- 1Nicholas School of the Environment, Duke University, Durham, NC, United States
- 2Department of Ecology and Evolutionary Biology, Yale University, New Haven, CT, United States
- 3USFS Forest Inventory and Analysis, Durham, NH, United States
- 4Univ. Grenoble Alpes, INRAE, LESSEM, St-Martin-d'Heres, France
Anticipating the next generation of forests requires understanding of recruitment responses to habitat change. Tree distribution and abundance depend not only on climate, but also on habitat variables, such as soils and drainage, and on competition beneath a shaded canopy. Recent analyses show that North American tree species are migrating in response to climate change, which is exposing each population to novel climate-habitat interactions (CHI). Because CHI have not been estimated for either adult trees or regeneration (recruits per year per adult basal area), we cannot evaluate migration potential into the future. Using the Masting Inference and Forecasting (MASTIF) network of tree fecundity and new continent-wide observations of tree recruitment, we quantify impacts for redistribution across life stages from adults to fecundity to recruitment. We jointly modeled response of adult abundance and recruitment rate to climate/habitat conditions, combined with fecundity sensitivity, to evaluate if shifting CHI explain community reorganization. To compare climate effects with tree fecundity, which is estimated from trees and thus is "conditional" on tree presence, we demonstrate how to quantify this conditional status for regeneration. We found that fecundity was regulated by temperature to a greater degree than other stages, yet exhibited limited responses to moisture deficit. Recruitment rate expressed strong sensitivities to CHI, more like adults than fecundity, but still with substantial differences. Communities reorganized from adults to fecundity, but there was a re-coalescence of groups as seedling recruitment partially reverted to community structure similar to that of adults. Results provide the first estimates of continent-wide community sensitivity and their implications for reorganization across three life-history stages under climate change.
Introduction
Extensive climate changes in North America since forest stands established over the last few centuries may have changed where tree species can recruit in the aftermath of recent diebacks (Ibáñez et al., 2007; Woodall et al., 2009; Bell et al., 2014; Serra-Diaz et al., 2016; Davis et al., 2019). The notion of a species' niche (Hutchinson, 1957; Austin, 2002), typically quantified with models for adult distribution and abundance explained by climate and habitat variables, may represent past conditions, when current stands were established. The regeneration niche recognizes that conditions affecting fecundity and seedling establishment can differ from adults (Grubb, 1977; Clark et al., 1998; Ibanez et al., 2006; Engler et al., 2009; Swab et al., 2012). Accumulating climate changes can progressively distance the habitats where regeneration can occur from current distributions of mature trees (Sharma et al., 2021). If climate effects depend on soils, drainage, and biotic variables, then climate-habitat interactions (CHI) will complicate responses (Ibanez et al., 2009; Clark et al., 2016; Serra-Diaz et al., 2016). The emerging misalignment of adults and their recruits will be especially severe if recruitment stages are most sensitive to climate (Sharma et al., 2021). Related concepts like a species' climate envelop (Pearson and Dawson, 2003; Thomas et al., 2004) or suitable habitat (Freckleton and Watkinson, 2002; Iverson et al., 2008), quantified with adult trees and current conditions, may not align with the conditions for seed production and seedling recruitment (Ibanez et al., 2006; Clark et al., 2011). In this paper we show that the differences in niche requirements quantified from adult distributions can diverge from those for fecundity and seedling recruitment, with important implications for responses to contemporary climate change.
Contemporary forest composition is determined by seed availability and recruitment success in the past. Both fecundity and seedling success could be sensitive to climate-habitat interactions (CHI) in ways that are not apparent from studies on adult physiology. In North America, fecundity is highest in warm, moist climates of the south-central continent (Clark et al., 2021). Seedling recruitment is accelerated by temperature in early-successional environments (Fridley and Wright, 2018), and seedlings could be more sensitive to climate change than are adult survival and seed production (García-Camacho et al., 2010; Walck et al., 2011). Recruitment responses to temperature depend on water availability, which might limit upslope forest range expansion (Lenoir et al., 2010; Crimmins et al., 2011; Kueppers et al., 2017). Local moisture gradients controlled by drainage and soil type interact with moisture deficits (Ibáñez et al., 2007; Clark et al., 2014; Serra-Diaz et al., 2016), amplifying the effects of aridification (Seyednasrollah and Clark, 2020). With warming over much of North America and changes in precipitation, the recruitment that follows twenty-first-century diebacks may differ from that of the past.
