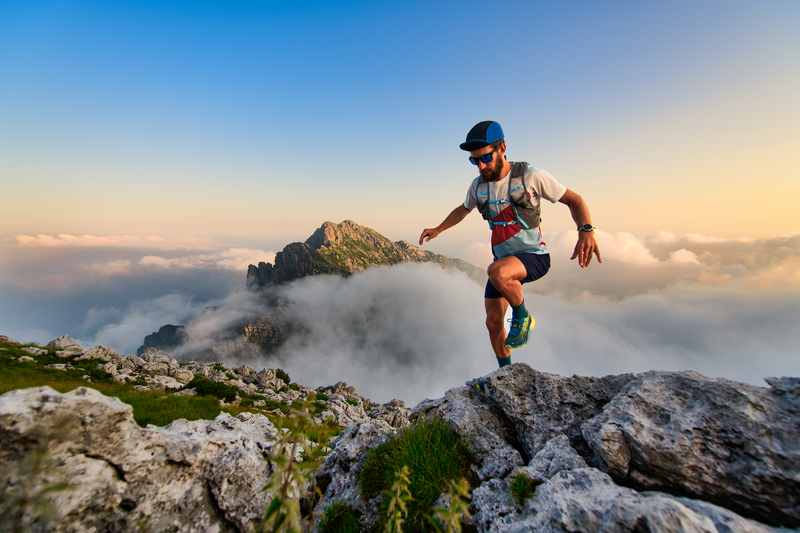
94% of researchers rate our articles as excellent or good
Learn more about the work of our research integrity team to safeguard the quality of each article we publish.
Find out more
REVIEW article
Front. Ecol. Evol. , 26 October 2021
Sec. Behavioral and Evolutionary Ecology
Volume 9 - 2021 | https://doi.org/10.3389/fevo.2021.742642
This article is part of the Research Topic Ecophysiological Adaptations Associated with Animal Migration View all 15 articles
Animals dynamically adjust their physiology and behavior to survive in changing environments, and seasonal migration is one life stage that demonstrates these dynamic adjustments. As birds migrate between breeding and wintering areas, they incur physiological demands that challenge their antioxidant system. Migrating birds presumably respond to these oxidative challenges by up-regulating protective endogenous systems or accumulating dietary antioxidants at stopover sites, although our understanding of the pre-migration preparations and mid-migration responses of birds to such oxidative challenges is as yet incomplete. Here we review evidence from field and captive-bird studies that address the following questions: (1) Do migratory birds build antioxidant capacity as they build fat stores in preparation for long flights? (2) Is oxidative damage an inevitable consequence of oxidative challenges such as flight, and, if so, how is the extent of damage affected by factors such as the response of the antioxidant system, the level of energetic challenge, and the availability of dietary antioxidants? (3) Do migratory birds ‘recover’ from the oxidative damage accrued during long-duration flights, and, if so, does the pace of this rebalancing of oxidative status depend on the quality of the stopover site? The answer to all these questions is a qualified ‘yes’ although ecological factors (e.g., diet and habitat quality, geographic barriers to migration, and weather) affect how the antioxidant system responds. Furthermore, the pace of this dynamic physiological response remains an open question, despite its potential importance for shaping outcomes on timescales ranging from single flights to migratory journeys. In sum, the antioxidant system of birds during migration is impressively dynamic and responsive to environmental conditions, and thus provides ample opportunities to study how the physiology of migratory birds responds to a changing and challenging world.
Mitochondria generate the cellular energy required by birds (and all animals) to fuel their basal metabolism and all other activity, including, for example, the impressive long-duration flights of migrating birds. As the cellular powerhouse, mitochondria are also the major site for generation of reactive species (RS), important molecules for signaling across the immune, inflammatory and antioxidant pathways (Dröge, 2002; Ristow et al., 2009; Sindler et al., 2013; McWilliams et al., 2020). However, at higher levels, RS can be deleterious by interacting with and stealing electrons from surrounding molecules (Halliwell and Gutteridge, 2007; Costantini, 2014). Thus, an effective antioxidant protection system has evolved to reduce or avoid the negative physiological effects of RS production, and the components of this system are shared by all eukaryotes (Cooper-Mullin and McWilliams, 2016; Skrip and McWilliams, 2016). Most pertinent to our review of how birds during migration maintain oxidative balance are several key aspects of animal physiology. First, increased metabolism, like that required for long-duration flapping flight, is associated with increased RS production that may overwhelm the antioxidant system, disrupt signaling, and cause oxidative imbalance or ‘stress’ and thus damage to lipids, proteins, and DNA in cells (Costantini, 2014; McWilliams et al., 2021). Second, the initiation and maintenance of an endogenous antioxidant response is likely energetically expensive (Costantini, 2014); thus, understanding how the antioxidant system of migrating birds contends with RS production is related to the energy costs of migration. Third, physiological demands change with the seasons, and thus understanding the pace and extent of these responses (i.e., phenotypic flexibility) is key to determining how birds contend with the challenges of migration in dynamic seasonal environments.
Birds have often been portrayed as exceptional vertebrates, given that they display relatively high metabolic rates and an associated increase in RS production, yet they are remarkably long-lived compared to mammals (Barja, 1998; Buttemer et al., 2010; Munshi-South and Wilkinson, 2010; Jimenez et al., 2019). Birds are also exceptional in that they primarily fuel high-intensity endurance exercise such as migratory flights by metabolizing fat (McWilliams et al., 2004; Jenni-Eiermann, 2017; Guglielmo, 2018) which has potential acute oxidative costs (Cooper-Mullin and McWilliams, 2016; Skrip and McWilliams, 2016). Fats, and especially polyunsaturated fats (PUFAs) that are common in birds during migration (Pierce and McWilliams, 2005, 2014; Guglielmo, 2018), are highly susceptible to oxidative damage (Hulbert et al., 2007; Montgomery et al., 2012; Cooper-Mullin and McWilliams, 2016; Skrip and McWilliams, 2016). The bird taxa that comprise 95% of extant species and which have the highest metabolic rates (Neoaves) all share a unique mutation in the major antioxidant regulation pathway that lowers the risk of macromolecular oxidative damage (Castiglione et al., 2020). Specifically, an ancestral mutation in the protein KEAP1, which suppresses the action of the transcription factor NRF2 (NF-E2-related factor 2), permits NRF2 to remain at elevated levels in the nucleus and activate expression of a host of antioxidant target genes (Castiglione et al., 2020). In vivo and in vitro experiments (Castiglione et al., 2020) demonstrate that this enhanced response lowers the risk of macromolecular oxidative damage and thus likely decreases oxidative stress. This higher baseline activation of the antioxidant response is potentially costly, although consumption of dietary antioxidants could reduce or alleviate the costs of upregulating the antioxidant response even further to contend with an oxidative challenge like migration.
In addition to the adapted master antioxidant response, birds rely on a multifaceted set of context-dependent regulatory mechanisms to quickly respond to the oxidative challenges associated with high-intensity flight fueled by oxidatively vulnerable fats. For example, they can upregulate endogenous antioxidant enzymes as well as synthesize endogenous sacrificial molecules (Cooper-Mullin and McWilliams, 2016; Skrip and McWilliams, 2016). They are also able to obtain exogenous antioxidants from their diet, which is especially pertinent for birds at stopover sites in the fall (Alan et al., 2013; Bolser et al., 2013). These various components of the antioxidant system (Figure 1) each have their own function and may interact in concert or independently depending on their location in the body, the type of RS generated, available antioxidant precursors or substrates, and exogenous resources.
Figure 1. During migration, a multitude of ecological and physiological factors that may be different between seasons (fall vs. spring migration) can alter a bird’s metabolic rate and subsequent reactive species (RS) production, as well as how the multifaceted antioxidant system responds to RS production during endurance flight (A) and during recovery from flight and preparations for the next flight while at a stopover site (B). The multifaceted antioxidant system consists of ingested dietary antioxidants, micromolecular sacrificial molecules (e.g., uric acid, a byproduct of protein catabolism), and endogenous enzymes that may respond to the production of RS produced primarily in mitochondria. Here, we layout some examples that likely underly observed variation in measurements of oxidative status during migratory flight and stopover. (A) The increase in metabolic rate during flight is influenced by ecological factors (e.g., distance between stopovers and barriers encountered during flight) and physiological factors (e.g., fat and protein and antioxidant stores, metabolic requirements including thermoregulation) that can affect RS production as well as antioxidant capacity and thus observed oxidative damage. (B) Habitat quality at a stopover site governs the resources available for refueling. For example, the amount and type of long-chain polyunsaturated fats (PUFA) or monounsaturated fats (MUFA) a bird consumes and stores during stopover can directly influence an individual’s RS production. Additionally, the amount and types of dietary antioxidants available (e.g., fruit abundance in the fall) influences an individual’s ability to rebuild antioxidant capacity at a stopover site. Thus, resources available at stopover sites (e.g., dietary antioxidants, fats, and proteins), as well as physiological factors (not shown), influence the rate and extent of recovery from the previous flight and preparations for the next flight.
One of the challenges of studying how the antioxidant system of birds responds to the physiological challenges of migration is that the primary instigator of the oxidative challenge, increased RS production with increased metabolism, is not yet measurable in whole organisms (Cooper-Mullin and McWilliams, 2016; Costantini, 2016, 2019). Rather, oxidative status must be inferred from measurements of key components of the antioxidant system (e.g., upregulation of antioxidant enzymes implies a response to increased RS production) and ideally simultaneous measures of RS-associated damage (e.g., an increase in lipid peroxidation products implies increased RS production that is insufficiently quenched by the antioxidant system). The primary ways in which RS are generated and how key components of the endogenous antioxidant system provide protection are shown in Figure 2. Another challenge of studying phenotypic flexibility in the antioxidant system of migratory birds is that the major site for generation of RS is the mitochondria, which will vary in density and activity across tissues (Costantini, 2019), as well as with age and exercise (Cooper-Mullin and McWilliams, 2016; Jimenez, 2018; Stier et al., 2019; Cooper-Mullin et al., 2021). In addition, RS can act as both damaging molecules and also as signaling molecules that can stimulate the endogenous antioxidant system and immune system (Halliwell and Gutteridge, 2007; Costantini, 2014, 2019; Cooper-Mullin and McWilliams, 2016). There are also a multitude of tests available to probe the response of the antioxidant system to ecologically relevant challenges, too often complicating comparisons across studies (Costantini, 2011, 2016, 2019; Skrip and McWilliams, 2016). Consequently, integrative studies (from mitochondria to cells to tissues to whole organism) that are also comparative (e.g., multiple tissues within the same individuals, migration-state vs. non-migration periods, multiple species that differ in migration strategy) are required to fully understand the antioxidant system of migratory birds within an ecological context. Given that such integrative and comparative studies of migratory birds are largely lacking, evidence to date provides a more piecemeal view of how the antioxidant system of migratory birds changes during migration.
Figure 2. Reactive species (RS) produced during metabolism can serve as beneficial signaling molecules although when in excess RS can cause damage to a variety of important molecules. The multifaceted antioxidant filter of birds can mitigate damage in several ways. The antioxidant filter consists of micromolecular sacrificial molecules, endogenous enzymes and dietary antioxidants. Uric acid is an example of a sacrificial molecule that donates an electron to RS and is oxidized to allantoin. The ratio of uric acid to allantoin can be measured and used as an indicator of oxidative balance. Glutathione peroxidase (GPx) is an example of an endogenously produced enzyme that catalyzes the glutathione (GSH) to glutathione disulfide (GSSG) cycle. During the reaction, GSH donates an electron to RS, and is oxidized to GSSG, which can be recycled back to GSH by glutathione reductase. Superoxide dismutases (SODs) and catalase (CAT) are two other important groups of endogenous antioxidant enzymes that operate in different cells and tissues to quench RS such as oxygen radicals. Vitamin E (α-tocopherol) is an example of a lipophilic antioxidant from the diet, and vitamin C (ascorbate) is an example of a hydrophilic antioxidant from the diet. As RS production increases during endurance flight, the antioxidant system must also be upregulated to prevent an increase in damage.
