- 1Department of Ecology and Evolutionary Biology, Neuroscience Program, University of California, Los Angeles, Los Angeles, CA, United States
- 2Moreton Bay Research Station, Centre for Marine Science, School of Biological Sciences, University of Queensland, Brisbane, QLD, Australia
Thousands of armed predatory species, distributed widely across the metazoan tree-of-life, consume only hard-shell or exoskeleton-bearing organisms (called “durophagy”). Prey armor clearly has evolved in response to selection by predators, but there is little evidence of the contrary, counter-adaptation by predators. Evolved consumer responses to prey, in general, might be more readily expressed in ways other than morphological traits, including via sensory cues. Here, we explored the chemosensory basis for durophagy in a model predator-prey system, and identified intimate associations between durophagous predators and their shelled prey. Barnacles (Balanus glandula and Semibalanus cariosus) bear hard shells and secrete, respectively, a 199 or 201 kDa glycoprotein ortholog (named “MULTIFUNCin”), with expression limited to the body armor (epidermis, cuticle, and live shell). To test for effects of MULTIFUNCin on predators, we constructed faux prey to mimic meaningful physical and chemical characteristics of live barnacles. In separate experiments, each consumer species was presented MULTIFUNCin, purified from either B. glandula or S. cariosus, at a typical armor concentration. All six predatory species (sea star, Pisaster ochraceus; whelks, Acanthinucella spirata, Nucella emarginata, N. ostrina, N. canaliculata, and N. lamellosa) attacked and ate MULTIFUNCin-infused faux prey significantly more than controls. Akin to barnacles, secretion of glycoprotein-rich extracellular matrices is common among armored prey species—from marine sponges to terrestrial vertebrates. Our results, therefore, suggest that chemosensory exploitation of glycoproteins could be widespread, with notable consequences for life on land and in the sea.
Introduction
A myriad of metazoan species have evolved armor in morphological defenses. The scales of fishes and reptiles, cuticles and carapaces of insects and crustaceans, as well as skeletons and shells of corals, mollusks, brachiopods, echinoderms, and sponges, all protect against the “slings and arrows” of a dangerous world (Kruppert et al., 2020). There is an intuitive appeal to viewing struggles among species as arms races between natural enemies (Brodie and Brodie, 1999). The evolution of armed predators may have led to the evolution of mineralized armor in prey since near the beginning of the Cambrian (Vermeij, 1989; Marshall, 2006; Wood and Zhuravlev, 2012).
The arms race analogy has been applied to a wide array of natural enemies, even though the degree of reciprocity and details of selection vary among antagonistic encounters. Predator-prey interactions, especially, may be characterized by asymmetrical selection that precludes arms races (selection on prey is thought to be stronger than on predators) (Dawkins and Krebs, 1979; Brodie and Brodie, 1999; Whitenack and Herbert, 2015). Elaborated jaws, and other weapons, increase the ability of consumers to capture prey. Such innovations also evolve in response to selection pressures imposed by higher-order predators and by competitors for food, space, and mates (Vermeij, 1994, 2013; Bicknell and Paterson, 2018; Aristide and Morlon, 2019). Enhanced capacity to capture prey can be an unintended consequence of escalation in a particular anti-predator, or anti-competitor, morphological or behavioral trait.
Prey armor clearly has evolved in response to selection from predators, but there is little evidence of the contrary, counter-adaptation by predators (Harvell, 1984; Vermeij, 1994, 2013; Kosloski and Allmon, 2015; Klompmaker et al., 2017). Evolved predator responses to prey might be more readily expressed in ways other than morphological traits, including via sensory cues. Essentially every living organism has a chemical sense to exploit valuable resources and ward off danger. Prey flee, hide, or elaborate armor in response to infochemicals emitted by durophagous consumers (Zimmer et al., 2006; Poulin et al., 2018; Weiss et al., 2018). Morphologically defended prey also release alarm substances when attacked. These compounds cue escape or defense in other, threatened individuals (Ono et al., 2003; Jacobson and Stabell, 2004; Smee and Weissburg, 2006). Surprisingly, little is known about the reciprocal interaction: do durophagous predators exploit molecules associated with armored prey? If so, are these cues species-, or taxon-specific, and are they expressed uniquely in prey body armor?
Hard-shelled barnacles are superior competitors for space within the higher-reaches of wave-swept shores. As foundation species, they provide resources to community members and act as determinants of biodiversity (Trussel et al., 2003; Maggi et al., 2015). Durophagy by sea stars (Pisaster ochraceus) and whelks (Acanthinucella spirata, Nucella emarginata, N. ostrina, N. canaliculata, and N. lamellosa) shapes barnacle (Balanus glandula and Semibalanus cariosus) populations, thereby impacting intertidal communities along the Pacific Ocean coast of North America (from the Aleutian Islands to central Baja, Table 1; Connell, 1961, 1970; Murdoch, 1969; Dayton, 1971; Navarrete, 1996). Sea stars explore the substrate with contact chemoreceptors located on tube feet (McClintock et al., 1994). In contrast, whelks have contact chemoreceptors distributed diffusely across the foot and concentrated on a retractable proboscis that probes substrate surfaces (Crisp, 1971; Hodgson and Brown, 1985). Whereas sea stars feed by first protruding their lower stomach into a barnacle, whelks penetrate the shell using a drill-like radula.
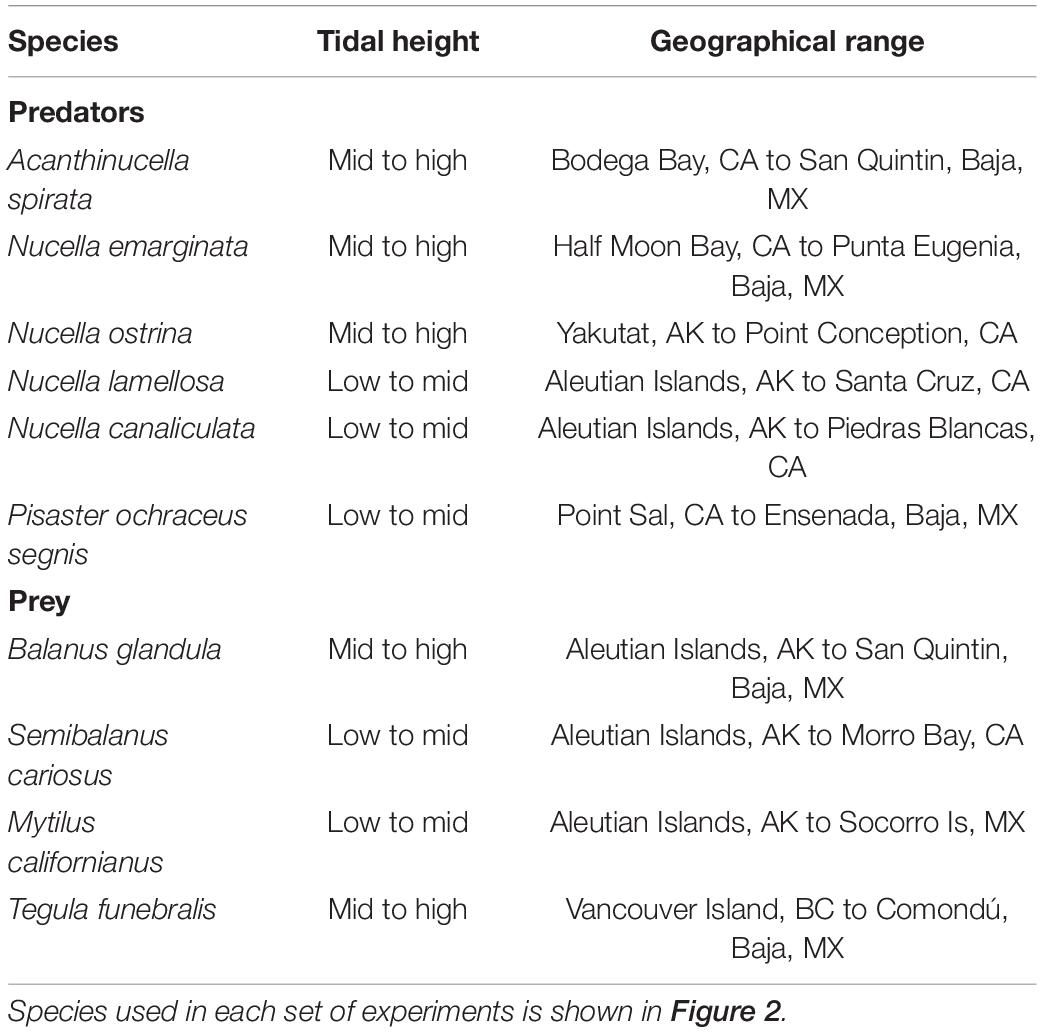
Table 1. Tidal heights and geographical ranges of study species along the North America Pacific Ocean coast.
Each barnacle species (B. glandula and S. cariosus) secretes, respectively, a 199 or 201 kDa glycoprotein ortholog (named “MULTIFUNCin,” in recognition of its multifunctional properties), with expression limited to the body armor (epidermis, cuticle, and live shell materials) (Ferrier et al., 2016a; Figure 1). To test for effects of MULTIFUNCin on consumers, we constructed faux prey to mimic meaningful physical and chemical properties of live barnacles. In separate experiments, MULTIFUNCin, purified from either B. glandula or S. cariosus, was presented alone at a typical armor concentration. All six predatory species attacked and ate MULTIFUNCin-infused faux prey significantly more than controls. These results are consistent with a chemosensory basis for durophagy, and suggest that mechanisms for glycoprotein recognition have converged across phylogenetically diverse taxa (sea stars and whelks) to promote the exploitation of a valuable, shared prey resource.