Under slow climate change (Dawson et al., 2019), species composition is expected to integrate the fitness contributions of fecundity, seedling success, and adult survival, each of which might respond to CHI in its own way. Species with similar responses to climate and habitat that occur together in communities now, may respond differently to future environmental change. The similarities between species might typically be inferred from their tendencies to co-occur in the habitats where mature individuals are most abundant. However, the many ways in which species may differ in the responses at each life stage could result in novel communities as suggested by no-analog communities in the past (Williams and Jackson, 2007). If contemporary climate changes have effects that differ by recruitment stage, then biodiversity projections require an understanding of CHI not only on adult distributions, but also for fecundity and seedling recruitment. This study examines how climate and habitat variables differ in their effects on seed production and seedling establishment and survival.
Comparing the habitat relationships for adults, seed production, and recruits requires comparable methods. Models for the biogeographic distribution of seeds (Clark et al., 2021; Sharma et al., 2021) are necessarily conditional on the abundance of trees that produce those seeds; we cannot know how much seed would be produced by a species where it does not grow. An unconditional estimate would require wide availability of trees planted throughout climate/habitat space. We define fecundity to be the expected annual seed mass produced by a tree of a given species, size, crowding, and habitat.
Because recruitment can only be observed near adults, it too must be quantified conditional on adult presence. Recruitment ρi refers to seedlings expected to cross a size threshold per ha per year at location i. Estimates of habitat controls on recruitment unconditional on adults would require seed availability throughout climate/habitat space; in fact, the climate/habitat space for recruitment is constrained within that already occupied by adults. Like fecundity, recruitment depends on the abundance of adults and thus must be modeled conditionally. Unlike fecundity, recruitment rates obtained from ingrowth to forest inventory plots are not explicitly referenced to adults (they are numbers per area per year) (Sharma et al., 2021), but they implicitly depend on adult presence.
For comparison with fecundity, the conditional model for recruitment dependent both on adult abundance a and climate/habitat is given by [ρ|a, x], where the bracket notation indicates a distribution of recruitment rates ρ conditional on adults a and climate/habitat x. To facilitate conditional analysis, these effects might be separable in a model, [ρ|g(a) + h(x)], where g(·) and h(·) are functions. Unfortunately, adults cannot be used as predictors of recruitment, because both are random–they both depend on environment, and they are both encountered as random variables when inventory plots are sampled. Clearly, the comparison of fecundity and recruitment requires joint analysis of recruits and adults [ρ, a|x] = [y|x], where y is a vector holding recruitment and adult abundances of all species, followed by conditioning on adults and environment, [ρ|a, x].
Using conditional models that allow us to compare the communities represented by joint fecundity and recruitment responses we expose a reorganization across life stages that will contribute to the next generation of forests. Changing niche space is summarized by the ways in which species reorganize in their responses from adults to fecundity to recruitment and by the CHI that are responsible for those changes.
Materials and Methods
Our goal is to jointly model recruits and adults of all species, thereby accommodating their mutual dependence structure, but then to isolate the effects of adults from direct CHI effects through conditioning. This conditional recruitment can then be compared with fecundity, which is necessarily conditional on the trees producing seeds. The fitted model provides a basis for defining communities in terms of the responses of each stage to CHI. The reorganization of these communities across life history stages is the basis for interpreting potential forest changes that account for recruitment responses.
Theoretical Development
Consider trees that occupy inventory plots sampled at intervals during which adults change in abundance due to survival of previously measured trees and ingrowth of new individuals that cross the minimum size threshold. Population growth rate can be expressed in terms of recruitment (per ha per yr) per adult abundance,
where rs is number of seedlings per ha of species s, t represents time (in years), and as indicates adult basal area (location i is suppressed to reduce clutter). Because it integrates information on size and numbers, adult abundance is typically expressed as basal area (m2 ha−1). Recruitment is expressed as seedlings per ha per year, obtained from the ingrowth number, plot area, and sample interval of FIA (Sharma et al., 2021). It is divided by adult basal area following Equation 1 to generate seedlings per adult basal area per year (m−2 year−1). Due to the small size of inventory plots, both tree basal area and recruitment are noisy variables. Recruits depend not only on adult abundances of the same species, but also on adults and recruits of other species (Zhu et al., 2015).