In this review, we focus on the extent to which birds during migration modulate their antioxidant capacity to protect against overwhelming oxidative damage caused by storing and burning fats during long-duration, energy-demanding flights (Skrip et al., 2015; Cooper-Mullin and McWilliams, 2016; Skrip and McWilliams, 2016). Other reviews have focused on the regulation of oxidative balance as an underlying driver of aging and longevity (Montgomery et al., 2012; Selman et al., 2012), as determining the sublethal effects of environmental contaminants (Bursian et al., 2017a; Dorr et al., 2019) including ingestion of industrial oil from oil spills (Bursian et al., 2017b; Pritsos et al., 2017), and as an important general indicator of bird health for field ornithologists (Cooper-Mullin and McWilliams, 2016; Hutton and McGraw, 2016; Skrip and McWilliams, 2016). Here, we review in turn the evidence from field and captive bird studies that address the following questions: (1) Do migratory birds build antioxidant capacity as they build fat stores in preparation for long flights? (2) Is oxidative damage an inevitable consequence of oxidative challenges such as flight, and, if so, how is the extent of damage affected by factors such as the response of the antioxidant system, the level of energetic challenge, and the availability of dietary antioxidants? (3) Do migratory birds ‘recover’ from the oxidative damage accrued during long-duration flights, and, if so, does the pace of this rebalancing of oxidative status depend on the quality of the stopover site? After reviewing the evidence to date, we provide some suggestions for future research that include much needed integrative and comparative studies.
The course of migration for most birds (Figure 3) involves an initial period of over-feeding (called hyperphagia) that enables birds to store appreciable energy, mostly as fat (90+% of body mass increase) as well as key nutrients including protein (King and Farner, 1965; Jenni and Jenni-Eiermann, 1998; McWilliams et al., 2004; Bishop and Butler, 2015; Guglielmo, 2018). These periods of intensive feeding and fattening are often associated with diet switching, most notably to fruits during fall migration (Rybczynski and Riker, 1981; Parrish, 1997; Smith et al., 2007, 2015; Carlisle et al., 2012). The duration of the intensive feeding and fattening periods can last a few days to several weeks (Schaub and Jenni, 2001; Chernetsov, 2006; O’Neal et al., 2018), and is influenced by a variety of ultimate and proximate factors such as migration strategy (e.g., long-distance and short-distance), weather, food availability, predation risk, endogenous cycles, and physiological state (Jenni and Schaub, 2003; Fusani et al., 2009; Goymann et al., 2010; Guillemette et al., 2012; Hou and Welch, 2016). Furthermore, migratory birds exhibit behavioral and physiological adjustments (e.g., reduced heart rate and body temperature, less active when not feeding) during hyperphagia that help to promote fattening by keeping their daily energy expenditure similar to or below non-hyperphagic periods (Carpenter and Hixon, 1988; Butler and Woakes, 2001; Wojciechowski and Pinshow, 2009; Guillemette et al., 2012). Birds during migration then alternate between fasting while flying and feeding and refueling upon arrival at migratory stopover sites in preparation for subsequent migratory flight(s). These rapid changes in feeding rate and food quality over short periods of time (i.e., hours to days) affect the structure and function of the digestive system in migratory birds (McWilliams et al., 2004; McWilliams and Karasov, 2014; Griego et al., 2021) and thus the dynamics of fat (and protein) metabolism and potentially the antioxidant system over the course of many flights and stopovers that constitute a typical migration in birds (Figure 3).
Figure 3. The time course of migration for birds typically involves a period of preparation in anticipation of migration, and then a series of flights while fasting alternating with periods of stopover, refeeding, and refueling along the way. (A) In preparation for migration, birds accumulate fat stores primarily by eating more (hyperphagia) and by carefully selecting their diets. Then during a given migration period, a typical migratory bird traveling between wintering and breeding areas must take several flights that are interspersed with layovers at stopover sites where the bird refuels in preparation for the next flight. Thus, birds during migration alternate between periods of high feeding rate at migratory stopover sites and periods without feeding as they travel between stopover sites. (B) Possible dynamics of the antioxidant (AO) system over the course of migration. This review outlines the evidence to date for such proposed changes in the antioxidant system of migratory birds.
Birds are also able to enhance their use of stored fats during migratory flights by changing the ‘quality’ of their fat stores to enhance the rate of mobilization and oxidation (McWilliams et al., 2021). Fatty acid composition of stored fat changes in birds during migration primarily in response to diet and to a lesser extent preferential metabolism of certain fatty acids (Pierce and McWilliams, 2005, 2014). Such changes in fat quality can affect flight performance during long-duration flights (Price, 2010; Carter et al., 2018; Guglielmo, 2018; McWilliams et al., 2020) as well as levels of oxidative damage (McWilliams et al., 2021). Several experimental and field studies suggest that the quality of fats consumed and stored by migratory birds affects circulating markers of oxidative damage. For example, European Starlings (Sturnus vulgaris) fed for many months on diets with more or less n − 6 PUFA (which then corresponded to the makeup of their fuel stores) exhibited significantly different levels of oxidative damage to lipids (plasma d-ROMs); specifically, birds composed of more n-6 PUFA had consistently higher damage (McWilliams et al., 2020). Similarly, captive White-throated Sparrows (Zonotrichia albicollis) that consumed diets with more PUFA had increased circulating oxidative damage (d-ROMs) (Alan and McWilliams, 2013). Common Blackbirds (Turdus merula) captured at a stopover site in northern Germany in autumn had a higher plasma fatty acid (FA) peroxidation index and higher non-enzymatic antioxidant capacity (Ferric Reducing Antioxidant Power, FRAP, and uric acid) but similar lipid peroxidation (malondialdehyde concentration, or MDA) compared to sympatric resident blackbirds (Eikenaar et al., 2017).
Given that fats, particularly PUFAs, are oxidatively vulnerable, fattening in preparation for migration might be expected to increase RS production and an associated antioxidant response. Evidence to date indicates that migrating birds prepare for the challenge of increased RS and that the response is condition-dependent. For example, amount of stored fat (as measured by either fat score or fat mass) was positively correlated with non-enzymatic antioxidant capacity (plasma OXY) in Blackpoll Warblers (Setophaga striata) and Red-eyed Vireos (Vireo olivaceus) at a New England stopover site during fall migration (Skrip et al., 2015), and Garden Warblers (Sylvia borin) at a Mediterranean coastal stopover site during spring migration (Costantini et al., 2007). However, no such correlation was found in Barn Swallows (Hirundo rustica) at the same Mediterranean coastal stopover site during spring migration (Costantini et al., 2007) nor in Northern Wheatear (Oenanthe oenanthe) at a stopover site on Helgoland in the North Sea during fall migration (Eikenaar et al., 2020a). Interestingly, stored fat was also positively correlated with oxidative damage (plasma d-ROMs), suggesting that fat stores and/or fattening may incur oxidative costs. Captive bird work further suggests that actively refueling birds prepare for oxidative challenges. Captive-reared Northern Wheatears that were photostimulated into ‘migration state’ in fall increased their non-enzymatic antioxidant capacity over time as compared to control (ad libitum-fed) birds. This increase in non-enzymatic antioxidant capacity was largely due to increases in uric acid, as indicated by highly correlated FRAP and uric acid assays (Eikenaar et al., 2016). However, the high variability in lipid peroxidation (red blood cell MDA) produced no consistent differences between control and migration-state birds (Eikenaar et al., 2016).
The studies we describe above have examined the oxidative status of songbirds that were either sampled during migration or held in captivity and photostimulated into migratory state. The only studies to date that directly compare the oxidative status of birds preparing for migration versus during other stages of their annual cycle (e.g., wintering) have been performed in a shorebird species, the Hudsonian Godwit (Limosa haemastica), and a migratory quail, the Common Quail (Coturnix coturnix). Gutiérrez et al. (2019) sampled blood from free-living godwits inhabiting an island in southern Chile at three time periods from January to March (wintering, fuelling, and pre-departure) and found that pre-departure birds preparing for their >10,000-km northbound migratory flight increased total antioxidant capacity (TAC) and reduced oxidative damage (TBARS), without changing enzyme activity (cytochrome c oxidase and citrate synthase). Marasco et al. (2021) compared oxidative status of captive quail during the migratory and non-migratory phases, the former phase induced using photoperiod manipulations that simulated autumn migration. In contrast to free-living godwits preparing for spring migration, the migratory-phase quail had higher levels of oxidative damage (TBARS) compared to non-migratory birds (in liver but not red blood cells or pectoral muscle), and no comparable measure of total antioxidant capacity was reported. In addition, whole-tissue enzyme assays revealed lower SOD activity in red blood cells and liver (but not pectoral muscle), and higher GPx activity in pectoral muscle (and sex-specific differences in red blood cells and liver). Marasco et al. (2021) suggested that the higher GPx activity and lower TBARS they found in muscles compared to liver of migratory-phase captive quail may indicate tissue-specific preferential protection, with pectoral muscle being “spared” from damage to support migratory flight. Determining whether the results from these two longitudinal studies are related to species-specific differences (e.g., life history and migration strategy), experimental conditions (e.g., captive vs. free-living birds), tissues sampled and assays used, or migratory season (i.e., autumn vs. vernal) requires many more such studies.
In sum, migratory birds seem to build some component of their antioxidant capacity concomitantly with fat stores (as seen in Blackpoll Warblers, Red-eyed Vireos, Garden Warblers, Barn Swallows, Northern Wheatears, Common Blackbirds, and Hudsonian Godwits). Furthermore, increased oxidative damage is a likely cost of increasing (or maintaining more) fat stores (as seen in Blackpoll Warblers, Red-eyed Vireos, Garden Warblers, Barn Swallows, and in liver of Common Quail), especially if these fats are composed of mostly PUFA (as seen in European Starlings and White-throated Sparrows). However, higher damage may not be inevitable as birds fatten prior to migration (as seen in Northern Wheatears, Common Blackbirds, Hudsonian Godwits), perhaps because different tissues, individual birds or bird species vary in the extent to which they build antioxidant capacity.
The metabolic costs of flying are long established (Bishop and Butler, 2015) as are the many physiological adjustments that enable the long-duration flights achieved by birds during migration, including increased oxidative enzymes (McClelland, 2004; Weber, 2011; Banerjee and Chaturvedi, 2016; Dick and Guglielmo, 2019; Carter et al., 2021) and fatty acid transport and oxidation (Guglielmo et al., 2002; McWilliams et al., 2004, 2021; Guglielmo, 2018), as well as hypertrophy of key organs such as pectoralis and liver (Marsh, 1984; Dietz et al., 1999; Piersma et al., 1999; Lindström et al., 2000; DeMoranville et al., 2019). These biochemical adjustments are associated with upregulation of genes responsible for regulating metabolism (PPARγ and PPARα), and key genes responsible for fat transport (FABPpm, CD36, and H-FABP) and fat oxidation (ATGL, LPL, and MCAD) among others (McFarlan et al., 2009; Zhang et al., 2015; Corder et al., 2016; DeMoranville et al., 2019). Furthermore, experimental studies demonstrate that long-distance flight training in a wind-tunnel upregulates genes involved in mitochondrial metabolism and fat utilization in pectoralis but not liver (DeMoranville et al., 2020). Below we review whether such metabolic upregulation is associated with oxidative challenges during a given flight and whether flight specifically results in oxidative damage and reduced antioxidant capacity measured immediately after.
Despite the importance of oxidative damage measures to understanding tradeoffs during migration, relatively few studies have measured the acute effects of flight on byproducts of RS production. Moreover, these studies have been spread across different study systems and measures of oxidative damage. As outlined below, the effects of acute flight on oxidative damage are more consistent in experimental studies, where flight time and speed are controlled, compared to field studies of free-living birds, and the extent of reported damage seems related to the extent of antioxidant system response. A commonly used measure of oxidative damage has been the d-ROMs test (Costantini, 2016; Skrip and McWilliams, 2016), which is most closely associated with lipid peroxidation but can reflect hydroperoxides of any origin (Davies, 2016; Ito et al., 2017). This assay is responsive to changes in oxidative status over a relatively short time scale (e.g., minutes to hours) and uses blood serum, making it both non-destructive and a more general indicator of circulating lipid peroxidation products than other more tissue-specific measures (e.g., in muscle; Abuja and Albertini, 2001; Colombini et al., 2016) and compared to other specific assays (e.g., MDA, see below).
