched drones on a daily basis and transferred them to small wooden cages, separated by colony and placed them back into their maternal hives to allow males to sexually mature (Peng et al., 2015). We retrieved the males at an age of 12–15 days for semen collection (Figure 2) using methods developed earlier in the context of artificially inseminating honey bee queens (Mackensen and Roberts, 1948; Ruttner, 1976; Baer and Schmid-Hempel, 2000). In brief, we released the males into a flight cage and allowed them to fly for about 10 min, before we collected and anesthetized them with chloroform to initiate the ejaculation process (Baer et al., 2009). To collect semen, we gently squeezed the abdomen of males between two fingers until the ejaculate eventually appeared at the tip of the endophallus. We collected ejaculates with a glass capillary attached to a syringe and filled with Hayes solution (0.15 M NaCl, 1.80 mM CaCl2, 2.68 mM KCl, 1.19 mM NaHCO3, adjusted to pH 8.7 using NaOH). We pooled ejaculates of 50–170 males per colony and transferred them into an Eppendorf tube and centrifuged samples for 20 min at 20, 800 × g at 4°C. We collected the supernatant (seminal fluid) and stored all samples at −80°C prior to further experimentation. We continued with the collection of ejaculates until we had obtained a minimum of 50 μL of seminal fluid from each of the eight colonies.
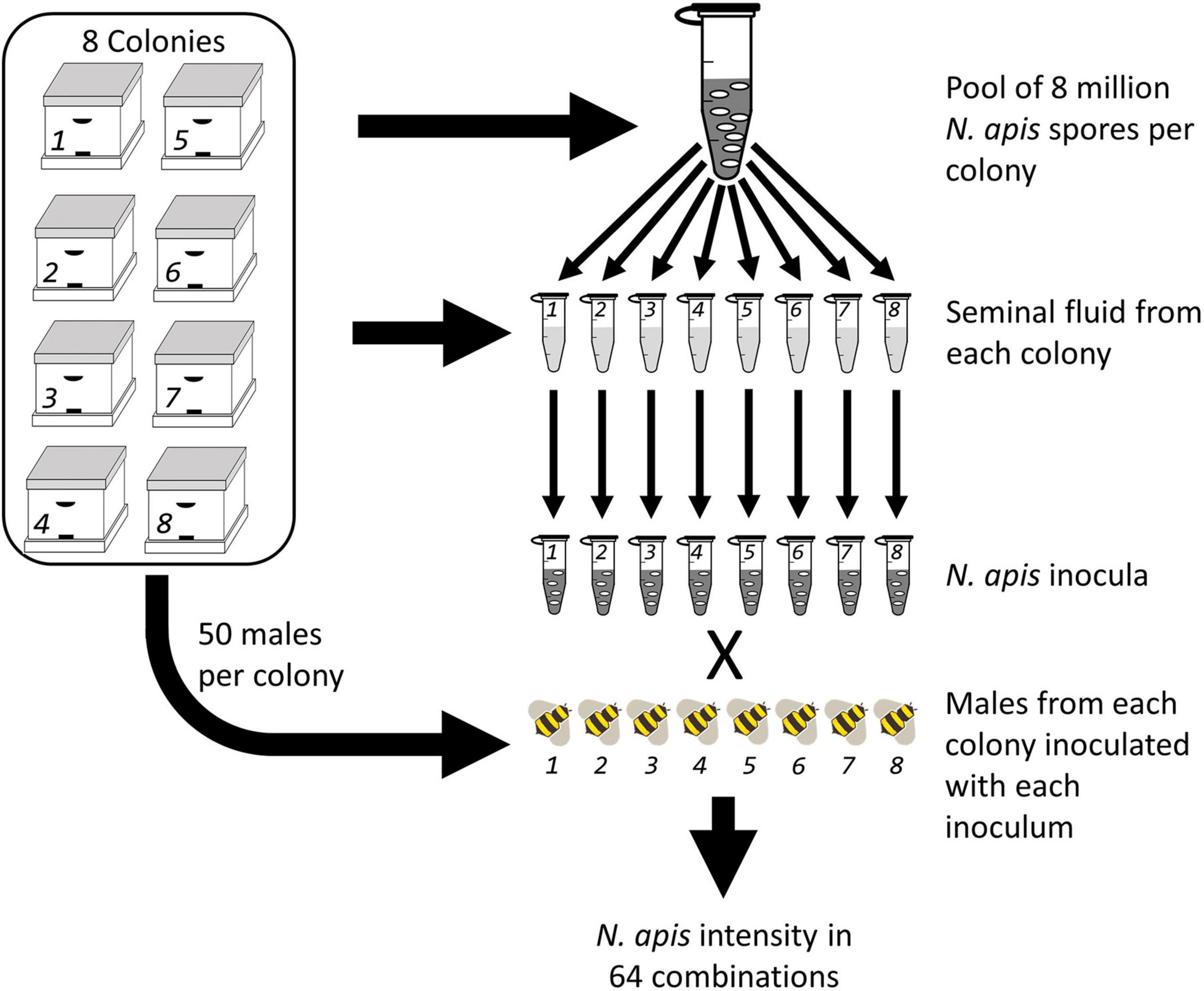
Figure 2. A fully crossed experimental design where eight colonies had Nosema apis spores collected and pooled, then aliquots of the master spore mix were treated with seminal fluid collected from each of the eight colonies creating eight inocula. Host drones were then bred from each colony and fed the eight inocula.
Nosema apis Spore Collection
To obtain a complete representation of N. apis spores from our experimental hives, we used a protocol developed earlier and as described in Peng et al. (2015). We collected a minimum of 50 honey bee workers from the entrances of each of the eight hives and freeze killed them at −20°C. Afterward, we dissected the midguts and pooled them into 2 mL Eppendorf tubes containing 1 mL of distilled de-ionized (DDi) water and added a 3 mm tungsten bead (Qiagen, Australia) to each sample. After briefly vortexing samples, we layered 0.5 mL of gut homogenate per colony onto 1.5 mL of 100% Percoll (Sigma-Aldrich, Australia), followed by centrifugation at 18,000 × g for 60 min at 4°C. We removed the supernatant and added 1.5 mL of DDi water to the pellet before vortexing and centrifugation for a second time at 20,700 × g for 5 min at 4°C. We repeated this step of washing the spore pellet an additional three times before we resuspended the final spore pellet in 100 μL of DDi water, and stored spores at −80°C. We quantified the spore concentration in each sample using a Neubauer hemocytometer (Laboroptic, United Kingdom). The above steps were repeated until we had collected a minimum of 8 million spores per colony, this required between 50 and 330 worker bees per colony. All N. apis spore samples were stored at –80°C prior to any further experimental work. Our earlier work confirmed that freezing of N. apis spores does not result in significant declines in their viability (Peng et al., 2014).
Parasite Exposure to Seminal Fluid
Prior to treating N. apis spores with the eight different seminal fluid samples, we created a N. apis master mix by pooling 8 million spores per colony (Figure 2). We mixed the spores by vortexing and aliquoted them into eight subsamples, containing 4.4 million spores each. These subsamples were then mixed with 44 μL of seminal fluid sample collected from each colony and incubated at room temperature in the dark for 5 min. The sample was vortexed again and aliquoted into eight more subsamples to be fed to male bees from each of the eight host colonies. These 64 inocula were stored at −20°C before using them for consequent male inoculations. Prior to the infection procedure, we thawed spores and diluted them with sucrose solution 100% (w/v) to a final concentration of 1,000 spores/μL.
Infection and Quantifying Spore Number
In order to quantify infectivity of our eight N. apis samples we fed them to male bees from the same eight colonies as previously used for seminal fluid and Nosema collections. We used male bees for this experiment because they develop from non-fertilized queen eggs, are haploid and therefore only carry maternal genes. This dramatically reduced the allelic diversity within our sample population of (male) hosts compared to diploid workers, especially since honeybee queens are polyandrous resulting in an average of 16–17 patrilines being represented in female offspring (Adams et al., 1977; Neumann and Moritz, 2000; Schlüns et al., 2005). We used newly hatched individuals for this experiment because previous work confirmed that they are free of N. apis infections (Bailey, 1955, 1968; Fries, 1993; Webster et al., 2008; Peng et al., 2015). To do this we bred a second round of males as described above (Figure 2). We used a fully crossed design, where we inoculated groups of brother males from every colony with N. apis spores treated with seminal fluid from drones from each of the eight colonies. Also, male bees were fed untreated N. apis spores to assess the effects of seminal fluid on spores. An additional male cohort of each colony was fed with sucrose solution as a control to confirm that infections are only present in individuals inoculated with spores. To do this we hand-fed an average of 52.29 (±1.33 SEM) males per treatment combination at an age between 1 and 5 days either 1,000 spores of N. apis in 1 μL of sucrose solution (100% w/v) or 1 μL of sucrose solution. Males were afterward kept in acrylic plastic cages, 100 mm × 75 mm × 120 mm (l × w × h), separated by colony and treatment to allow infections to develop. Males receive their food through workers via trophallaxis, and we therefore added 40 newly emerged workers from non-experimental colonies into each cage to support males during maturation (Williams et al., 2013). We kept all cages in an incubator at 32°C and ∼60% (RH) and provided bees with sucrose solution (150% w/v) ad libitum. We quantified mortality and removed dead bees on a daily basis. We freeze killed males 6 days post infection and stored them at −20°C.
We dissected the guts of thawed males and transferred them to an Eppendorf tube each containing 30–100 μL of DDi water and two 3 mm tungsten beads (Qiagen, Australia). The samples were vortexed until homogenized and 2 × 6 μL subsample was transferred with a pipette into a Neubauer hemocytometer (Laboroptic, United Kingdom). We counted the number of visible spores using a hemocytometer, in two technical replicates per male, using a Leica DM 1000 microscope at 40× magnification. We used the average number of spores counted in the technical replicates and calculated infection intensity (average number of counted spores × 50,000 = spores per 1 ml), i.e., the total number of spores present in an individual male. To confirm the absence of N. apis spores in controls, we dissected the guts of five drones from each colony which were fed sucrose solution only.
Chitinase Activity of Seminal Fluid Treatments
We used a commercially available chitinase assay kit (CS0980, Sigma-Aldrich) according to the manufacturer’s instructions to measure chitinase activities in seminal fluid. Because we were interested in the chitinase mode of action, i.e., exo- or endo-, and whether different genotypes (colonies) show different activity levels, we used all three substrates provided with the kit. 4-Nitrophenyl N-acetyl-β-D-glucosaminide and 4-Nitrophenyl N, N′-diacetyl-β-D-chitobioside both quantify exochitinase activity (specifically β-N-acetylglucosaminidase and chitobiosidase activity) whereas 4-Nitrophenyl β-D-N,N′,N″-triacetylchitotriose quantifies endochitinase activity. Protein concentration of seminal fluid samples were determined using a Bradford assay (#23238, Thermo Scientific, United States). Then, 1 μL of sample (0.6 μg of protein) was incubated with 99 μL of substrate solution for 30 min at 37°C. To stop the reaction 200 μL of 0.04 M sodium carbonate was added. We measured samples in a photo spectrometer at a wavelength of 405 nm. Chitinase activity was calculated as Units/mL = (Absorbance sample – Absorbance blank) × 0.05 × 0.3 × Dilution factor/Absorbance standard × Time × Volume of sample. One unit is defined as 1 μmole of p-nitrophenol released from the substrate per minute at pH 4.8 at 37°C.