Generalized Joint Attribution Modeling (GJAM) allows us to jointly quantify CHI effects on both adults and recruitment due to its allowance for different data types and the dominance of zero values–most species are absent from most observations (Clark et al., 2017). From the fitted joint model we obtain a conditional distribution that isolates effects of CHI from adults, which can then be compared between species and with responses of tree abundance and fecundity.
Inputs to the model are predictors and responses for i = 1, …, n inventory plots. Predictors occupy the length-Q vector xi, including climate, habitat, and their interactions. Recruitment rates and adult basal areas of all S species occupy a length-2S observation vector yis consisting of S adult basal areas and S recruitment rates. Corresponding to the observation vector yis is a latent vector wi having elements wis that are equal to yis when wis ≥ 0 and negative otherwise (Clark et al., 2017). The latent w is censored at zero, allowing us to combine the continuous abundance with discrete zero values. For our application the basic GJAM model simplifies to
where is a length-2S vector holding the (uncensored, latent) abundances of species as S recruitment rates ρi (the discrete version of Equation 1) and S adult abundances ai (basal area, m2 ha−1), with mean vector μi = Bxi, and 2S × Q coefficient matrix
The two components of B describe the effects of CHI on the recruitment and adult observations. The covariance matrix takes up additional dependence between all recruits and adults. The 2S × 2S covariance can be partitioned as
This joint distribution of recruitment and adults allows us to isolate the contributions of adult abundances and CHI impacts on recruitment as a conditional distribution,
There are now two matrices of coefficients for recruitment rate, one S × S matrix for effects of adults, , and another S × Q matrix for effects of CHI, C = Bρ − ABa. Importantly, this capacity to condition on adult basal area offers an opportunity to compare the effects of CHI on seedling recruitment with that for fecundity, which, again, is necessarily conditioned on adult abundance. Following Clark et al. (2017), a joint sensitivity across all species to climate/habit covariates can be obtained using
The length-Q sensitivity vector fρ summarizes the importance of each covariate for conditional recruitment. Additionally, species correlation in response of recruitment to climate/habit can be quantified using response matrix
where V is the covariance in predictors in the design matrix. Similar species can have similar responses to environment conditions (columns in C) that can be amplified by large variation in V, and vice versa. The S × S Eρ matrix thus quantifies species correlation in their responses to climate/habitat conditions (Clark et al., 2017). Adult sensitivity fa and response matrix Ea can be quantified in the same way using
Data
Data used in this study include forest inventories (adult trees and recruits), fecundity data, soils, climate, and local habitat conditions (Table 1). Tree abundance were collected from 196,765 plots of the USDA Forest Inventory and Analysis (FIA) dataset in the United States (Gray et al., 2012), including over 4.6 million trees for 112 abundant species. Since the year 2009 (Woodall et al., 2009), FIA data that consistently samples forest trees across the demographic spectrum (seedlings to adults) and across space and time in all US forests have been used in numerous studies to examine the dynamics of range shifts (Zhu et al., 2011; Bell et al., 2014; Fei et al., 2017; Sharma et al., 2021). Basal area (m2 ha−1) of adult trees (a) and recruitment rate (ρ) of small seedlings per adult basal area per year (m−2 year−1) were evaluated using FIA. Adult tree basal area was calculated directly from diameter of individual trees. We defined numbers of recruits as live saplings with diameter between 2.5 cm and 12.7 cm and the FIA code RECONCILEDCD == 1 (i.e., an ingrowth tree) at forested and remeasured plots. Remeasured plots were identified using the Population Plot Stratum Assignment (PPSA) table (Woudenberg et al., 2010). We calculated recruitments per year by dividing the number of recruits by the sampling interval (REMPER in PPSA table) and then scaled it by the expansion factor, which depends on plot size, from the Population Stratum table (Woudenberg et al., 2010). Finally, we divided recruits per year by the adult basal area at each plot. We quantified recruitment success through recruitment rates instead of seedling abundances because seedlings are not individually tracked between censuses and thus cannot offer an estimate of rates. The K-Means algorithm (Hartigan and Wong, 1979) was used to aggregate plots on the basis of CHI and location into 1-ha clusters to increase the signal-to-noise ratio (Iverson and Prasad, 1998; Zhu et al., 2014).
Fecundity data were obtained from the Masting Inference and Forecasting (MASTIF) network (Clark et al., 2021), including 233,052 trees and 2,221,148 3-years from 149 species. Fecundity is compiled from two types of raw data, seed traps and crop counts (Clark et al., 2021). Fecundity sites are listed at the website MASTIF as detailed in Clark et al. (2019) and Clark et al. (2021).
