- 1Office of Undergraduate Research, University of Virginia, Charlottesville, VA, United States
- 2Department of Environmental Sciences, University of Virginia, Charlottesville, VA, United States
- 3Blandy Experimental Farm, University of Virginia, Boyce, VA, United States
Artificial light at night (ALAN) can impact the trophic structure of assemblages of ground-dwelling invertebrates, and changes in such assemblages can affect decomposition in terrestrial systems due to the various functional roles of these invertebrates, including microbial grazing, comminution of litter, and predation of other invertebrates, that can directly or indirectly affect plant-litter breakdown. Despite this, we are unaware of any studies that have evaluated the effects of ALAN on the breakdown of plant litter in a terrestrial ecosystem. We sought to answer whether ALAN affects litter breakdown via its effects on a community of ground-dwelling arthropods using two field experiments. In one experiment, we manipulated the presence of ALAN and the size classes of soil invertebrates that could enter mesh bags containing plant litter (litterbags). We found that the rate of plant-litter breakdown increased with the mesh size of litterbags but was unaffected by presence of ALAN. In a second field experiment carried out to examine the effects of ALAN on the trophic structure of litter-layer invertebrate communities, while controlling for potential effects of ALAN on vegetation, we again found that ALAN did not affect litter breakdown despite the fact that ALAN increased the abundances of secondary and tertiary consumers. Our finding that larger assemblages of ground-dwelling secondary and tertiary consumer invertebrates under ALAN did not slow litter breakdown through increased top-down control of detritivores suggests ALAN may disrupt predator-prey interactions in litter-layer communities.
Introduction
Artificial light at night (hereafter ALAN) is a widespread sensory pollutant which currently affects nearly a quarter of the terrestrial surface of our planet (Gaston et al., 2014; Falchi et al., 2016, 2019; Kyba et al., 2017). It is widely considered to have extensive ecological consequences across levels of biological organization ranging from the organism (e.g., physiology and behavior) to the ecosystem (Longcore and Rich, 2004; Gaston et al., 2013, 2014). Most research documenting effects of ALAN has occurred at the organismal and population levels (Longcore and Rich, 2004; Hölker et al., 2010; Gaston et al., 2015; Sanders and Gaston, 2018). Perhaps the best evidence that ALAN affects higher levels of organization are studies that have shown effects on multi-trophic structure or dynamics (Meyer and Sullivan, 2013; Bennie et al., 2018a; Grenis and Murphy, 2019; Sullivan et al., 2019). At the community level there is increasing recognition of the potential for ALAN to disrupt pollination (Knop et al., 2017; Macgregor et al., 2017; Giavi et al., 2020) as well as invertebrate food-web dynamics (Bennie et al., 2018a; Sanders et al., 2018; Maggi et al., 2019). However, effects of ALAN on the ecosystem processes of nutrient transfer and decomposition are largely unknown.
Decomposition is a critical ecosystem process driving nutrient transfer from dead organic matter to plant-available forms, which in turn can affect plant growth and carbon fixation (Bardgett, 2005). The structure and trophic dynamics of ground-dwelling invertebrate assemblages have profound effects on the decomposition of organic matter in terrestrial ecosystems (Moran et al., 1996; Heneghan et al., 1998; Schmitz, 2009; Hawlena et al., 2012; Tonin et al., 2018). For example, the presence of predatory invertebrates can slow decomposition by limiting the activity of detritivores, which break down plant material in the litter layer (Kajak, 1995; Lawrence and Wise, 2000; Schmitz et al., 2010; Hawlena et al., 2012). The opposite has also been found, wherein the absence of predatory invertebrates slows decomposition, which could be attributed to competition among prey invertebrates in the litter layer (Lawrence and Wise, 2004; Melguizo-Ruiz et al., 2020). Artificial light at night is known to impact the composition of invertebrate assemblages (Meyer and Sullivan, 2013; Davies et al., 2017; Manfrin et al., 2017; Desouhant et al., 2019) and their trophic dynamics (Sanders et al., 2015, 2018; Bennie et al., 2018a; Manfrin et al., 2018; Sanders and Gaston, 2018). Multiple studies have documented that ground-dwelling invertebrate assemblages under ALAN have higher abundances of predators such as arachnids and carabid beetles than those found in areas that are dark at night (Davies et al., 2012, 2017; Manfrin et al., 2017; McMunn et al., 2019; Willmott et al., 2019). The attraction of predators to ALAN-affected areas has been predicted to lead to increased top-down control (Sanders and Gaston, 2018). Given that (a) large ground-dwelling predatory invertebrates are capable of initiating trophic cascades (Moran et al., 1996; Schmitz, 2007, 2009; Hawlena et al., 2012) and that (b) greater numbers of these are observed in light-polluted conditions (Wolff, 1982; Davies et al., 2012, 2017; Holzhauer et al., 2015), ALAN may elicit indirect effects on decomposition by increasing the strength of top-down control over detritivores. Despite the observed effects of ALAN on trophic structure of ground-dwelling invertebrates (Davies et al., 2012, 2017; Meyer and Sullivan, 2013; Manfrin et al., 2017), the effects of ALAN on decomposition of organic matter in terrestrial systems are poorly understood.
Three interacting factors most strongly influence the rate of decomposition in terrestrial systems: abiotic conditions, litter nutritional quality (primarily nitrogen content), and the composition of soil fauna and microorganisms (Swift et al., 1979; Wardle et al., 2004; Hättenschwiler et al., 2005; García-Palacios et al., 2016). In the litter-layer food web there are typically up to three levels of consumers: primary, secondary, and tertiary. Primary consumers, including microorganisms (bacteria and fungi) and invertebrate detritivores, feed directly on dead plant matter. Primary consumers drive most terrestrial decomposition (Swift et al., 1979; McGuire and Treseder, 2010). Detritivores enhance the activity of bacteria and fungi by fragmenting plant material, thereby increasing attackable surface area, and by depositing frass (Vossbrinck et al., 1979; Beare et al., 1992; Coleman et al., 2004). Secondary consumers, in contrast, can slow the breakdown of plant matter through their consumption of bacteria, fungi, or detritivores. In systems where decomposition is primarily driven by bacterial or fungal pathways, secondary consumers which regulate bacterial and fungal populations include protozoa, nematodes, and mites (Santos et al., 1981; Ruess and Ferris, 2004). Tertiary consumers can enhance the rate of decomposition by releasing microbial decomposers from predation from secondary consumers (Hedlund and Ohrn, 2000; Lawrence and Wise, 2004), and can also slow decomposition by exerting top-down effects on detritivores (Kajak, 1995; Lawrence and Wise, 2000; Hawlena et al., 2012).
We conducted two studies to explore the effects of ALAN on the breakdown of plant litter in a temperate grassland ecosystem through its effects on litter-layer fauna. First, to test for potential effects of ALAN on decomposition mediated by multitrophic interactions (e.g., trophic cascades), we carried out a field experiment in which we manipulated the presence/absence of ALAN and the size classes of soil fauna. We did this by quantifying rates of litter breakdown, or proportion decomposed over time, within litterbags of three different mesh sizes in plots that were exposed to ALAN or ambient light levels at night. Exclusion of soil organisms of different body sizes from plant litter has previously been used successfully to draw conclusions about how subsets of the invertebrate community belonging to different size classes influence decomposition (Vossbrinck et al., 1979; Setälä et al., 1996; Bradford et al., 2002). As litterbag mesh size is increased, the assemblages of invertebrates that establish within the litterbags increase in maximum organism body size, species richness (Bradford et al., 2002; Cole et al., 2006), abundance and diversity of secondary and tertiary consumers, and functional complexity (Setälä et al., 1996; Bradford et al., 2002; Smith and Bradford, 2003). A second field experiment was carried out to test effects of ALAN alone on the trophic composition of soil arthropods and plant litter breakdown, while controlling for potential effects of ALAN on vegetation (Bennie et al., 2016, 2018b; Grenis and Murphy, 2019), which could potentially affect invertebrate assemblages or litter decomposition. We manipulated the presence/absence of ALAN (as above), while controlling for potential effects of ALAN on above-ground vegetation by mowing all plots prior to the experiment. To characterize effects of ALAN alone on invertebrate trophic structure, we used litterbags of a single large mesh size. Based on previous findings documenting higher abundances of ground-dwelling predatory invertebrates under ALAN compared with unlit areas (e.g., Davies et al., 2012, 2017), and that tertiary consumers can slow decomposition (Kajak, 1995; Lawrence and Wise, 2000; Hawlena et al., 2012), we predicted that ALAN would reduce rates of litter breakdown indirectly via increased top-down control of primary consumers by secondary and/or tertiary consumers.