Statistical Procedures
All statistical analyses were run using R version 3.4.1 for Macintosh (R Core Team, 2017). We analyzed our data using several different measures related to colony resistance and immune competence as follows: mortality rate being the fraction of bees that died during the experiment, parasite prevalence being the fraction of bees that became infected as well as infection intensity, being the number of spores present in the gut of each bee.
Chitinase Activity in Seminal Fluid
For seminal fluid chitinase activity, colony averages of activity for β-N-acetylglucosaminidase, chitobiosidase and endochitinase were analyzed using a linear mixed effect model with chitinase type as a fixed factor and colony as a random factor. Secondly we used one-way ANOVAs to compare the activity of chitinase (β-N-acetylglucosaminidase, chitobiosidase, and endochitinase) between colonies. Tukey’s method for post hoc analysis was used for multiple-comparisons between colonies and between chitinase type.
The Effect of Cages
We looked at the effect of housing bees in cages. A one-way ANOVA with cage as a fixed factor was used to assess the effect of housing bees in cages on mortality, infection intensity and probability of infection. Whereas average mortality was not different among cages (Supplementary Figure 1A and Supplementary Table 1A), average prevalence and infection intensity did vary among cages (Supplementary Figures 1B,C and Supplementary Tables 1B,C). The latter two measures were also correlated with one another on a per cage basis (Supplementary Figure 2). We therefore treated measures within a cage as non-independent. Consequently, with cages as a nesting factor, we could not estimate an interaction term for the factors “colony” and “SF treatment,” since one cage was identical to a particular interaction level (e.g., males from a specific colony being exposed to one of the Nosema spore treatments). Therefore, the main effects of colony and treatment were the focus of this study but we performed a second explorative analysis where cage was ignored as a blocking factor to investigate the interaction.
Host Resistance/Parasite Virulence Measures
We used one-way ANOVAs to compare mortality, infection intensities and probability of infection between seminal fluid-treated spores and controls (untreated spores). The effect of treatment (the origin of the seminal fluid applied) and colony as fixed factors for mortality, probability of infection and infection intensity were analyzed using a two-way ANOVA. For the infection intensity analysis, all individuals with no observed Nosema spores were removed from this analysis as “non-infected.” Prior to statistical analysis, we transformed mortality and probability of infection data using arcsine-square root of x (with x being the proportion of dead bees or proportion of infected bees per cage), and infection intensities using log(1 + x) (x, number of spores per bee) to normalize the data and meet the requirements for the analysis of variance. Infection intensities were averaged per cage to account for the cage effect. For infection intensities, a second analysis was performed using a linear mixed effect model, with colony and treatment as fixed effects and cage as a blocking (random) factor, and allowed individual bee intensities to be used. In addition to using the proportion of dead bees and the proportion of infected bees per cage we analyzed the effect of treatment and colony on infection prevalence (host status: “infected” vs. “not infected”) and the likelihood of survival for all animals using generalized linear models with cage as a random factor, using binomial distributions and the logit link function. However, the infection prevalence model did not converge enough to yield reliable results but could produce estimated effects of colonies and treatments.
In order to investigate potential host-parasite interactions we analyzed the data ignoring cage as a blocking factor, which allowed us to calculate the interaction term for the fixed factors colony and treatment. Infection intensities were analyzed using a two-way ANOVA with the fixed factors treatment and colony as well as their interaction. Infection prevalence was analyzed with a generalized linear model, using binomial distribution and the logit link function.
One-way ANOVAs were used to compare self (seminal fluid treatment from their own colony) and foreign effects (seminal fluid treatments from other colonies). We analyzed the relationship between chitinase activity of seminal fluid and colony host infection intensities using Pearson correlation. Finally, the relationship between average colony infection intensities and average seminal fluid treatment infection intensities were analyzed using Spearman correlation.
Results
Chitinase Activity of Seminal Fluid Treatments
To test the genotypic effects of antimicrobial activity in seminal fluid (SF) we first measured chitinase activity in SF pooled from eight colonies. Activities of β-N-acetylglucosaminidase, chitobiosidase and endochitinase in honey bee SF are shown in Figure 3. While both exo- and endo- chitinase activity were found in all colonies, the exochitinase β-N-acetylglucosaminidase had significantly higher activity compared to chitobiosidase (LMM, Tukey’s post hoc: z-value = 18.91, P < 0.0001), and endochitinase (z-value = 19.47, P < 0.0001). Honey bee genotype (colony) significantly affected endochitinase (one-way ANOVA: F7,8 = 5.42, P = 0.0149), β-N-acetylglucosaminidase (F7,8 = 9.88, P = 0.0022) and chitobiosidase (F7,8 = 17.07, P = 0.00032) activities (Table 1 and Figure 3).
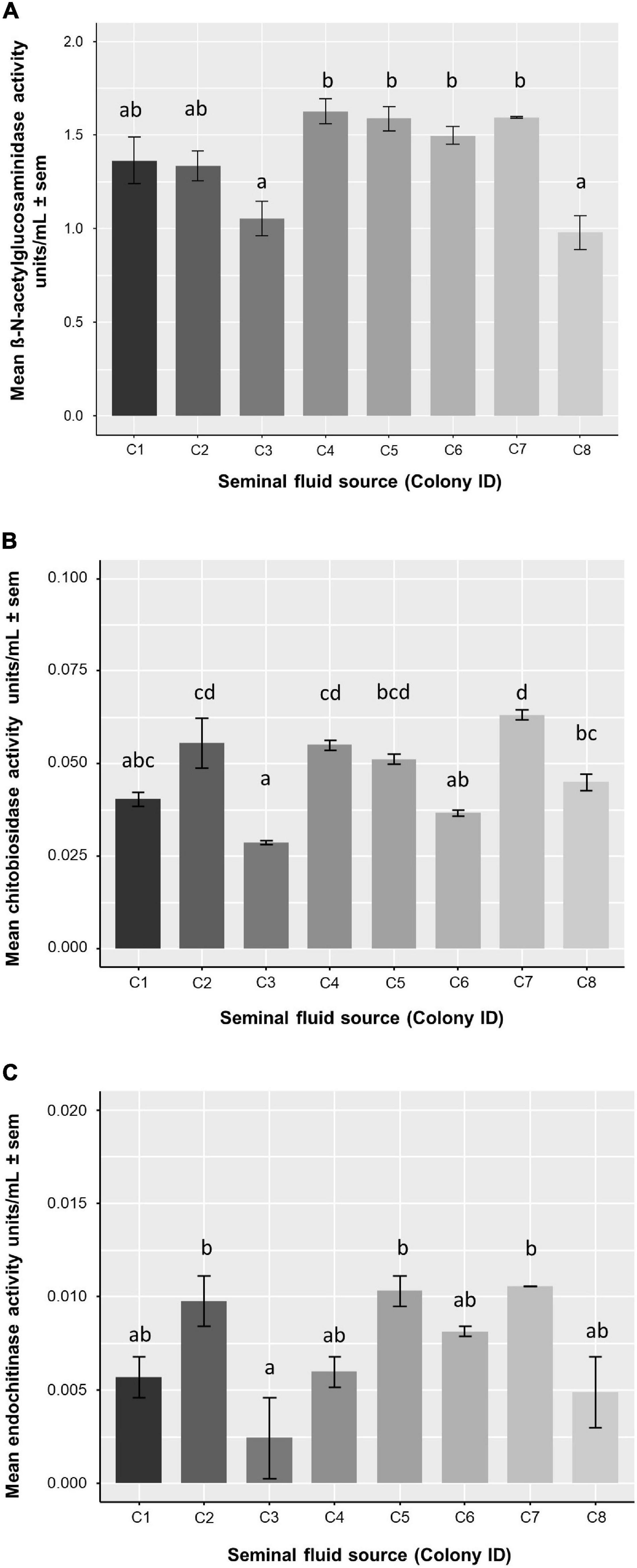
Figure 3. Mean chitinase activity of honey bee seminal fluid per colony (genotype). One-way ANOVAs were used for each type of enzymatic activity. Letters denote significance of post hoc analysis using the Tukey’s method for p-value adjustment. Sample size for each bar, n = 2. (A) β-N-acetylglucosaminidase activity was significantly different between colonies (F7,8 = 9.877, P = 0.0022) (B) Chitobiosidase activity was significantly different between colonies (F7,8 = 17.07, P = 0.00032) (C) Endochitinase activity was significantly different between colonies (F7,8 = 5.416, P = 0.0149).
Mortality Rate and Survival
A total of 3,733 male bees (466.62 ± 35.74 mean ± SEM per colony) became available for N. apis inoculations and a further 414 males (51.75 ± 5.43 mean ± SEM per colony) were fed sucrose solution as a control. Nosema apis spores were not detected in the control bees examined (five males per colony), confirming the expected absence of background infections. When we analyzed mortality rate 6 days post infection among all N. apis inoculated bees as a proxy for parasite virulence, we found a mean mortality of 12.6% (n = 3,733 bees). For the core set of eight colonies × eight SF treatments (in 64 cages), excluding bees fed untreated spores, the mean mortality was 12.8% (n = 3,351 bees). We analyzed mortality percentages and found no effect of SF treatment (two-way ANOVA: F7,49 = 1.35, P = 0.249), but colony had a significant effect (F7,49 = 10.21, P < 0.0001, Figure 4 and Supplementary Table 2A). Bees of colony C1 survived best, followed by C6, whereas those of colony C3 survived the least. Interestingly, treatment T3 (spores exposed to SF from colony 3) also had the lowest survival, and T6 had the highest survival probability (Figure 4). We also compared the mortality rate of N. apis inoculated bees to control bees (sucrose solution) and found parasite exposure did not significantly affect bee mortality (one-way ANOVA: F1,77 = 0.34, P = 0.56).