Environmental covariates for modeling adult basal area, fecundity, and recruitment are summarized in Table 1. We obtained stand age and site moisture class from FIA field records, the latter characterized using local land form, topographical position, and soil (Woudenberg et al., 2010). Soil characteristics, including proportion of soil type (i.e., clay and sand) and cation exchange capacity (i.e., an indicator for soil fertility), were derived from Hengl et al. (2017). We calculated weighted average soil conditions based on uncertainty layers for three soil depths (i.e., 0–5 cm, 5–15 cm, and 15–30 cm). Monthly Climate data from Terraclimate (Abatzoglou et al., 2018) and CHELSA (Karger et al., 2017) were used to derive annual mean temperature and accumulated moisture deficit. Deficit was defined as the difference between potential evapotranspiration and precipitation at region scales, which differs from the moisture class that quantified plot-scale moisture availability to trees.
Analysis
Fecundity modeling required individual tree attributes, including diameter and shade class using the 5-point scale of FIA and NEON, in addition to site variables used for adults and recruits. The MASTIF model accommodates dependencies between individual trees and within trees over time using the R package MASTIF (Clark et al., 2019). Seed production for the 233,052 3-year observations in MASTIF was fitted to tree attributes and CHI.
As discussed in the section Theoretical background, adult abundances and recruitment, a and ρ, were fitted jointly in GJAM, with summaries including joint sensitivity and species correlation in response to CHI. Similar to Ea in Equation 11 and Eρ in Equation 9, the species correlation in fecundity was quantified as
where Bf is the coefficient matrix of fecundity responses of each species to each predictor in x, and Vf is the covariance of predictors. The fecundity sensitivity was quantified as
where Σf,f is the covariance matrix for fecundity.
To track community reorganization from adults to fecundity to recruits we summarized changes in the response matrix E. In this case, the community is defined in terms of species responses to predictors in the model. Hierarchical clustering was implemented on the 87 species that were included in both FIA and MASTIF sites using the R function hclust in the stats package (R Core Team, 2020). Adult abundances (Ea), conditional recruitment (Eρ), and fecundity (Ef) were clustered separately. Communities were identified by names of the most abundant species in clusters extracted from Ea. We used an alluvial diagram (Rosvall and Bergstrom, 2010) to summarize the tendency for community reorganization with climate change across the three demographic stages (i.e., adults, fecundity, and recruits).
Results
Taken over the entire map and all species, temperature is the most important source of variation in adults and conditional recruitment (black labels in Figure 1), and it is behind shade class (not shown) for fecundity (green labels in Figure 1). The other climate-related variable, moisture deficit, also plays a large role for adults and recruitment, but it contributes much less to variation in fecundity. Adult trees show higher sensitivities to soil texture (% sand and clay) and to deficit than does conditional recruitment (points are below the 1:1 line in Figure 1). Site moisture status and CEC show equivalent contributions to adults and recruitment. By contrast, temperature, stand age, and the deficit-moisture interaction have larger impacts on conditional recruitment than on adult abundance (Figure 1). While having high sensitivity to shade class and tree diameter (not shown), fecundity shows an even stronger response to temperature than do adults or recruitment (Figure 1). After temperature and individual tree attributes, soil-related variables have less effects on fecundity than recruitment and adults.