Materials and Methods
Experimental Design
We conducted two experiments at Blandy Experimental Farm (BEF) in Boyce, Virginia, United States (39.0640°N, 78.0652°W). In the fall of 2017, we conducted an experiment to test the independent and interacting effects of ALAN and invertebrate size classes on decomposition of plant litter. The experiment was carried out in eight replicate plots, half exposed to ALAN and half receiving no added ALAN. Within each plot, we measured the decomposition of litter within mesh bags (litterbags), using litterbags with three different mesh sizes (0.1, 2, and 4 mm) to manipulate the size classes of fauna that could gain access to the litter. In the fall of 2018, we carried out a second experiment to for two primary reasons: (1) to control for potential effects of ALAN on vegetation (Bennie et al., 2016, 2018b; Grenis and Murphy, 2019), which could potentially affect invertebrate assemblages or litter decomposition, and (2) to test effects of ALAN on the trophic composition of soil arthropods. This experiment was carried out in ten replicate plots, five with ALAN added and five with no ALAN added. To control for potential effects of ALAN on vegetation, we minimized differences in vegetation height and biomass by mowing all plots prior to the start of the experiment. To examine the effects of ALAN on arthropod trophic composition, we deployed litterbags in the second experiment that would allow us to recover larger numbers of arthropods than we could in the previous experiment. This was accomplished by using litterbags that were larger, contained more litter, and had only the largest mesh size (4 mm).
Experiment 1: Untangling Effects of Artificial Light at Night and Invertebrate Size Classes on Decomposition
This experiment was carried out in the Native Plant Meadow at BEF. Dominant vegetation in the meadow consisted of warm season, C4 grasses including switchgrass (Panicum virgatum), Indian grass (Sorghastrum nutans), and big bluestem (Andropogon gerardii). Controlled burning is the primary management practice used to prevent succession of the meadow from grassland to forest. The most recent controlled burn prior to the experiment was carried out in the spring of 2017.
The experiment was carried out in eight 20-m diameter circular plots arranged in a paired design. Within each of four pairs of plots, one plot was randomly assigned to receive artificial light at night (hereafter ALAN plot). The other plot received no addition of ALAN, and thus only was lit with ambient diurnal sunlight, moonlight, and starlight (hereafter ambient-light plot). There were no barriers to restrict movement of invertebrates into, or out of, a plot. Within a pair, plots had a minimum distance of 10-m between edges, and pairs were a minimum of 20-m apart. Each plot represented a replicate. Each ALAN plot was illuminated from dusk to dawn by four broad-spectrum (4922 K) 12 W LED (Bullet®, RAB Lighting Inc., Northvale, New Jersey, United States) floodlights. The emission spectrum of this LED model is provided in the electronic (Supplementary Figure 1). Each LED floodlight was attached to the top of a 3 m post in the center of the plot. All floodlights were aimed downward, with a slight deflection of 25° outward toward the edge of the plot. To hold physical structure constant across treatments, we installed identical posts without floodlights at the center of ambient-light plots. The plots were originally established in 2015 (Firebaugh and Haynes, 2016), and ALAN was manipulated throughout the summers of 2015 and 2016. For this study, ALAN was manipulated continuously beginning in the spring (April) through the fall including the study period, which ran from August through October 2017.
At the end of the growing season (August 17, 2017), we placed mesh bags (litterbags) containing litter within each plot. The litterbags were placed 1 m from the central post. At this distance from the central post, we measured nighttime light intensity 1 m above the ground to minimize the blocking of light by the vegetation canopy, recording intensities of 193.16 ± 5.0 lux (mean ± 1 SD) in the ALAN plots and 0.014 ± 0.012 lux in the ambient-light plots. The light levels in our plots were well within the range for canopy level Illuminance reported by Bennie et al. (2016) which extends from 30 to 1200 lux depending on vertical distance from the light source in question. Lower values have been reported elsewhere in the literature (Bennie et al., 2016; Grenis and Murphy, 2019), and we assume that the light levels reaching the litter layer were significantly lower and more variable than at 1 m above the ground.
We set out litterbags in groups of three, with one bag of each of the three mesh sizes per group. Within a group, we arranged litterbags so that none overlaid the other, and all were staked down to maintain contact with the soil surface. We placed six groups in each plot, with the direction of each group relative to the central pole chosen haphazardly.
To obtain standardized litter for the experiment, we grew switchgrass, Panicum virgatum, in a greenhouse at BEF during the summer of 2017. For a detailed description of the methods of grass propagation and growth (see Supplementary Material Methods). We harvested green leaf material on August 12, 2017, by trimming blades to the collar, and then oven dried it at 40°C for ∼72 h. Prior to placing the leaf material into the litterbags, all leaf material was intermixed to maximize homogeneity among samples. We placed 0.72 ± 0.25 g of dried leaf material into each litterbag (W × L, 9 × 9 cm). The edges of the bags were sealed using a heat sealer.
To explore which invertebrates mediated ALAN-induced changes in decomposition, we manipulated the size classes of fauna that could gain access to the litter by using litterbags with three different mesh sizes: 0.1, 2, and 4 mm. These mesh sizes exclude (in order) all macrofauna and mesofauna, all macrofauna, and some macrofauna (Setälä et al., 1996; Bradford et al., 2002; Smith and Bradford, 2003). Litter-inhabiting organisms with body sizes < 0.1 mm include bacteria, fungi, protozoa, and nematoda (Wallwork, 1970; Swift et al., 1979; Wall and Moore, 1999); these organisms directly (and indirectly in the case of protozoa and nematoda) effectuate nutrient cycling (McGuire and Treseder, 2010). Mesofauna (body size between 0.1 and 2 mm, Wallwork, 1970; Swift et al., 1979) include (but are not limited to) Collembolans (springtails), Acari (mites), Isoptera (termites) in addition to larval organisms (Wall and Moore, 1999; Cole et al., 2006). Mesofauna are responsible for modification of the microbial community, comminution of litter, and in some instances predation of other invertebrates (Swift et al., 1979; Vossbrinck et al., 1979; Scheu and Setälä, 2002). Macrofauna (body size > 2 mm, Wallwork, 1970; Swift et al., 1979) include Araneae, Hymenoptera, and Coleoptera (larvae and adults), along with larvae and nymphs from other orders. Litter-dwelling macrofauna are responsible for comminution of litter and predation of secondary and tertiary invertebrates (Scheu and Setälä, 2002; Briones, 2014).
We expected that primary and secondary consumer microorganisms would gain access into the litterbags with the 0.1 mm mesh, but that arthropod tertiary consumers, along with arthropod secondary consumers whose body sizes exceeded 0.1 mm in diameter, would be excluded (Wallwork, 1970; Swift et al., 1979). We expected that primary, secondary, and tertiary consumers would all gain access into the litterbags with the 2 and 4 mm mesh sizes, but that fewer tertiary consumers would be excluded from the 4-mm mesh size litterbags than from the 2-mm mesh size litterbags (Wallwork, 1970; Swift et al., 1979; Bradford et al., 2002; Cole et al., 2006).
A small but growing body of literature highlights some of the effects of ALAN on plants in roadside or semi-natural conditions. ALAN from street-lighting has direct and indirect effects on the plant community and its herbivores (Bennie et al., 2018a, b; Grenis and Murphy, 2019). We therefore considered it plausible that ALAN-induced changes in plant biomass could affect the rate of plant litter decomposition. For example, increased density of standing senesced vegetation could attract detritivores or affect the litter-layer microclimate. To test for potential effects of ALAN in our experimental plots on plant growth, we estimated the density of aboveground grass biomass (g/m2) in each plot from the mean biomass harvested from five 0.3-m2 quadrats placed randomly within each plot. The biomass was harvested in early August, 2017, dried for 5 days at 55°C, and subsequently weighed.
Experiment 2: Effects of Artificial Light at Night on Decomposition and Litter-Layer Invertebrates While Controlling Aboveground Vegetation
Because ALAN can affect vegetation (Bennie et al., 2016, 2018b; Grenis and Murphy, 2019), which could potentially affect invertebrate assemblages or litter decomposition, we carried out a second field experiment in the fall of 2018 in a different set of plots at BEF. Like in the first experiment (carried out in 2017), we measured rates of plant litter breakdown in litterbags; however, in the second experiment we took steps to minimize differences across plots in the structure and biomass of aboveground vegetation via two means. First, the plots used in the 2018 experiment were not exposed to ALAN during the 2018 growing season; in the prior experiment, ALAN was manipulated throughout the growing season as well as during the fall decomposition experiment. In 2018, ALAN was not manipulated until August 16, 2 days before litterbags were placed in the plots. Second, we mowed all plots 2 days prior to the start of the 2018 experiment to further minimize differences in aboveground vegetation structure and biomass across plots.
This experiment was carried out in ten 1-m diameter field plots that were arrayed in a grid pattern with 5 m between adjacent plots. Half of the plots were selected at random to receive ALAN, while the other half received ambient light only. As with our first study, each plot represented a replicate. A plot was exposed to ALAN by one broad-spectrum 12 W LED (same model as in Experiment 1) floodlight, which was positioned on the underside of the horizontal arm of a light post at a height of 3 m and aimed directly downward over the center of the plot. Identical light posts were established for all plots (both ALAN-plots and ambient-light plots). There were no barriers to prevent movement in or out of plots. In this experiment, we were able to measure nighttime light intensity at ground level (the top of the litter layer) because the vegetation was mowed. Nighttime light intensity in the ALAN plots was 126.8 ± 7.32 lux (mean ± 1 SD) and 0.4 ± 0.21 lux in the ambient-light plots.