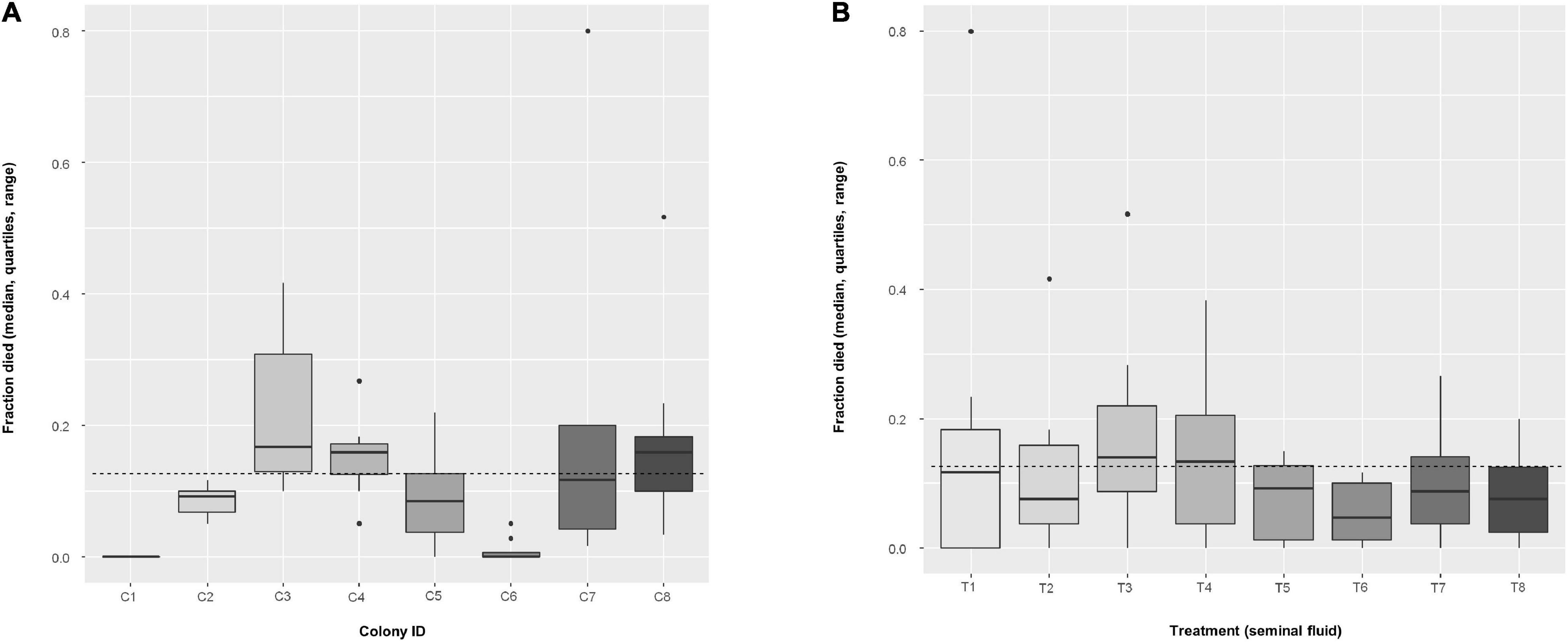
Figure 4. Boxplots of fraction of dead bees per cage (n = 8 cages, mean of 52.4 ± 1.4 bees per cage) using the non-transformed values, among (A) colonies, and (B) among treatments. Dashed line indicates overall mean. In an additive, two-way ANOVA with arcsin(sqrt)-transformed values, factor Colony had a significant effect (F7,49 = 10.213, P < 0.0001), but Treatment not (F7,49 = 1.348, P = 0.249).
In addition to using the average mortality rate per factor (Colony and Treatment), we also analyzed the likelihood of surviving until the reference day (6 days post infection). All bees in the experiment that were exposed to spores treated with SF were included (n = 3,351 bees), regardless of whether they were infected or not. We found that all colonies had highly significant effects (single-factor GLM: P < 0.0001), whereas no treatments were significantly different to the reference level (treatment T1; Figure 4). Further details are found in the Supplementary Table 4, and z-values for each colony comparison to the reference colony (C1) can be found in Supplementary Table 5.
Probability of Infection
The fraction of infected bees per cage at 6 days post infection varied among colonies (two-way ANOVA: F7,49 = 5.18, P = 0.0002) but not among treatments (F7,49 = 0.98, P = 0.453, Figure 5 and Supplementary Table 2B). A logistic regression analysis confirmed colony as a significant effect, finding all colonies significantly different to the reference colony (C1) (see Table 2 for colony z-values and P-values), but also found some treatment effects, with treatment T3 (z-value = −2.045, P = 0.041) and T4 (z-value = −2.55, P = 0.011) being significantly different to the reference treatment (T1) (Table 2). From Figure 5, colony C1 was particularly resistant to infection whereas colony C8 had a high prevalence (virtually all bees infected), the remainder being somewhere around a middle value. For treatments, SF from colony C1 (treatment T1) produced high prevalence across all colonies, whereas Treatment T3 and T4 impeded infection. Again, the remainder had effects around a mean value.
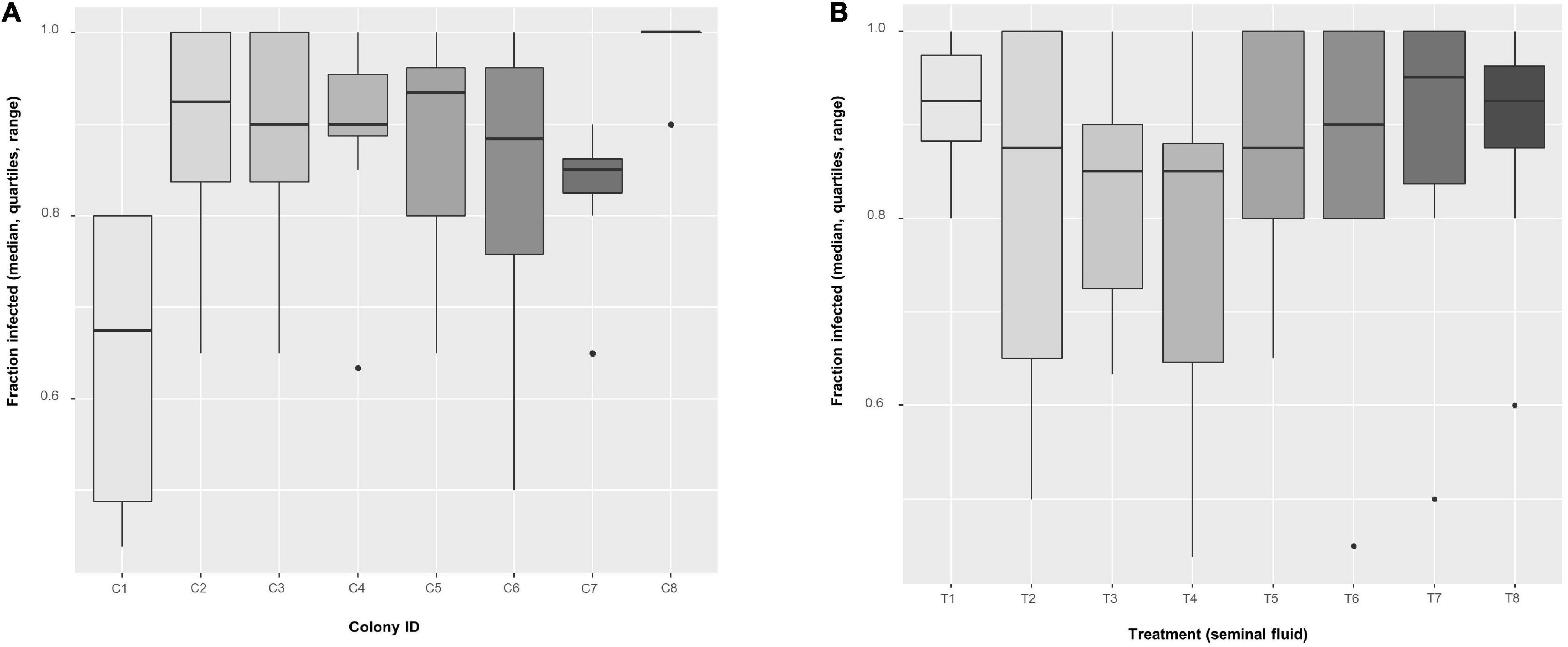
Figure 5. Boxplots of the fraction of infected bees per cage (n = 8 cages, mean of 52.4 ± 1.4 bees per cage) using the non-transformed values, among (A) colonies and (B) among treatments. In an additive, two-way ANOVA with arcsin(sqrt)-transformed values, factor Colony had a significant effect (F7,49 = 5.179, P = 0.0002), but Treatment not (F7,49 = 0.985, P = 0.453).
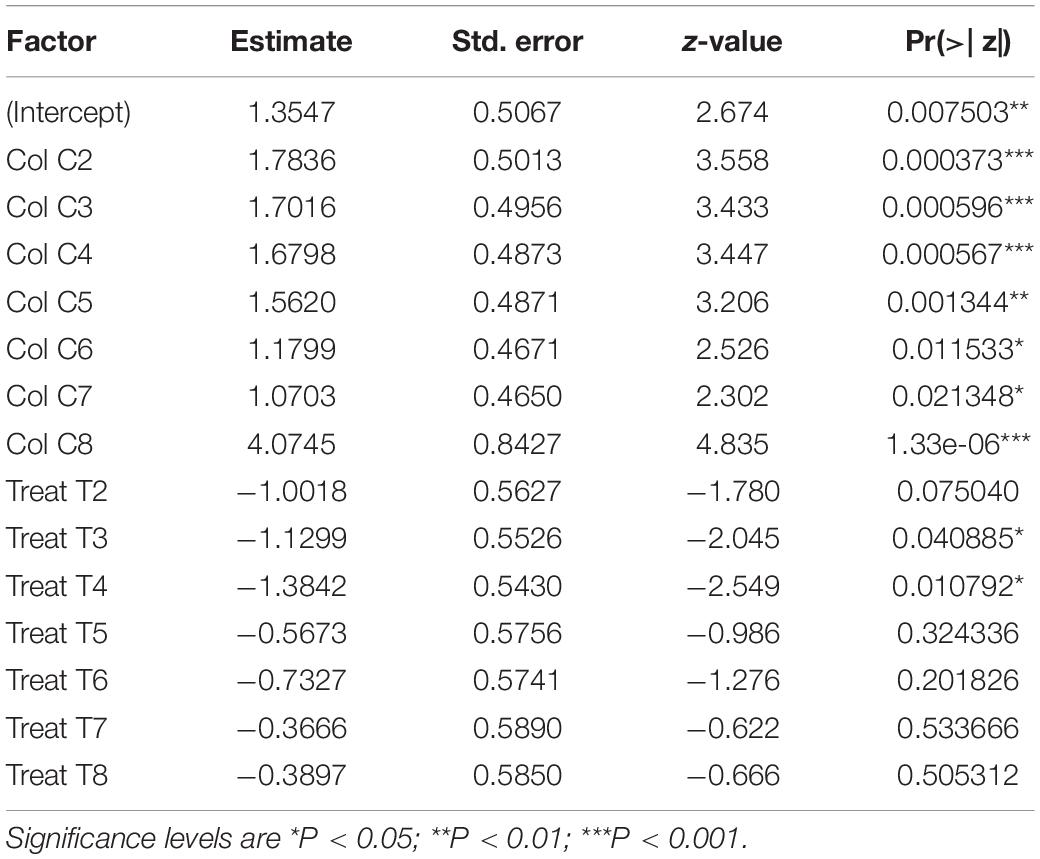
Table 2. Fixed effects for the probability of becoming infected, estimated from a GLM-model, with cage as blocked (random) factor, and colony and treatment as within-subject (fixed) factors.
Infection Intensity
We quantified intensity in a total of n = 1,481 male bees (n = 1,332 bees were infected with SF treated spores + 149 bees were infected with untreated spores). On average, we checked 20.81 ± 0.39 bees per colony and SF treatment combination. A total of n = 1,130 bees, with 17.65 ± 0.44 bees per colony and treatment combination, were found to be infected and no spores were found in the remainder. Hence, the average prevalence of infection in this set of treated spores was 84.8%. The following analyses are based on infected bees only.