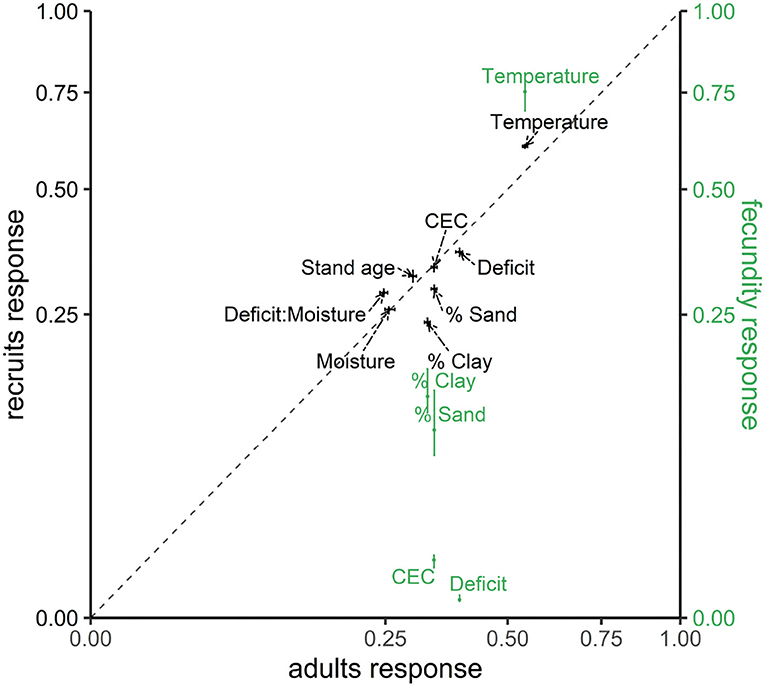
Figure 1. Joint sensitivity taken over all species for adult abundances fa, conditional recruits fρ (left y-axis with black color), and fecundity ff (right y-axis with green color). Sensitivity is shown as a fraction of the total variance explained for each variable. Posterior medians are show with 95% credible intervals (whiskers). The species-level sensitivity of fecundity to climate-habitat interactions (CHI) are detailed in Supplementary Figure S2.
The high sensitivity to temperature and then moisture deficit in Figure 1 results from large contrasts across Ba (adults) and C (conditional recruitment rate) coefficients for these variables in Figure 2. Variables that contribute small sensitivity in Figure 1 are those for which there is limited contrast in responses across species.
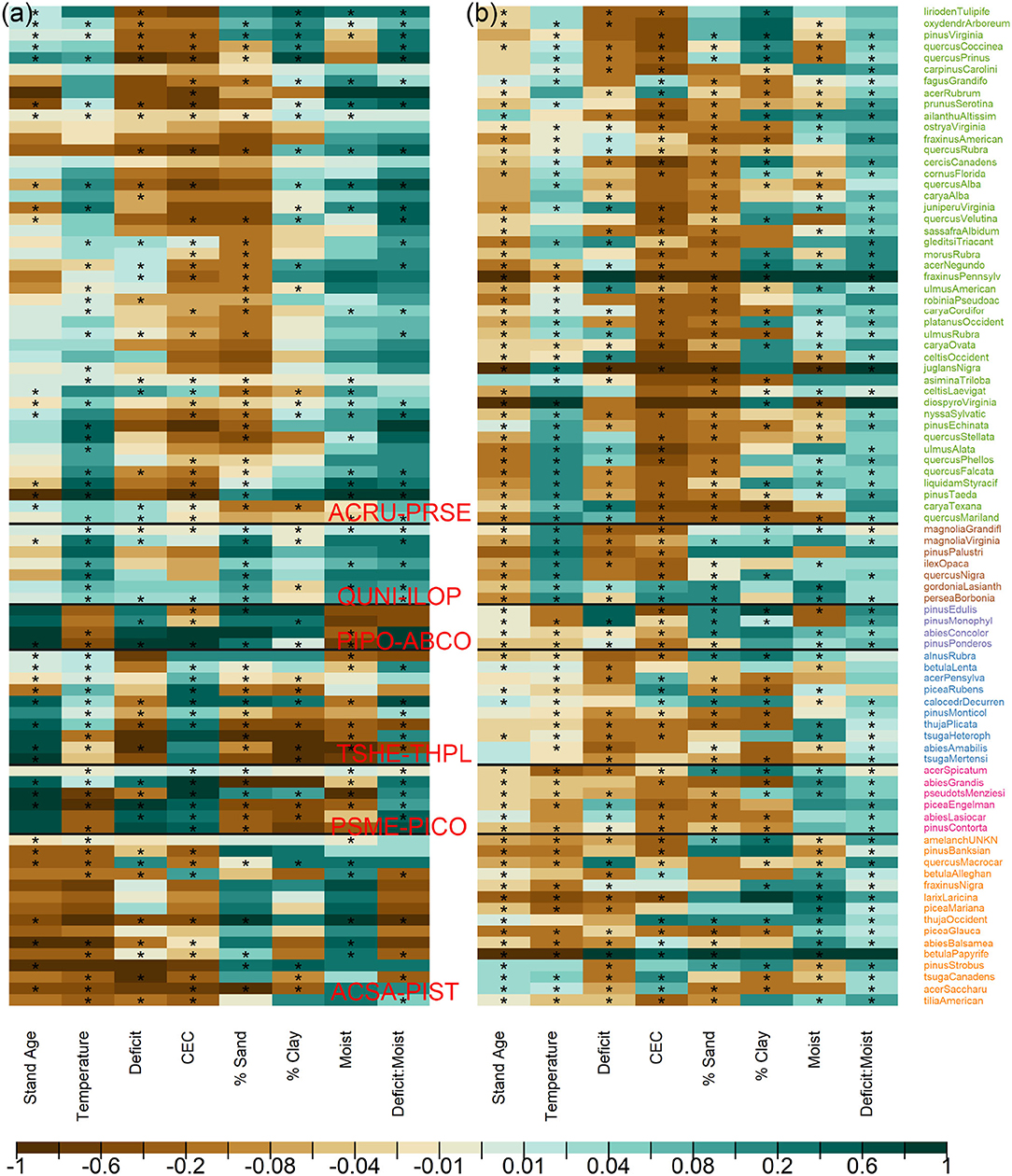
Figure 2. Species responses to climate/habitat for (a) adults in Ba and (b) conditional recruits in C. Stars indicate that the 95% credible interval does not contain zero. Colors for the species names follow adult communities in Figures 3a, 4, with horizontal lines separating clusters. Coefficient colors are scaled proportionally to the 97.5% of the estimated ranges for both adults and conditional recruits.