In this experiment, we intended to use plant litter that closely resembled litter occurring in the grasslands at our study site. Thus, we collected senesced leaves in August 2018 from standing C4 grasses in the BEF Native Plant Meadow (> 50 m from sources of ALAN). The nitrogen content of the collected leaf litter was 1.02 ± 0.33 (% of total mass mean ± SD). The leaf litter was dried at 50°C for 5 days, homogenized, and then placed into litterbags.
To increase our ability to characterize effects of ALAN on invertebrate trophic structure, we took two steps to increase the numbers of invertebrates captured. First, we used a mesh size (4 mm) that excluded only large macrofauna. Second, we used larger litterbags (W × L, 10 × 20 cm) containing more litter material (3.0 ± 0.05 g) than in the 2017 experiment (0.72 ± 0.25 g). The edges of the bags were sealed using a heat sealer, and all litterbags were staked down to maintain contact with the ground surface when deployed in the plots.
Data Collection and Analysis
Experiment 1: Untangling Effects of Artificial Light at Night and Invertebrate Size Classes on Decomposition
To examine the effects of ALAN on litter breakdown over time, we retrieved half of the litterbags from each plot after 31 days, and the remaining half after 61 days. Immediately after retrieval, we then removed invertebrates from the litterbags using Tulgren extraction carried out over 24 h. Following extraction of the invertebrates, we dried the litter at 50°C for 24 h and removed any residual soil or debris by hand. We estimated the proportion of litter broken down, or decomposed, as (1–massfinal/massinitial). We then pulverized the litter samples into fine powder using a ball mill (Cianflone Scientific LLC, Pittsburgh, PA, United States) and performed combustion analysis to determine final nitrogen content (Flash 2000 Elemental Analyzer, Thermo Fisher ScientificTM, Hampton NH, United States). To obtain initial litter nitrogen content, 0.3 g sub-samples of the litter placed into each litterbag were collected for combustion analysis.
Statistical analyses were run using the mean of response variables because plots were considered true replicates in our study. We tested the effects of ALAN and litterbag mesh size on the mean proportion of litter broken down and the nitrogen content of remaining litter within each plot using linear mixed effects (LME) models. The fixed effects in the LME models were ALAN, mesh size, their interaction, time in the field, and aboveground grass biomass. The random effects of plot pairs were modeled using random intercepts. Mean change in litter nitrogen was transformed using a power transformation (x3) to improve normality of residuals (Williamson and Gaston, 1999). The LME models were fitted using the “lmer” package (Bates et al., 2015) implemented in the program R (R Core Team, 2018). Post hoc pairwise comparisons based on least-squares means with p-values adjusted using the Tukey method were carried out using the R package “emmeans” (Lenth et al., 2019). In this experiment, we did not recover a sufficient number of invertebrates from the litterbags to explore how their abundances were affected by the experimental manipulations.
Experiment 2: Effects of Artificial Light at Night on Decomposition and Litter-Layer Invertebrates While Controlling Aboveground Vegetation
We deployed six litterbags in each of the plots on August 18, 2018, and collected them 116 days later. Upon retrieval from the field, invertebrates were extracted following the method used in Experiment 1. We identified invertebrates to order or family level, whichever was needed to determine their trophic position (primary consumers, secondary consumers, or secondary/tertiary consumers). We estimated the proportion of litter decomposed and nitrogen content using the same procedures described in Experiment 1.
We tested the effects of ALAN on the mean proportion of litter broken down (1 – massfinal/massinitial) and mean nitrogen content of remaining litter using one-way ANOVAs. We examined the effect of ALAN on the ground-dwelling invertebrate community in two ways. First, we tested for the effect of ALAN on the mean total number of invertebrates identified using a one-way ANOVA. Total number of invertebrates was log(x + 1) transformed to reduce heterogeneity of variance. Second, we investigated the effect of ALAN on the three trophic groups present in our invertebrate community: primary consumers, secondary consumers, and secondary/tertiary consumers. Because of non-independence of potentially interacting trophic levels, we tested for a multivariate effect of ALAN on abundances of the three trophic groups using MANOVA. In the event of a significant multivariate effect, we tested the effects of ALAN on each of the trophic groups using univariate ANOVA (Quinn and Keough, 2002). Prior to these tests, the abundances of each trophic group were Box-Cox transformed to improve normality (Olivier and Norberg, 2010). We ran this test using the “manova” function in the “stats” package.
Given the proximity of the experimental plots (5 m between adjacent plots), it is possible that some litter-layer invertebrates may have visited litterbags in more than one experimental plot. This could potentially cause non-independence of the measures of invertebrate abundance from different plots. Through trophic interactions, non-independence in invertebrate abundance could translate into non-independence in measures of litter breakdown. We addressed these possibilities by testing for spatial autocorrelation in the residuals from the MANOVA and each ANOVA model (Ver Hoef and Cressie, 1993). Moran’s I tests implemented in the “ape” package (Paradis and Schliep, 2019) showed there was no significant spatial autocorrelation in the residuals from any of the models.
Results
Untangling Effects of Artificial Light at Night and Invertebrate Size Classes on Decomposition
Mean daily precipitation during this experiment (August 17to October 17) was 3.18 mm. This is 11% higher than mean daily precipitation for this range of dates over the previous 30 years (1986–2016) at our study site.
We observed a marginally significant interactive effect of ALAN and mesh size on the proportion of litter broken down (P = 0.06, Table 1), potentially reflecting a stronger effect of ALAN on the proportion of litter broken down or removed in litterbags with the largest mesh size than with the intermediate and smallest mesh sizes (14, 8, and 6% higher under ALAN than under ambient light after 60 days, respectively, Figure 1). The proportion of material broken down differed significantly between litterbag mesh sizes (p < 0.001, Table 1), with the least loss of litter with the mesh size that excluded all but microorganisms (smallest mesh size) and the most breakdown in the mesh that excluded only large macrofauna (largest mesh size; Figure 1). After 60 days, there was a 20% difference in proportion broken down in the largest mesh size compared with the smallest mesh size. The proportion of leaf litter broken down tended to be higher under ALAN than under ambient light; however, this difference was not statistically significant (P = 0.07, Table 1 and Figure 1). There was no significant effect of aboveground grass biomass on the proportion of litter that broke down (P = 0.14, Table 1).
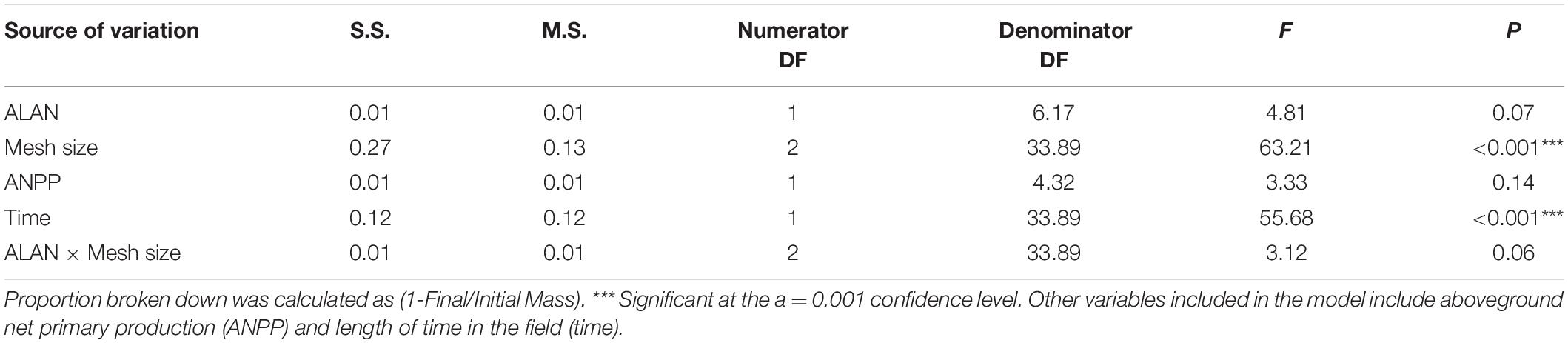
Table 1. Results of a linear-mixed-effects model to investigate the interactive effects of artificial light at night (ALAN) and litterbag mesh size on litter breakdown.