In all, average infection intensity per cage varied among colonies (two-way ANOVA: F7,49 = 11.86, P < 0.0001) but not among SF treatments (F7,49 = 1.29, P = 0.276) (Figure 6 and Supplementary Table 2C). Three colonies (C1, C4, and C6) showed low spore intensities, whereas C8 was heavily infected. Treatment T4, i.e., SF coming from C4, appears to have the strongest effect on spore intensity. For confirmation, we also analyzed spore loads in each bee (with cage as the blocking (random) factor) and found that colony had a significant effect (LMM: F7,49 = 12.32, P < 0.0001) but not treatment (F7,49 = 1.348, P = 0.249; Table 3); echoing the analysis based on average infection intensity per cage. When we looked at the relationship between spore load and chitinase activity in SF from the same colony, we found that higher activity of the most abundant chitinase, β-N-acetylglucosaminidase, was significantly associated with lower host spore loads (Pearson’s r = −0.837, P = 0.0095, Figure 7A). The same was found for chitobiosidase and endochitinase activities although neither correlation was statistically significant (Pearson’s r = −0.218, P = 0.604, Figure 7B and Pearson’s r = −0.315, P = 0.45, Figure 7C).
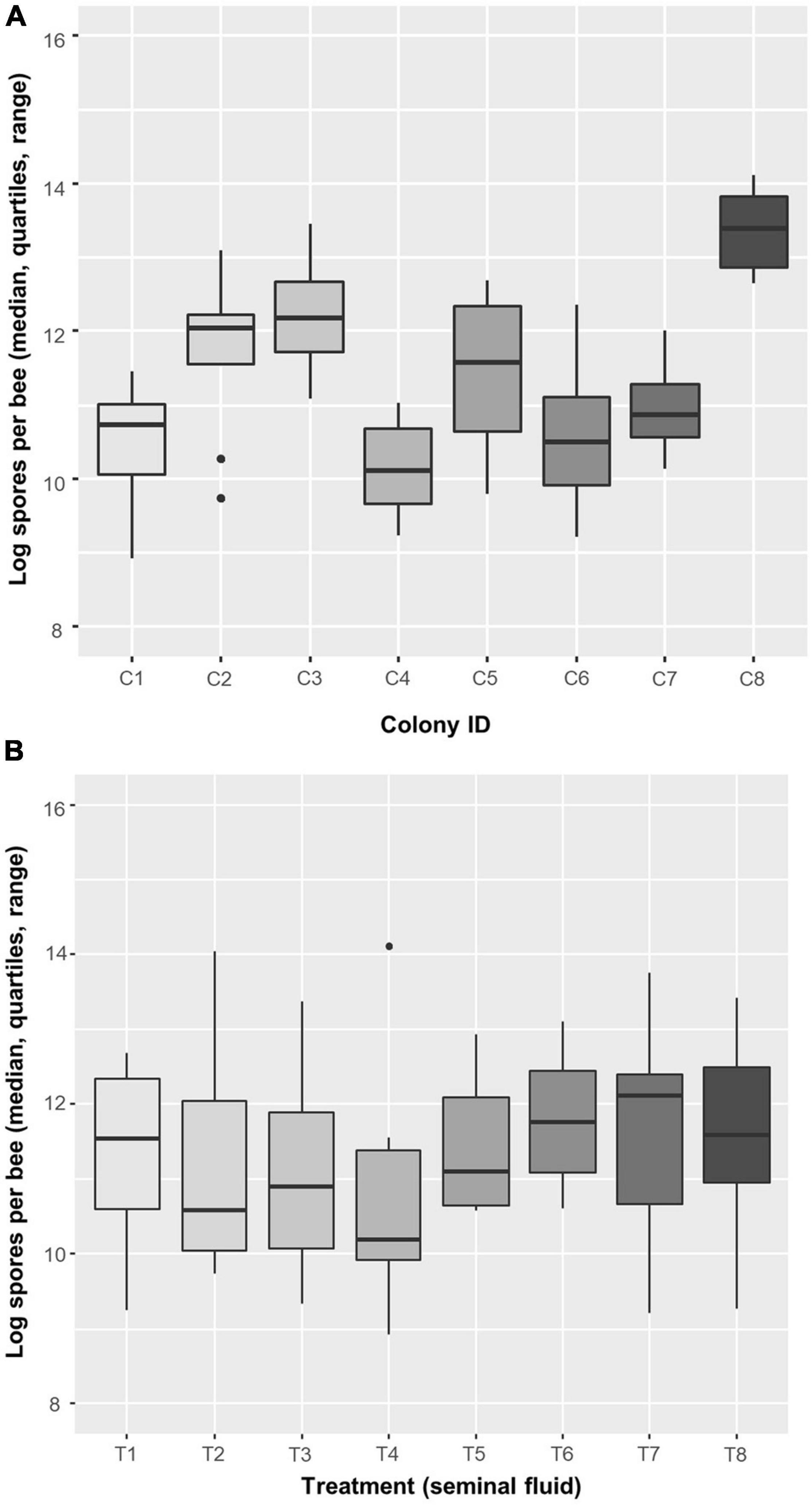
Figure 6. Boxplots of the mean infection intensity [log-transformed spores per bee; log(1 + spores)] per cage (n = 8 cages, mean of 52.4 ± 1.4 bees per cage) among (A) colonies and (B) treatments. In an additive, two-way ANOVA with log-transformed values, factor Colony had a significant effect (F7,49 = 11.862, P < 0.0001), but Treatment did not (F7,49 = 1.288, P = 0.276). Data are for infected bees only.
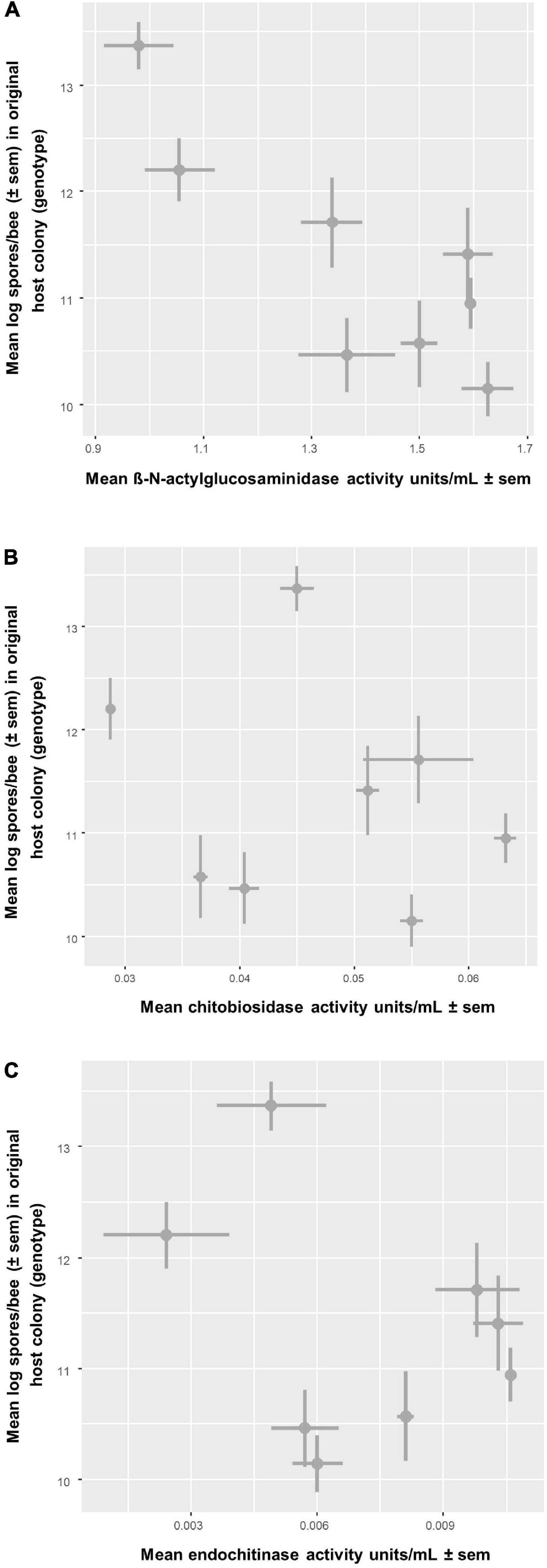
Figure 7. Relationship between chitinase activity of seminal fluid from a colony (x-axis) and mean infection intensity of Nosema apis inoculated bees from the same colony (y-axis). Infection intensity is the mean of cages per colony, n = 8 comparisons, and chitinase activity is the mean of technical replicates taken from a seminal fluid pool, n = 2. The plot shows the correlation with infection intensity and three different types of enzymatic activity: (A) β-N-acetylglucosaminidase activity had a significant negative correlation with infection intensity (Pearson’s r = –0.837, P = 0.0095) (B) Chitobiosidase activity had a negative correlation with infection intensity (Pearson’s r = –0.218, P = 0.604) (C) Endochitinase activity had a negative correlation with infection intensity (Pearson’s r = –0.315, P = 0.45).
Explorative Analysis for Host × Parasite Interactions in Infection Intensity and Probability of Infection
Figure 8 shows a summary plot for infection intensity of all infected bees. Looking at the results in more detail the colony effect is stronger on infection intensity than the SF treatments. When analyzing this graph by ignoring cage as a blocking factor, we found highly significant effects for both main factors (two-way ANOVA: Colony F7,1066 = 75.71, P < 0.0001 and Treatment F7,1066 = 9.91, P < 0.0001) and their interaction (F49,1066 = 5.60, P < 0.0001), again with colony having a stronger effect (Supplementary Table 6). When analyzing the probability of infection ignoring cage as a blocking factor, colony was not significant but there were significant effects of treatment T4 (GLM: z-value = −2.47, P = 0.0135) and T6 (z-value = −2.21, P = 0.0269), while none of the interaction terms reached significance (Supplementary Table 7).