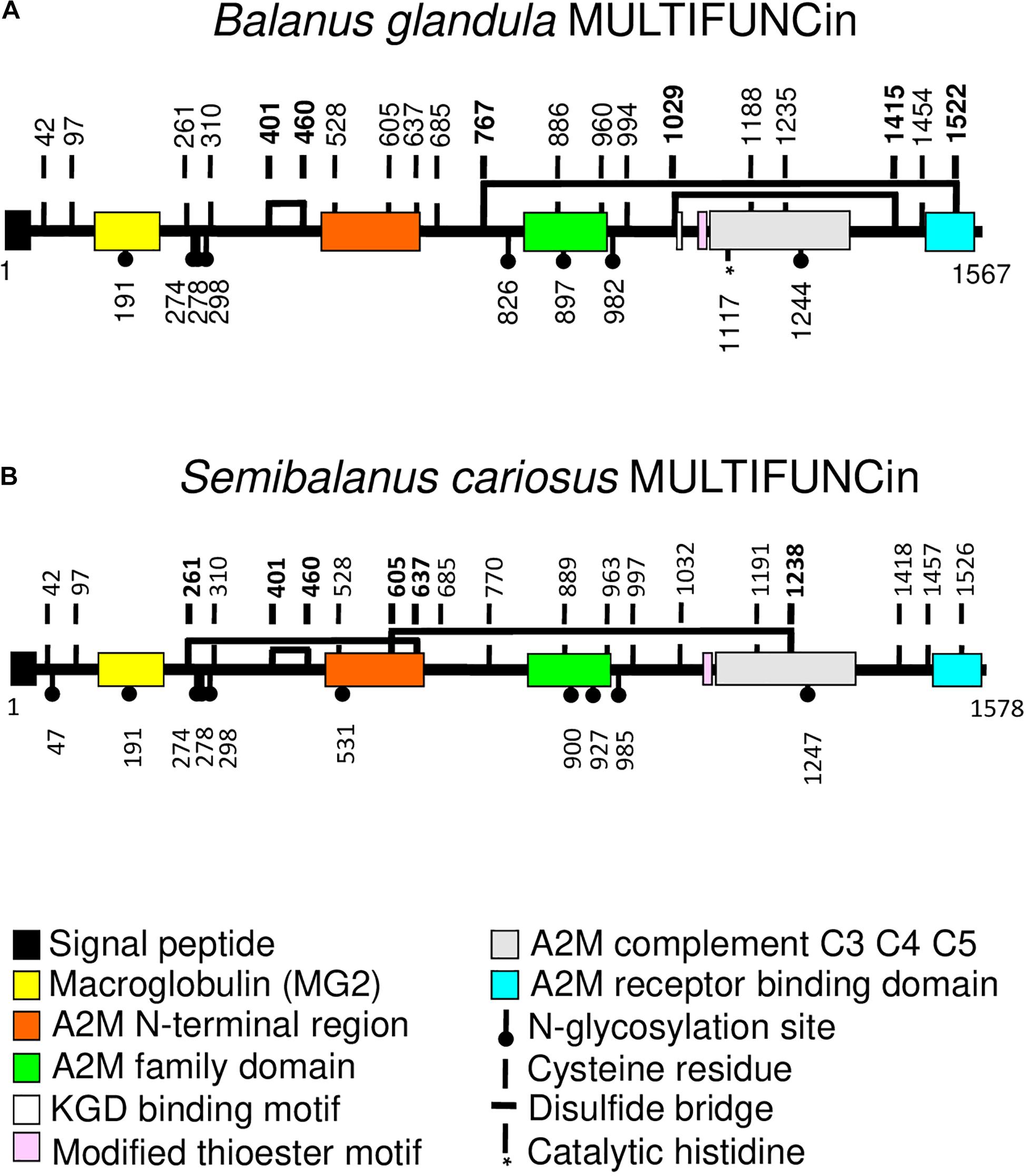
Figure 1. Structural architectures and domain organizations of (A) Balanus glandula MULTIFUNCin and (B) Semibalanus cariosus MULTIFUNCin. These 199 and 201 kDa glycoprotein orthologs, respectively, are expressed uniquely in the armor (epidermis, cuticle, and new shell material) of each species (Ferrier et al., 2016a). The two compounds and their encoding genes share 82% amino acid and 83% nucleotide sequence homologies. Several, shared, conserved domains place both substances within the α2-macroglobulin subgroup of the thioester-containing protein family. A signal peptide, modified thioester motif, and other notable domains and regions are highlighted by color-specific rectangles. Predicted amino acid positions of N-glycosylation sites are provided (closed circles), as well as the site of a putative catalytic histidine (*) in B. glandula MULTIFUNCin. Vertical lines with bold numbers denote cysteine positions. The estimated locations of disulfide bridges are marked with horizontal lines connecting bold cysteine residues. A2M, α2-macroglobulin; KGD, lysine-glycine-aspartic acid; C3, C4, and C5, complement component factor proteins 3, 4, and 5. Each MULTIFUNCin molecule consists of 1,567 or 1,578 amino acid residues (1 = amino terminus; 1,567 or 1,578 = carboxy terminus).
Materials and Methods
Isolation and Purification of MULTIFUNCin Proteins
Overview
Chemical fractionation and isolation of MULTIFUNCin followed established procedures (Ferrier et al., 2016a,b). Elsewhere, translations of complete amino acid and nucleotide sequences are described for each molecule and its encoding gene (GenBank accession numbers: KC152471 for B. glandula MULTIFUNCin, and KC152472 for S. cariosus MULTIFUNCin) (Figure 1; Ferrier et al., 2016a). For each barnacle species (Balanus glandula and Semibalanus cariosus), MULTIFUNCin purification steps comprised, in succession, (1) extraction of animal shells and tissues with trishydroxymethylaminomethane-HCl (hereafter, tris-HCl)-buffered saline, (2) ammonium sulfate ([NH4]2SO4) protein precipitation, (3) size-exclusion chromatography, (4) lentil-lectin (LCA) affinity chromatography, and finally, (5) SDS-PAGE protein separation with electroelution of purified compounds. Purity and concentration of MULTIFUNCin were confirmed using matrix-assisted laser desorption ionization time-of-flight mass spectrometry (Voyager DE-STR, Applied Biosystems, Foster City, CA) (Ferrier et al., 2016a).
Tissue Extraction and Protein Precipitation
Live barnacles (0.4–1.0 cm shell height) were collected at field sites, flash frozen in liquid nitrogen, and either transported directly (Balanus glandula from Malibu, CA), or shipped on dry ice via overnight courier (B. glandula from Cattle Point, WA, and Semibalanus cariosus from Bodega, CA), to our UCLA laboratory. Individuals from each collection site were separated, crushed, and homogenized at high speed (using a Waring blender) in a 1:1.5 (v/v) of 50 mmol L–1 tris-HCl (pH 7.5) buffer. Resulting homogenates were stirred on ice for 120 min, rough filtered through gauze (nominal 80 μm pore diameter), and centrifuged at 40,000 × g (for 30 min at 4°C). Supernatants were filtered to 0.45-μm, and eluates served as “crude extracts.”
Initial purification steps involved separating eluate components on the basis of solubility through stepwise ammonium sulfate precipitation. Bioactive proteins were isolated from other, organic, molecules starting with a 35%, increasing to 70%, and ending with 100% (NH4)2SO4 saturation. Ammonium sulfate was added to each barnacle crude extract and stirred at 4°C for 10 min, then centrifuged at 40,000 × g (4°C for 15 min), before the supernatant was removed. MULTIFUNCin was retained as a component in precipitate of the 35–70% saturation solution (Ferrier et al., 2016a). Each pellet was resuspended in 2 mL of 50 mmol L–1 tris-HCl (pH 7.5) and dialyzed [Slide-A-LyzerTM dialysis cassettes, 5 kDa molecular weight cutoff (MWCO, hereafter); Thermo Fisher Scientific, Waltham, MA] against and diluted with 0.45 μm-filtered seawater, as needed.
Size-Exclusion and Lentil-Lectin Affinity Chromatography of Barnacle Bioactive Precipitate
Resuspended precipitates were fractionated using size-exclusion (SEC) and lentil-lectin (LCA) affinity chromatography. A HiPrep 16/60 high resolution S-200 sephacryl gel size-exclusion column (SEC, GE Healthcare, Waukesha, WI) was eluted at 0.8 mL min–1 with 50 mmol L–1 Tris-HCl (pH 7.5) buffer on a BiologicTM fast performance liquid chromatography (FPLC) system (Bio-Rad, Hercules, CA). MULTIFUNCin was retained in the 150–530 kDa SEC peak (Ferrier et al., 2016a).
Each peak was collected, and divided into two samples based on the presence/absence of glycan groups. A 16/40 column (GE Healthcare) was packed with LCA sepharose 4B beads (GE Healthcare), and equilibrated with a FPLC system running buffer [0.5 mol L–1 NaCl, 25 mmol L–1 tris-HCl (pH 7.5)] at 0.8 mL min–1. Concentrated (1 mg mL–1) 150–530 kDa peak material was washed through the LCA beads with an additional 150 mL of equilibrated buffer. MULTIFUNCin, bound to the LCA beads following the wash, was removed with 150 mL of elution buffer [0.2 mol L–1 methyl α,D-mannopyranoside, 0.5 mol L–1 NaCl, 25 mmol L–1 tris-HCl (at pH 7.5)] increasing linearly from 0 to 100% over 30 s at the same flow rate. It was then concentrated using ultrafiltration (MWCO = 10 kDa).