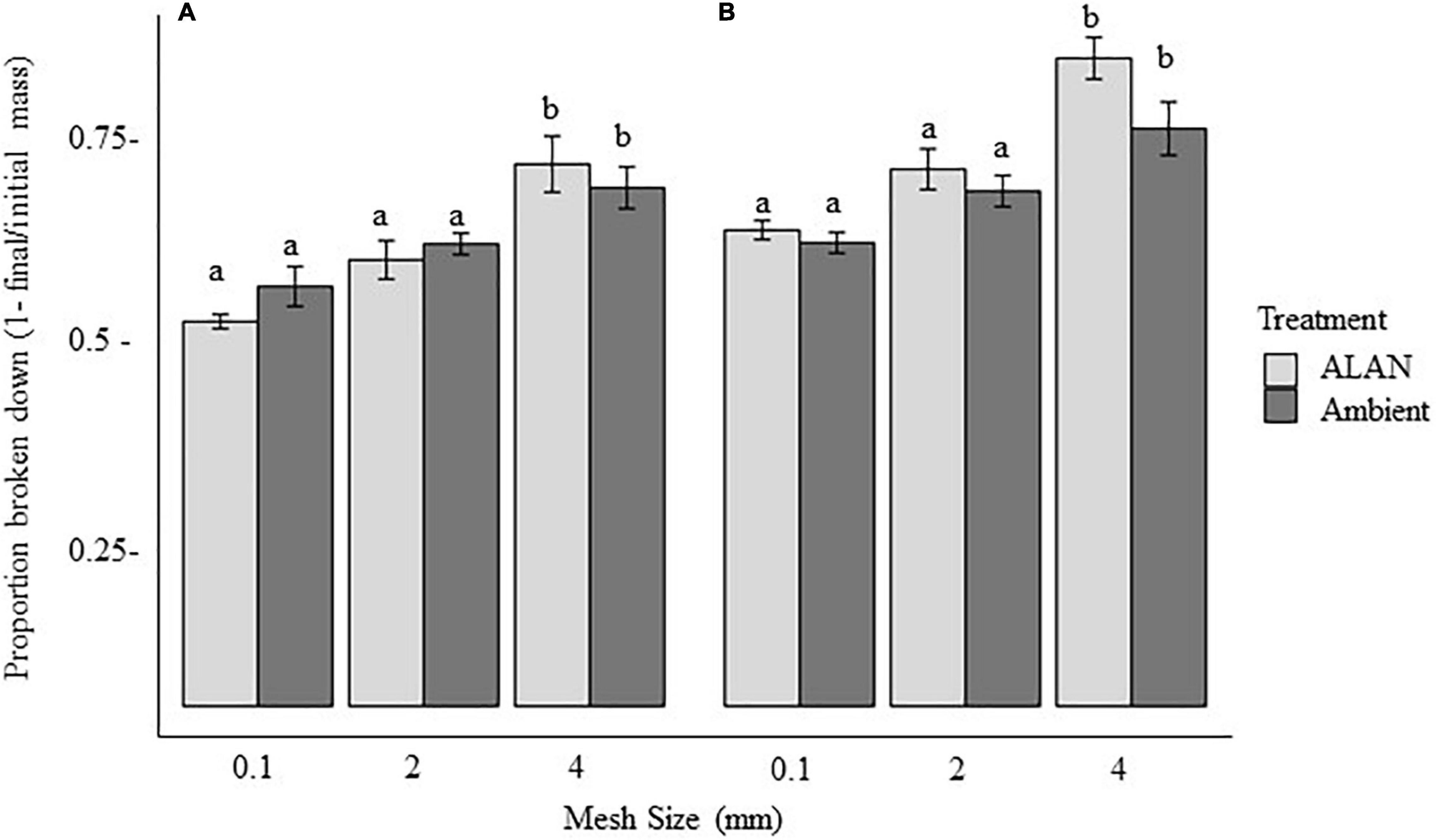
Figure 1. Effect of litterbag mesh size and artificial light at night (ALAN) on the proportion of leaf litter lost (mean ± SE) after (A) 30 days or (B) 60 days in the field. Means significantly different (within the 30- or 60-day exposure times) are marked by different letters (P < 0.05, based on Tukey least-squares means comparisons).
Mean initial nitrogen content the litter for this experiment was 3.36 ± 0.32 (mean ± SE, % of total mass) and mean nitrogen content of the litter remaining after 30 and 60 days was 3.25 ± 0.46%. We found no evidence that the nitrogen content of the remaining litter was affected by any of our experimental factors (Table 2 and Figure 2).
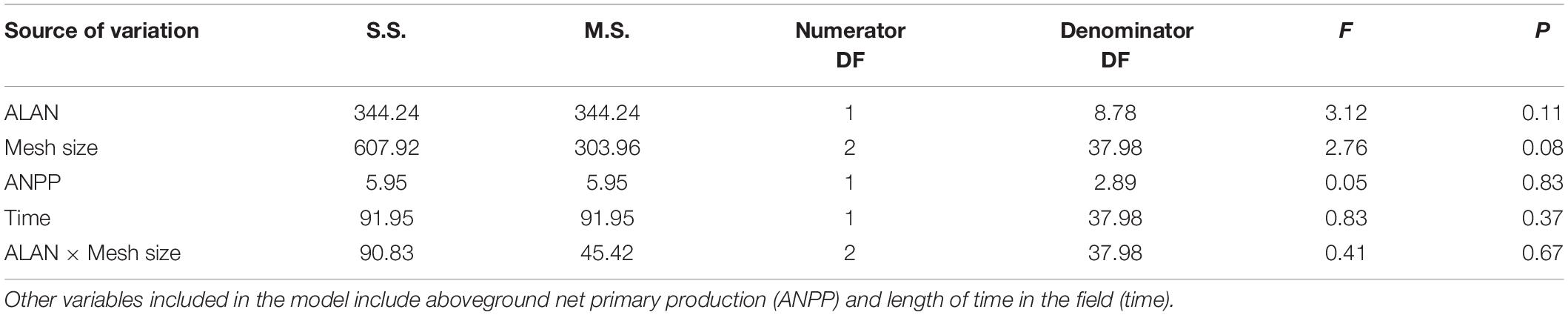
Table 2. Results of a linear-mixed-effects model to investigate the interactive effects of artificial light at night (ALAN) and litterbag mesh size on remaining nitrogen (%) in litter.
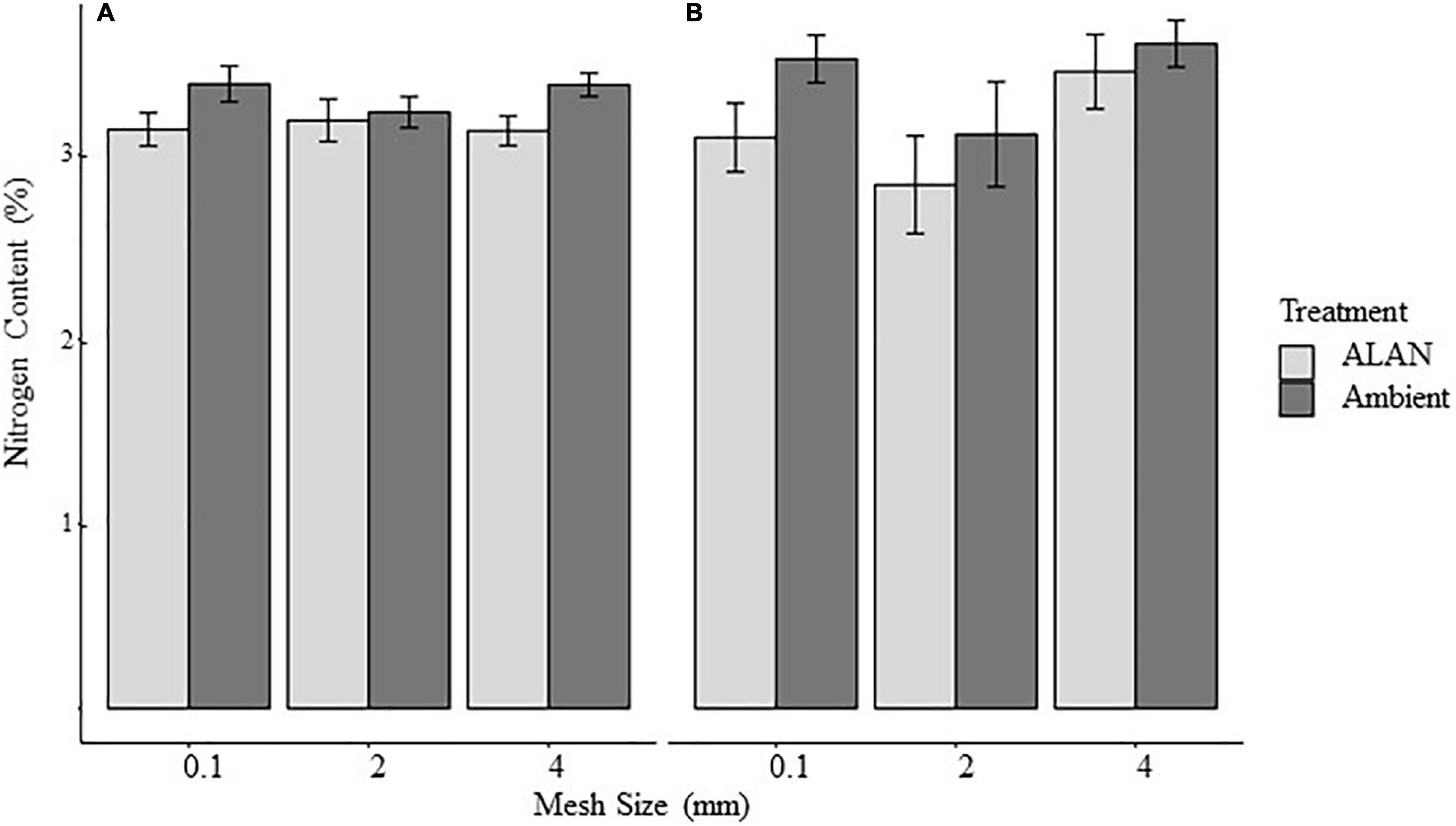
Figure 2. Effect of litterbag mesh size and artificial light at night (ALAN) on the nitrogen content of litter retrieved from the field after (A) 30 days or (B) 60 days. Nitrogen is expressed as percent of tissue (mean ± SE).