Oxidative damage to lipids has usually been found to not change or has increased after flight across the ca. dozen studies to date, and this variation in response can be explained in part by the extent of response of the antioxidant system to the physiological challenges of the flight. In one of the first studies to investigate the acute effects of flight, pigeons (Columbia livia) flown for long durations (310 ± 31 min) had 54% higher lipid damage than in birds flown short durations (80 ± 15 min) or not flown (Costantini et al., 2008). In contrast, flight duration (range: 65–205 min) was not related to d-ROMs levels in Northern Bald Ibis on a managed migration (Geronticus eremita; Bairlein et al., 2015). Similarly, several wind-tunnel studies on starlings (S. vulgaris) that involved weeks of flight training leading to long final flights up to 6 h have not found any change in d-ROMs associated with flight, either within individuals pre- and post-flight or between individuals that were either flown or not flown (McWilliams et al., 2020; Frawley et al., 2021). In contrast, starlings involved in a similar 2-week wind-tunnel flight training decreased d-ROMs after flight compared to pre-flight indicating a potential hormetic response (DeMoranville, 2020). Zebra Finches (Taeniopygia guttata) that underwent 3 h of short-burst flight, a flight type that is more aerobically intense than sustained flight (Nudds and Bryant, 2001), showed a significantly greater increase in d-ROMs over those 3 h than unflown birds (Costantini et al., 2013). Furthermore, birds flown at the greatest speed intensity (0.9 km/h) had the highest levels of d-ROMs and birds flown at a slower speed (0.3 km/h) had intermediate levels (Costantini et al., 2013). Over a longer period of flight training, Zebra Finches flown for 2 h at 2.4 km/h per day consistently showed no acute changes in oxidative damage to lipids on a given day of flight training, and no difference in d-ROMs was detected between trained and untrained individuals over 1.5 months of daily flying (Cooper-Mullin et al., 2019). However, these same individuals exhibited a consistent increase in an antioxidant enzyme (GPx) that may have protected them against oxidative damage. Finally, correlational field studies of free-living, migrating Garden Warblers (S. borin; Skrip et al., 2015) and Nathusius’ bats (Pipistrellus nasthusii; Costantini et al., 2018) found that d-ROMs were highest in plasma samples taken most recently after migratory flight, while a study that manipulated the effort of flight by plucking wing feathers of Great Tits (Parus major; Vaugoyeau et al., 2015) found no difference in d-ROMs between handicapped and control individuals. Some of this inconsistency in results between studies seems related to the extent of flight costs and duration. Notably, the level of oxidative damage measured by the d-ROMs test in both European Starlings and Yellow-rumped Warblers (Setophaga coronata) flown for known durations in wind-tunnel experiments was highest in individuals flown for longer and that had higher energy expenditure during flight (Dick and Guglielmo, 2019; DeMoranville, 2020). Likewise, free-living Red-eyed Vireos had lower oxidative damage compared to Blackpoll Warblers caught on an island stopover site (Block Island and Rhode Island), presumably because the warblers had to fly farther to reach the stopover site (Skrip et al., 2015).
Given the susceptibility of PUFA to oxidative damage (Cooper-Mullin and McWilliams, 2016; Skrip and McWilliams, 2016), some studies use the concentration of malondialdehyde (MDA) to assess oxidative damage because it is a relatively stable peroxidation byproduct of long-chain PUFAs that are common in cell membranes (Halliwell and Gutteridge, 2007; Ayala et al., 2014). MDA levels were elevated in blood samples taken from Budgerigars (Melopsittacus undulatus) 24 h after completing 5 days of mild escape-flight training, although this effect was largely dependent on whether or not individuals had been supplemented with antioxidants (Larcombe et al., 2008). Conversely, natural variation in aerobic activity (including flight) of Budgerigars was unrelated to MDA concentrations (Larcombe et al., 2015). Likewise, MDA concentration did not differ between Common Blackbirds presumed to be year-round residents versus presumed to have recently completed a migratory flight (Eikenaar et al., 2017). Therefore, studies that examine oxidative damage to lipids (i.e., d-ROMs and MDA) show varied responses to flight, likely due to variation in flight intensity and the extent to which a bird’s antioxidant system responds in preparation for and during flight.
Oxidative damage to protein, measured as the concentration of protein carbonyls (Halliwell and Gutteridge, 2007; Davies, 2016), has also been assessed in response to flight given the potential for muscle damage (Guglielmo et al., 2001; Dick and Guglielmo, 2019). Plasma protein carbonyls were significantly higher in Zebra Finches flown for 3 h at 0.9 km/hr compared to individuals flown at 0.3 km/hr or unflown individuals (Costantini et al., 2013). Similarly, concentration of protein carbonyls in the flight muscle of Yellow-rumped Warblers was positively related to the duration of flight in a wind tunnel and was significantly higher in individuals flown for 6 h than for unflown individuals (Dick and Guglielmo, 2019). Meanwhile, in a field study on European Robins (Erithacus rubecula) during migration, plasma concentrations of protein carbonyls were highest among birds caught at night and therefore presumably directly out of endurance flight (Jenni-Eiermann et al., 2014).
In sum, studies on the acute effects of flight on oxidative damage provide evidence for an increase in damage after flight (as assessed using d-ROMs, MDA, and protein carbonyls) but certainly not in all cases. Only protein carbonyls have consistently increased in response to flight, although there are few studies to date that have used this measure. Clearly, levels of oxidative damage are dynamic and seem responsive to a host of ecologically relevant factors, including duration of flight and diet, which complicates comparisons between studies especially when different assays are used.
Birds can protect themselves from exercise-induced increases in RS production using a robust endogenous antioxidant system (Figure 1). Whether a bird depletes its antioxidant capacity, or increases it in response to flight, may depend on ecologically relevant factors such as individual condition (e.g., fat stores and oxidative damage levels), access to dietary antioxidants, and the amount of exercise. Enzymatic and non-enzymatic antioxidants may also respond differently to acute oxidative challenges, indicating that these components may serve different or complimentary roles in the antioxidant system.
Enzymatic antioxidants, including glutathione peroxidase (GPx), superoxide dismutase (SOD), and catalase (CAT), are the most commonly assayed enzymes in avian studies and have been shown in most cases to rapidly increase in activity in response to flight. For example, European Robins caught during nocturnal migratory flight had higher GPx activity compared to birds on stopover (Jenni-Eiermann et al., 2014), and Yellow-rumped Warblers flown in a wind tunnel had higher SOD after flight than individuals at rest (Dick and Guglielmo, 2019). The response of enzymatic antioxidants in flight-trained European Starlings to an approximately 3-h flight in a wind tunnel depended on availability of dietary antioxidants (Frawley et al., 2021). Specifically, starlings fed a diet not supplemented with anthocyanin, a dietary antioxidant, had decreased GPx activity immediately after flight, whereas GPx activity did not change after flight for starlings fed a diet supplemented with anthocyanins (Frawley et al., 2021). Furthermore, Zebra Finches flown for short bursts had similar serum GPx, CAT, and SOD activity after flight compared to unflown control birds (Costantini et al., 2013). Zebra Finches flown for 2 h had elevated GPx activity immediately after flight, and this acute increase in GPx activity was consistently observed even after ca. 2 and 6 weeks of daily flight, indicating that birds were able to rapidly downregulate GPx activity when the oxidative challenge had passed (Cooper-Mullin et al., 2019). Interestingly, daily flight by Zebra Finches over 1.5 months increased coordination between the enzymatic (GPx) and non-enzymatic components of the antioxidant system (Cooper-Mullin et al., 2019). Furthermore, the inclusion of dietary precursors for these enzymatic antioxidants (e.g., selenium for GPx) can increase the activity of the enzyme during flight (Zigo et al., 2017). The common theme that emerges from these studies is that the activity of enzymatic antioxidants rapidly changes during bird flight, but the extent of these changes is related to the availability of dietary antioxidants and the response of other components of the antioxidant system.
Non-enzymatic endogenous antioxidants include sacrificial molecules such as glutathione (GSH) and uric acid, which arise from a diverse set of metabolic pathways and cycles and have varying metabolic uses (Tsahar et al., 2006; Carro et al., 2012; Cooper-Mullin and McWilliams, 2016). Uric acid, a byproduct of protein catabolism and a powerful antioxidant, has been found to consistently increase after flight in several field and lab studies. European Starlings flown in wind tunnels had elevated serum uric acid immediately after up to 6 h of flight (Carter et al., 2020; McWilliams et al., 2020; Frawley et al., 2021). White-crowned Sparrows (Zonotrichia leucophrys) that were flown at high speeds in a hop/hover wheel (Tsahar et al., 2006) and pigeons flown for 4 h (Gannes et al., 2001) also had increased uric acid concentrations after flight. Serum uric acid was also higher in Garden Warblers, Pied Flycatchers (Ficedula hypoleuca), and European Robins on active migration compared to birds at rest or refueling on stopover (Jenni-Eiermann and Jenni, 1991). Given that it is a byproduct of protein catabolism during exercise, uric acid may serve as a particularly important antioxidant for flying birds.
The complexity of interpreting uric acid measurements as a marker of non-enzymatic antioxidant capacity can be avoided by using the OXY-adsorbent test, a common serum-based assay that provides a general snapshot of a bird’s ability to quench RS. Generally, OXY has been shown to decrease after flight, indicating that birds deplete antioxidant capacity in response to an acute challenge. Pigeons showed a 19% drop in serum antioxidant capacity after being flown for 200 km (ca. 310 ± 31 min, Costantini et al., 2008), and Zebra Finches depleted antioxidant capacity immediately after 2 h of flight and then increased OXY after getting a reprieve from regular daily flying (Cooper-Mullin et al., 2019). European Starlings also reduced OXY (and oxidative damage, noted above) immediately after flights in a wind tunnel, and the extent of this change was more apparent in birds that expended more energy during their longest flights (DeMoranville, 2020). OXY also changed in European Starlings immediately after flights, although the direction and extent of the change depended on season (fall vs. spring migration) and whether diets were supplemented with antioxidants (Frawley et al., 2021).
In summary, there is some evidence that enzymatic antioxidants, particularly GPx, may serve as a first line of defense for birds faced with an oxidative challenge, although research is scarce on the acute responses of other major antioxidant enzymes, particularly CAT and SOD. Likewise, non-enzymatic endogenous antioxidants such as uric acid consistently increase after flight, suggesting they may be functionally important. In contrast, other components of non-enzymatic antioxidant capacity as measured with the OXY test usually decrease after flight, and the extent of this acute change in OXY after flight depends on the acute response of enzymatic antioxidants such as GPx when regular daily flying is prolonged (Cooper-Mullin et al., 2019). Consumption of dietary antioxidants by European Starlings affects the response of enzymatic antioxidants as well as non-enzymatic antioxidants (DeMoranville et al., 2021; Frawley et al., 2021) and has a multitude of related effects: antioxidant consumption reduces corticosterone production in flight-trained birds, and thus potentially protects against the costs of high glucocorticoids (Casagrande et al., 2020), and enhances the breeding condition of males (Carbeck et al., 2018). The role of corticosterone during preparation for migration, in departure during migration (e.g., from stopover sites), and in support of sustained migratory flights has been recently reviewed by Bauer and Watts (2021). Importantly, Cooper-Mullin et al. (2021) used stable isotope-labeled vitamin E to reveal that consumed dietary antioxidants are absorbed and reach muscle mitochondria of Zebra Finches but only when they are regularly exercised (2 h of regular daily flying). Further studies are needed that simultaneously measure multiple components of the antioxidant system and the contribution of dietary antioxidants to better understand how birds respond to the oxidative demands of flight and migration.