SDS-PAGE Protein Separation and Electroelution of Purified MULTIFUNCin
Final purification involved protein separation and isolation through preparative SDS-PAGE. Each sample (1 mL), containing MULTIFUNCin, was conditioned with 5X Laemmli buffer and loaded onto a 7.5% acrylamide (acrylamide:bis = 29:1) slab gel (16 × 22 cm). It was run on ice at 180 V, until clear separation of molecular markers. All protein bands were visualized with imidazole-zinc reverse staining. From each gel, the MULTIFUNCin band was excised with a sterile scalpel, de-stained in Laemmli buffer, and recovered from the acrylamide via electroelution. Each gel fragment, containing MULTIFUNCin, was put into a Spectra/Por regenerated cellulose dialysis tubing (1 kDa MWCO, 8 mL volume; Spectrum Medical Corp., Long Beach, CA), sealed, and placed between the electrodes of a western blotting rig. Both tubing and rig were filled with Laemmli buffer, stirred, and cooled on ice. An electric current (100 V) was applied for 1 h. Isolated MULTIFUNCin migrated out of each fragment and into the buffer. Following electroelution, selected fragments were removed and stained with Coomassie G-250 dye to check protein recovery (which averaged 65%). The buffers were exchanged, through dialysis, to 50 mmol L–1 tris-HCl (pH 7.5). Purified MULTIFUNCin was concentrated 10-fold through ultrafiltration (MWCO = 10 kDa), dialyzed (Slide-A-LyzerTM dialysis cassettes, 5,000 Da MWCO) again, and diluted with 0.45 μm-filtered seawater, as required for bioassay. When not used immediately in bioassay, MULTIFUNCin was stored at −80°C in tris-HCl buffer.
Bioassays of MULTIFUNCin as a Contact Cue to Predation
Overview
Experiments tested for MULTIFUNCin effects on durophagy. Six, armed, consumer species were chosen for bioassays, based on their important roles in structuring rocky-intertidal communities (Connell, 1961, 1970; Paine, 1966, 1974; Murdoch, 1969; Navarrete, 1996). Three of these species are more abundant in northern latitudes (Nucella ostrina, N. canaliculata, and N. lamellosa), whereas the other three prevailed in southern latitudes (Acanthinucella spirata, N. emarginata, and Pisaster ochraceus segnis) (Table 1). Each selected consumer species naturally preys on dense patches of barnacles (Balanus glandula, Semibalanus cariosus), mussels (Mytilus californianus), turban snails (Tegula funebralis), and, less often, other invertebrate taxa (Feder, 1959; Paine, 1966; Murdoch, 1969; Connell, 1970; Dayton, 1971; Navarrete, 1996).
Faux Prey
Faux prey were fabricated for use in laboratory experiments. All soft tissues, along with cuticles, were removed with sterile instruments from barnacles (Balanus glandula and Semibalanus cariosus, 0.4–0.6 cm shell heights for experiments on whelks; 0.8–1.0 cm shell heights for sea stars). The empty shells were combusted for 12 h at 150°C, bathed for 15 min in a stirred, 5% (v/v) HCl solution (in tris-HCl buffer), and scrubbed thoroughly with Nanopure-grade deionized water to eliminate any residual organics. Cleaned, empty, barnacle shells served as physical replicas (“faux”) of the live animals.
Prey chemistry was simulated in flavored gels. Sodium carboxymethylcellulose (11.7% w/v) was mixed with 0.45-μm filtered seawater and purified MULTIFUNCin (from B. glandula or S. cariosus), then cured for 10 min at 4°C. Gels also were made using crude extracts of mussels or turban snails (see section “Materials and Methods” in “Tissue extraction” for barnacles), bovine serum albumin (BSA, 4 × 10–7 molar), or 0.45-μm filtered seawater alone. These alternative extracts and BSA served as organic enrichment controls in bioassays on chemical prey preferences. Once prepared, each gel had the consistency of prey flesh, and a 0.3–0.6 mL aliquot of gel was injected into a cleaned, empty, barnacle shell for immediate use in experiments. Concentrations of MULTIFUNCin (75 μg mL–1, about 4 × 10–7 molar) were the same across all prepared gels and live barnacle shell materials. Similarly, molar concentrations of BSA and MULTIFUNCin were the same across all prepared gels. Total protein levels in mussel and turban snail crude extracts were established using the Bradford method with bovine serum albumin as a standard (Bradford, 1976). Relative to MULTIFUNCin gels, total protein concentrations (w/v) were approximately 50 times higher in mussel and turban snail crude extract gels.
Animal Maintenance and Holding Procedures
Bioassays were performed over 2 years during June–September, when sea stars and whelks fed most actively in native habitats. Predators were collected from locations in Northern California (Horseshoe Cove and Mussel Point, Bodega Bay), Southern California (Broad Beach, Malibu), and Washington (Cattle Point, San Juan Island). These consumers were collected and either transported directly (Acanthinucella spirata, Nucella emarginata, and Pisaster ochraceus from Malibu, CA), or shipped on ice via overnight courier (N. lamellosa from Cattle Point, and N. ostrina and N. canaliculata from Bodega) to our laboratory. Consumers were fed an ad libitum diet of barnacles, mussels, and turban snails for 1–2 weeks, and then fasted for 3–5 days before testing.
The experimental set-up consisted of a 28,000-L seawater reservoir (oceanic quality, 33 psu salinity) with particle filtration (5-μm cut-off), UV sterilization (as needed), and computer-controlled water/air temperature (±1°C off set-points) and light cycle (L:D 14:10, light on at 0630 h). Animals from Malibu, Bodega, and Cattle Point were held and tested, respectively, at water temperatures of 19–21, 13–15, or 9–11°C. During animal holding periods and experiments, lighting (full spectrum, General Electric Daylight Ultra) was maintained at a level (75 μmol m–2 s–1) simulating field habitats during late afternoon. Each species was held separately at a density of 12–15 m–2 (whelks) or 3–4 m–2 (sea stars) with continuously recirculating seawater (45 L min–1), replenished twice per week.
General Experimental Procedures
All bioassays were performed in Plexiglas arenas (see section “Results” below for more details). These tanks were 60 cm (length) × 60 cm (width) × 15 cm (depth) for sea stars, or 10 cm × 10 cm × 5 cm for whelks. A constant supply of single-pass, 5-μm filtered seawater (1 L min–1) was delivered to every arena, held at ambient ocean temperature and salinity (see “Animal maintenance” above). Each tank was washed thoroughly with 1% (v/v) HCl in Nanopure water after every trial, and then rinsed with Nanopure water and seawater to eliminate any residual organics. All trials were performed between 10:00 and 18:00 h. An individual animal was tested once only in a given experiment.
Chemical Prey Preference Tests
For consistency and comparison of results to previous studies (Ferrier et al., 2016a,b; Zimmer et al., 2016a), we performed each experiment as a preference test (Figure 2). The suite of experiments, conducted here, provided the most meaningful information in the fewest number of trials. Three sets of experiments were performed. In Set 1, we compared the consummatory responses of five whelk species. A trial began when a single whelk (1.5–3.0 cm shell length) was placed at the center of an arena and presented with 12 faux prey. The 12 faux prey were divided into three groups of four that encircled the whelk. Each group contained one MULTIFUNCin (prepared using Balanus glandula from Malibu), one mussel crude extract, one turban snail crude extract, and one filtered seawater treatment. The exact position of each prey type within a given group was selected using a random numbers table. Each trial lasted until the predator selected, attacked and consumed a single faux prey, terminating after 1 h regardless.