The similarities in Figure 2 between species define communities based on the responses to predictors that have important variation (Figure 3). For example, species in ACSA-PIST of Figure 3a share affinities for low temperatures, low CEC, and high moisture (bottom of Figure 2a). Similar affinities, but with many species-specific differences, characterize their recruitment rates (bottom of Figure 2b). Together these species differences determine the community structure in Figure 3c. Overall, adult responses in matrix Ea cluster as clear species assemblages (red blocks in Figure 3a along the diagonal) with distinct biogeographical patterns (Figure 4A). From cool-moist to warm-dry in the West are TSHE-THPL, PSME-PICO, and PIPO-ABCO. From cool to warm in the East are ACSA-PIST, ARCU-PRSE, and QUNI-ILOP.
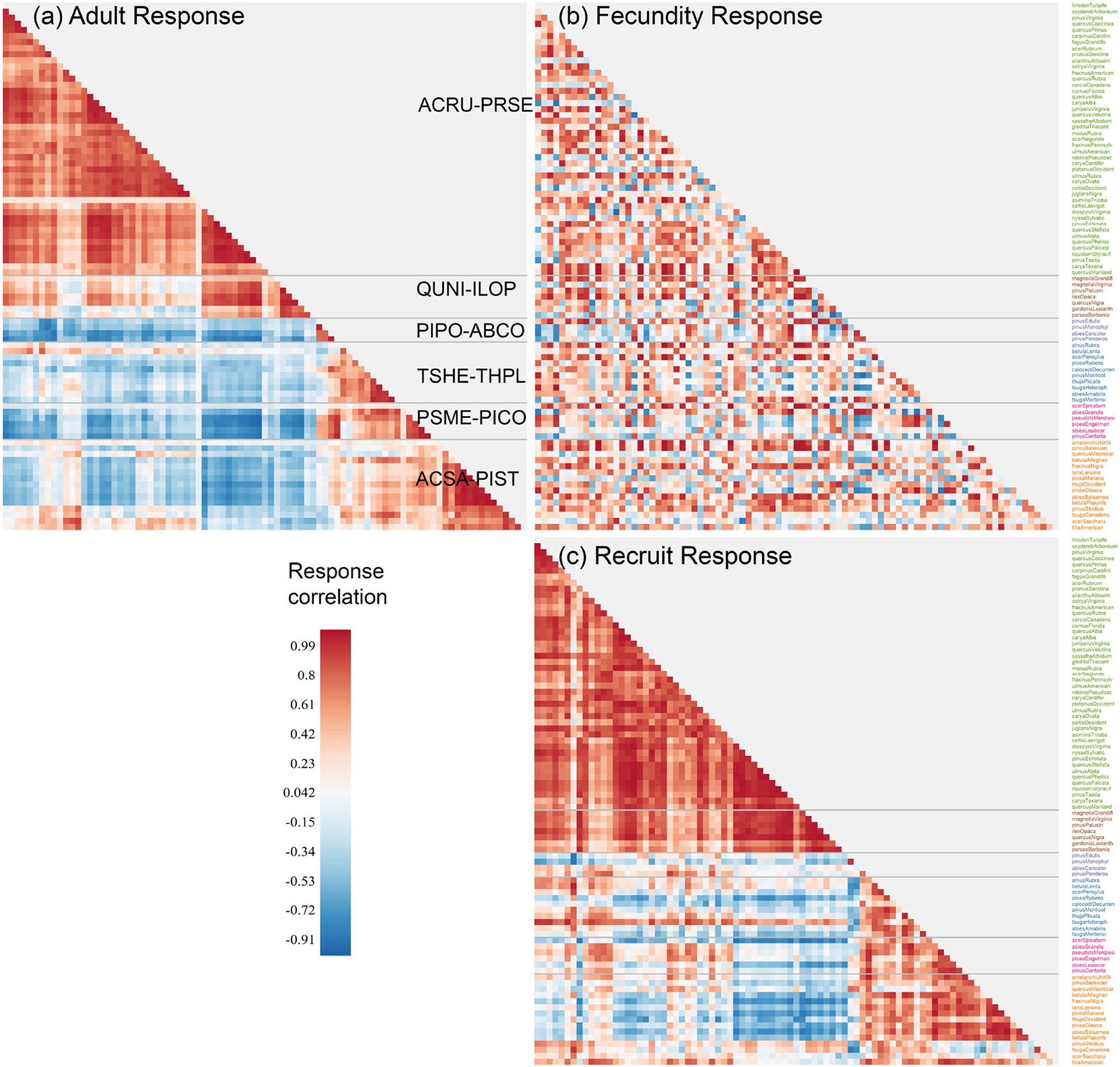
Figure 3. The response matrices for (a) adult (Ea), (b) fecundity (Ef), and (c) conditional recruitment (Eρ). Rows in Ef and Eρ are ordered by clusters estimated for adult responses Ea, with communities separated by dashed lines. Community names are the first two letters of genus and species for the two most abundant species (see Supplementary Figure S1 and Supplementary Table S1).
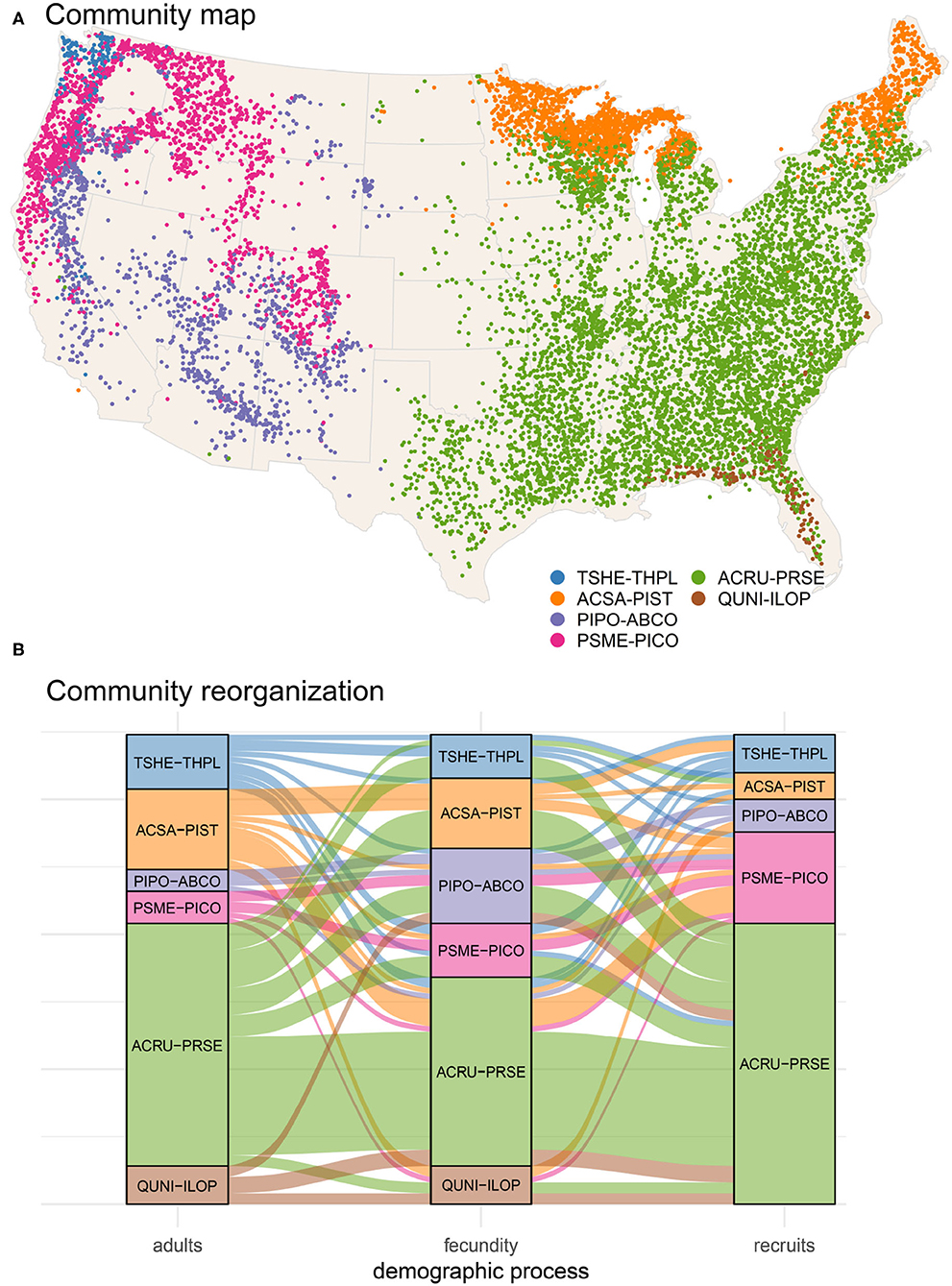
Figure 4. Map of community assemblages (A) and reorganization as an alluvial diagram (B). Community clustering is based on adult tree response matrix (Figure 3a). Separate maps for each type can be found in Supplementary Figure S1. (B) Shifts in assemblages across the three demographic stages (adult, fecundity, and conditional recruits) in eighty-seven species. Flows are color-coded by adult membership in communities mapped in (A).