When a given flight is associated with an acute increase in oxidative damage and an acute decrease in antioxidant capacity, then the interpretation is relatively straightforward: higher metabolic rate during exercise increases the escape of radicals from the electron transport chain, RS diffuse from the mitochondria to the cytosol and intercellular spaces, and then they are quenched by certain aspects of the antioxidant system. Here we discuss possible mechanisms that can explain other results (e.g., no oxidative damage or change in certain components of the antioxidant system).
When no acute changes in oxidative damage or antioxidant capacity are observed following flight, one possible interpretation is that flight did not increase the amount of radicals escaping from the electron transport chain. There are several, not mutually exclusive, ways that this could be achieved: production of electrons (in the form of reduced nicotinamide adenine dinucleotide, NADH) by the citric acid cycle could be limited by reduced mass-specific activity of the cycle (Tretter and Adam-Vizi, 2005). Alternately, electrons are less likely to escape and form radicals if they are rapidly combined with protons and oxygen to form water. In addition, uncoupling proteins may prevent the formation of large gradients that might produce a scarcity of protons to combine with electrons (Cooper-Mullin and McWilliams, 2016). Electron escape and RS production might also be regulated by the concentration and activity of cytochrome oxidase, which catalyzes the combination of electrons, protons and oxygen (Bode et al., 2013). Although these factors have not been studied extensively in birds or in relation to aerobic activity, variation in RS production across species and tissues could help explain the range of acute responses to flight in birds documented across studies to date.
Inconsistent responses of oxidative damage and antioxidant capacity to flight may also be the result of complex interactions between RS production and the antioxidant system of birds. As a general model, we might assume that RS are continuously produced in animal tissues at a certain rate and that this rate increases during flight (Halliwell and Gutteridge, 2007). Simultaneously, RS and byproducts of RS damage may be neutralized at a certain rate through the actions of antioxidant enzymes, sacrificial molecules, and dietary compounds (Figure 1; Cooper-Mullin and McWilliams, 2016). Combined, this means that RS, and therefore measured concentrations of damaged compounds, will accumulate and increase when the rate of RS production is greater than the rate of neutralization and decrease when the rate of neutralization is greater. If the rate of RS neutralization is static, then damage will accumulate throughout the period of flight and then decrease during the following recovery period. However, it is well established that the antioxidant system can flexibly respond and can increase capacity by upregulating the expression of antioxidant enzymes, upregulating the production of sacrificial molecules, and increasing the transport of stored antioxidants into target tissues (Cooper-Mullin and McWilliams, 2016; Costantini, 2019). Moreover, it is reasonable to expect the rate of RS neutralization to be upregulated proportionally with the accumulation of RS and RS byproducts (Dröge, 2002).
In Figure 4, we present a conceptual model that demonstrates how the extent of measurable oxidative damage associated with a given flight may depend on the pace and extent of antioxidant upregulation, and the intensity of the flight. In particular, a simple exponential response of neutralization to RS concentration can limit the accumulation of RS during flight and lead to more rapid reductions following flight. Meanwhile, the addition of a lag in response, due to the time required for signaling and the synthesis of antioxidants (Ji et al., 2006; Sthijns et al., 2016), is enough to create a simple hormetic response where accumulated damage decreases in the middle of the flight [Figure 4, High RS – AOX (lag)]. Hormesis can also depend on the intensity of an oxidative challenge (Nikolaidis et al., 2012; Costantini, 2014), meaning that milder activity can, at times, produce a muted antioxidant response and therefore higher levels of accumulated RS byproducts [Figure 4, Low RS – AOX (lag)]. All of these relatively simple factors can interact to produce considerable variation in measurement of damage, which will depend on the timing of sample collection, the tissue collected, the intensity of activity, and the regulatory responsiveness of the study species. Furthermore, there is evidence that the antioxidant response may differ between seasons and tissues, and that access to dietary antioxidants may boost or attenuate certain antioxidant responses (Frawley et al., 2021) and this can depend on regular exercise (Cooper-Mullin et al., 2019, 2021). In sum, the antioxidant response is not static, and acquiring a better understanding of the pace and extent of antioxidant up- and down-regulation under ecologically relevant situations is needed.
Figure 4. Conceptual model of the accumulation of RS byproducts measured by oxidative damage assays. The cumulative sum of the rates of RS production and neutralization over time (A,B) produce the net accumulated oxidative damage at a given time point (C,D). The rate of RS production increases to a set level during flight (shaded areas) and then decreases during recovery, with the set level higher for more strenuous flight (A) than moderate flight (B). The rate of scalable antioxidant activity changes exponentially in response to accumulated damage, and therefore rates of RS neutralization (AOX) also vary between strenuous and moderate flight. Moreover, the rate of RS neutralization becomes more variable and increases more dramatically with a greater delay in response time [AOX (lag)]. Subsequent measurements of oxidative damage will depend on the responsiveness of the antioxidant system and the timing of the measurement, with strenuous flight (C) sometimes resulting in lower measurements of damage than moderate flight (D).
Birds arriving at a stopover site are faced with the prospect of taking off again in a few days to reach their next stopover or final destination. In many cases, these birds will have experienced oxidative damage from long-distance flight (see previous section), which may have compromised their structural or stored fats and/or proteins. In addition to contending with the consequences of this damage, birds must also build fuel stores to prepare for their next flight. On stopover, therefore, a bird should recover from the damage it has experienced and prepare (both in terms of accumulating energy and antioxidants) for the next oxidatively challenging activity (i.e., flight to the next destination and/or breeding).
In the context of oxidative status, we define ‘recovery’ by migratory birds as a decrease in oxidative damage to fats and proteins and a potential concomitant increase in chiefly non-enzymatic antioxidant capacity. As we describe below, evidence exists that birds do ‘recover’ on stopover, but their capacity to do so may depend on time, a bird’s condition, and the resources it finds at the stopover site. In songbirds, experimental evidence has shown that birds are what they eat (the fatty acids they consume are incorporated into fat stores), birds use what they are (birds catabolize their fat stores for flight and thermoregulation), and what birds are influences physiological performance (Pierce and McWilliams, 2005, 2014; McWilliams et al., 2020). For instance, the molecular make-up of dietary fatty acids contributes to oxidative damage (Cooper-Mullin and McWilliams, 2016; Skrip and McWilliams, 2016). Starlings fed a PUFA-rich diet experienced higher oxidative damage than those fed a MUFA-rich diet (McWilliams et al., 2020), indicating that the type of fat birds consume on stopover likely affects the scope of potential recovery from oxidative damage incurred during flight. If birds have access to dietary antioxidants on stopover, then it is likely that these exogenous antioxidants could increase the scope of recovery by, for example, protecting fat stores. However, no study has directly examined this possibility in the wild. Post-flight recovery research on migratory birds is still emerging, characterized by a series of ‘firsts.’
The first evidence of potential acute recovery on stopover came from actively migrating European Robins (Erithacus rubelica) caught flying at night through a Swiss mountain pass in autumn (Jenni-Eiermann et al., 2014). Birds captured at night out of flight had higher oxidative damage to proteins (circulating protein carbonyls) than birds captured while resting during the day (Jenni-Eiermann et al., 2014). However, the timeframe over which recovery occurred was unknown, because damage was not tracked over time within individuals. The first study to track oxidative damage in relation to time after a long-distance flight found that circulating oxidative damage to lipids (d-ROMs) decreased among recaptured Garden Warblers the longer they were at a Mediterranean stopover site in spring [between 1 and 192 h (8 days)]; opportunistic intra-individual sampling (n = 2) confirmed that damage decreased within birds over a matter of days (Skrip et al., 2015). Taking ‘the next logical step’ after Skrip et al.’s (2015) cross-sectional finding, Eikenaar et al. (2020b) longitudinally tracked changes in damage within individuals and confirmed that migrating songbirds are capable of recovering from oxidative damage on stopover. Northern Wheatears caught out of spring migration in Germany and held in captivity for 3 days decreased their oxidative damage to polyunsaturated fats (MDA) and appeared to stabilize that damage at a consistent level, suggesting that there may be a threshold of low damage or ‘oxidative set-point’ (Eikenaar et al., 2020b) that birds seek to reach before taking their next long-distance flight.
Taken together, these three studies provide the first evidence that songbirds are capable of recovering from oxidative damage after flight. How they do so, however, remains an open question. Do birds repair damaged molecules, replace them, or a combination of both? Do resources available at a given stopover site or the physiological condition of an individual bird influence how birds address oxidative damage (Figure 1)? The minimum time within which birds begin to recover after a flight is also uncertain. Migrating songbirds held in handling bags for short periods before blood sampling (30 min, Costantini et al., 2007; 20–192 min, Skrip et al., 2015) exhibited no change in oxidative measures. However, Gray Catbirds (Dumetella carolinensis) caught during autumn migration and kept overnight had increased uric acid and decreased levels of residual total antioxidant capacity within at least a 12-h period (Cohen et al., 2008a).
Evidence is also mounting that birds are capable of recovering their antioxidant capacity while on stopover. Although time on stopover and circulating non-enzymatic antioxidant capacity (OXY) were not correlated in the cross-sectional analysis of Garden Warblers described above, antioxidant capacity did increase in the two Garden Warblers opportunistically resampled over the course of several days, hinting that such recovery is possible (Skrip et al., 2015). An experimental study with flight-trained European Starlings (DeMoranville, 2020) was the first to demonstrate that songbirds can recover antioxidant capacity after long-distance flight. Starlings depleted their circulating non-enzymatic antioxidant capacity (OXY) during 15 days of nearly daily flight in a wind tunnel (totaling >640 km), but within 2 days of rest (i.e., no flight), birds recovered to their baseline (pre-flight-training) levels independent of whether they were supplemented with water-soluble antioxidants (i.e., anthocyanin) (DeMoranville, 2020). There is evidence that restoration of antioxidant capacity in metabolically active tissues is mediated by the transcription of antioxidant enzyme proteins. For example, during recovery, these same flight-trained starlings had greater mRNA levels of antioxidant enzymes in the liver (CAT, SOD2, and GPX1) and pectoralis (SOD2) compared to unflown starlings, while lipid damage levels were similar (DeMoranville et al., 2021). Like recovery from damage, recovery of antioxidant capacity, however, remains to be mechanistically explained.
Consumption of dietary antioxidants on stopover may contribute to recovery of antioxidant capacity, and migratory birds seem able to select diets that provide fats and antioxidants that can assist or accelerate this recovery (Sapir et al., 2004; Cohen et al., 2009). Migratory songbirds at an offshore New England stopover site during autumn preferred fruits from species with higher fat content and selected fruits rich in dietary antioxidants (Alan et al., 2013; Bolser et al., 2013). Birds can select diets using solely antioxidant content, as shown by a series of food-choice experiments involving Blackcaps (Sylvia atricapilla) (Catoni et al., 2008a, b; Schaefer et al., 2014). Given a choice of food supplemented with anthocyanins or not, Blackcaps preferred a supplemented diet (Catoni et al., 2008a; Schaefer et al., 2008). Sources of dietary antioxidants during spring migration are understudied but may include seeds (Cohen et al., 2008b; Beaulieu and Schaefer, 2013), insects (Catoni et al., 2008a; Eeva et al., 2010), leaves (Catoni et al., 2008a), and fruits (Cohen et al., 2009; Alan and McWilliams, 2013; Bolser et al., 2013). It is highly likely that birds take advantage of fruits in tropical areas prior to or during spring migration, as suggested by a geolocator study that found migrating Red-Eyed Vireos make an unexpectedly long stopover in Colombia during spring (Callo et al., 2013).