Experiment 2: Effects of Artificial Light at Night on Decomposition and Litter-Layer Invertebrates While Controlling Aboveground Vegetation
During the 120-day period of this experiment, mean daily rainfall was 4.75 mm. This is approximately 78% greater than during the previous 30 years.
In the second experiment, we did not observe an effect of ALAN on the proportion of leaf litter that broke down after 120 days [F(1, 8) = 0.648, P = 0.44]. The nitrogen content of the remaining litter was also not significantly affected by ALAN [F(1, 8) = 1.544, P = 0.249].
We recovered and identified 348 invertebrates belonging to 7 orders from the experimental litterbags (Supplementary Figure 2 and Supplementary Table 1). On average there were 65% more invertebrates in litterbags exposed to ALAN compared to ambient light [F(1, 8) = 12.33, P = 0.008, Figure 3]. There was a significant multivariate effect of ALAN on the abundances of primary, secondary, and secondary/tertiary consumers (Pillai’s trace = 0.75, F = 6.025, P = 0.031). Litterbags exposed to ALAN contained 4.6 times more secondary consumers [F(1, 8) = 6.688, P = 0.032] and 3.5 times as many secondary/tertiary consumers [F(1, 8 = 5.563, P = 0.045] than litterbags exposed only to ambient light. In contrast, there was no significant effect of ALAN on the abundance of primary consumers [F(1, 8) = 0.134, P = 0.724].
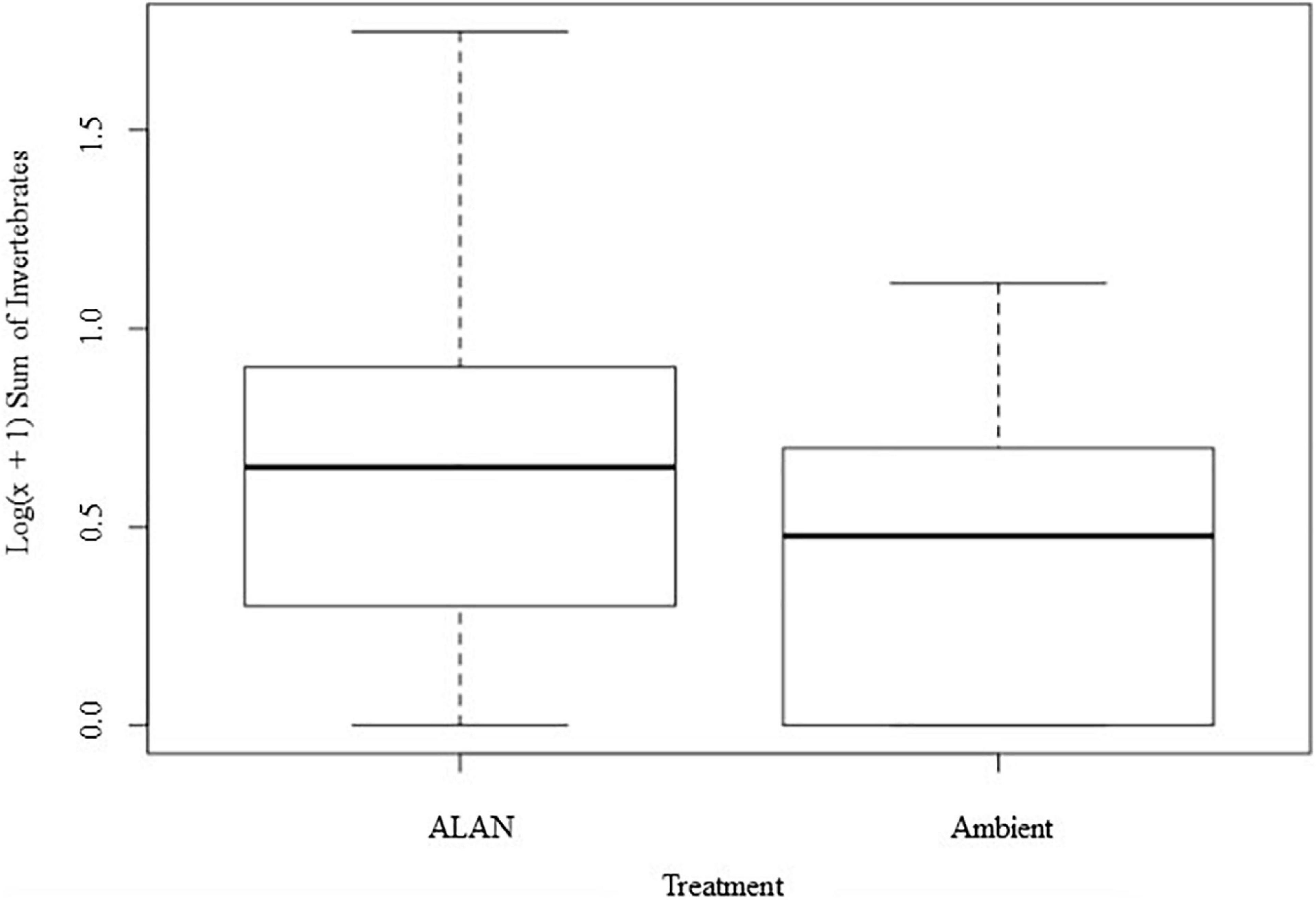
Figure 3. Effect of artificial light at night (ALAN) on log-transformed number of invertebrates recovered and identified from litterbags for each light treatment. Data are represented in a box and whisker plot indicating the median, interquartile range, and minimum and maximum values.
Discussion
To our knowledge, this is the first study to evaluate the effects of ALAN on the breakdown of plant litter in a terrestrial ecosystem. However, a recent study showed that ALAN-induced changes in microbial communities can impact the decomposition of plant litter in polluted streams (Pu et al., 2019). Consistent with prior research (Bradford et al., 2002), we found that the rate of breakdown of leaf litter within litterbags increased with mesh size (Table 1 and Figure 1). The phenomenon of greater litter breakdown with larger mesh sizes is thought to be caused by biotic factors including increased litter shredding and removal by detritivores as well as abiotic factors such as light, moisture, and temperature (Lecerf, 2017). We also observed that the proportion of leaf litter broken down was, on average, 11% higher under ALAN compared with ambient-lit plots in 2017 (although this effect was not significant, P = 0.07; Figure 1). The proportion of litter broken down was 10% higher, on average, after 60 days in 2017 than after 120 days in 2018. This is likely the result of the higher nutritional quality of the litter used in 2017 (3.36 ± 0.32%N) than in 2018 (1.02 ± 0.33%N). Litter of poor nutritional quality, or having lower nitrogen content, decomposes slowly compared to nutrient-rich litter, which is favored by detritivores (Smith and Bradford, 2003; Hessen et al., 2004). The lower amounts of breakdown in 2018 may have reduced our ability to detect potential effects of ALAN on litter breakdown in our second experiment. Lower foliar nitrogen has been reported for C4 plants (like those used in both of our experiments) compared with C3 plants (Sage and Pearcy, 1987). It is possible that ALAN may be more likely to affect the breakdown of nutrient-rich than nutrient-poor plant tissues, but further research is needed to resolve this question.