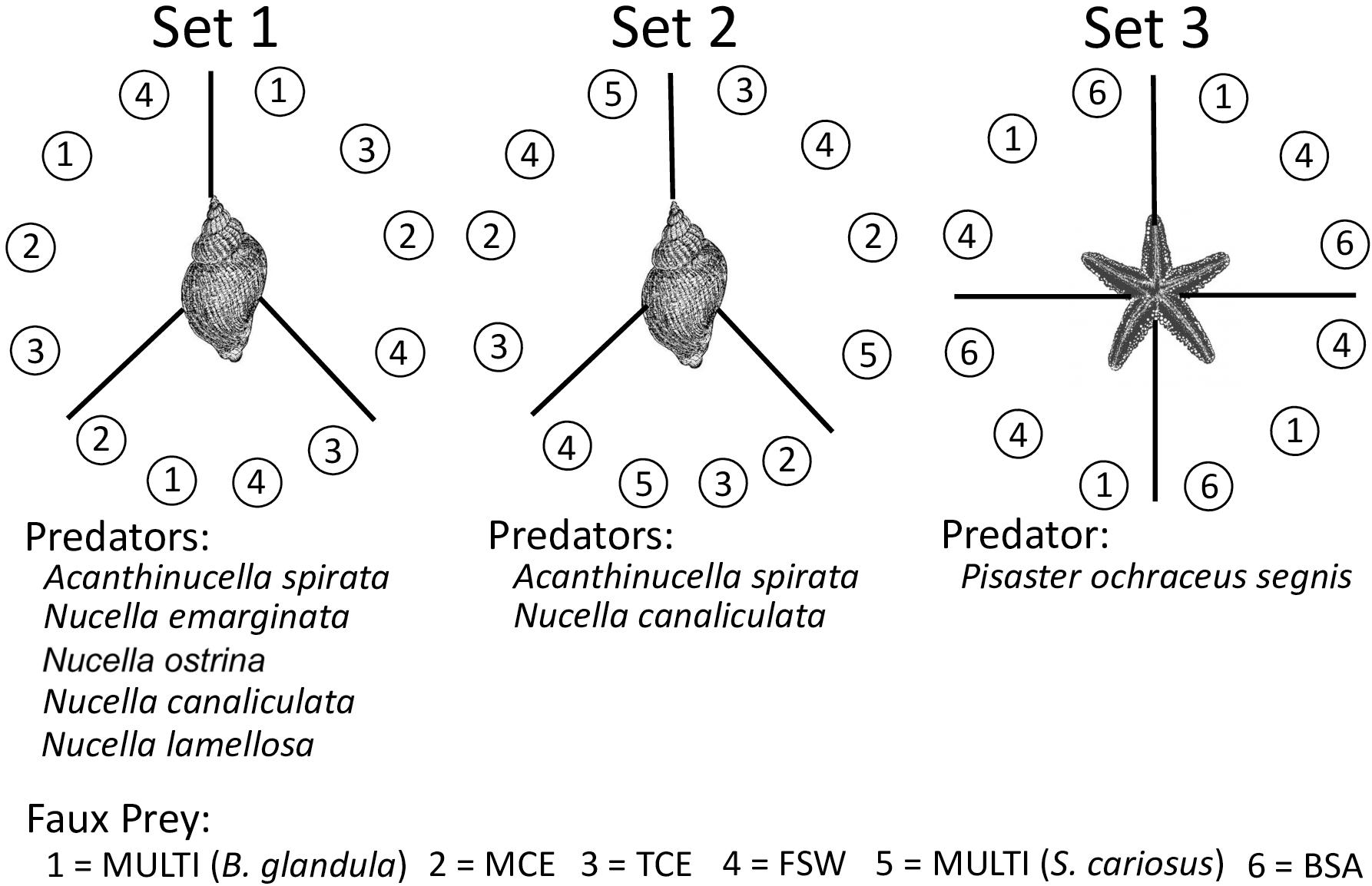
Figure 2. A schematic depicting Set 1, Set 2, and Set 3 experiments. Images are not drawn to scale. Each circle, with a number inside, represents a single faux prey that contains gel filled with (1) MULTIFUNCin from Balanus glandula (MULTI), (2) mussel crude extract (MCE), (3) turban snail crude extract (TCE), (4) filtered seawater (FSW), (5) MULTIFUNCin from Semibalanus cariosus (MULTI), or (6) bovine serum albumin (BSA). Each trial was stopped after a whelk or sea star consumed a single faux prey, or after 1 h without feeding. Set 1 experiments were replicated for five whelk species (Acanthinucella spirata, Nucella emarginata, N. lamellosa, N. ostrina, and N. canaliculata); Set 2 experiments were replicated for two whelk species (A. spirata and N. canaliculata); a single Set 3 experiment was performed for the sea star, Pisaster ochraceus. One predator was placed per trial and never reused. For each trial in every experiment, 12 faux prey were positioned equidistantly so as to surround the centrally positioned predator. In Sets 1 and 2, the 12 faux prey were divided into three groups of four. Each group contained one MULTI, one MCE, one TCE, and one FSW. The exact position of each prey type within a given group was selected using a random numbers table, and the random order changed from trial-to-trial. Similar methods were applied in the Set 3 experiment, except here, the 12 faux prey were divided into four groups of three: MULTI, FSW, and BSA. When given a choice, sea stars ate only mussel impregnated gels and not MULTIFUNCin impregnated gels in preliminary trials. A different organic enrichment control (BSA) therefore was needed. We used bovine serum albumin to be consistent with our previous studies (Zimmer et al., 2016b, 2017).
Set 2 experiments were performed identically to set 1 with two notable exceptions. Here, (1) trials were limited to two whelk species (Acanthinucella spirata and Nucella canaliculata), and (2) MULTIFUNCin was purified from Semibalanus cariosus. These tests were truncated because of the considerable time and effort needed in purifying large amounts of MULTIFUNCin for meaningful bioassay. Consummatory responses in Set 1 tests were nearly identical across all five whelk species (see section “Results,” below), rendering the re-testing of each whelk species redundant.
Set 3 consisted of a single experiment. In initial trials (n = 5), sea stars preyed only on mussel-crude-extract gels, and not on MULTIFUNCin-gels (Binomial test: P = 0.031). This result was not surprising, given that sea stars prefer mussels over all other tested prey (Paine, 1966, 1974; Landenberger, 1968; Dayton, 1971). Consequently, we repeated chemical preference tests for sea stars, substituting bovine serum albumin (BSA, an organic enrichment control) for crude extracts, together with filtered seawater and MULTIFUNCin (purified from Balanus glandula collected at Malibu) faux prey. The use of BSA was consistent with our previous studies (Zimmer et al., 2016b, 2017). Similar to Sets 1 and 2, a trial began when a single sea star (length: 7–12 cm from distal tip of longest arm to center of oral disc) was placed in the center of an arena and presented with 12 faux prey. The 12 faux prey were divided into four groups of three that encircled the sea star. As in Sets 1 and 2, the exact position of each prey type within a group was determined using a random numbers table. Each trial lasted until the predator selected, attacked and consumed a faux prey, terminating after 1 h regardless.
Results
When faux prey were prepared using Balanus glandula from Malibu populations, feeding preferences were not significantly different among the five whelk species (Chi-square test for heterogeneity with Williams’ correction for small sample sizes: χ2 = 8.896, df = 16, P = 0.928) (Figures 3A,C–F). In performing this analysis, we included a category for null responses, as well as separate categories for positive responses to each of the four experimental treatments (MULTI, MCE, TCE, and FSW). Given no significant difference, data were subsequently pooled across all five species before further analyses. This last procedure greatly increased statistical power in multiple comparisons among the experimental treatments. Combined, whelk consumers fed significantly more often on faux prey with MULTIFUNCin as opposed to seawater [Sign test with Bonferroni’s correction (α = 0.05/6 = 0.008): P < 0.00001], turban snail crude extract (P < 0.00001), and mussel crude extract (P < 0.0001). Each whelk fed only on one faux prey per trial. In addition, whelks attacked and ate significantly more faux prey with mussel crude extract than with seawater (P = 0.0018), but not with turban snail crude extract (P = 0.067). There was no significant difference in whelk reactions to turban snail crude extract and seawater (P = 0.193). Thus, overall, attack and feeding by whelks were paramount in response to the single molecule, MULTIFUNCin. As conservative tests of selectivity for this glycoprotein, MULTIFUNCin concentration (75 μg mL–1; about 4 × 10–7 molar) was approximately 50 times lower than total concentrations (w/v) of protein in crude extracts.
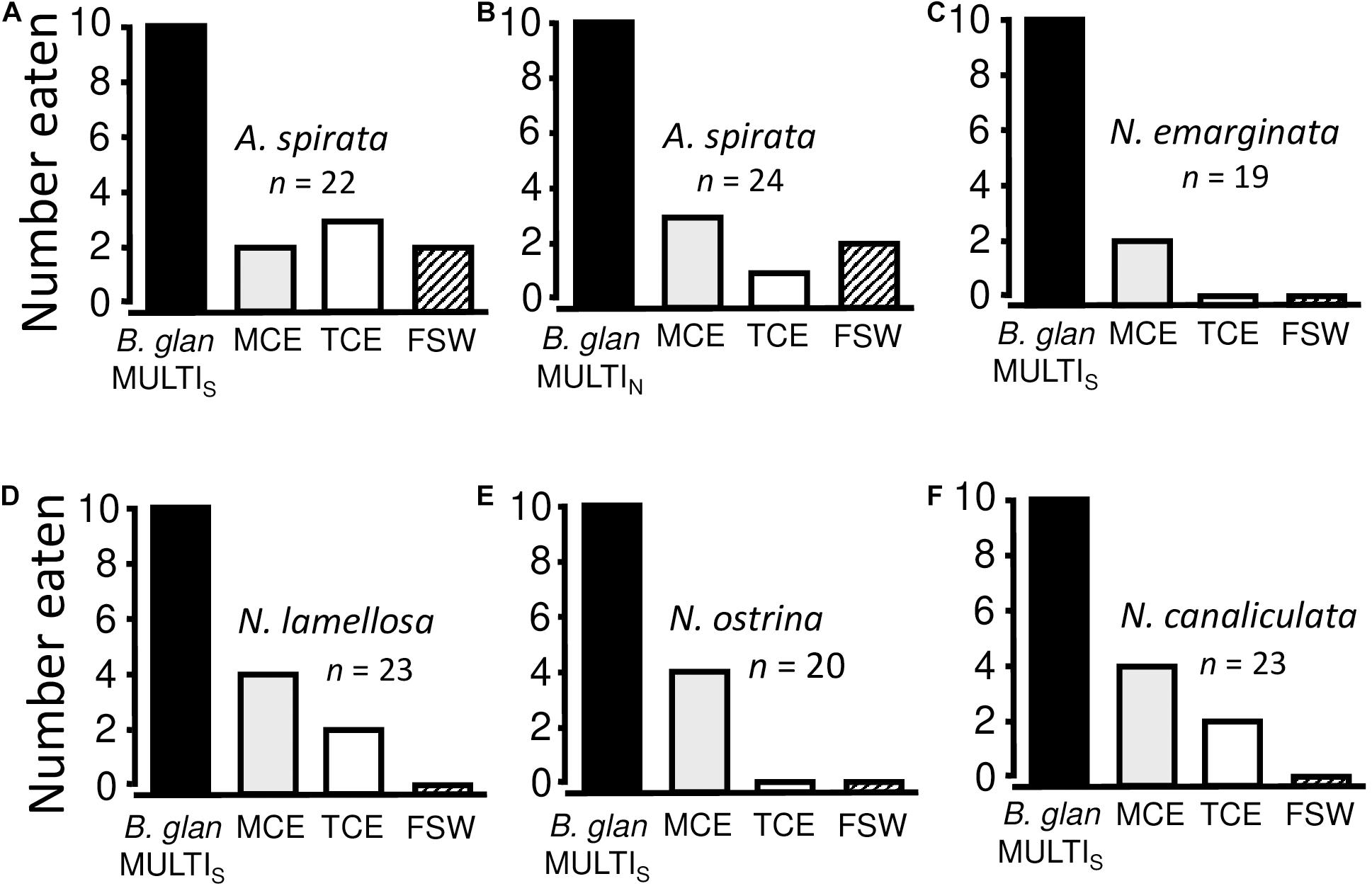
Figure 3. Feeding preferences of five whelk species for faux prey. FSW, 0.45 μm-filtered seawater infused gels; TCE, turban snail crude extract; MCE, mussel crude extract; B. glan MULTIS or N, MULTIFUNCin isolated either from a southern (Malibu, CA) or a northern (Cattle Point, WA) population of barnacles, Balanus glandula. All gels were presented in cleaned, acid-washed, barnacle tests. n = number of replicate trials. Each predator was allowed to consume no more than one faux prey in a given trial. As shown in (A), there were 22 total replicate trials (=n); a predator ate one of the four faux prey types (10 MULTI + 2 MCE + 3 TCE + 2 FSW) in 17 trials; and, a predator failed to feed on faux prey in 22–17 = 5 trials. Separate experiments were performed with (A,B) Acanthinucella spirata, (C) Nucella emarginata, (D) Nucella lamellosa, (E) Nucella ostrina, and (F) N. canaliculata. For each experiment, a bar depicts the total number of replicate trials in which a given faux prey type was eaten. All whelk species fed readily on TCE- and MCE-infused gels in the absence of MULTI-infused gels.