Recent experiments with actively migrating birds in autumn in New England have revealed the benefits of consuming dietary antioxidants and how antioxidant capacity and fat stores interactively influence the time passerines spend on stopover (Cooper-Mullin and McWilliams, 2021). For example, gavage-feeding dietary anthocyanins to Hermit Thrushes (Catharus guttatus) on an ad libitum diet boosted their ability to build non-enzymatic antioxidant capacity (OXY; Cooper-Mullin and McWilliams, 2021). Furthermore, birds on an ad libitum diet increased non-enzymatic antioxidant capacity (OXY) compared to birds on a diet designed to maintain their weight at capture (Cooper-Mullin and McWilliams, 2021). Studies in mammals have identified anthocyanins as enhancers of the NRF2 antioxidant pathway that activates the expression of antioxidant genes (Shih et al., 2007; Aboonabi and Singh, 2015; Tian et al., 2019; Aboonabi et al., 2020). Dietary antioxidants may also help songbirds increase enzymatic antioxidant capacity by stimulating the same pathway. For example, European Starlings supplemented with anthocyanins had higher mRNA levels of antioxidant enzymes associated with combating reactive species production in the mitochondria (SOD2) and lipid peroxidation in the peroxisome (CAT) in flight muscle compared to unsupplemented birds (DeMoranville et al., 2021). How exogenous and endogenous antioxidants interact in birds on stopover while recovering or refueling still needs more investigation.
The studies described above demonstrate that birds (evidence mostly in songbirds) can decrease oxidative damage and build antioxidant capacity rapidly, in a matter of days after a migratory flight. These studies also demonstrate that dietary anthocyanins (Catoni et al., 2008a; Schaefer et al., 2008), and flavonoids (Catoni et al., 2008b), as well as dietary PUFA (Alan and McWilliams, 2013; DeMoranville et al., 2020; McWilliams et al., 2020), influence a songbird’s oxidative status and stopover length (Cooper-Mullin and McWilliams, 2021). However, how the quantity or quality of a bird’s fat stores, the energetic demands of previous flights, and the available resources at a stopover aid or hinder this recovery is unclear. The challenge of refueling on stopover is twofold for birds: they have to accumulate fatty acids that make them more efficient fliers (Price, 2010; Ferretti et al., 2019; McWilliams et al., 2020), but they also need to protect those fats as well as recover from the previous flight (Metzger and Bairlein, 2011; Skrip et al., 2015). Future research should focus on the oxidative challenges that recovery and refueling simultaneously pose to migrating birds.
The components of the antioxidant system of birds (Figures 1, 2) are broadly similar to that of all aerobic organisms; however, there are several unique aspects of the avian antioxidant system that are important and relevant to our understanding of how birds during migration maintain oxidative balance. First, a unique mutation in the major antioxidant regulation pathway enables 95+% of bird species to maintain a constitutively active master antioxidant response that lowers the risk of macromolecular oxidative damage associated with relatively high metabolic rates (Castiglione et al., 2020). Second, the antioxidant system of migratory birds is able to flexibly and rapidly respond to the oxidative challenges of flying and fasting (Figure 3) during the course of migration, with dietary antioxidants and protein catabolism (i.e., uric acid production) playing key roles in maintaining oxidative balance. Given that uric acid is a byproduct of protein catabolism in birds, it may serve as a particularly important antioxidant for flying birds as they contend with variation in both reactive species production and the capacity of their antioxidant system.
Our review of the evidence to date suggests that in preparation for a migratory flight (1a) migratory birds increase some component(s) of their antioxidant capacity (notably, non-enzymatic antioxidant capacity and circulating uric acid) as they build fat stores, although increasing fat may come at the cost of increased oxidative damage; and (1b) the extent of oxidative damage associated with the increase in fat stores may be related to the extent to which birds simultaneously build antioxidant capacity in preparation for a migratory flight. (2) The acute effects of long-duration flight often include an increase in lipid damage (d-ROMs and MDA), although not in all cases, and an increase in markers of protein damage (protein carbonyls) in all cases thus far documented. Importantly, few studies document how the many components of antioxidant capacity (enzymatic, non-enzymatic, and dietary; Figure 1) respond during a given flight in relation to oxidative damage. Evidence to date suggests that enzymatic antioxidants, particularly GPx, as well as uric acid, a non-enzymatic endogenous antioxidant (Figure 2), rapidly increase in response to flight or onset of migration state. Importantly, the extent of such increases can be tissue-specific (GPx) or depend on availability of dietary antioxidants which suggests that the costs of enzymatic antioxidants and protein catabolism may be reduced when dietary antioxidants are available. Many more studies are needed that focus on the acute effects of flight before we adequately understand how such a dynamic antioxidant system responds to a host of ecologically relevant factors, including extent of flight and diet. (3) Migratory birds seem able to recover from oxidative damage accrued during long-duration flights while also rebuilding antioxidant capacity, although the extent of recovery seems to depend on bird condition (i.e., quantity and quality of a bird’s remaining fat stores), and the available resources at a stopover site, including dietary antioxidants.
Given the dynamic and responsive nature of the antioxidant system, we maintain that integrative studies (from mitochondria to cells to tissues to whole organism) that are also comparative (e.g., multiple tissues within the same individuals, migration-state vs. non-migration periods, multiple species that differ in migration strategy) are required to fully understand the antioxidant system of migratory birds within an ecological context. Below we outline some future studies that would improve our understanding of this dynamic physiological system.
The ability to use endogenous and exogenous antioxidants varies among individuals depending on body condition, recent oxidative challenges, and ecological factors (Dick and Guglielmo, 2019; Loughland and Seebacher, 2020; Cooper-Mullin et al., 2021). This individual variation extends to the specific antioxidant strategies available to birds during oxidative challenges, as individuals may also rely on alternate antioxidants to protect against damage (DeMoranville, 2020). Future longitudinal studies that examine intraindividual antioxidant responses to oxidative challenges (e.g., flight, high PUFA diets, lack of dietary antioxidants, breeding, and temperature acclimatization) will help us understand the protective strategies available to birds during specific life history stages. Especially informative would be longitudinal studies that compare tissues that vary in metabolic rate, mitochondrial density, and potentially in their capacity to maintain oxidative balance (e.g., DeMoranville et al., 2020, 2021; Frawley et al., 2021).
More controlled captive-bird experiments as well as field studies are needed that test the proposed hypothesis that as birds build fat stores in preparation for migration they also build certain components of their antioxidant capacity. More specifically, does the extent of the building of antioxidant capacity during preparation for migration determine the extent of observed oxidative damage. For example, to date there has been no longitudinal study of wild songbirds to parallel the multi-season assessment of Gutiérrez et al. (2019) who sampled Hudsonian Godwits at three time periods from January to March (wintering, fuelling, and pre-departure), the latter as they prepared for their >10,000-km northbound migratory flight. Marasco et al.’s (2021) study of captive-reared migratory Common Quails reminds us that controlled experiments using photoperiod manipulations to induce migration state in birds can provide important complementary (and at times contradictory) information about how the antioxidant system changes during preparation for migration. Tracking the oxidative and metabolic status of songbirds in the months and weeks leading up to departure would be particularly valuable to understanding in situ preparation, especially in the context of available dietary fat and antioxidant resources.
It remains unknown what amount of damage is considered too much damage. Our understanding of the varying response of songbirds to oxidative stress in migration and other contexts would be greatly supported by a more robust understanding of the phenomenon of oxidative damage. In particular, the time course over which the processes of RS production, antioxidant upregulation/mobilization, and RS neutralization occur during flight remains unknown. One way to evaluate this conundrum is to conduct studies that link oxidative status (antioxidant capacity and oxidative damage) to measures of short- and long-term performance (e.g., flight efficiency, speed and timing of migration, foraging, refueling, and fecundity). Doing so within the context of important life history stages such as migration and breeding would reveal potential carryover effects (McWilliams et al., 2021). Only one study to date (Skrip et al., 2016) has examined how antioxidant status during repeated flights change antioxidant allocation during subsequent breeding. At least in that model species (Zebra finch), use of dietary antioxidants to maintain oxidative balance during repeated twice-daily flights over months affected female allocation of dietary antioxidants to eggs. No studies to date of a migratory bird have determined the consequences of such carryover effects (with dietary antioxidants serving as the currency) from one stage of the annual cycle to the next on reproductive success.
There is a considerable amount of crosstalk among major physiological pathways including those involved in metabolism (PPARs, glucocorticoid production), immunity, inflammation (NF-κB), and antioxidants (NRF2 and PPARs) due to shared/multifunctional transcription factors that respond to similar factors like exercise, RS production, and dietary antioxidants (Finck and Kelly, 2006; Syeda et al., 2019). One such recent study examined these shared transcription factors and their downstream genes in a migratory bird and found that flying over the course of 2 weeks and consuming antioxidants influenced both metabolic and antioxidant mRNA expression in a tissue specific manner (DeMoranville et al., 2020, 2021). Studies that integrate across multiple systems including the metabolic, antioxidant, immune, inflammatory, endocrine, will help assess the overall state of the birds and determine if modulating the antioxidant system, for example, leads to tradeoffs with other physiological states. One such recent study of this type suggests a possible trade-off between the immune and antioxidant systems in a migratory bird (Eikenaar et al., 2018).
No study to date has examined the extent to which dietary antioxidants are directly used to protect accumulating fat stores and/or contribute to recovery from oxidative damage incurred during flight. The recent study by Cooper-Mullin et al. (2021) used stable-isotope labeled Vitamin E to demonstrate for the first time that consumed dietary antioxidants are absorbed and transported to the mitochondria of flight muscle, but only if birds are exercising. What remains to be demonstrated is how these and other types of dietary antioxidants are used during metabolism, and the extent to which they reduce the need to upregulate more costly components of the antioxidant system such as enzymes. Metabolism of all nutrients starts with processing in and absorption through the gut, and this is influenced by the gut microbiome (Clark and Mach, 2017). The extent to which variation in the gut microbiome of birds while in migration affects the availability and utilization of ingested antioxidants is not known, although such information has important implications for both our understanding of how birds work (i.e., their physiology) and also how to ensure that conservation and management efforts provide what they require during migration (Bahrndorff et al., 2016; Trevelline et al., 2019).
All authors listed have made a substantial, direct and intellectual contribution to the work, and approved it for publication.
The authors were supported by a grant from the National Science Foundation to SM and BP (IOS-1354187), and by The University of Rhode Island, College of the Environment and Life Sciences.
The authors declare that the research was conducted in the absence of any commercial or financial relationships that could be construed as a potential conflict of interest.
All claims expressed in this article are solely those of the authors and do not necessarily represent those of their affiliated organizations, or those of the publisher, the editors and the reviewers. Any product that may be evaluated in this article, or claim that may be made by its manufacturer, is not guaranteed or endorsed by the publisher.
Aboonabi, A., and Singh, I. (2015). Chemopreventive role of anthocyanins in atherosclerosis via activation of Nrf2-ARE as an indicator and modulator of redox. Biomed. Pharmacother. 72, 30–36. doi: 10.1016/j.biopha.2015.03.008
Aboonabi, A., Singh, I., and Rose’ Meyer, R. (2020). Cytoprotective effects of berry anthocyanins against induced oxidative stress and inflammation in primary human diabetic aortic endothelial cells. Chem. Biol. Interact. 317:108940. doi: 10.1016/j.cbi.2020.108940
Abuja, P. M., and Albertini, R. (2001). Methods for monitoring oxidative stress, lipid peroxidation and oxidation resistance of lipoproteins. Clin. Chim. Acta 306, 1–17. doi: 10.1016/s0009-8981(01)00393-x
Alan, R. R., and McWilliams, S. R. (2013). Oxidative stress, circulating antioxidants, and dietary preferences in songbirds. Comp. Biochem. Physiol. Part B 164, 185–193. doi: 10.1016/j.cbpb.2012.12.005
Alan, R. R., McWilliams, S. R., and McGraw, K. J. (2013). The importance of antioxidants for avian fruit selection during autumn migration. Wilson J. Ornithol. 125, 513–525. doi: 10.1676/13-014.1
Ayala, A., Muñoz, M. F., and Argüelles, S. (2014). Lipid peroxidation: production, metabolism, and signaling mechanisms of malondialdehyde and 4-hydroxy-2-nonenal. Oxid. Med. Cell. Longev. 2014:360438. doi: 10.1155/2014/360438
Bahrndorff, S., Alemu, T., Alemneh, T., and Lund Nielsen, J. (2016). The microbiome of animals: implications for conservation biology. Int. J. Genomics 2016:5304028. doi: 10.1155/2016/5304028
Bairlein, F., Fritz, J., Scope, A., Schwendenwein, I., Stanclova, G., van Dijk, G., et al. (2015). Energy expenditure and metabolic changes of free-flying migrating northern bald ibis. PLoS One 10:e0134433. doi: 10.1371/journal.pone.0134433
Banerjee, S., and Chaturvedi, C. M. (2016). Migratory preparation associated alterations in pectoralis muscle biochemistry and proteome in Palearctic-Indian emberizid migratory finch, red-headed bunting Emberiza bruniceps. Comp. Biochem. Physiol. Part D 17, 9–25. doi: 10.1016/j.cbd.2015.11.001
Barja, G. (1998). Mitochondrial free radical production and aging in mammals and birds. Ann. N. Y. Acad. Sci. 854, 224–238. doi: 10.1111/j.1749-6632.1998.tb09905.x
Bauer, C. M., and Watts, H. E. (2021). Corticosterone’s roles in avian migration: assessment of three hypotheses. Horm. Behav. 135:105033. doi: 10.1016/j.yhbeh.2021.105033
Beaulieu, M., and Schaefer, H. M. (2013). Rethinking the role of dietary antioxidants through the lens of self-medication. Anim. Behav. 86, 17–24. doi: 10.1016/j.anbehav.2013.05.022
Bishop, P. J., and Butler, P. J. (2015). “Flight,” in Sturkie’s Avian Biology, ed. C. G. Scanes (New York, NY: Academic Press Inc).