We detected greater abundances of invertebrates in litterbags exposed to ALAN compared with ambient light in our 2018 experiment (P = 0.008, Figure 3). Consistent with previous research on effects of ALAN on trophic structure within ground-dwelling arthropods (Davies et al., 2012, 2017), we found that ALAN increased the abundances of invertebrate secondary and/or tertiary consumers but had no effect on the abundance of primary consumers (Figure 4). Addition of ALAN may have slightly increased the rate at which plant litter decomposed (Table 1 and Figure 1); however, there was no significant effect of ALAN on litter breakdown (P = 0.07). Thus, our prediction that ALAN would reduce rates of litter breakdown indirectly via increased top-down control of primary consumers by secondary and/or tertiary consumers was not supported. This prediction may have been based on an oversimplified view of food-web dynamics in the litter layer. Greater local abundance of predators can sometimes lead to increased intraguild predation, releasing primary consumers from top-down control (Finke and Denno, 2005). This might explain why litterbags with larger mesh sizes tend to have higher abundance, diversity, and food web complexity of litter-dwelling fauna, but rates of plant litter decomposition tend to be high despite the presence of secondary and tertiary consumers (Swift et al., 1979; Vossbrinck et al., 1979; Bradford et al., 2002; Bokhorst and Wardle, 2013; Liu et al., 2019). Higher intraguild predation under ALAN could potentially explain the lack of an effect of ALAN on primary consumer abundance and litter decomposition in our study. However, experimental approaches that allow direct control over food web structure, combined with manipulations of ALAN, will likely be needed to fully understand how ALAN alters predator-prey interactions among soil fauna.
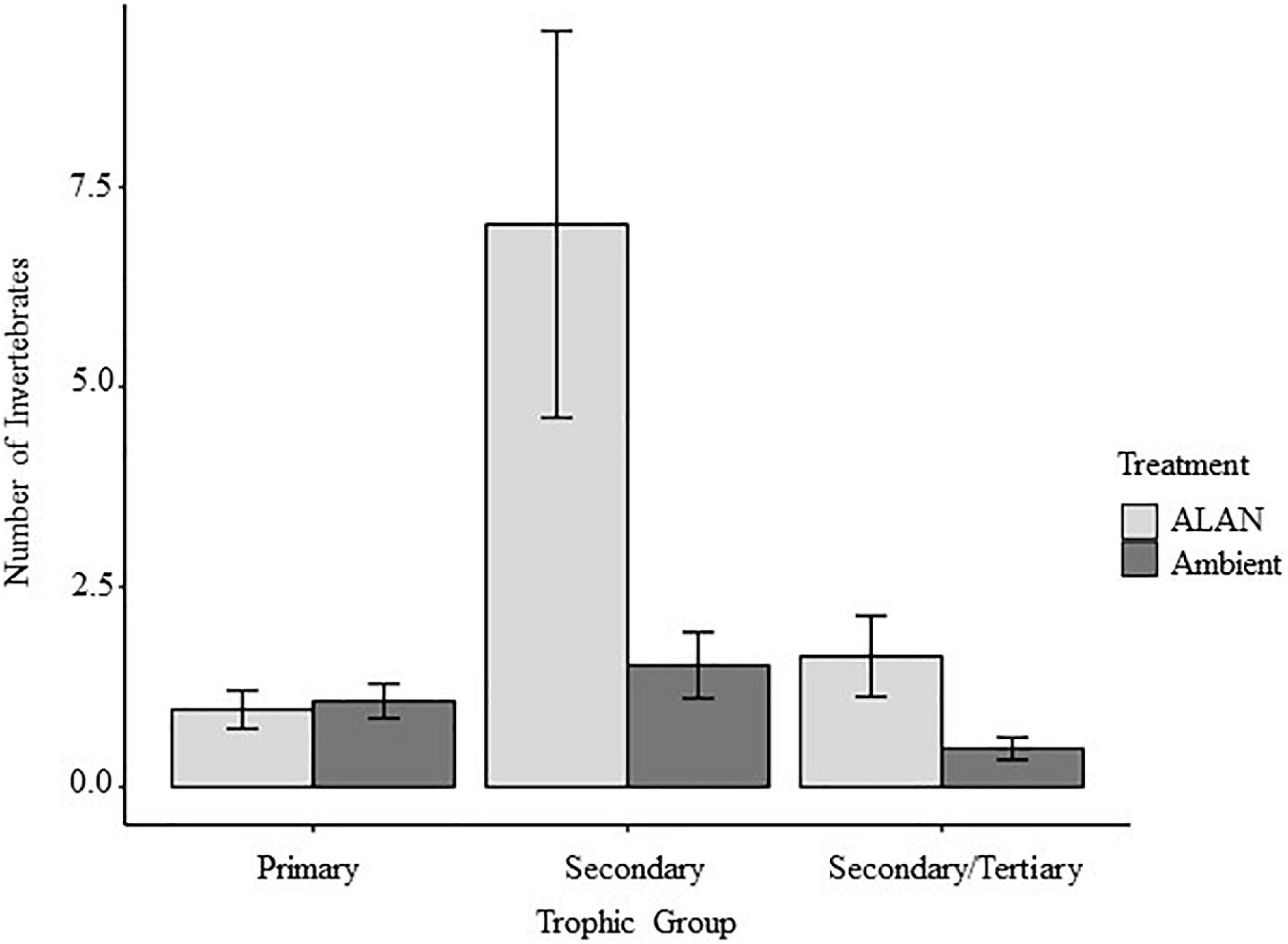
Figure 4. Effects artificial light at night (ALAN) on numbers of invertebrate primary consumers, secondary consumers, and secondary/tertiary consumers (mean ± SE) recovered from litterbags.
This work confirms the findings of others (Davies et al., 2012, 2017) that ALAN leads to higher local densities of ground-dwelling predaceous invertebrates. However, effects of ALAN on secondary and tertiary consumer invertebrates may differ by habitat type or depending on the taxa present. For example, Manfrin et al. (2017) found that, in a riparian environment, in contrast to the grassland ecosystem studied here, ALAN increased abundances of Araneae but decreased abundances of carabid beetles. Contrary to our prediction, we found that increased abundance of arthropod secondary/tertiary consumers under ALAN did not suppress the breakdown of plant litter. This indicates that detritivores in the litter layer may not be subjected to increased predation under ALAN. Further work is needed to elucidate the activities of predaceous ground-dwelling invertebrates under ALAN, for example by investigating potential increases in intraguild predation. In addition, trophic dynamics under point sources of ALAN takes place within a matrix of darker habitats. Although ALAN is known to influence the movement behaviors of many arthropod taxa (Degen et al., 2016; Manfrin et al., 2017; Duarte et al., 2019), little is known about how ALAN affects the net attraction of litter layer invertebrates. Determining how ALAN impacts net spatial fluxes of invertebrates of different trophic ranks will be critical for understanding the consequences of ALAN for ecosystem dynamics across landscapes.
Data Availability Statement
The raw data supporting the conclusions of this article will be made available by the authors, without undue reservation.
Author Contributions
MH, HE, and KH conceived, designed the experiments, revised, and edited the manuscript. MH performed the experiments and wrote the initial manuscript. MH and KH analyzed the data. All authors contributed to the article and approved the submitted version.
Funding
Financial support for this research was provided by a fellowship supported by the Foundation of the State Arboretum of Virginia.
Conflict of Interest
The authors declare that the research was conducted in the absence of any commercial or financial relationships that could be construed as a potential conflict of interest.
Publisher’s Note
All claims expressed in this article are solely those of the authors and do not necessarily represent those of their affiliated organizations, or those of the publisher, the editors and the reviewers. Any product that may be evaluated in this article, or claim that may be made by its manufacturer, is not guaranteed or endorsed by the publisher.
Acknowledgments
We would like to recognize the following individuals for their contributions to this work: Alex Oliver, Brandon Fox, Dennis Heflin, Aaron Mills, Meg Miller, Ariel Firebaugh, and the BEF Administration and Staff.
Supplementary Material
The Supplementary Material for this article can be found online at: https://www.frontiersin.org/articles/10.3389/fevo.2021.748983/full#supplementary-material
References
Bates, D., Mächler, M., Bolker, B., and Walker, S. (2015). Fitting linear mixed-effects models using lme4. J. Stat. Softw. 67, 1–48. doi: 10.18637/jss.v067.i01
Beare, M. H., Parmelee, R. W., Hendrix, P. F., Cheng, W., Coleman, D. C., and Crossley, D. A. Jr. (1992). Microbial and faunal interactions and effects on litter nitrogen and decomposition in agroecosystems. Ecol. Monogr. 62, 569–591. doi: 10.2307/2937317
Bennie, J., Davies, T. W., Cruse, D., and Gaston, K. J. (2016). Ecological effects of artificial light at night on wild plants. J. Ecol. 104, 611–620. doi: 10.1111/1365-2745.12551
Bennie, J., Davies, T. W., Cruse, D., Inger, R., and Gaston, K. J. (2018a). Artificial light at night causes top-down and bottom-up trophic effects on invertebrate populations. J. Appl. Ecol. 55, 2698–2706. doi: 10.1111/1365-2664.13240
Bennie, J., Davies, T. W., Cruse, D., Bell, F., and Gaston, K. J. (2018b). Artificial light at night alters grassland vegetation species composition and phenology. J. Appl. Ecol. 55, 442–450. doi: 10.1111/1365-2664.12927
Bokhorst, S., and Wardle, D. A. (2013). Microclimate within litter bags of different mesh size: implications for the “arthropod effect”on litter decomposition. Soil Biol. Biochem. 58, 147–152.