A strong genetic cline separates southern (Monterey Bay, southward) from northern (Half Moon Bay through Alaska) populations of B. glandula (Wares and Cunningham, 2005). The experiment with Acanthinucella spirata, therefore, was repeated using faux prey with purified MULTIFUNCin from barnacles collected at our northernmost locale, Cattle Point, WA. Chemical preferences of A. spirata were independent of the MULTIFUNCin source population (Chi-square test for heterogeneity with Williams’ correction for small sample sizes: χ2 = 1.838, df = 4, P = 0.772) (Figures 3A,B). Consequently, all other experiments were performed using only the southern barnacle variant in order to save time and purified material.
MULTIFUNCin also was isolated from a second barnacle species, Semibalanus cariosus. Results were non-significantly different between bioassays using the Semibalanus cariosus MULTIFUNCin and each of two whelk species (Acanthinucella spirata and Nucella canaliculata) (Chi-square test for heterogeneity with Williams’ correction: χ2 = 2.772, df = 4, P = 0.621) (Figures 4A,B). Combined, whelks significantly preferred S. cariosus MULTIFUNCin over mussel and turban snail crude extracts, and over seawater (Sign test with Bonferroni’s correction, α = 0.008: P ≤ 0.0061, all comparisons). In addition, findings were similar for S. cariosus MULTIFUNCin and Balanus glandula MULTIFUNCin (compare Figures 3A–4A, Figures 3F–4B) (Chi-square tests for heterogeneity with Williams’ correction: χ2 ≤ 5.480, df = 4, P ≥ 0.286, both comparisons). Each whelk species did not discriminate between faux prey containing MULTIFUNCin from one or the other barnacle species (unpublished data). The two compounds, therefore, are likely quantitatively equivalent with respect to chemosensory recognition.
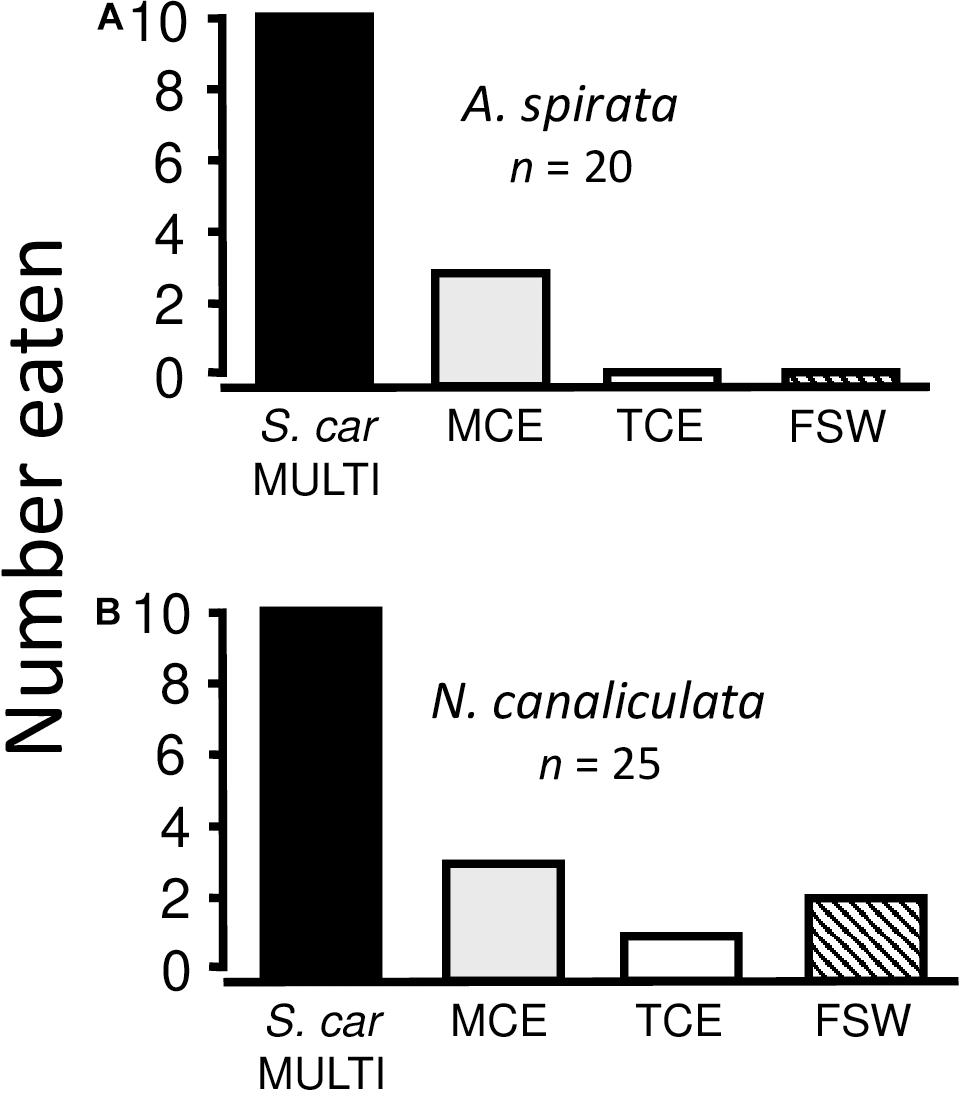
Figure 4. Feeding preferences of two whelk species (A, Acanthinucella spirata; B, Nucella canaliculata) for faux prey. All conditions are as described in Figures 2, 3 legends. In this case, MULTIFUNCin was isolated from the barnacle, Semibalanus cariosus. n = number of replicate trials.
Bioassays with sea stars were limited in scope. The number of trials was restricted by system failures of our aged FPLC, which occurred after whelk and during sea star experiments. When substituting BSA for crude extracts, sea stars fed significantly more often on MULTIFUNCin-laced faux prey (Figure 5). Here, sea stars preferred MULTIFUNCin over both organic enrichment and seawater (absence of organics) controls (Sign tests with Bonferroni’s correction, α = 0.0167: P ≤ 0.0059, both comparisons).