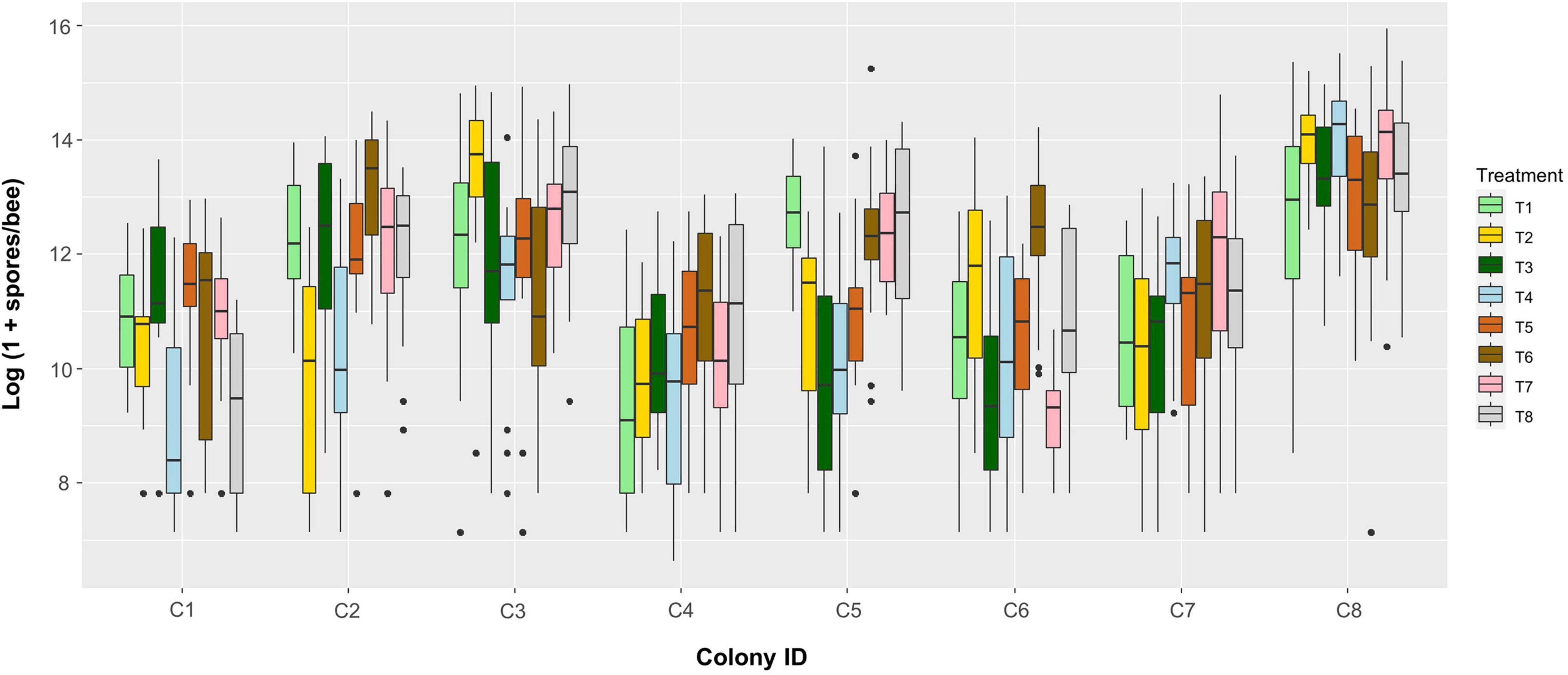
Figure 8. Boxplot of Nosema apis infection intensities (spores per bee, log-transformed) among colonies (bottom axis) and treatments (colors, see legend). Each box contains the data from one cage (n = 64 cages in total).
Comparing the Effects of Seminal Fluid on the Measures
The treatment where N. apis spores were not exposed to SF served as a control for the effect of applying specific SF, i.e., from a given colony. Average mortality per cage did not differ between exposing bees to spores previously treated by SF (mean = 0.116 ± 0.017 SE, n = 64 cages) or not exposing them (0.108 ± 0.04., n = 7) (one-way ANOVA: F1,69 = 0.0001, P = 0.99). The probability of infection, however, differed between controls (0.971 ± 0.019, n = 7) and those exposed to specific SF treated spores (0.853 ± 0.019, n = 64) (Figure 9A, one-way ANOVA: F1,69 = 5.57, P = 0.02). Similarly, mean infection intensity per cage was lower for specific SF treated spores (log(1 + x) spore load: 11.351 ± 0.165, n = 64) as compared to non-exposed spores (log(1 + x) spore load: 12.533 ± 0.367, n = 7) (Figure 9B, one-way ANOVA: F1,69 = 5.386, P = 0.023).
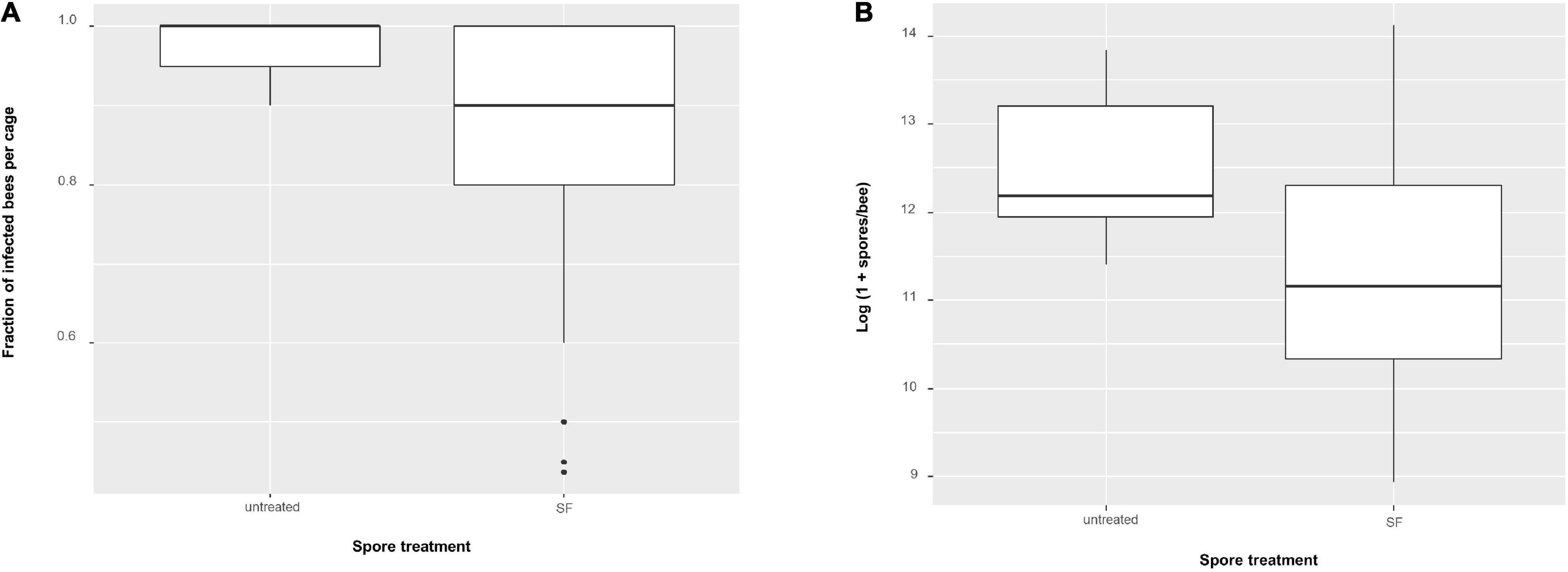
Figure 9. Boxplots showing (A) fraction of Nosema apis infected bees per cage using non-transformed values and (B) N. apis infection intensities (spores per bee, log-transformed). Comparing untreated spores (n = 7 cages) and seminal fluid (SF) treated spores (n = 64 cages).
Comparing Measures From Self vs. Foreign Combinations
There could be a difference such that colonies become more infected when spores were first treated with SF from their own colony as compared to the average effect of SF from all other colonies.
With the three measures, we found no difference in mean mortality between bees exposed to spores treated with SF from their own colony (0.077 ± 0.034, n = 8) as compared to when exposed to spores treated with SF from all other colonies (mean = 0.121 ± 0.019 SE, n = 56 cages) (one-way ANOVA: F1,62 = 0.321, P = 0.57). Similarly, no difference was found for the fraction of infected bees per cage if either exposed to spores treated with SF of their own colony (0.810 ± 0.058, n = 8) or exposed to spores treated with SF of all other colonies (0.859 ± 0.02, n = 56 cages) (one-way ANOVA: F1,62 = 0.63, P = 0.43). Also, no difference was found for the mean infection intensities in bees infected with spores treated with SF of their own colony [log(1 + x) spore load: 11.31 ± 0.513, n = 8] vs. spores treated with SF of all other colonies [log(1 + x) spore load: 11.36 ± 0.177, n = 56] (one-way ANOVA: F1,62 = 0.0075, P = 0.931). We also related the effect on infection intensities through each colony. However, no correlation between colony infection intensity and treatment infection intensity was found (Spearman’s r = 0.095, P = 0.84, Figure 10).
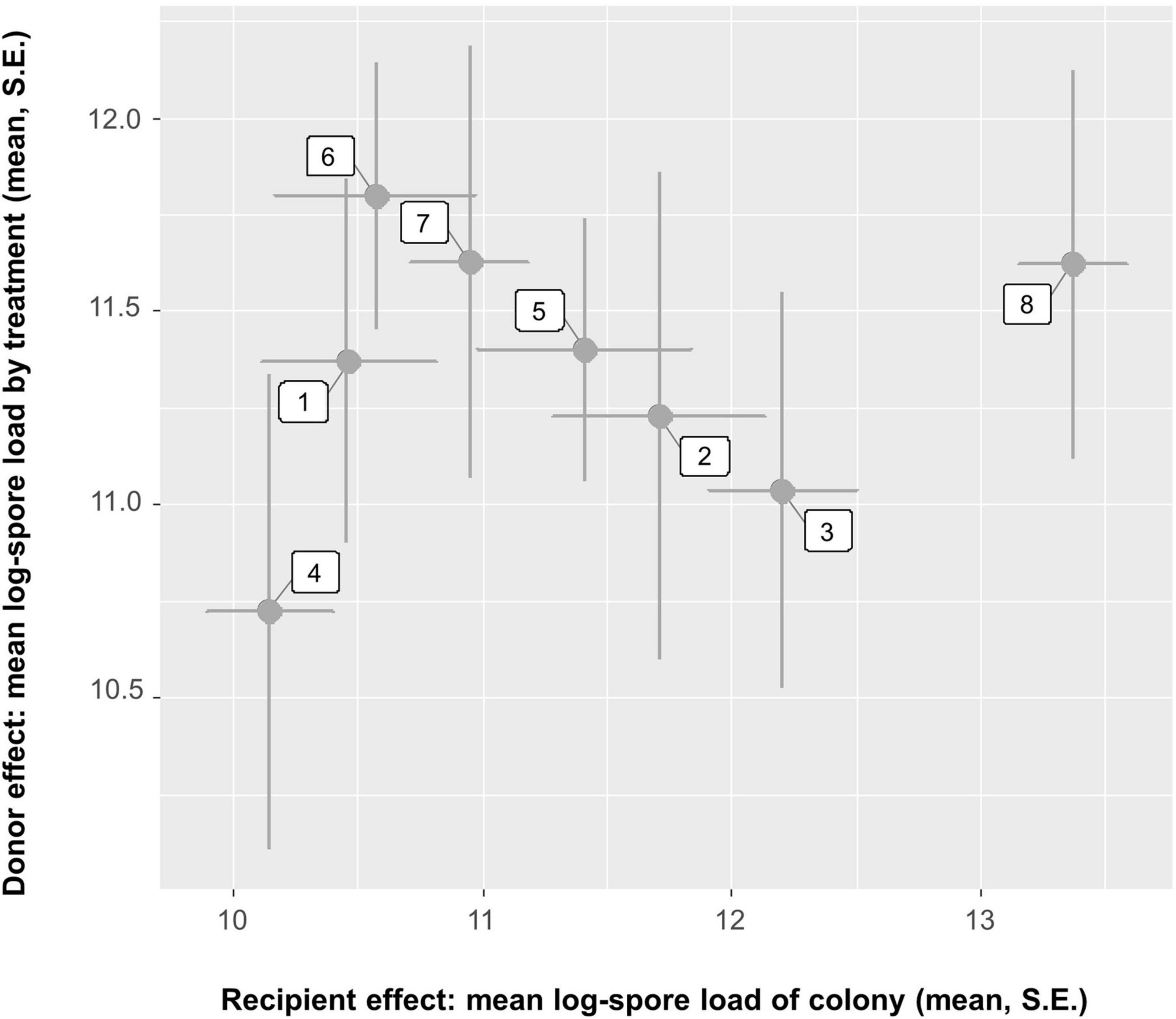
Figure 10. Relationship between exposing bees from a colony (x-axis) and applying seminal fluid from the same colony (y-axis) on the Nosema apis infection intensity in these bees. There is a negative correlation (Spearman’s r = 0.095, P = 0.84, n = 8 comparisons). The plot shows mean and SE per cage and combination.