Bode, M., Longen, S., Morgan, B., Peleh, V., Dick, T. P., Bihlmaier, K., et al. (2013). Inaccurately assembled cytochrome c oxidase can lead to oxidative stress-induced growth arrest. Antioxid. Redox Signal. 18, 1597–1612. doi: 10.1089/ars.2012.4685
Bolser, J. A., Alan, R. R., Smith, A. D., Li, L., Seeram, N. P., and McWilliams, S. R. (2013). Birds select fruits with more anthocyanins during autumn migration. Wilson J. Ornithol. 124, 97–108. doi: 10.1676/12-057.1
Bursian, S. J., Alexander, C. R., Cacela, D., Cunningham, F. L., Dean, K. M., Dorr, B. S., et al. (2017a). Overview of avian toxicity studies for the deepwater horizon natural resource damage assessment. Ecotoxicol. Environ. Saf. 142, 1–7. doi: 10.1016/j.ecoenv.2017.03.046
Bursian, S. J., Dean, K. M., Harr, K. E., Kennedy, L., Link, J. E., Maggini, I., et al. (2017b). Effect of oral exposure to artificially weathered Deepwater Horizon crude oil on blood chemistries, hepatic antioxidant enzyme activities, organ weights and histopathology in western sandpipers (Calidris mauri). Ecotoxicol. Environ. Saf. 146, 91–97. doi: 10.1016/j.ecoenv.2017.03.045
Butler, P. J., and Woakes, A. J. (2001). Seasonal hypothermia in a large migrating bird: saving energy for fat deposition? J. Exp. Biol. 204, 1361–1367. doi: 10.1242/jeb.204.7.1361
Buttemer, W. A., Abele, D., and Costantini, D. (2010). From bivalves to birds: oxidative stress and longevity. Funct. Ecol. 24, 971–983. doi: 10.1111/j.1365-2435.2010.01740.x
Callo, P. A., Morton, E. S., and Stutchbury, B. J. M. (2013). Prolonged spring migration in the red-eyed vireo (Vireo olivaceus). Auk 130, 240–246. doi: 10.1525/auk.2013.12213
Carbeck, K. M., DeMoranville, K. J., D’Amelio, P. B., Goymann, W., Trost, L., Pierce, B., et al. (2018). Environmental cues and dietary antioxidants affect breeding behavior and testosterone of male European starlings (Sturnus vulgaris). Horm. Behav. 103, 36–44. doi: 10.1016/j.yhbeh.2018.05.020
Carlisle, J. D., Olmstead, K. L., Richart, C. H., and Swanson, D. L. (2012). Food availability, foraging behavior, and diet of autumn migrant landbirds in the Boise foothills of southwestern Idaho. Condor 114, 449–461. doi: 10.1525/cond.2012.100209
Carpenter, F. L., and Hixon, M. A. (1988). A new function for torpor: fat conservation in a wild migrant hummingbird. Condor 90, 373–378. doi: 10.2307/1368565
Carro, M. D., Settle, T., and Klandorf, H. (2012). “The role of uric acid in the avian species,” in Uric Acid, eds S. Castillo and E. Maldonado (Hauppauge, NY: Nova Science Publishers, Inc).
Carter, W. A., Cooper-Mullin, C., and McWilliams, S. R. (2018). Turnover of muscle lipids and response to exercise differs between neutral and polar fractions in a model songbird, the zebra finch. J. Exp. Biol. 221:jeb68823.
Carter, W. A., DeMoranville, K. J., Pierce, B. J., and McWilliams, S. R. (2020). The effects of dietary linoleic acid and hydrophilic antioxidants on basal, peak, and sustained metabolism in flight-trained European starlings. Ecol. Evol. 10, 1552–1566. doi: 10.1002/ece3.6010
Carter, W. A., DeMoranville, K. J., Pierce, B. J., and McWilliams, S. R. (2021). Dietary linoleic acid, antioxidants, and flight training influence the activity of oxidative enzymes in European Starlings (Sturnus vulgaris). J. Comp. Physiol. B. 191, 357–370.
Casagrande, S., DeMoranville, K. J., Trost, L., Pierce, B., Bryła, A., Dzialo, M., et al. (2020). Dietary antioxidants attenuate the endocrine stress response during long-duration flight of a migratory bird. Proc. R. Soc. Biol. Sci. 287:20200744. doi: 10.1098/rspb.2020.0744
Castiglione, G. M., Xu, Z., Zhou, L., and Duh, E. J. (2020). Adapatation of the master antioxidant response connects metabolism, lifespan and feather development pathways in birds. Nat. Commun. 11, 1–15.
Catoni, C., Peters, A., and Martin Schaefer, H. (2008a). Life history trade-offs are influenced by the diversity, availability and interactions of dietary antioxidants. Anim. Behav. 76, 1107–1119. doi: 10.1016/j.anbehav.2008.05.027
Catoni, C., Schaefer, H. M., and Peters, A. (2008b). Fruit for health: the effect of flavonoids on humoral immune response and food selection in a frugivorous bird. Funct. Ecol. 22, 649–654. doi: 10.1111/j.1365-2435.2008.01400.x
Chernetsov, N. (2006). Habitat selection by nocturnal passerine migrants en route: mechanisms and results. J. Ornithol. 147, 185–191. doi: 10.1007/s10336-006-0064-6
Clark, A., and Mach, N. (2017). The crosstalk between the gut microbiota and mitochondria during exercise. Front. Physiol. 8:319. doi: 10.3389/fphys.2017.00319
Cohen, A. A., Hau, M., and Wikelski, M. (2008a). Stress, metabolism, and antioxidants in two wild passerine bird species. Physiol. Biochem. Zool. 81, 463–472. doi: 10.1086/589548
Cohen, A. A., McGraw, K. J., and Robinson, W. D. (2009). Serum antioxidant levels in wild birds vary in relation to diet, season, life history strategy, and species. Oecologia 161, 673–683. doi: 10.1007/s00442-009-1423-9
Cohen, A. A., McGraw, K. J., Wiersma, P., Williams, J. B., Robinson, W. D., Robinson, T. R., et al. (2008b). Interspecific associations between circulating antioxidant levels and life−history variation in birds. Am. Nat. 172, 178–193. doi: 10.1086/589456
Colombini, F., Carratelli, M., and Alberti, A. (2016). Oxidative stress, d-ROMs test, and ceruloplasmin. Free Radic. Res. 50, 447–453. doi: 10.3109/10715762.2015.1136063
Cooper-Mullin, C., and McWilliams, S. R. (2016). The role of the antioxidant system during intense endurance exercise: lessons from migrating birds. J. Exp. Biol. 219, 3684–3695. doi: 10.1242/jeb.123992
Cooper-Mullin, C., and McWilliams, S. R. (2021). Fat stores and antioxidant capacity affect stopover duration decisions in migratory passerines: an experimental approach. Front. Ecol. Evol. (in press)
Cooper-Mullin, C., Carter, W. A., Amato, R. S., Podlesak, D., and McWilliams, S. R. (2021). Dietary vitamin E reaches the mitochondria in the flight muscle of zebra finches but only if they exercise. PLoS One 16:e0253264. doi: 10.1371/journal.pone.0253264
Cooper-Mullin, C., Carter, W. A., and McWilliams, S. R. (2019). Acute effects of intense exercise on the antioxidant system in birds: does exercise training help? J. Exp. Biol. 222:jeb210443.
Corder, K. R., DeMoranville, K. J., Russell, D. E., Huss, J. M., and Schaeffer, P. J. (2016). Annual life-stage regulation of lipid metabolism and storage and association with PPARs in a migrant species: the gray catbird (Dumetella carolinensis). J. Exp. Biol. 219, 3391–3398.
Costantini, D. (2011). On the measurement of circulating antioxidant capacity and the nightmare of uric acid. Methods Ecol. Evol. 2, 321–325. doi: 10.1111/j.2041-210X.2010.00080.x
Costantini, D. (2014). Oxidative Stress And Hormesis In Evolutionary Ecology And Physiology: A Marriage Between Mechanistic And Evolutionary Approaches. Heidelberg: Springer-Verlag.
Costantini, D. (2016). Oxidative stress ecology and the d-ROMs test: facts, misfacts and an appraisal of a decade’s work. Behav. Ecol. Sociobiol. 70, 809–820. doi: 10.1007/s00265-016-2091-5
Costantini, D. (2019). Understanding diversity in oxidative status and oxidative stress: the opportunities and challenges ahead. J. Exp. Biol. 222:jeb194688. doi: 10.1242/jeb.194688
Costantini, D., Cardinale, M., and Carere, C. (2007). Oxidative damage and anti-oxidant capacity in two migratory bird species at a stop-over site. Comp. Biochem. Physiol. C Toxicol. Pharmacol. 144, 363–371. doi: 10.1016/j.cbpc.2006.11.005
Costantini, D., Dell’Ariccia, G., and Lipp, H.-P. (2008). Long flights and age affect oxidative status of homing pigeons (Columba livia). J. Exp. Biol. 211, 377–381. doi: 10.1242/jeb.012856
Costantini, D., Lindecke, O., Pçtersons, G., and Voigt, C. C. (2018). Migratory flight imposes oxidative stress in bats. Curr. Zool. 65, 147–153. doi: 10.1093/cz/zoy039
Costantini, D., Monaghan, P., and Metcalfe, N. B. (2013). Loss of integration is associated with reduced resistance to oxidative stress. J. Exp. Biol. 216, 2213–2220. doi: 10.1242/jeb.083154
Davies, M. J. (2016). Protein oxidation and peroxidation. Biochem. J. 473, 805–825. doi: 10.1042/bj20151227
DeMoranville, K. J. (2020). The Frequent Flyer’s Guide to Migration: How Songbird Metabolism and Oxidative Status Respond to Endurance Flight and Diet Quality. Ph.D. Dissertation. Kingston, RI: University of Rhode Island.