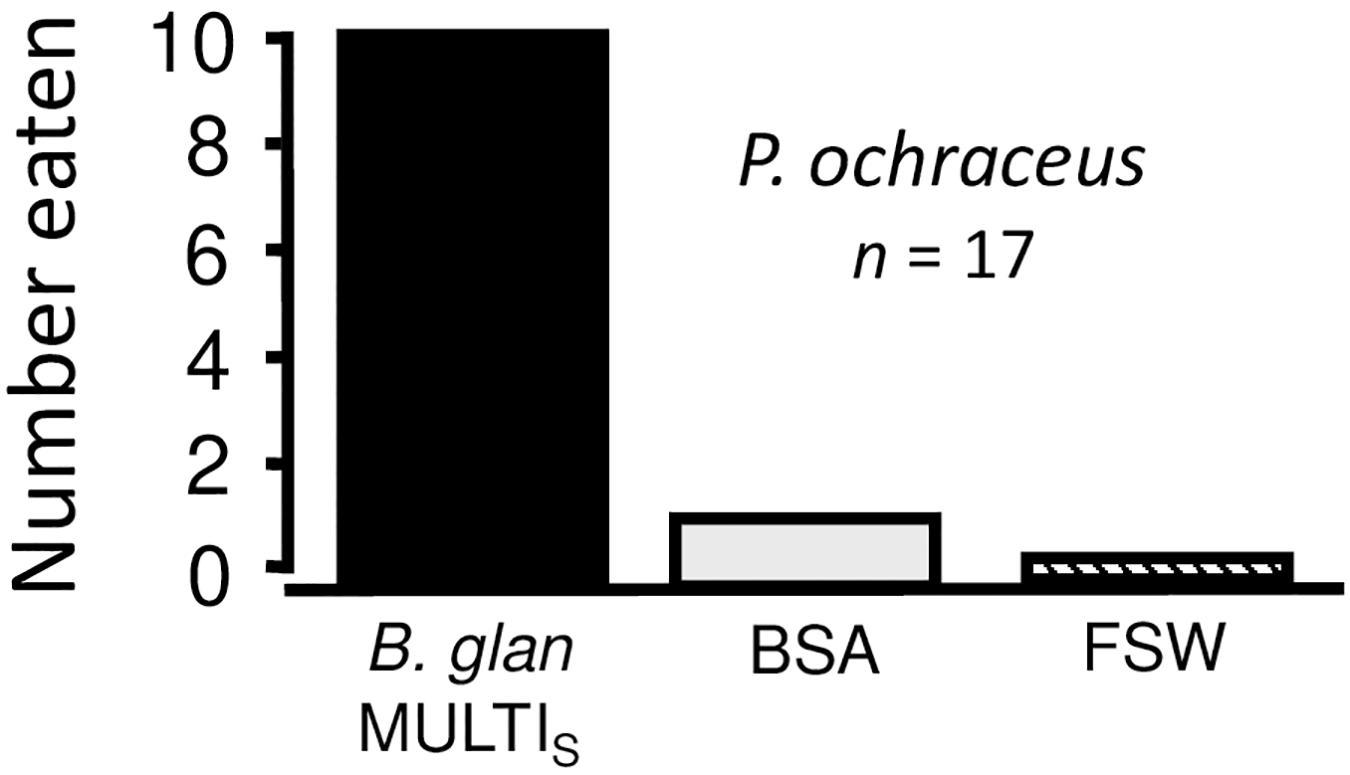
Figure 5. Feeding preferences of sea stars (Pisaster ochraceus) for faux prey. All conditions are as described in Figures 2, 3 legends. In this case, we used bovine serum albumin (BSA) as permeant in place of MCE and TCE. BSA thus served as an organic enrichment control. n = number of replicate trials.
Discussion
Many armed predatory species have specialized diets and feed only on hard-shell or exoskeleton-bearing organisms. The degree to which these exploiters and victims have evolved in response to one another has been difficult to discern. This problem has been especially challenging in regard to the predators. For example, development of specialized weaponry may enhance the likelihood for prey capture and subjugation. Yet, such innovation commonly evolves as a consequence of selection pressures imposed, not by the prey, but by competitors and/or higher-order consumers (Dietl and Kelley, 2002; Vermeij, 2013). In addition to morphological traits, species-, or taxon-specific chemical cues would provide a meaningful axis for evaluation of relations among enemy combatants. It has been well established that armored prey exhibit strong behavioral and morphological responses to unique infochemicals released by specific (and deadly) durophagous consumers (Poulin et al., 2018; Rittschar et al., 2020). In contrast, until now, there has been little evidence for the reciprocal interaction.
This study identifies chemosensory exploitation by armed consumers as an evolved predatory response to armored prey. Specifically, our results show that all six tested consumer species attacked and ate MULTIFUNCin-infused faux barnacle prey significantly more than controls. In fact, MULTIFUNCin alone was sufficient to trigger a predatory response. After 670 million years of genetic isolation (Ayala et al., 1998), molecular mechanisms for chemical recognition differ considerably among protostomes (including mollusks) and deuterostomes (including echinoderms) (Bargmann, 2006; Cummins et al., 2009; Derby et al., 2016). Yet, for sea star and whelk consumers, glycoprotein detection has converged across the protostome-deuterostome division to promote durophagy on a valuable, shared, prey resource.
Resistant (or tolerant) consumers have evolved the ability to recognize toxins and poisons using chemosensory mechanisms. Thus, select predatory species can dine on specific, chemically defended prey species (Bernays et al., 2002; Hwang et al., 2007; Wink, 2018). Such biotic interactions play seminal roles, shaping community structure and function and, in some cases, triggering arms races and co-evolution especially among plants and herbivores (Anderson and Mitchell-Olds, 2011; Bruce, 2015). Unlike chemical defense compounds, molecules uniquely associated with armored defenses would impact ecological relationships without the need for toxin resistance or tolerance in source and/or consumer species. Given freedom from these evolutionary constraints, chemosensory exploitation of prey body armor is likely widespread, acting as a critical ecological determinant.
Barnacles are widely distributed on wave-swept shores along the entire North America Pacific Ocean coast (see Table 1). Here, we identify a potential community role for chemosensory exploitation by armed consumers. In response to a glycoprotein cue (MULTIFUNCin), predation by sea stars, and especially by whelks, could limit the abundances and distributions of prey (barnacle) populations, thereby altering the availability of community resources, including food and space (Feder, 1959; Connell, 1961, 1970; Dayton, 1971). Such changes would lead to new “winners” and “losers” in a sweepstakes among subordinate and dependent species, thus initiating cascades of community-wide impacts (Connell, 1972; Maggi et al., 2015).
Shared, conserved domains place MULTIFUNCin in the α2-macroglobulin (A2M) subgroup of the thioester-containing protein (TEP) family (Ferrier et al., 2016a). The A2Ms are vital to structural defenses (Armstrong and Quigley, 1999) and for the attack and subjugation of surface-colonizing microbial pathogens (Chu and Pizzo, 1994; Blandin and Levashina, 2004; Budd et al., 2004; Armstrong, 2010). MULTIFUNCin orthologs also cue habitat colonization by conspecific larvae and possibly act as pheromones (Dreanno et al., 2006; Zimmer et al., 2016a). To maintain these critical, ancestral, functions, we propose that MULTIFUNCin has been subjected to purifying selection in barnacles. Purifying (or “negative”) selection would eliminate deleterious mutant alleles in order to maintain stringent binding affinities and other, essential molecular properties. Accordingly, MULTIFUNCin would evolve as an honest and reliable cue in chemosensory exploitation by armed predators.
Chemosensory exploitation extends beyond barnacles and their predators on rocky, wave-swept shores. Akin to barnacles, mussels (Mytilus californianus, M. edulis, and M. galloprovincialis) dominate space and act as foundation species on wave-swept shores (at intermediate and/or low tidal levels) (Paine, 1966; Dayton, 1971; Suchanek, 1992). Each mussel species produces and secretes a glycoprotein ortholog (named KEYSTONEin, molecular weights: 28.1–29.6 kDa) which acts, singularly, to cue sea star, crab, and whelk predation (Zimmer et al., 2016b, 2017). These orthologs are uniquely associated with mussel body armor (extrapallial fluid, epidermis, periostracum, and shell materials). A large, conserved, globular domain places KEYSTONEin within the Complement Component 1 Domain Containing (C1qDC) protein family (Nonaka and Kimura, 2006; Carland and Gerwick, 2010). C1q proteins are part of the classical complement pathway and are critical especially to the innate immune systems of invertebrates (Gestal et al., 2010; Gerdol et al., 2011). For mussels, these compounds are also secreted by the mantle epidermis and act in shell formation through Ca2+ binding and subsequent calcium carbonate deposition (Hattan et al., 2001; Yin et al., 2005).
Chemical recognition via surface glycoproteins is not limited to species on wave-swept shores (Wyatt, 2014). The molecules serve, for example, as signals when bound in the integuments of rotifers, crustaceans, sea hares, squids, insects, amphibians (newts), and mice (Snell and Carmona, 1994; Painter et al., 2004; Dreanno et al., 2006; Snell et al., 2009; Woodley, 2010; Cummins et al., 2011; Matsuura, 2012; Roberts et al., 2012). They also act in communication between metazoan sperm and egg, and function as stringent pre-zygotic barriers that block hybridization among gametes of different species (Vacquier, 1998; Wilburn and Swanson, 2016).
Often requisite for internal (cell level) or external (organismal level) communication, glycoproteins are well suited for chemosensory exploitation. A prime example is the targeting of host-expressed mucin and collagen by pathogenic bacteria for attachment and colony establishment (Roos and Jonsson, 2002). In fact, thousands of armored metazoan species—from marine sponges to terrestrial vertebrates—secrete glycoprotein-rich extracellular matrices (Sarashina and Endo, 2006; Ehrlich, 2010; Wang and Nilsen-Hamilton, 2013). As an evolved counter-adaptation by durophagous predators, chemosensory exploitation of glycoproteins may well have notable effects across the metazoan tree-of-life.
Data Availability Statement
The original contributions presented in the study are included in the article/supplementary material; further inquiries can be directed to the corresponding author.
Author Contributions
RZ, GF, and CZ: conceptualization, methodology, formal analysis, investigation, writing—review, and editing. RZ: writing—original draft. RZ and CZ: project administration, funding acquisition. All authors contributed to the article and approved the submitted version.
Funding
This research was supported by awards from the U.S. National Science Foundation and the UCLA Council on search.
Conflict of Interest
The authors declare that the research was conducted in the absence of any commercial or financial relationships that could be construed as a potential conflict of interest.
Publisher’s Note
All claims expressed in this article are solely those of the authors and do not necessarily represent those of their affiliated organizations, or those of the publisher, the editors and the reviewers. Any product that may be evaluated in this article, or claim that may be made by its manufacturer, is not guaranteed or endorsed by the publisher.
Acknowledgments
The authors express their sincere gratitude to the UCLA undergraduates (Michal Ross, Margaret McGonigle, Erin Eastwood, Noelle Bidegainberry, and Christopher Leber) who provided valuable contributions to the project.