Discussion
Although our overall analyses did not reveal significant effects for seminal fluid, our findings provide several lines of support that seminal fluid can indeed be defined as a genetically determined filter acting on N. apis spore genotypes, generating distinct spore subpopulations that differ in their ability to trigger infections in new hosts. In the paragraphs below, we summarize our results and discuss them within this framework:
1. Firstly, we assessed the genotypic diversity in chitinase activity of seminal fluid as a measure of antimicrobial activity. The main type of chitinase action in seminal fluid is unknown, so multiple substrates were used. We found that seminal fluid contains both exo- and endo- chitinase activity, implying that seminal fluid contains multiple chitinases which can cleave chitin in different ways, either at internal sites of the chain or externally from the non-reducing ends. The presence of several chitinases in seminal fluid is likely, considering the chitinase previously identified, chitinase 5 (GB53565) (Grassl et al., 2016), is an endochitinase, and in our present study we predominantly found exo- type activity. We found significantly higher β-N-acetylglucosaminidase activity compared to chitobiosidase or endochitinase. This enzyme progressively catalyses chitin hydrolysis, and often works alongside chitobiosidase or endochitinase, but here we find it be the main activity. As expected, there was a significant genotypic effect on β-N-acetylglucosaminidase activity, as seen with two colonies having significantly lower enzymatic activities.
We also found a significant negative correlation between colony infection intensity and β-N-acetylglucosaminidase activity of the same colony. We speculate that the β-N-acetylglucosaminidase activity in seminal fluid are markers for bee (genotype) resistance, as immune proteins identified in seminal fluid have also been identified in the somatic tissues of males (Grassl et al., 2016; Holt et al., in preparation). In fact, we could expect that chitinases are also produced in the gut, providing some form of local antifungal response toward ingested N. apis spores. Chitinases are known to be present in the honey bee gut, specifically the acidic mammalian chitinase (GB46749) and the chitinase 5 (GB53565) (Houdelet et al., 2021), the latter being the same chitinase identified in seminal fluid previously (Grassl et al., 2016). Larvae have also shown deregulation of the AmelCht chitinase (GB15116), in response to infection with the fungal pathogen Ascosphaera apis (chalkbrood), and this chitinase shows high sequence similarity to gut-specific chitinases identified in the locust (Locusta migratoria manilensis) and the mosquito (Anopheles gambiae) (Aronstein et al., 2010). Chitinases are present in the gut of other insects as well and can be involved in digestive processes and regulation of the peritrophic membrane (Shen and Jacobs-Lorena, 1997; Girard and Jouanin, 1999; Daimon et al., 2003; Zhu et al., 2008; Arakane and Muthukrishnan, 2010; Pesch et al., 2017).
2. We confirm our earlier findings that seminal fluid has significant antimicrobial effects because parasite prevalence and intensity were significantly lower in bees inoculated with seminal fluid exposed spores compared to non-exposed ones. Hence, applying the specific SF had, on average, more effect in reducing the infectiousness of N. apis spores than when spores had not been treated with SF at all. Moreover, there is a mechanistic basis for this (see point 1 above). Effects of seminal fluid exposure on N. apis intensities have been shown before (Peng et al., 2016), but we now confirm this to be the case for prevalence as well, i.e., the killing effect of seminal fluid was sometimes efficient enough to prevent infections in some of the inoculated hosts. Similar to previous work (Martín-Hernández et al., 2011; Peng et al., 2015), we also confirm an overall low virulence of N. apis in Western Australian honey bees, as parasite exposure did not significantly increase mortality in the first 6 days post infection. This relatively benign impact of the parasite on the host population could explain why we did not find stronger genetic effects in our analyses, given that parasite virulence is a key driver of host parasite coevolution. Additionally, higher mortalities have been reported with spore doses higher than what we used (Martín-Hernández et al., 2011).
3. Ignoring cage as a factor in our statistical analyses allowed us to calculate seminal fluid treatment × host genotype interaction for a tentative, explorative analysis. This interaction was statistically significant for infection intensity but not for probability of infection. Ignoring cage for the analysis of infection intensity and probability of infection has some justification, given the biology of N. apis. For local infections to be spreading within a given cage, the spores need to first replicate in the gut of the male bee, after which they are excreted when bees defecate, thereby contaminating surfaces and food sources where they can be a source of additional infections to other bees. Although it can take as little as 3 days post infection for new spores to be produced and released (Fries, 1988, 1989; Czekońska, 2007), the spore doses we used (1,000 spores/bee) typically take longer periods of time (≥4 days) before being able to spread to additional hosts (Fries, 1988). Given the additional time required for secondary infections to develop in their host, the earliest signs of any such infections would only become visible 6–8 days after our initial inoculation. Hence, we conclude that our observations of infection intensity and probability of infection reflect the outcome of the primary exposure as part of the experimental inoculation treatment and are therefore largely independent. However, this obviously ignores possible secondary effects of stress, or behavioral changes that might have taken place in different cages, and thus may have affected the final outcome according to cage. The significant seminal fluid treatment × host genotype interaction for infection intensity is in line with the idea of non-random antimicrobial effects of seminal fluid, and that the parasite fate inside a host is dependent on host genetics. Such genotype-by-genotype interactions are well documented in other invertebrates, such as the mosquito (Anopheles gambiae) and the malaria parasite Plasmodium falciparum (Lambrechts et al., 2005), the bumblebee (Bombus terrestris) and its gut parasite Crithidia bombi (Schmid-Hempel, 2001; Mallon et al., 2003; Sadd and Barribeau, 2013) or the freshwater crustacean Daphnia magna and its bacterial parasite Pasteuria ramosa (Carius et al., 2001). Future work is needed to confirm this finding, for example by replicating individual treatment combinations using replicates, which will allow to separate statistical effects of cage from treatment and host colony.
4. As expected, our data indicate that host colony had a stronger effect on our measurements compared to the seminal fluid treatment. Whereas our inoculated host male bees were able to recognize and combat infections over the timeframe of the experiment, seminal fluid as a secretion collected from non-infected males lacked this time frame and the host genomic capabilities to respond to the presence of N. apis spores in any adaptive way. Despite this, we still found significant effects for seminal fluid treatment for some combinations within our common garden matrix. A lack of knowledge about the genetic diversity present in our samples (both on the host and on the parasite level) and the absence of a clear-cut understanding of the underlying genomic mechanisms defining host resistance as well as parasite virulence precluded us from making accurate predictions about the expected outcomes within each of the cells of our common garden experiment (Figure 1). Our findings indicate that these effects are present but seem not as strong as might be anticipated. This implies that – although immune molecules present in bees show some form of specificity toward individual parasites or strains - they do not seem to be as strongly linked as seen in other organisms with more complex immune systems such as higher vertebrates. Our findings ask for further work to specifically identify the molecules responsible for the N. apis killing effect and quantify their genomic or proteomic variation, as well as to quantify differential spore survival between strains. This is feasible, as serial passage experiments can be used as selection regimes to generate different Nosema isolates that can then be studied comparatively, either phenotypically when presented to different host genotypes or molecularly as genetic differences in virulence genes.
5. Our experimental setup might be seen as somewhat artificial, given that males would never become inoculated with spores that were exposed to the seminal fluid of other males. Albeit true, we want to point out here that such filtering effects representing our experimental setup have biological relevance. First, Nosema is a sexually transmitted disease in honey bees and can be transferred to queens during mating and trigger new infections (Peng et al., 2015; Roberts et al., 2015). In such a scenario, the mixing of ejaculates and seminal fluids from multiple males within the sexual tract could result in Nosema spores becoming exposed to innate immune molecules from multiple males. This also means that even ejaculates of uninfected males are likely to get exposed to N. apis spores, and could partially explain why Grassl et al. (2016) found seminal fluid of uninfected males were capable of killing N. apis spores to a similar extent to that of infected males. As a result, queens face N. apis spores inside their sexual tract that have been exposed and filtered to some degree by the seminal fluids of her mates before these spores will eventually also become exposed to the innate immune system of the queen. Such an effect would provide an additional benefit of polyandry and genetic diversity among mating males, in addition to benefits that have already been documented on the colony level (Baer and Schmid-Hempel, 1999; Hughes and Boomsma, 2004; Seeley and Tarpy, 2007). Our research confirms the majority of immune proteins identified in seminal fluid (Grassl et al., 2016) are also present in the somatic tissue (Holt et al., in preparation). Therefore, ingested N. apis spores would undergo a comparable filtering effect in the midgut of individuals before they could infect epithelial cells to be replicated, and potentially passed on to nest mates. Genetic diversity of these immune molecules within members of the hives, especially among workers, might then define the transmission dynamics between individuals and the spread of N. apis through members of the colony. Our findings, therefore, provide some insights into the possible mechanisms that underlay earlier findings confirming significant beneficial effects of increased genetic diversity on parasitism in social insects (Shykoff and Schmid-Hempel, 1991; Liersch and Schmid-Hempel, 1998; Baer and Schmid-Hempel, 1999; Tarpy, 2003; Tarpy and Seeley, 2006; Seeley and Tarpy, 2007), as well as the evolution of polyandry in social insects with large and long-lived societies (Hamilton, 1987; Sherman et al., 1988; Schmid-Hempel, 1998; Brown and Schmid-Hempel, 2003).
This study provides evidence that the antimicrobial activity of seminal fluid in honey bees is non-random. This supports the idea that the innate immune system offers more than just broad and non-specific protection in insects. We provide empirical evidence that chitinases present in the seminal fluid could be the molecular agents that are drivers of the observed antimicrobial activity. Future studies could now focus to unravel the exact molecular mechanisms that allow these proteins to neutralize N. apis spores. It would also be interesting to unravel whether such differences affect male reproductive success and fitness, for example by quantifying how differences in immune competence between males impacts their survival and mating flight activities. The identification of individual antimicrobial molecules and a general understanding about their functioning as Nosema killing agents could also be used in the future for the development of novel medications. Naturally occurring variation in antimicrobial activity of these molecules could be exploited to maximize their efficiency or develop targeted treatments, for example against particular virulent strains.
Data Availability Statement
The raw data supporting the conclusions of this article will be made available by the authors, without undue reservation.
Author Contributions
BB and PS-H designed the study. SH, NC, BB, and JG conducted the experiments. PS-H, SH, and JG analyzed the data. BB, SH, PS-H, and JG contributed toward the writing of the manuscript. All authors reviewed the manuscript and agreed to its submission.
Funding
This work was supported by the facilities of the Australian Research Council Centre of Excellence in Plant Energy Biology (CE140100008) and funded by the Australian Research Council grants (LP100100438, DP130100087, and LP130100029), and ARC Future Fellowships (FT110100105) to BB. JG and SH acknowledge support from the Cooperative Research Centre for Honey Bee Products (Program 3.1). This research was also supported by an Australian Government Research Training Program (RTP) Scholarship and The University of Western Australia.
Conflict of Interest
The authors declare that the research was conducted in the absence of any commercial or financial relationships that could be construed as a potential conflict of interest.