DeMoranville, K. J., Carter, W. A., Pierce, B. J., and McWilliams, S. R. (2020). Flight training in a migratory bird drives metabolic gene expression in the flight muscle but not liver, and dietary fat quality influences select genes. Am. J. Physiol. Regul. Integr. Comp. Physiol. 319, R637–R652. doi: 10.1152/ajpregu.00163.2020
DeMoranville, K. J., Carter, W. A., Pierce, B. J., and McWilliams, S. R. (2021). Flight and dietary antioxidants influence antioxidant expression and activity in a migratory bird. Integr. Org. Biol. (in press)
DeMoranville, K. J., Corder, K. R., Hamilton, A., Russell, D. E., Huss, J. M., and Schaeffer, P. J. (2019). PPAR expression, muscle size, and metabolic rates across the Gray catbird’s annual cycle are greatest in preparation for fall migration. J. Exp. Biol. 45056:jeb198028. doi: 10.1242/jeb.198028
Dick, M. F., and Guglielmo, C. G. (2019). Flight muscle protein damage during endurance flight is related to energy expenditure but not dietary polyunsaturated fatty acids in a migratory bird. J. Exp. Biol. 222(Pt 5):jeb187708. doi: 10.1242/jeb.187708
Dietz, M. W., Piersma, T. T., and Dekinga, A. (1999). Body-building without power training: endogenously regulated pectoral muscle hypertrophy in confined shorebirds. J. Exp. Biol. 202, 2831–2837. doi: 10.1242/jeb.202.20.2831
Dorr, B. S., Hanson-Dorr, K. C., Assadi-Porter, F. M., Selen, E. S., Healy, K. A., and Horak, K. E. (2019). Effects of repeated sublethal external exposure to deep water horizon oil on the avian metabolome. Sci. Rep. 9, 1–13. doi: 10.1002/9781119027928.ch1
Dröge, W. (2002). Free radicals in the physiological control of cell function. Physiol. Rev. 82, 47–95. doi: 10.1152/physrev.00018.2001
Eeva, T., Helle, S., Salminen, J. P., and Hakkarainen, H. (2010). Carotenoid composition of invertebrates consumed by two insectivorous bird species. J. Chem. Ecol. 36, 608–613. doi: 10.1007/s10886-010-9796-0
Eikenaar, C., Hegemann, A., Packmor, F., Kleudgen, I., and Isaksson, C. (2020a). Not just fuel: energy stores are correlated with immune function and oxidative damage in a long-distance migrant. Curr. Zool. 66, 21–28. doi: 10.1093/cz/zoz009
Eikenaar, C., Isaksson, C., and Hegemann, A. (2018). A hidden cost of migration? Innate immune function versus antioxidant defense. Ecol. Evol. 8, 2721–2728. doi: 10.1002/ece3.3756
Eikenaar, C., Jönsson, J., Fritzsch, A., Wang, H. L., and Isaksson, C. (2016). Migratory refueling affects non-enzymatic antioxidant capacity, but does not increase lipid peroxidation. Physiol. Behav. 158, 26–32. doi: 10.1016/j.physbeh.2016.02.033
Eikenaar, C., Källstig, E., Andersson, M. N., Herrera-Dueñas, A., and Isaksson, C. (2017). Oxidative challenges of avian migration: a comparative field study on a partial migrant. Physiol. Biochem. Zool. 90, 223–229. doi: 10.1086/689191
Eikenaar, C., Winslott, E., Hessler, S., and Isaksson, C. (2020b). Oxidative damage to lipids is rapidly reduced during migratory stopovers. Funct. Ecol. 34, 1215–1222. doi: 10.1111/1365-2435.13540
Ferretti, A., Maggini, I., Lupi, S., Cardinale, M., and Fusani, L. (2019). The amount of available food affects diurnal locomotor activity in migratory songbirds during stopover. Sci. Rep. 9:19027. doi: 10.1038/s41598-019-55404-3
Finck, B. N., and Kelly, D. P. (2006). PGC-1 coactivators: inducible regulators of energy metabolism in health and disease. J. Clin. Invest. 116, 615–622. doi: 10.1172/JCI27794
Frawley, A. E., DeMoranville, K. J., Carbeck, K. M., Trost, L., Bryła, A., Dzialo, M., et al. (2021). Season, anthocyanin supplementation, and flight training have mixed effects on the antioxidant system of migratory European Starlings. Ornithology 138, 1–16. doi: 10.1093/ornithology/ukab023
Fusani, L., Cardinale, M., Carere, C., and Goymann, W. (2009). Stopover decision during migration: physiological conditions predict nocturnal restlessness in wild passerines. Biol. Lett. 5, 302–305. doi: 10.1098/rsbl.2008.0755
Gannes, L. Z., Hatch, K. A., and Pinshow, B. (2001). How does time since feeding affect the fuels pigeons use during flight? Physiol. Biochem. Zool. 74, 1–10. doi: 10.1086/319315
Goymann, W., Spina, F., Ferri, A., and Fusani, L. (2010). Body fat influences departure from stopover sites in migratory birds: evidence from whole-island telemetry. Biol. Lett. 6, 478–481. doi: 10.1098/rsbl.2009.1028
Griego, M., Desimone, J., Ramirez, M. G., and Gerson, A. R. (2021). Aminopeptidase-N modulation assists lean mass anabolism during refuelling in the white-throated sparrow. Proc. R. Soc. B 288:20202348. doi: 10.1098/rspb.2020.2348
Guglielmo, C. G. (2018). Obese super athletes: fat-fueled migration in birds and bats. J. Exp. Biol. 221:jeb165753. doi: 10.1242/jeb.165753
Guglielmo, C. G., Haunerland, N. H., Hochachka, P. W., and Williams, T. D. (2002). Seasonal dynamics of flight muscle fatty acid binding protein and catabolic enzymes in a migratory shorebird. Am. J. Physiol. Regul. Integr. Comp. Physiol. 282, R1405–R1413. doi: 10.1152/ajpregu.00267.2001
Guglielmo, C. G., Piersma, T., and Williams, T. D. (2001). A sport-physiological perspective on bird migration: evidence for flight-induced muscle damage. J. Exp. Biol. 2690, 2683–2690. doi: 10.1242/jeb.204.15.2683
Guillemette, M., Richman, S. E., Portugal, S. J., and Butler, P. J. (2012). Behavioural compensation reduces energy expenditure during migration hyperphagia in a large bird. Funct. Ecol. 26, 876–883. doi: 10.1111/j.1365-2435.2012.01993.x
Gutiérrez, J. S., Sabat, P., Castañeda, L. E., Contreras, C., Navarrete, L., Peña-Villalobos, I., et al. (2019). Oxidative status and metabolic profile in a long-lived bird preparing for extreme endurance migration. Sci. Rep. 9:17616. doi: 10.1038/s41598-019-54057-6
Halliwell, B., and Gutteridge, J. (2007). Free Radicals in Biology and Medicine. Oxford: Oxford University Press.
Hou, L., and Welch, K. C. (2016). Premigratory ruby-throated hummingbirds, Archilochus colubris, exhibit multiple strategies for fuelling migration. Anim. Behav. 121, 87–99. doi: 10.1016/j.anbehav.2016.08.019
Hulbert, A. J., Pamploa, R., Buffenstein, R., and Buttemer, W. A. (2007). Life and death: metabolic rate, membrane composition, and life span of animals. Physiol. Rev. 87, 1175–1213. doi: 10.1152/physrev.00047.2006
Hutton, P., and McGraw, K. J. (2016). Urban impacts on oxidative balance and animal signals. Front. Ecol. Evol. 4:54. doi: 10.3389/fevo.2016.00054
Ito, F., Ito, T., Suzuki, C., Yahata, T., Ikeda, K., and Hamaoka, K. (2017). The application of a modified d-ROMs test for measurement of oxidative stress and oxidized high-density lipoprotein. Int. J. Mol. Sci. 18:454. doi: 10.3390/ijms18020454
Jenni, L. L., and Schaub, M. (2003). “Behavioural and physiological reactions to environmental variation in bird migration: a review,” in Avian Migration, eds P. Berthold, E. Gwinner, and E. Sonnenschein (Berlin: Springer).
Jenni, L., and Jenni-Eiermann, S. S. (1998). Fuel supply and metabolic constraints in migrating birds. J. Avian Biol. 29, 521–528. doi: 10.2307/3677171
Jenni-Eiermann, S. (2017). Energy metabolism during endurance flight and the post-flight recovery phase. J. Comp. Physiol. A 203, 431–438. doi: 10.1007/s00359-017-1150-3
Jenni-Eiermann, S., and Jenni, L. (1991). Metabolic responses to flight and fasting in night-migrating passerines. J. Comp. Physiol. B 161, 465–474. doi: 10.1007/BF00257901
Jenni-Eiermann, S., Jenni, L., Smith, S., and Costantini, D. (2014). Oxidative stress in endurance flight: an unconsidered factor in bird migration. PLoS One 9:e97650. doi: 10.1371/journal.pone.0097650
Ji, L. L., Gomez-Cabrera, M. C., and Vina, J. (2006). Exercise and hormesis: activation of cellular antioxidant signaling pathway. Ann. N. Y. Acad. Sci. 1067, 425–435. doi: 10.1196/annals.1354.061
Jimenez, A. G. (2018). “The same thing that makes you live can kill you in the end”: exploring the effects of growth rates and longevity on cellular metabolic rates and oxidative stress in mammals and birds. Integr. Comp. Biol. 58, 544–548. doi: 10.1093/icb/icy090
Jimenez, A. G., O’Connor, E. S., Tobin, K. J., Anderson, K. N., Winward, J. D., Fleming, A., et al. (2019). Does cellular metabolism from primary fibroblasts and oxidative stress in blood differ between mammals and birds? The (lack-thereof) scaling of oxidative stress. Integr. Comp. Biol. 59, 953–969. doi: 10.1093/icb/icz017
King, J. R., and Farner, D. S. (1965). Studies of fat deposition in migratory birds. Ann. N. Y. Acad. Sci. 131, 422–440. doi: 10.1111/j.1749-6632.1965.tb34808.x
Larcombe, S. D., Tregaskes, C. A., Coffey, J. S., Stevenson, A. E., Alexander, L., and Arnold, K. E. (2008). The effects of short-term antioxidant supplementation on oxidative stress and flight performance in adult budgerigars Melopsittacus undulatus. J. Exp. Biol. 211, 2859–2864. doi: 10.1242/jeb.017970
Larcombe, S. D., Tregaskes, C. A., Coffey, J., Stevenson, A. E., Alexander, L. G., and Arnold, K. E. (2015). Oxidative stress, activity behaviour and body mass in captive parrots. Conserv. Physiol. 3, 1–10. doi: 10.1093/conphys/cov045
Lindström, A., Kvist, A., Piersma, T., Dekinga, A., and Dietz, M. W. (2000). Avian pectoral muscle size rapidly tracks body mass changes during flight, fasting and fueling. J. Exp. Biol. 203, 913–919. doi: 10.1242/jeb.203.5.913
Loughland, I., and Seebacher, F. (2020). Differences in oxidative status explain variation in thermal acclimation capacity between individual mosquitofish (Gambusia holbrooki). Funct. Ecol. 34, 1380–1390. doi: 10.1111/1365-2435.13563
Marasco, V., Sebastiano, M., Costantini, D., Pola, G., and Fusani, L. (2021). Controlled expression of the migratory phenotype affects oxidative status in birds. J. Exp. Biol. 224:jeb233486. doi: 10.1242/jeb.233486
Marsh, R. L. (1984). Adaptations of the gray catbird dumetella carolinensis to long-distance migration: flight muscle hypertrophy associated with elevated body mass. Physiol. Zool. 57, 105–117. doi: 10.1086/physzool.57.1.30155973
McClelland, G. B. (2004). Fat to the fire: the regulation of lipid oxidation with exercise and environmental stress. Comp. Biochem. Physiol. B Biochem. Mol. Biol. 139, 443–460. doi: 10.1016/j.cbpc.2004.07.003
McFarlan, J. T., Bonen, A., and Guglielmo, C. G. (2009). Seasonal upregulation of fatty acid transporters in flight muscles of migratory white-throated sparrows (Zonotrichia albicollis). J. Exp. Biol. 212, 2934–2940. doi: 10.1242/jeb.031682
McWilliams, S. R., and Karasov, W. H. (2014). Spare capacity and phenotypic flexibility in the digestive system of a migratory bird: defining the limits of animal design. Proc. R. Soc. B 281:20140308. doi: 10.1098/rspb.2014.0308
McWilliams, S. R., Guglielmo, C., Pierce, B., and Klaassen, M. (2004). Flying, fasting, and feeding in birds during migration: a nutritional and physiological ecology perspective. J. Avian Biol. 35, 377–393. doi: 10.1111/j.0908-8857.2004.03378.x
McWilliams, S. R., Ramenofsky, M., and Pierce, B. J. (2021). “Physiological challenges of migration,” in Sturkie’s Avian Physiology, eds C. G. Scanes and S. Dridi (Cambridge, MA: Academic Press).