References
Anderson, J. T., and Mitchell-Olds, T. (2011). Ecological genetics and genomics of plant defences: evidence and approaches. Funct. Ecol. 25, 312–324. doi: 10.1111/j.1365-2435.2010.01785.x
Aristide, L., and Morlon, H. (2019). Understanding the effect of competition during evolutionary radiations: an integrated model of phenotypic and species diversification. Ecol. Lett. 22, 2006–2017. doi: 10.1111/ele.13385
Armstrong, P. B. (2010). Role of α2-macroglobulin in the immune response of invertebrates. Invert. Survival J. 7, 165–180.
Armstrong, P. B., and Quigley, J. P. (1999). α2-macroglobulin: an evolutionarily conserved arm of the innate immune system. Dev. Comp. Immunol. 23, 375–390. doi: 10.1016/s0145-305x(99)00018-x
Ayala, F. J., Rzhetsky, A., and Ayala, F. J. (1998). Origin of the metazoan phyla: molecular clocks confirm paleontological estimates. Proc. Natl. Acad. Sci. U.S.A. 95, 606–611. doi: 10.1073/pnas.95.2.606
Bargmann, C. I. (2006). Comparative chemosensation from receptors to ecology. Nature 444, 295–301. doi: 10.1038/nature05402
Bernays, E. A., Chapman, R. F., and Hartmann, T. (2002). A highly sensitive taste receptor cell for pyrrolizidine alkaloids in the lateral galeal sensillum of a polyphagous caterpillar, Estigmene acrea. J. Comp. Physiol. A 188, 715–723. doi: 10.1007/s00359-002-0345-3
Bicknell, R. D. C., and Paterson, J. R. (2018). Reappraising the early evidence of durophagy and drilling predation in the fossil record. Biol. Rev. 93, 754–784. doi: 10.1111/brv.12365
Blandin, S., and Levashina, E. A. (2004). Thioester-containing proteins and insect immunity. Molec. Immunol. 40, 903–908. doi: 10.1016/j.molimm.2003.10.010
Bradford, M. M. (1976). A rapid and sensitive method for quantification of microgram quantities of protein utilizing the principle of protein-dye binding. Anal. Biochem. 72, 248–254. doi: 10.1006/abio.1976.9999
Brodie, E. D. 3rd., and Brodie, E. D. Jr. (1999). Predator-prey arms races. BioScience 49, 557–568. doi: 10.2307/1313476
Bruce, T. J. A. (2015). Interplay between insects and plants: dynamic and complex interactions that have coevolved over millions of years but act in milliseconds. J. Exp. Bot. 66, 455–465. doi: 10.1093/jxb/eru391
Budd, A., Blandin, S., Levashina, E. A., and Gibson, T. J. (2004). Bacterial α2-macroglobulins: colonization factors acquired by horizontal gene transfer from the metazoan genome? Genome Biol. 5:R38. doi: 10.1186/gb-2004-5-6-r38
Carland, T. M., and Gerwick, L. (2010). The C1q domain containing proteins: where do they come from and what do they do? Dev. Comp. Immunol. 34, 785–790. doi: 10.1016/j.dci.2010.02.014
Chu, C. T., and Pizzo, S. V. (1994). Alpha 2-macroglobulin, complement, and biologic defense: antigens, growth factors, microbial proteases, and receptor ligation. Lab. Invest. 71, 792–812.
Connell, J. H. (1961). Effects of competition, predation by Thais lapillus, and other factors on natural populations of the barnacle Balanus balanoides. Ecol. Monogr. 31, 61–104. doi: 10.2307/1950746
Connell, J. H. (1970). A predator-prey system in the marine intertidal region. I. Balanus glandula and several predatory species of Thais. Ecol. Monogr. 40, 49–78. doi: 10.2307/1942441
Connell, J. H. (1972). Community interactions on marine rocky, intertidal shores. Annu. Rev. Ecol. Syst. 3, 169–192. doi: 10.1146/annurev.es.03.110172.001125
Crisp, D. J. (1971). Structure and abundance of receptors of the unspecialized epithelium of Nassarius reticulatus (Gastropoda, Prosobranchia). J. Mar. Biol. Assoc., U.K. 51, 865–890. doi: 10.1017/s0025315400018026
Cummins, S. F., Boal, J. G., Buresch, K. C., Kuanpradit, C., Sobhon, P., Holm, J. B., et al. (2011). Extreme aggression in male squid induced by a Beta-MSP-like pheromone. Curr. Biol. 21, 322–327. doi: 10.1016/j.cub.2011.01.038
Cummins, S. F., Erpenbeck, D., Zou, Z., Claudianos, C., Moroz, L. L., Nagle, G. T., et al. (2009). Candidate chemoreceptor subfamilies differentially expressed in the chemosensory organs of the mollusk Aplysia. BMC Biol. 7:28. doi: 10.1186/1741-7007-7-28
Dawkins, R., and Krebs, J. R. (1979). Arms races between and within species. Proc. R. Soc. Lond. B 205, 489–511. doi: 10.1098/rspb.1979.0081
Dayton, P. K. (1971). Competition, disturbance, and community organization: the provision and subsequent utilization of space in a rocky intertidal community. Ecol. Monogr. 41, 351–389. doi: 10.2307/1948498
Derby, C. D., Kozma, M. T., Senatore, A., and Schmidt, M. (2016). Molecular mechanisms of reception and perireception in crustacean chemoreception: a comparative review. Chem. Senses 41, 381–398. doi: 10.1093/chemse/bjw057
Dietl, G. P., and Kelley, P. H. (2002). The fossil record of predator-prey races: co-evolution and escalation hypotheses. Paleontol. Soc. Pap. 8, 353–374. doi: 10.1017/s1089332600001157
Dreanno, C., Matsumura, K., Dohmae, N., Takio, K., Hirota, H., Kirby, R. A., et al. (2006). An α2-macroglobulin-like protein is the cue to gregarious settlement of the barnacle Balanus amphitrite. Proc. Natl. Acad. Sci. U.S.A. 103, 14396–14401. doi: 10.1073/pnas.0602763103
Ehrlich, H. (2010). Chitin and collagen as universal and alternative templates in biomineralization. Internat. Geol. Rev. 52, 661–699. doi: 10.1080/00206811003679521
Feder, H. M. (1959). The food of the starfish Pisaster ochraceus along the California coast. Ecology 40, 721–724. doi: 10.2307/1929828
Ferrier, G. A., Kim, S. J., Kaddis, C. S., Loo, J. A., Zimmer, C. A., and Zimmer, R. K. (2016a). MULTIFUNCin: a multifunctional protein cue induces habitat selection by, and predation on, barnacles. Integrat. Comp. Biol. 56, 901–913.
Ferrier, G. A., Zimmer, C. A., and Zimmer, R. K. (2016b). Chemical ecology of wave-swept shores: the primacy of contact cues in predation by whelks. Biol. Bull. 231, 207–215. doi: 10.1086/691068
Gerdol, M., Manfrin, C., De Moro, G., Figueras, A., Novoa, B., Venier, P., et al. (2011). The C1q domain containing proteins of the Mediterranean mussel Mytilus galloprovincialis: a widespread and diverse family of immune-related molecules. Dev. Comp. Immunol. 35, 635–643. doi: 10.1016/j.dci.2011.01.018
Gestal, C., Pallavicini, A., Venier, P., Novoa, B., and Figueras, A. (2010). MgC1q: a novel C1q-domain-containing protein involved in the immune response of Mytilus galloprovincialis. Dev. Comp. Immunol. 34, 926–934. doi: 10.1016/j.dci.2010.02.012
Harvell, C. D. (1984). Predator-induced defense in a marine bryozoan. Science 224, 1357–1359. doi: 10.1126/science.224.4655.1357
Hattan, S. J., Laue, T. M., and Chasteen, N. D. (2001). Purification and characterization of a novel calcium-binding protein from the extrapallial fluid of the mollusk, Mytilus edulis. J. Biochem. 276, 4461–4468. doi: 10.1074/jbc.M006803200
Hodgson, A. N., and Brown, A. C. (1985). Contact chemoreception by the propodium of the sandy beach whelk Bullia digitalia (Gastropoda, Nassariidae). Comp. Biochem. Physiol. A 82, 425–427. doi: 10.1016/0300-9629(85)90878-3
Hwang, P., Noguchi, T., and Hwang, D. F. (2007). Neurotoxin tetrodotoxin as an attractant for toxic snails. Fish. Sci. 70, 1106–1112. doi: 10.1055/s-2005-917664
Jacobson, H. P., and Stabell, O. B. (2004). Antipredator behaviour mediated by chemical cues: the role of conspecific alarm signaling and predator labelling in the avoidance response of a marine gastropod. Oikos 104, 43–50. doi: 10.1111/j.0030-1299.2004.12369.x
Klompmaker, A. A., Kowalewski, M., Huntley, J. W., and Finnegan, S. (2017). Increase in predator-prey size ratios throughout the Phanerozoic history of marine ecosystems. Science 356, 1178–1180. doi: 10.1126/science.aam7468
Kosloski, M. E., and Allmon, W. D. (2015). Macroecology and evolution of a crab ‘super predator’, Menippe mercenaria (Menippidae), and its gastropod prey. Biol. J. Linnean Soc. 116, 571–581. doi: 10.1111/bij.12622
Kruppert, S., Chu, F., Stewart, M. C., Schmitz, L., and Summers, A. P. (2020). Ontogeny and potential function of poacher armor (Actinopterygii: agonidae). J. Morphol. 281, 1018–1028. doi: 10.1002/jmor.21223
Landenberger, D. E. (1968). Studies on selective feeding in the Pacific starfish Pisaster in southern California. Ecology 49, 1062–1075. doi: 10.2307/1934490
Maggi, E., Milazzo, M., Graziano, M., Chemello, R., and Benedetti-Cecchi, L. (2015). Latitudinal- and local-scale variations in a rocky intertidal interaction web. Mar. Ecol. Prog. Ser. 534, 39–48.