Publisher’s Note
All claims expressed in this article are solely those of the authors and do not necessarily represent those of their affiliated organizations, or those of the publisher, the editors and the reviewers. Any product that may be evaluated in this article, or claim that may be made by its manufacturer, is not guaranteed or endorsed by the publisher.
Acknowledgments
We thank Tiffane Bates (The University of Western Australia) for help with the breeding of bees, Barbara Baer-Imhoof (University of California Riverside) for help in collecting the seminal fluid samples and bee inoculations, and the beekeepers of Western Australia for their continuous support of our research, in particular the Better Bees breeding program. We are also grateful to the reviewers CP and LS, whose feedback improved the manuscript.
Supplementary Material
The Supplementary Material for this article can be found online at: https://www.frontiersin.org/articles/10.3389/fevo.2021.755226/full#supplementary-material
References
Adams, J., Rothman, E. D., Kerr, W. E., and Paulino, Z. L. (1977). Estimation of the number of sex Alleles and Queen matings from diploid male frequencies in a population of Apis mellifera. Genetics 86, 583–596. doi: 10.1093/genetics/86.3.583
Al Naggar, Y., and Baer, B. (2019). Consequences of a short time exposure to a sublethal dose of flupyradifurone (Sivanto) pesticide early in life on survival and immunity in the honeybee (Apis mellifera). Sci. Rep. 9, 19753. doi: 10.1038/s41598-019-56224-1
Arakane, Y., and Muthukrishnan, S. (2010). Insect chitinase and chitinase-like proteins. Cell. Mol. Life Sci. 67, 201–216. doi: 10.1007/s00018-009-0161-9
Aronstein, K. A., Murray, K. D., and Saldivar, E. (2010). Transcriptional responses in honey bee larvae infected with chalkbrood fungus. BMC Genomics 11:391. doi: 10.1186/1471-2164-11-391
Aufauvre, J., Biron, D. G., Vidau, C., Fontbonne, R., Roudel, M., Diogon, M., et al. (2012). Parasite-insecticide interactions: a case study of Nosema ceranae and Fipronil synergy on honeybee. Sci. Rep. 2:326. doi: 10.1038/srep00326
Baer, B., and Schmid-Hempel, P. (1999). Experimental variation in polyandry affects parasite loads and fitness in a bumble-bee. Nature 397, 151–154. doi: 10.1038/16451
Baer, B., and Schmid-Hempel, P. (2000). The artificial insemination of bumblebee queens. Insectes Soc. 47, 183–187. doi: 10.1007/pl00001699
Baer, B., Heazlewood, J. L., Taylor, N. L., Eubel, H., and Millar, A. H. (2009). The seminal fluid proteome of the honeybee Apis mellifera. Proteomics 9, 2085–2097. doi: 10.1002/pmic.200800708
Bailey, L. (1955). The epidemiology and control of Nosema disease of the honey-bee. Ann. Appl. Biol. 43, 379–389. doi: 10.1111/j.1744-7348.1955.tb02488.x
Biesmeijer, J. C., Roberts, S. P., Reemer, M., Ohlemüller, R., Edwards, M., Peeters, T., et al. (2006). Parallel declines in pollinators and insect-pollinated plants in Britain and the Netherlands. Science 313, 351–354. doi: 10.1126/science.1127863
Bird, G., Wilson, A. E., Williams, G. R., and Hardy, N. B. (2021). Parasites and pesticides act antagonistically on honey bee health. J. Appl. Ecol. 58, 997–1005. doi: 10.1111/1365-2664.13811
Brodschneider, R., Gray, A., Adjlane, N., Ballis, A., Brusbardis, V., Charrière, J.-D., et al. (2018). Multi-country loss rates of honey bee colonies during winter 2016/2017 from the Coloss survey. J. Apic. Res. 57, 452–457. doi: 10.1080/00218839.2018.1460911
Brown, M. J. F., and Paxton, R. J. (2009). The conservation of bees: a global perspective. Apidologie 40, 410–416. doi: 10.1051/apido/2009019
Brown, M. J. F., and Schmid-Hempel, P. (2003). The evolution of female multiple mating in social Hymenoptera. Evolution 57, 2067–2081. doi: 10.1111/j.0014-3820.2003.tb00386.x
Carius, H. J., Little, T. J., and Ebert, D. (2001). Genetic variation in a host-parasite association: potential for coevolution and frequency-dependent selection. Evolution 55, 1136–1145. doi: 10.1111/j.0014-3820.2001.tb00633.x
Cooper, D., and Eleftherianos, I. (2017). Memory and specificity in the insect immune system: current perspectives and future challenges. Front. Immunol. 8:539. doi: 10.3389/fimmu.2017.00539
Cox-Foster, D. L., Conlan, S., Holmes, E. C., Palacios, G., Evans, J. D., Moran, N. A., et al. (2007). A metagenomic survey of microbes in honey bee colony collapse disorder. Science 318, 283–287. doi: 10.1126/science.1146498
Czekońska, K. (2007). Influence of carbon dioxide on Nosema apis infection of honeybees (Apis mellifera). J. Invertebr. Pathol. 95, 84–86. doi: 10.1016/j.jip.2007.02.001
Daimon, T., Hamada, K., Mita, K., Okano, K., Suzuki, M. G., Kobayashi, M., et al. (2003). A Bombyx mori gene, Bmchi-H, encodes a protein homologous to bacterial and Baculovirus Chitinases. Insect Biochem. Mol. Biol. 33, 749–759. doi: 10.1016/s0965-1748(03)00084-5
Fries, I. (1988). Infectivity and multiplication of Nosema apis Z. in the ventriculus of the honey bee. Apidologie 19, 319–328. doi: 10.1051/apido:19880310
Fries, I. (1989). Observations on the development and transmission of Nosema apis Z. in the ventriculus of the honeybee. J. Apic. Res. 28, 107–117. doi: 10.1080/00218839.1989.11100830
Fries, I. (1993). Nosema Apis—a parasite in the honey bee colony. Bee World 74, 5–19. doi: 10.1080/0005772x.1993.11099149
Fries, I., Chauzat, M.-P., Chen, Y.-P., Doublet, V., Genersch, E., Gisder, S., et al. (2013). Standard methods for Nosema research. J. Apic. Res. 52, 1–28. doi: 10.1007/978-3-319-23534-9_1
Genersch, E. (2010). Honey bee pathology: current threats to honey bees and beekeeping. Appl. Microbiol. Biotechnol. 87, 87–97. doi: 10.1007/s00253-010-2573-8
Genersch, E., Von Der Ohe, W., Kaatz, H., Schroeder, A., Otten, C., Büchler, R., et al. (2010). The German bee monitoring project: a long term study to understand periodically high winter losses of honey bee colonies. Apidologie 41, 332–352. doi: 10.1051/apido/2010014
Girard, C., and Jouanin, L. (1999). Molecular cloning of a gut-specific Chitinase cDNA from the beetle Phaedon cochleariae. Insect Biochem. Mol. Biol. 29, 549–556. doi: 10.1016/s0965-1748(99)00029-6
Goblirsch, M. (2018). Nosema ceranae disease of the honey bee (Apis mellifera). Apidologie 49, 131–150. doi: 10.1007/s13592-017-0535-1
Goulson, D., Nicholls, E., Botías, C., and Rotheray, E. L. (2015). Bee declines driven by combined stress from parasites, pesticides, and lack of flowers. Science 347:1255957. doi: 10.1126/science.1255957
Grassl, J., Holt, S., Cremen, N., Peso, M., Hahne, D., and Baer, B. (2018). Synergistic effects of pathogen and pesticide exposure on honey bee (Apis mellifera) survival and immunity. J. Invertebr. Pathol. 159, 78–86. doi: 10.1016/j.jip.2018.10.005
Grassl, J., Peng, Y., Baer-Imhoof, B., Welch, M., Millar, A. H., and Baer, B. (2016). Infections with the sexually transmitted pathogen Nosema apis trigger an immune response in the seminal fluid of honey bees (Apis mellifera). J. Proteome Res. 16, 319–334. doi: 10.1021/acs.jproteome.6b00051
Gray, A., Adjlane, N., Arab, A., Ballis, A., Brusbardis, V., Charrière, J.-D., et al. (2020). Honey bee colony winter loss rates for 35 countries participating in the coloss survey for winter 2018–2019, and the effects of a New Queen on the risk of colony winter loss. J. Apic. Res. 59, 744–751. doi: 10.1080/00218839.2020.1797272
Gray, A., Brodschneider, R., Adjlane, N., Ballis, A., Brusbardis, V., Charriere, J.-D., et al. (2019). Loss rates of honey bee colonies during winter 2017/18 in 36 Countries participating in the coloss survey, including effects of forage sources. J. Apic. Res. 58, 479–485. doi: 10.1080/00218839.2019.1615661
Hamid, R., Khan, M. A., Ahmad, M., Ahmad, M. M., Abdin, M. Z., Musarrat, J., et al. (2013). Chitinases: an update. J. Pharm. Bioallied Sci. 5, 21–29. doi: 10.4103/0975-7406.106559
Hamilton, W. D. (1987). “Kinship, recognition, disease, and intelligence: constraints of social evolution,” in Animal Societies: Theories and Facts, eds Y. Ito, J. L. Brown, and J. Kikkawa (Tokyo: Japan Scientific Societies Press).
Higes, M., Martín, R., and Meana, A. (2006). Nosema ceranae, a New microsporidian parasite in honeybees in Europe. J. Invertebr. Pathol. 92, 93–95. doi: 10.1016/j.jip.2006.02.005
Higes, M., Martín-Hernández, R., Botías, C., Bailón, E. G., González-Porto, A. V., Barrios, L., et al. (2008). How natural infection by Nosema ceranae causes honeybee colony collapse. Environ. Microbiol. 10, 2659–2669. doi: 10.1111/j.1462-2920.2008.01687.x
Higes, M., Martín-Hernández, R., Garrido-Bailón, E., González-Porto, A. V., García-Palencia, P., Meana, A., et al. (2009). Honeybee colony collapse due to Nosema ceranae in professional apiaries. Environ. Microbiol. Rep. 1, 110–113. doi: 10.1111/j.1758-2229.2009.00014.x
Hoffmann, J. A. (1995). Innate immunity of insects. Curr. Opin. Immunol. 7, 4–10. doi: 10.1016/0952-7915(95)80022-0
Hoffmann, J. A. (2003). The immune response of Drosophila. Nature 426, 33–38. doi: 10.14202/vetworld.2018.1043-1046
Hoffmann, J. A., Reichhart, J.-M., and Hetru, C. (1996). Innate immunity in higher insects. Curr. Opin. Immunol. 8, 8–13. doi: 10.1016/s0952-7915(96)80098-7
Houdelet, C., Sinpoo, C., Chantaphanwattana, T., Voisin, S. N., Bocquet, M., Chantawannakul, P., et al. (2021). Proteomics of anatomical sections of the gut of Nosema-infected western honeybee (Apis mellifera) reveals different early responses to Nosema Spp. Isolates J. Proteome Res. 20, 804–817. doi: 10.1021/acs.jproteome.0c00658
Hughes, W. O. H., and Boomsma, J. J. (2004). Genetic diversity and disease resistance in leaf-cutting ant societies. Evolution 58, 1251–1260. doi: 10.1554/03-546
Jacques, A., Laurent, M., Consortium, E., Ribière-Chabert, M., Saussac, M., Bougeard, S., et al. (2017). A Pan-European epidemiological study reveals honey bee colony survival depends on beekeeper education and disease control. PLoS One 12:e0172591. doi: 10.1371/journal.pone.0172591
Klein, A.-M., Vaissiere, B. E., Cane, J. H., Steffan-Dewenter, I., Cunningham, S. A., Kremen, C., et al. (2007). Importance of pollinators in changing landscapes for world crops. Proc. Royal Soc. Lond. B 274, 303–313. doi: 10.1098/rspb.2006.3721
Kurtz, J. (2005). Specific memory within innate immune systems. Trends Immunol. 26, 186–192. doi: 10.1016/j.it.2005.02.001
Lambrechts, L., Halbert, J., Durand, P., Gouagna, L. C., and Koella, J. C. (2005). Host genotype by parasite genotype interactions underlying the resistance of Anopheline mosquitoes to Plasmodium falciparum. Malar. J. 4:3. doi: 10.1186/1475-2875-4-3
Le Conte, Y., and Navajas, M. (2008). Climate change: impact on honey bee populations and diseases. Rev. Sci. Techn. 27, 499–510.