Bradford, M. A., Tordoff, G. M., Eggers, T., Hefin Jones, T., and Newington, J. E. (2002). Microbiota, fauna, and mesh size interactions in litter decomposition. Oikos 99, 317–323. doi: 10.1034/j.1600-0706.2002.990212.x
Briones, M. J. I. (2014). Soil fauna and soil functions: a jigsaw puzzle. Front. Environ. Sci. Eng. China 2:7. doi: 10.3389/fenvs.2014.00007
Cole, L., Bradford, M. A., Shaw, P. J. A., and Bardgett, R. D. (2006). The abundance, richness and functional role of soil meso- and macrofauna in temperate grassland—a case study. Appl. Soil Ecol. 33, 186–198. doi: 10.1016/j.apsoil.2005.11.003
Coleman, D. C., Crossley, D. A., and Hendrix, P. F. (2004). “Soil food webs: detritivory and microbivory in soils,” in Fundamentals of Soil Ecology, eds D. C. Coleman, D. A. Crossley, and P. F. Hendrix (Cambridge, MA: Academic Press), 227–246. doi: 10.1016/b978-012179726-3/50007-1
Davies, T. W., Bennie, J., Cruse, D., Blumgart, D., Inger, R., and Gaston, K. J. (2017). Multiple night-time light-emitting diode lighting strategies impact grassland invertebrate assemblages. Glob. Change Biol. 23, 2641–2648.
Davies, T. W., Bennie, J., and Gaston, K. J. (2012). Street lighting changes the composition of invertebrate communities. Biol. Lett. 8, 764–767. doi: 10.1098/rsbl.2012.0216
Degen, T., Mitesser, O., Perkin, E. K., Weiß, N.-S., Oehlert, M., Mattig, E., et al. (2016). Street lighting: sex-independent impacts on moth movement. J. Anim. Ecol. 85, 1352–1360. doi: 10.1111/1365-2656.12540
Desouhant, E., Gomes, E., Mondy, N., and Amat, I. (2019). Mechanistic, ecological, and evolutionary consequences of artificial light at night for insects: review and prospective. Entomol. Exp. Appl. 167, 37–58. doi: 10.1111/eea.12754
Duarte, C., Quintanilla-Ahumada, D., Anguita, C., Manríquez, P. H., Widdicombe, S., Pulgar, J., et al. (2019). Artificial light pollution at night (ALAN) disrupts the distribution and circadian rhythm of a sandy beach isopod. Environ. Pollut. 248, 565–573. doi: 10.1016/j.envpol.2019.02.037
Falchi, F., Cinzano, P., Duriscoe, D., Kyba, C. C. M., Elvidge, C. D., Baugh, K., et al. (2016). The new world atlas of artificial night sky brightness. Sci. Adv. 2:e1600377. doi: 10.1126/sciadv.1600377
Falchi, F., Furgoni, R., Gallaway, T. A., Rybnikova, N. A., Portnov, B. A., Baugh, K., et al. (2019). Light pollution in USA and Europe: the good, the bad and the ugly. J. Environ. Manage. 248:109227. doi: 10.1016/j.jenvman.2019.06.128
Finke, D. L., and Denno, R. F. (2005). Predator diversity and the functioning of ecosystems: the role of intraguild predation in dampening trophic cascades. Ecol. Lett. 8, 1299–1306. doi: 10.1111/j.1461-0248.2005.00832.x
Firebaugh, A., and Haynes, K. J. (2016). Experimental tests of light-pollution impacts on nocturnal insect courtship and dispersal. Oecologia 182, 1203–1211. doi: 10.1007/s00442-016-3723-1
García-Palacios, P., Ashley Shaw, E., Wall, D. H., and Hättenschwiler, S. (2016). Temporal dynamics of biotic and abiotic drivers of litter decomposition. Ecol. Lett. 19, 554–563. doi: 10.1111/ele.12590
Gaston, K. J., Bennie, J., Davies, T. W., and Hopkins, J. (2013). The ecological impacts of nighttime light pollution: a mechanistic appraisal. Biol. Rev. 88, 912–927. doi: 10.1111/brv.12036
Gaston, K. J., Duffy, J. P., Gaston, S., Bennie, J., and Davies, T. W. (2014). Human alteration of natural light cycles: causes and ecological consequences. Oecologia 176, 917–931. doi: 10.1007/s00442-014-3088-2
Gaston, K. J., Visser, M. E., and Hölker, F. (2015). The biological impacts of artificial light at night: the research challenge. Philos. Trans. R. Soc. Lond. B Biol. Sci. 370:20140133. doi: 10.1098/rstb.2014.0133
Giavi, S., Blösch, S., Schuster, G., and Knop, E. (2020). Artificial light at night can modify ecosystem functioning beyond the lit area. Sci. Rep. 10:11870. doi: 10.1038/s41598-020-68667-y
Grenis, K., and Murphy, S. M. (2019). Direct and indirect effects of light pollution on the performance of an herbivorous insect. Insect Sci. 26, 770–776. doi: 10.1111/1744-7917.12574
Hättenschwiler, S., Tiunov, A. V., and Scheu, S. (2005). Biodiversity and litter decomposition in terrestrial ecosystems. Annu. Rev. Ecol. Evol. Syst. 36, 191–218. doi: 10.1146/annurev.ecolsys.36.112904.151932
Hawlena, D., Strickland, M. S., Bradford, M. A., and Schmitz, O. J. (2012). Fear of predation slows plant-litter decomposition. Science 336, 1434–1438. doi: 10.1126/science.1220097
Hedlund, K., and Ohrn, M. S. (2000). Tritrophic interactions in a soil community enhance decomposition rates. Oikos 88, 585–591. doi: 10.1034/j.1600-0706.2000.880315.x
Heneghan, L., Coleman, D. C., Zou, X., Crossley, D. A., and Haines, B. L. (1998). Soil microarthropod community structure and litter decomposition dynamics: a study of tropical and temperate sites. Appl. Soil Ecol. 9, 33–38. doi: 10.1016/S0929-1393(98)00050-X
Hessen, D. O., Ågren, G. I., Anderson, T. R., Elser, J. J., and de Ruiter, P. C. (2004). Carbon sequestration in ecosystems: the role of stoichiometry. Ecology 85, 1179–1192. doi: 10.1890/02-0251
Hölker, F., Wolter, C., Perkin, E. K., and Tockner, K. (2010). Light pollution as a biodiversity threat. Trends Ecol. Evol. 25, 681–682. doi: 10.1016/j.tree.2010.09.007
Holzhauer, S. I. J., Franke, S., Kyba, C. C. M., Manfrin, A., Klenke, R., Voigt, C. C., et al. (2015). Out of the dark: establishing a large-scale field experiment to assess the effects of artificial light at night on species and food webs. Sustain. Sci. Pract. Policy 7, 15593–15616. doi: 10.3390/su71115593
Kajak, A. (1995). The role of soil predators in decomposition processes. Eur. J. Entomol. 92, 573–580.
Knop, E., Zoller, L., Ryser, R., Gerpe, C., Hörler, M., and Fontaine, C. (2017). Artificial light at night as a new threat to pollination. Nature 548, 206–209. doi: 10.1038/nature23288
Kyba, C. C. M., Kuester, T., de Miguel, A. S., Baugh, K., Jechow, A., Hölker, F., et al. (2017). Artificially lit surface of Earth at night increasing in radiance and extent. Sci. Adv. 3:e1701528. doi: 10.1126/sciadv.1701528
Lawrence, K. L., and Wise, D. H. (2000). Spider predation on forest-floor Collembola and evidence for indirect effects on decomposition. Pedobiologia 44, 33–39. doi: 10.1078/S0031-4056(04)70026-8
Lawrence, K. L., and Wise, D. H. (2004). Unexpected indirect effect of spiders on the rate of litter disappearance in a deciduous forest. Pedobiologia 48, 149–157. doi: 10.1016/j.pedobi.2003.11.001
Lecerf, A. (2017). Methods for estimating the effect of litterbag mesh size on decomposition. Ecol. Model. 362, 65–68. doi: 10.1016/j.ecolmodel.2017.08.011
Lenth, R., Singmann, H., Love, J., Buerkner, P., and Herve, M. (2019). Package ‘emmeans’: Estimated Marginal Means, Aka Least-Squares Means. Version 1.4. 3.01. CRAN.