Marshall, C. R. (2006). Explaining the Cambrian “explosion” of animals. Annu. Rev. Earth Planet. Sci. 34, 355–384. doi: 10.1146/annurev.earth.33.031504.103001
Matsuura, K. (2012). Multifunctional queen pheromone and maintenance of reproductive harmony in termite colonies. J. Chem. Ecol. 38, 746–754. doi: 10.1007/s10886-012-0137-3
McClintock, J. B., Baker, B. J., Slattery, M., Hamann, M., Kopitzke, R., and Heine, J. (1994). Chemotactic tube-foot responses of a spongivore sea star Perknaster fuscus to organic extracts from Antarctic sponges. J. Chem. Ecol. 20, 859–870. doi: 10.1007/BF02059583
Murdoch, W. W. (1969). Switching in general predators: experiments on predator specificity and stability of prey populations. Ecol. Monogr. 39, 335–354. doi: 10.2307/1942352
Navarrete, S. A. (1996). Variable predation: effects of whelks on a mid-intertidal successional community. Ecol. Monogr. 66, 301–321. doi: 10.2307/2963520
Nonaka, M., and Kimura, A. (2006). Genomic view of the evolution of the complement system. Immunogenetics 58, 701–713. doi: 10.1007/s00251-006-0142-1
Ono, M., Terabe, H., Hori, H., and Sasaki, M. (2003). Insect signaling: components of giant hornet alarm pheromone. Nature 103, 91–93. doi: 10.1038/424637a
Paine, R. T. (1966). Food web complexity and species diversity. Am. Nat. 100, 65–75. doi: 10.1086/282400
Paine, R. T. (1974). Intertidal community structure. Experimental studies on the relationship between a dominant competitor and its principal predator. Oecologia 15, 93–120. doi: 10.1007/BF00345739
Painter, S. D., Cummins, S. F., Nichols, A. E., Akalal, D. B., Schein, C. H., Braun, W., et al. (2004). Structural and functional analysis of Aplysia attractins: a family of water-borne protein pheromones with interspecific attractiveness. Proc. Natl. Acad. Sci. U.S.A. 101, 6929–6933. doi: 10.1073/pnas.0306339101
Poulin, R. X., Lavoie, S., Siegel, K., Gaul, D. A., Weissburg, M. J., and Kubanek, J. (2018). Chemical encoding of risk perception and predator detection among estuarine invertebrates. Proc. Natl. Acad. Sci. U.S.A. 115, 662–667. doi: 10.1073/pnas.1713901115
Rittschar, S., Narayana, V. K. B., Rabus, M., and Laforsch, C. (2020). Uncovering the chemistry behind inducible morphological defences in the crustacean Daphnia magna via micro-Raman spectroscopy. Sci. Rep. 10:22408. doi: 10.1038/s41598-020-79755-4
Roberts, S. A., Davidson, A. J., McLean, L., Beynon, R. J., and Hurst, J. L. (2012). Pheromonal induction of spatial learning in mice. Science 338, 1462–1465. doi: 10.1126/science.1225638
Roos, S., and Jonsson, H. (2002). A high-molecular-mass cell-surface protein from Lactobacillus reuteri 1063 adheres to mucus components. Microbiology 148, 433–442. doi: 10.1099/00221287-148-2-433
Sarashina, I., and Endo, K. (2006). Skeletal matrix proteins of invertebrate animals: comparative analysis of their amino acid sequences. Paleontol. Res. 10, 311–336.
Smee, D. L., and Weissburg, M. W. (2006). Hard clams (Mercenaria mercenaria) evaluate predation risk using chemical signals from predators and injured conspecifics. J. Chem. Ecol. 32, 605–619. doi: 10.1007/s10886-005-9021-8
Snell, T. W., and Carmona, M. J. (1994). Surface glycoproteins in copepods: potential signals for mate recognition. Hydrobiologia 292, 255–264. doi: 10.1007/BF00229949
Snell, T. W., Shearer, T., Smith, H., Kubanek, J., Gribble, K., and Welch, D. M. (2009). Genetic determinants of mate recognition in Brachionus manjavacas (Rotifera). BMC Biol. 7:60. doi: 10.1186/1741-7007-7-60
Suchanek, T. (1992). Extreme biodiversity in the marine environment: mussel bed communities of Mytilus californianus. Northwest Environ. J. 8, 150–152.
Trussel, G. C., Ewanchuk, P. J., and Bertness, M. D. (2003). Trait-mediated effects in rocky intertidal food chains: predator risk cues alter prey feeding rates. Ecology 84, 629–640. doi: 10.1890/0012-9658(2003)084[0629:tmeiri]2.0.co;2
Vacquier, V. D. (1998). Evolution of gamete recognition proteins. Science 281, 1995–1998. doi: 10.1126/science.281.5385.1995
Vermeij, G. J. (1994). The evolutionary interaction among species: selection, escalation, and coevolution. Annu. Rev. Ecol. Syst. 25, 219–236. doi: 10.1146/annurev.es.25.110194.001251
Vermeij, G. J. (2013). On escalation. Annu. Rev. Earth Planet. Sci. 41, 1–19. doi: 10.1093/acprof:oso/9780198289531.003.0001
Wang, L., and Nilsen-Hamilton, M. (2013). Biomineralization proteins: from vertebrates to bacteria. Frontiers Biol. 8, 234–246. doi: 10.1007/s11515-012-1205-3
Wares, J. P., and Cunningham, C. W. (2005). Diversification before the most recent glaciation in Balanus glandula. Biol. Bull. 208, 60–68. doi: 10.2307/3593101
Weiss, L. C., Albada, B., Becker, S. M., Mechkelmann, S. V., Klein, J., Meyer, M., et al. (2018). Identification of Chaoborus kairomone chemicals that induce defences in Daphnia. Nat. Chem. Biol. 14, 1133–1139. doi: 10.1038/s41589-018-0164-7
Whitenack, L. B., and Herbert, G. S. (2015). Did shell-crushing crabs trigger an escalatory arms race in the aftermath of a late Neogene regional mass extinction event? An experimental test. J. Exp. Mar. Biol. Ecol. 417, 57–65. doi: 10.1016/j.palaeo.2014.09.026
Wilburn, D. B., and Swanson, W. J. (2016). From molecules to mating: rapid evolution and biochemical studies of reproductive proteins. J. Proteom. 135, 12–15. doi: 10.1016/j.jprot.2015.06.007
Wink, M. (2018). Plant secondary metabolites modulate insect behavior – Steps towards addiction. Front. Physiol. 9:364. doi: 10.3389/fphys.2018.00364
Wood, R., and Zhuravlev, A. Y. (2012). Escalation and ecological selectivity of minerology in the Cambrian radiation of skeletons. Earth-Sci. Rev. 115, 249–261. doi: 10.1016/j.earscirev.2012.10.002
Woodley, S. K. (2010). Pheromonal communication in amphibians. J. Comp. Physiol. A 196, 713–727. doi: 10.1007/s00359-010-0540-6
Wyatt, T. D. (2014). Proteins and peptides as pheromone signals and chemical signatures. Anim. Behav. 97, 273–280. doi: 10.1016/j.anbehav.2014.07.025
Yin, Y., Huang, J., Paine, M. L., Reinhold, V. N., and Chasteen, N. D. (2005). Structural characterization of the major extrapallial fluid proteins of the Mollusc Mytilus edulis: implications for function. Biochemistry 44, 10720–10731. doi: 10.1021/bi0505565
Zimmer, R. K., Ferrier, G. A., Kim, S. J., Kaddas, C. S., Zimmer, C. A., and Loo, J. A. (2016a). A multifunctional chemical cue drives opposing demographic processes and structures ecological communities. Ecology 97, 2232–2239. doi: 10.1002/ecy.1455
Zimmer, R. K., Ferrier, G. A., Kim, S. J., Ogorzalek Loo, R. R., Zimmer, C. A., and Loo, J. A. (2017). Keystone predation and molecules of keystone significance. Ecology 98, 1710–1721. doi: 10.1002/ecy.1849
Zimmer, R. K., Ferrier, G. A., and Zimmer, C. A. (2016b). KEYSTONEin: a glycoprotein cue drives predation on mussels and structures rocky intertidal communities. Mar. Ecol. Progr. Ser. 560, 199–206. doi: 10.3354/meps11939
Keywords: chemical cue, feeding stimulant, durophagy, sensory ecology, predation
Citation: Zimmer RK, Ferrier GA and Zimmer CA (2021) Chemosensory Exploitation and Predator-Prey Arms Races. Front. Ecol. Evol. 9:752327. doi: 10.3389/fevo.2021.752327
Received: 02 August 2021; Accepted: 14 October 2021;
Published: 12 November 2021.
Edited by:
Linda C. Weiss, Ruhr University Bochum, GermanyReviewed by:
Dörthe Becker, The University of Sheffield, United KingdomKathrin Otte, University of Cologne, Germany
Copyright © 2021 Zimmer, Ferrier and Zimmer. This is an open-access article distributed under the terms of the Creative Commons Attribution License (CC BY). The use, distribution or reproduction in other forums is permitted, provided the original author(s) and the copyright owner(s) are credited and that the original publication in this journal is cited, in accordance with accepted academic practice. No use, distribution or reproduction is permitted which does not comply with these terms.
*Correspondence: Richard K. Zimmer, ekBiaW9sb2d5LnVjbGEuZWR1
†Present address: Graham A. Ferrier, Pfizer Incorporated, New York, NY, United States