Lemaitre, B., and Hoffmann, J. (2007). The host defense of Drosophila melanogaster. Annu. Rev. Immunol. 25, 697–743.
Liersch, S., and Schmid-Hempel, P. (1998). Genetic variation within social insect colonies reduces parasite load. Proc. R. Soc. Lond. B. Biol. Sci. 265, 221–225.
Mackensen, O., and Roberts, W. C. (1948). A Manual for the Artificial Insemination of Queen Bees. Washington, DC: US Department of Agriculture, Agricultural Research Administration, Bureau of Entomology and Plant Quarantine.
Mallon, E. B., Loosli, R., and Schmid-Hempel, P. (2003). Specific versus nonspecific immune defense in the Bumblebee, Bombus terrestris L. Evolution 57, 1444–1447. doi: 10.1111/j.0014-3820.2003.tb00351.x
Martín-Hernández, R., Botías, C., Barrios, L., Martínez-Salvador, A., Meana, A., Mayack, C., et al. (2011). Comparison of the energetic stress associated with experimental Nosema ceranae and Nosema apis infection of honeybees (Apis mellifera). Parasitol. Res. 109, 605–612. doi: 10.1007/s00436-011-2292-9
Memmott, J., Craze, P. G., Waser, N. M., and Price, M. V. (2007). Global warming and the disruption of plant–pollinator interactions. Ecol. Lett. 10, 710–717. doi: 10.1111/j.1461-0248.2007.01061.x
Neumann, P., and Carreck, N. L. (2010). Honey bee colony losses. J. Apic. Res. 49, 1–16. doi: 10.3896/ibra.1.49.1.01
Neumann, P., and Moritz, R. F. A. (2000). Testing genetic variance hypotheses for the evolution of polyandry in the honeybee (Apis mellifera L.). Insectes Soc. 47, 271–279. doi: 10.1007/pl00001714
Ollerton, J., Winfree, R., and Tarrant, S. (2011). How many flowering plants are pollinated by animals? Oikos 120, 321–326. doi: 10.1111/j.1600-0706.2010.18644.x
Peng, Y., Baer-Imhoof, B., Millar, A. H., and Baer, B. (2015). Consequences of Nosema apis infection for male honey bees and their fertility. Sci. Rep. 5:10565. doi: 10.1038/srep10565
Peng, Y., Grassl, J., Millar, A. H., and Baer, B. (2016). Seminal fluid of honeybees contains multiple mechanisms to combat infections of the sexually transmitted pathogen Nosema apis. Proc. Royal Soc. Lond. B 283, 20151785. doi: 10.1098/rspb.2015.1785
Peng, Y., Lee-Pullen, T. F., Heel, K., Millar, A. H., and Baer, B. (2014). Quantifying spore viability of the honey bee pathogen Nosema apis using flow cytometry. Cytometry A 85, 454–462. doi: 10.1002/cyto.a.22428
Pesch, Y. Y., Riedel, D., and Behr, M. (2017). Drosophila Chitinase 2 is expressed in chitin producing organs for cuticle formation. Arthropod Struct. Dev. 46, 4–12. doi: 10.1016/j.asd.2016.11.002
Potts, S. G., Biesmeijer, J. C., Kremen, C., Neumann, P., Schweiger, O., and Kunin, W. E. (2010). Global pollinator declines: trends, impacts and drivers. Trends Ecol. Evol. 25, 345–353. doi: 10.1016/j.tree.2010.01.007
R Core Team. (2017). R: A Language and Environment for Statistical Computing. 3.4, 1 Edn. Vienna: R Foundation for Statistical Computing.
Ratnieks, F. L. W., and Carreck, N. L. (2010). Clarity on honey bee collapse? Science 327, 152–153. doi: 10.1126/science.1185563
Roberts, K. E., Evison, S. E. F., Baer, B., and Hughes, W. O. H. (2015). The cost of promiscuity: sexual transmission of Nosema microsporidian parasites in polyandrous honey bees. Sci. Rep. 5:10982. doi: 10.1038/srep10982
Rosenkranz, P., Aumeier, P., and Ziegelmann, B. (2010). Biology and control of varroa destructor. J. Invertebr. Pathol. 103, S96–S119.
Sadd, B. M., and Barribeau, S. M. (2013). Heterogeneity in infection outcome: lessons from a bumblebee-trypanosome system. Parasite Immunol. 35, 339–349. doi: 10.1111/pim.12043
Schlüns, H., Moritz, R. F. A., Lattorff, H. M. G., and Koeniger, G. (2005). Paternity Skew in seven species of honeybees (Hymenoptera: Apidae: Apis). Apidologie 36, 201–209. doi: 10.1051/apido:2005006
Schmid-Hempel, P. (2001). On the evolutionary ecology of host-parasite interactions: addressing the question with regard to bumblebees and their parasites. Sci. Nat. 88, 147–158. doi: 10.1007/s001140100222
Seeley, T. D., and Tarpy, D. R. (2007). Queen promiscuity lowers disease within honeybee colonies. Proc. Royal Soc. Lond. B 274, 67–72. doi: 10.1098/rspb.2006.3702
Shen, Z., and Jacobs-Lorena, M. (1997). Characterization of a novel gut-specific chitinase gene from the human malaria vector Anopheles gambiae. J. Biol. Chem. 272, 28895–28900. doi: 10.1074/jbc.272.46.28895
Sherman, P. W., Seeley, T. D., and Reeve, H. K. (1988). Parasites, pathogens, and polyandry in social hymenoptera. Am. Nat. 131, 602–610. doi: 10.1086/284809
Shykoff, J. A., and Schmid-Hempel, P. (1991). Genetic relatedness and Eusociality: parasite-mediated selection on the genetic composition of groups. Behav. Ecol. Sociobiol. 28, 371–376. doi: 10.1007/bf00164387
Siviter, H., Bailes, E. J., Martin, C. D., Oliver, T. R., Koricheva, J., Leadbeater, E., et al. (2021). Agrochemicals interact synergistically to increase bee mortality. Nature 596, 389–392. doi: 10.1038/s41586-021-03787-7
Tarpy, D. R. (2003). Genetic diversity within honeybee colonies prevents severe infections and promotes colony growth. Proc. R. Soc. Lond. B 270, 99–103. doi: 10.1098/rspb.2002.2199
Tarpy, D. R., and Seeley, T. D. (2006). Lower disease infections in honeybee (Apis mellifera) colonies headed by polyandrous vs monandrous queens. Sci. Nat. 93, 195–199. doi: 10.1007/s00114-006-0091-4
Thimmegowda, G. G., Mullen, S., Sottilare, K., Sharma, A., Mohanta, S. S., Brockmann, A., et al. (2020). A field-based quantitative analysis of sublethal effects of air pollution on pollinators. Proc. Natl. Acad. Sci. U.S.A. 117, 20653–20661. doi: 10.1073/pnas.2009074117
van der Sluijs, J. P., Simon-Delso, N., Goulson, D., Maxim, L., Bonmatin, J.-M., and Belzunces, L. P. (2013). Neonicotinoids, bee disorders and the sustainability of pollinator services. Curr. Opin. Environ. Sustain. 5, 293–305. doi: 10.1016/j.cosust.2013.05.007
van der Zee, R., Pisa, L., Andonov, S., Brodschneider, R., Charrière, J.-D., Chlebo, R., et al. (2012). Managed honey bee colony losses in Canada, China, Europe, Israel and Turkey, for the Winters of 2008–9 and 2009–10. J. Apic. Res. 51, 100–114.
vanEngelsdorp, D., Hayes, J., Underwood, R. M., and Pettis, J. S. (2010). A survey of honey bee colony losses in the United States, fall 2008 to spring 2009. J. Apic. Res. 49, 7–14. doi: 10.3896/ibra.1.49.1.03
Vega, K., and Kalkum, M. (2012). Chitin, chitinase responses, and invasive fungal infections. Int. J. Microbiol. 2012, 920459.
Webster, T. C., Thacker, E. M., Pomper, K., Lowe, J., and Hunt, G. (2008). Nosema apis infection in honey bee (Apis mellifera) Queens. J. Apic. Res. 47, 53–57. doi: 10.3896/ibra.1.47.1.08
Williams, G. R., Alaux, C., Costa, C., Csaki, T., Doublet, V., Eisenhardt, D., et al. (2013). Standard methods for maintaining adult Apis mellifera in cages under in vitro laboratory conditions. J. Apic. Res. 52, 1–36.
Woodcock, B. A., Bullock, J. M., Shore, R. F., Heard, M. S., Pereira, M. G., Redhead, J., et al. (2017). Country-specific effects of neonicotinoid pesticides on honey bees and wild bees. Science 356, 1393–1395. doi: 10.1126/science.aaa1190
Keywords: Nosema apis, virulence, infectivity, host parasite interactions, β-N-acetylglucosaminidase, chitinase activity
Citation: Holt S, Cremen N, Grassl J, Schmid-Hempel P and Baer B (2021) Genetic Variation in Antimicrobial Activity of Honey Bee (Apis mellifera) Seminal Fluid. Front. Ecol. Evol. 9:755226. doi: 10.3389/fevo.2021.755226
Received: 08 August 2021; Accepted: 18 November 2021;
Published: 09 December 2021.
Edited by:
Alison McAfee, North Carolina State University, United StatesReviewed by:
Lars Straub, University of Bern, SwitzerlandChristian W. W. Pirk, University of Pretoria, South Africa
Copyright © 2021 Holt, Cremen, Grassl, Schmid-Hempel and Baer. This is an open-access article distributed under the terms of the Creative Commons Attribution License (CC BY). The use, distribution or reproduction in other forums is permitted, provided the original author(s) and the copyright owner(s) are credited and that the original publication in this journal is cited, in accordance with accepted academic practice. No use, distribution or reproduction is permitted which does not comply with these terms.
*Correspondence: Shannon Holt, c2hhbm5vbi5ob2x0QHJlc2VhcmNoLnV3YS5lZHUuYXU=; Boris Baer, Ym9yaXMuYmFlckB1Y3IuZWR1