Liu, Y., Wang, L., He, R., Chen, Y., Xu, Z., Tan, B., et al. (2019). Higher soil fauna abundance accelerates litter carbon release across an alpine forest-tundra ecotone. Sci. Rep. 9:10561. doi: 10.1038/s41598-019-47072-0
Longcore, T., and Rich, C. (2004). Ecological light pollution. Front. Ecol. Environ. 2, 191–198. doi: 10.1890/1540-92952004002[0191:ELP]2.0.CO;2
Macgregor, C. J., Evans, D. M., Fox, R., and Pocock, M. J. O. (2017). The dark side of street lighting: impacts on moths and evidence for the disruption of nocturnal pollen transport. Glob. Change Biol. 23, 697–707. doi: 10.1111/gcb.13371
Maggi, E., Bongiorni, L., Fontanini, D., Capocchi, A., Dal Bello, M., Giacomelli, A., et al. (2019). Artificial light at night erases positive interactions across trophic levels. Funct. Ecol. 895:201. doi: 10.1111/1365-2435.13485
Manfrin, A., Lehmann, D., van Grunsven, R. H. A., Larsen, S., Syväranta, J., Wharton, G., et al. (2018). Dietary changes in predators and scavengers in a nocturnally illuminated riparian ecosystem. Oikos 127, 960–969. doi: 10.1111/oik.04696
Manfrin, A., Singer, G., Larsen, S., Weiß, N., van Grunsven, R. H. A., Weiß, N.-S., et al. (2017). Artificial light at night affects organism flux across ecosystem boundaries and drives community structure in the recipient ecosystem. Front. Environ. Sci. Eng. China 5:61. doi: 10.3389/fenvs.2017.00061
McGuire, K. L., and Treseder, K. K. (2010). Microbial communities and their relevance for ecosystem models: decomposition as a case study. Soil Biol. Biochem. 42, 529–535. doi: 10.1016/j.soilbio.2009.11.016
McMunn, M. S., Yang, L. H., Ansalmo, A., Bucknam, K., Claret, M., Clay, C., et al. (2019). Artificial light increases local predator abundance, predation rates, and herbivory. Environ. Entomol. 48, 1331–1339.
Melguizo-Ruiz, N., Jiménez-Navarro, G., De Mas, E., Pato, J., Scheu, S., Austin, A. T., et al. (2020). Field exclusion of large soil predators impacts lower trophic levels and decreases leaf-litter decomposition in dry forests. J. Anim. Ecol. 89, 334–346. doi: 10.1111/1365-2656.13101
Meyer, L. A., and Sullivan, S. M. P. (2013). Bright lights, big city: influences of ecological light pollution on reciprocal stream–riparian invertebrate fluxes. Ecol. Appl. 23, 1322–1330. doi: 10.1890/12-2007.1
Moran, M. D., Rooney, T. P., and Hurd, L. E. (1996). Top-down cascade from a bitrophic predator in an old-field community. Ecology 77, 2219–2227. doi: 10.2307/2265715
Olivier, J., and Norberg, M. M. (2010). Positively skewed data: revisiting the box-cox power transformation. Int. J. Psychol. Res. 3, 68–95.
Paradis, E., and Schliep, K. (2019). ape 5.0: an environment for modern phylogenetics and evolutionary analyses in R. Bioinformatics 35, 526–528.
Pu, G., Zeng, D., Mo, L., He, W., Zhou, L., Huang, K., et al. (2019). Does artificial light at night change the impact of silver nanoparticles on microbial decomposers and leaf litter decomposition in streams? Environ. Sci. Nano 6, 1728–1739. doi: 10.1039/C9EN00081J
Quinn, G. P., and Keough, M. J. (2002). Experimental Design and Data Analysis for Biologists. Cambridge: Cambridge University Press.
R Core Team (2018). R: A Language and Environment for Statistical Computing. Vienna: R Foundation for Statistical Computing.
Ruess, L., and Ferris, H. (2004). Decomposition pathways and successional changes. Nematol. Monogr. Perspect. 2, 547–556.
Sage, R. F., and Pearcy, R. W. (1987). The nitrogen use efficiency of C3 and C4 plants: II. Leaf nitrogen effects on the gas exchange characteristics of Chenopodium album (L.) and Amaranthus retroflexus. Plant Physiol. 84, 959–963.
Sanders, D., and Gaston, K. J. (2018). How ecological communities respond to artificial light at night. J. Exp. Zool. A Ecol. Integr. Physiol. 329, 394–400. doi: 10.1002/jez.2157
Sanders, D., Kehoe, R., Cruse, D., van Veen, F. J. F., and Gaston, K. J. (2018). Low levels of artificial light at night strengthen top-down control in insect food web. Curr. Biol. 28, 2474–2478.e3. doi: 10.1016/j.cub.2018.05.078
Sanders, D., Kehoe, R., Tiley, K., Bennie, J., Cruse, D., Davies, T. W., et al. (2015). Artificial nighttime light changes aphid-parasitoid population dynamics. Sci. Rep. 5:15232. doi: 10.1038/srep15232
Santos, P. F., Phillips, J., and Whitford, W. G. (1981). The role of mites and nematodes in early stages of buried litter decomposition in a desert. Ecology 62, 664–669. doi: 10.2307/1937734
Scheu, S., and Setälä, H. (2002). “Multitrophic interactions in decomposer food,” in Multitrophic Level Interactions, eds T. Tscharntke and B. A. Hawkins (Cambridge: Cambridge University Press), 223.
Schmitz, O. J. (2009). Effects of predator functional diversity on grassland ecosystem function. Ecology 90, 2339–2345.
Schmitz, O. J., Hawlena, D., and Trussell, G. C. (2010). Predator control of ecosystem nutrient dynamics. Ecol. Lett. 13, 1199–1209. doi: 10.1111/j.1461-0248.2010.01511.x
Setälä, H., Marshall, V. G., and Trofymow, J. A. (1996). Influence of body size of soil fauna on litter decomposition and 15N uptake by poplar in a pot trial. Soil Biol. Biochem. 28, 1661–1675. doi: 10.1016/s0038-0717(96)00252-0
Smith, V. C., and Bradford, M. A. (2003). Litter quality impacts on grassland litter decomposition are differently dependent on soil fauna across time. Appl. Soil Ecol. 24, 197–203. doi: 10.1016/S0929-1393(03)00094-5
Sullivan, S. M. P., Hossler, K., and Meyer, L. A. (2019). Artificial lighting at night alters aquatic-riparian invertebrate food webs. Ecol. Appl. 29:e01821. doi: 10.1002/eap.1821
Swift, M. J., Heal, O. W., Anderson, J. M., and Anderson, J. M. (1979). Decomposition in Terrestrial Ecosystems. Berkeley, CA: University of California Press.
Tonin, A. M., Pozo, J., Monroy, S., Basaguren, A., Pérez, J., and Gonçalves, J. F. Jr., et al. (2018). Interactions between large and small detritivores influence how biodiversity impacts litter decomposition. J. Anim. Ecol. 87, 1465–1474. doi: 10.1111/1365-2656.12876
Ver Hoef, J. M., and Cressie, N. (1993). “Spatial statistics: analysis of field experiments,” in Design and Analysis of Ecological Experiments, eds S. M. Scheiner and J. Gurevitch (New York, NY: Chapman and Hall), 319–341.
Vossbrinck, C. R., Coleman, D. C., and Woolley, T. A. (1979). Abiotic and biotic factors in litter decomposition in a sermiarid grassland. Ecology 60, 265–271. doi: 10.2307/1937654
Wall, D. H., and Moore, J. C. (1999). Interactions underground: soil biodiversity, mutualism, and ecosystem processes. Bioscience 49, 109–117. doi: 10.2307/1313536
Wardle, D. A., Bardgett, R. D., Klironomos, J. N., Setälä, H., van der Putten, W. H., and Wall, D. H. (2004). Ecological linkages between aboveground and belowground biota. Science 304, 1629–1633. doi: 10.1126/science.1094875
Williamson, M., and Gaston, K. J. (1999). A simple transformation for sets of range sizes. Ecography 22, 674–680. doi: 10.1111/j.1600-0587.1999.tb00516.x
Willmott, N. J., Henneken, J., Elgar, M. A., and Jones, T. M. (2019). Guiding lights: foraging responses of juvenile nocturnal orb-web spiders to the presence of artificial light at night. Ethology 125, 289–297. doi: 10.1111/eth.12852
Keywords: light pollution, ecosystem function, trophic structure, trophic cascade, grassland
Citation: Hey MH, Epstein HE and Haynes KJ (2021) Artificial Light at Night Impacts the Litter Layer Invertebrate Community With No Cascading Effects on Litter Breakdown. Front. Ecol. Evol. 9:748983. doi: 10.3389/fevo.2021.748983
Received: 28 July 2021; Accepted: 30 September 2021;
Published: 20 October 2021.
Edited by:
Gail Lisa Patricelli, University of California, Davis, United StatesReviewed by:
Jeff Holmquist, University of California, Los Angeles, United StatesGina Marie Wimp, Georgetown University, United States
Copyright © 2021 Hey, Epstein and Haynes. This is an open-access article distributed under the terms of the Creative Commons Attribution License (CC BY). The use, distribution or reproduction in other forums is permitted, provided the original author(s) and the copyright owner(s) are credited and that the original publication in this journal is cited, in accordance with accepted academic practice. No use, distribution or reproduction is permitted which does not comply with these terms.
*Correspondence: Kyle J. Haynes, a2poOHdAdmlyZ2luaWEuZWR1