McWilliams, S., Pierce, B., Wittenzellner, A., Langlois, L., Engel, S., Speakman, J. R., et al. (2020). The energy savings-oxidative cost trade-off for migratory birds during endurance flight. eLife 9:e60626. doi: 10.7554/eLife.60626
Metzger, B. J., and Bairlein, F. (2011). Fat stores in a migratory bird: a reservoir of carotenoid pigments for times of need? J. Comp. Physiol. B 181, 269–275. doi: 10.1007/s00360-010-0511-9
Montgomery, M. K., Buttemer, W. A., and Hulbert, A. J. (2012). Does the oxidative stress theory of aging explain longevity differences in birds? II. Antioxidant systems and oxidative damage. Exp. Gerontol. 47, 211–222. doi: 10.1016/j.exger.2011.11.014
Munshi-South, J., and Wilkinson, G. S. (2010). Bats and birds: exceptional longevity despite high metabolic rates. Ageing Res. Rev. 9, 12–19. doi: 10.1016/j.arr.2009.07.006
Nikolaidis, M. G., Kyparos, A., Spanou, C., Paschalis, V., Theodorou, A. A., and Vrabas, I. S. (2012). Redox biology of exercise: an integrative and comparative consideration of some overlooked issues. J. Exp. Biol. 215, 1615–1625. doi: 10.1242/jeb.067470
Nudds, R. L., and Bryant, D. M. (2001). Exercise training lowers the resting metabolic rate of zebra finches, Taeniopygia guttata. Funct. Ecol. 15, 458–464. doi: 10.1046/j.0269-8463.2001.00546.x
O’Neal, B. J., Stafford, J. D., Larkin, R. P., and Michel, E. S. (2018). The effect of weather on the decision to migrate from stopover sites by autumn-migrating ducks. Mov. Ecol. 6, 1–10. doi: 10.1186/s40462-018-0141-5
Parrish, J. D. (1997). Patterns of frugivory and energetic condition in Nearctic landbirds during autumn migration. Condor 99, 681–697. doi: 10.2307/1370480
Pierce, B. J., and McWilliams, S. R. (2005). Seasonal changes in composition of lipid stores in migratory birds: causes and consequences. Condor 107:269. doi: 10.1650/7809
Pierce, B. J., and McWilliams, S. R. (2014). The fat of the matter: how dietary fatty acids can affect exercise performance. Integr. Comp. Biol. 54, 903–912. doi: 10.1093/icb/icu098
Piersma, T., Gudmundsson, G. A., and Lilliendahl, K. (1999). Rapid changes in the size of different functional organ and muscle groups during refueling in a long-distance migrating shorebird. Physiol. Biochem. Zool. 72, 405–415. doi: 10.1086/316680
Price, E. R. (2010). Dietary lipid composition and avian migratory flight performance: development of a theoretical framework for avian fat storage. Comp. Biochem. Physiol. Mol. Integr. Physiol. 157, 297–309. doi: 10.1016/j.cbpa.2010.05.019
Pritsos, K. L., Perez, C. R., Muthumalage, T., Dean, K. M., Cacela, D., Hanson-Dorr, K., et al. (2017). Dietary intake of Deepwater Horizon oil-injected live food fish by double-crested cormorants resulted in oxidative stress. Ecotoxicol. Environ. Saf. 146, 62–67. doi: 10.1016/j.ecoenv.2017.06.067
Ristow, M., Zarse, K., Oberbach, A., Klöting, N., Birringer, M., Kiehntopf, M., et al. (2009). Antioxidants prevent health-promoting effects of physical exercise in humans. Proc. Natl. Acad. Sci. U.S.A. 2, 2–7. doi: 10.1073/pnas.0903485106
Rybczynski, R., and Riker, D. K. (1981). A temperate species-rich assemblage of migrant frugivorous birds. Auk 98, 176–179.
Sapir, N., Abramsky, Z., Shochat, E., and Izhaki, I. (2004). Scale-dependent habitat selection in migratory frugivorous passerines. Naturwissenschaften 91, 544–547. doi: 10.1007/s00114-004-0564-2
Schaefer, H. M., McGraw, K., and Catoni, C. (2008). Birds use fruit colour as honest signal of dietary antioxidant rewards. Funct. Ecol. 22, 303–310. doi: 10.1111/j.1365-2435.2007.01363.x
Schaefer, H. M., Valido, A., and Jordano, P. (2014). Birds see the true colours of fruits to live off the fat of the land. Proc. R. Soc. B 281:20132516. doi: 10.1098/rspb.2013.2516
Schaub, M., and Jenni, L. (2001). Stopover durations of three warbler species along their autumn migration route. Oecologia 128, 217–227. doi: 10.1007/s004420100654
Selman, C., Blount, J. D., Nussey, D. H., and Speakman, J. R. (2012). Oxidative damage, ageing, and life-history evolution: where now? Trends Ecol. Evol. 27, 570–577. doi: 10.1016/j.tree.2012.06.006
Shih, P. H., Yeh, C. T., and Yen, G. C. (2007). Anthocyanins induce the activation of phase II enzymes through the antioxidant response element pathway against oxidative stress-induced apoptosis. J. Agric. Food Chem. 55, 9427–9435. doi: 10.1021/jf071933i
Sindler, A. L., Reyes, R., Chen, B., Ghosh, P., Gurovich, A. N., Kang, L. S., et al. (2013). Age and exercise training alter signaling through reactive oxygen species in the endothelium of skeletal muscle arterioles. J. Appl. Physiol. 114, 681–693. doi: 10.1152/japplphysiol.00341.2012
Skrip, M. M., and McWilliams, S. R. (2016). Oxidative balance in birds: an atoms-to-organisms-to-ecology primer for ornithologists. J. Field Ornithol. 87, 1–20. doi: 10.1111/jofo.12135
Skrip, M. M., Bauchinger, U., Goymann, W., Fusani, L., Cardinale, M., Alan, R. R., et al. (2015). Migrating songbirds on stopover prepare for, and recover from, oxidative challenges posed by long-distance flight. Ecol. Evol. 5, 3198–3209. doi: 10.1002/ece3.1601
Skrip, M. M., Seeram, N. P., Yuan, T., Ma, H., and McWilliams, S. R. (2016). Dietary antioxidants and flight exercise in female birds affect allocation of nutrients to eggs: how carry-over effects work. J. Exp. Biol. 219, 2716–2725. doi: 10.1242/jeb.137802
Smith, S. B., McPherson, K. H., Backer, J. M., Pierce, B. J., Podlesak, D. W., and Mcwilliams, S. R. (2007). Fruit quality and consumption by songbirds during autumn migration. Wilson J. Ornithol. 119, 419–428. doi: 10.1676/06-073.1
Smith, S. B., Miller, A. C., Merchant, C. R., and Sankoh, A. F. (2015). Local site variation in stopover physiology of migrating songbirds near the south shore of Lake Ontario is linked to fruit availability and quality. Conserv. Physiol. 3, 1–14. doi: 10.1093/conphys/cov036
Sthijns, M. M. J. P. E., Weseler, A. R., Bast, A., and Haenen, G. R. M. M. (2016). Time in redox adaptation processes: from evolution to hormesis. Int. J. Mol. Sci. 17:1649. doi: 10.3390/ijms17101649
Stier, A., Bize, P., Hsu, B. Y., and Ruuskanen, S. (2019). Plastic but repeatable: Rapid adjustments of mitochondrial function and density during reproduction in a wild bird species. Biol. Lett. 15, 9–13. doi: 10.1098/rsbl.2019.0536
Syeda, M. Z., Fasae, M. B., Yue, E., Ishimwe, A. P., Jiang, Y., Du, Z., et al. (2019). Anthocyanidin attenuates myocardial ischemia induced injury via inhibition of ROS-JNK-Bcl-2 pathway: new mechanism of anthocyanidin action. Phytother. Res. 33, 3129–3139. doi: 10.1002/ptr.6485
Tian, X., Xin, H., Paengkoum, P., Paengkoum, S., Ban, C., and Sorasak, T. (2019). Effects of anthocyanin-rich purple corn (Zea mays L.) stover silage on nutrient utilization, rumen fermentation, plasma antioxidant capacity, and mammary gland gene expression in dairy goats. J. Anim. Sci. 97, 1384–1397. doi: 10.1093/jas/sky477
Tretter, L., and Adam-Vizi, V. (2005). Alpha-ketoglutarate dehydrogenase: a target and generator of oxidative stress. Philos. Trans. R. Soc. B 360, 2335–2345. doi: 10.1098/rstb.2005.1764
Trevelline, B. K., Fontaine, S. S., Hartup, B. K., and Kohl, K. D. (2019). Conservation biology needs a microbial renaissance: a call for the consideration of host-associated microbiota in wildlife management practices. Proc. R. Soc. B 286:20182448. doi: 10.1098/rspb.2018.2448
Tsahar, E., Arad, Z., Izhaki, I., and Guglielmo, C. G. (2006). The relationship between uric acid and its oxidative product allantoin: a potential indicator for the evaluation of oxidative stress in birds. J. Comp. Physiol. B 176, 653–661. doi: 10.1007/s00360-006-0088-5
Vaugoyeau, M., Decencière, B., Perret, S., Karadas, F., Meylan, S., and Biard, C. (2015). Is oxidative status influenced by dietary carotenoid and physical activity after moult in the great tit (Parus major)? J. Exp. Biol. 218: 2106–2115. doi: 10.1242/jeb.111039
Weber, J.-M. (2011). Metabolic fuels: regulating fluxes to select mix. J. Exp. Biol. 214, 286–294. doi: 10.1242/jeb.047050
Wojciechowski, M. S., and Pinshow, B. (2009). Heterothermy in small, migrating passerine birds during stopover: use of hypothermia at rest accelerates fuel accumulation. J. Exp. Biol. 212, 3068–3075. doi: 10.1242/jeb.033001
Zhang, Y., King, M. O., Harmon, E., Eyster, K., and Swanson, D. L. (2015). Migration-induced variation of fatty acid transporters and cellular metabolic intensity in passerine birds. J. Comp. Physiol. B Biochem. Syst. Environ. Physiol. 185, 797–810. doi: 10.1007/s00360-015-0921-9
Keywords: oxidative balance, bird migration, antioxidants, ecophysiological plasticity, exercise physiology
Citation: McWilliams S, Carter W, Cooper-Mullin C, DeMoranville K, Frawley A, Pierce B and Skrip M (2021) How Birds During Migration Maintain (Oxidative) Balance. Front. Ecol. Evol. 9:742642. doi: 10.3389/fevo.2021.742642
Received: 16 July 2021; Accepted: 06 October 2021;
Published: 26 October 2021.
Edited by:
Ivan Maggini, University of Veterinary Medicine Vienna, AustriaReviewed by:
Cas Eikenaar, Institute of Avian Research, GermanyCopyright © 2021 McWilliams, Carter, Cooper-Mullin, DeMoranville, Frawley, Pierce and Skrip. This is an open-access article distributed under the terms of the Creative Commons Attribution License (CC BY). The use, distribution or reproduction in other forums is permitted, provided the original author(s) and the copyright owner(s) are credited and that the original publication in this journal is cited, in accordance with accepted academic practice. No use, distribution or reproduction is permitted which does not comply with these terms.
*Correspondence: Scott McWilliams, c3JtY3dpbGxpYW1zQHVyaS5lZHU=
Disclaimer: All claims expressed in this article are solely those of the authors and do not necessarily represent those of their affiliated organizations, or those of the publisher, the editors and the reviewers. Any product that may be evaluated in this article or claim that may be made by its manufacturer is not guaranteed or endorsed by the publisher.
Research integrity at Frontiers
Learn more about the work of our research integrity team to safeguard the quality of each article we publish.