Conditional recruitment Eρ does not find all six of the community types identified in adult basal area, instead separating southeastern forests as distinct from everything else (Figures 3a,c). The similarities come from the tendency for adult abundance and recruitment rate of the same species to respond similarly. This similarity was not a foregone conclusion from the fact that recruitment and adults occur in basically the same geographic spaces, because recruitment here is taken conditional on adult abundance. The conditional responses separate the effects of adult abundance, so there is opportunity for recruitment sensitivities to depart from those obtained for adults. The important differences result from lower climate sensitivity in recruitment rate than in adult distributions. The differences between Figures 3a,c would not have emerged from a standard, unconditional model, which would have simply showed that recruitment occurs where the adults are.
By contrast with recruitment, the fecundity response matrix Ef bears little resemblance to both adults and recruits (Figure 3b); fecundity response to CHI diverges from the life stages on either side of it. This divergence takes the form of a braided alluvial diagram in Figure 4B, where community reorganization occurs in 57% of species from adults to fecundity and in 64% of species from fecundity to recruits. The adult to fecundity divergence is followed by a partial re-coalescence, with 69% of species returning to the adult community at the recruitment stage. Species in the largest group ARCU-PRSE and PIPO-ABCO scatter across other groups from adults to fecundity, while many return to their adult groups at the recruitment stage. Despite this generally conservative tendency, nearly a third of all species (31%) change groups from adults to recruits. Recruits from the adult community QUNI-ILOP (Forida) merge with ARCU-PRSE, a shift toward conditions that are generally cooler than where adults are found. As adults, the community PSME-PICO combines western species (Figure 4A) with others from the Upper Midwest and Northeast (Supplementary Figure S1). The eastern and Midwestern species (e.g., Abies balsamea, Betula papyrifera, Fraxinus nigra, and Larix laricina) cluster as adults with ACSA-PIST but as recruits with PSME-PICO. Similarly, the eastern Acer saccharum and Betula alleghaniensis belong to ACSA-PIST as adults, whereas recruitment aligns with the predominantly western group TSHE-THPL (see also Supplementary Figure S1).
Discussion
Three main patterns emerge from responses of recruitment stages that will influence the composition of twenty-first-century forests. At a coarse scale, similarities in adult and recruitment responses (Figure 2) suggest a degree of continuity from current to future forests. Secondly, the divergence of fecundity responses from those of both adults and recruitment, with its higher sensitivity to temperature and soil types (Figure 1), can be expected to bias the transition from adults to recruits for each species in different ways. Finally, the non-trivial differences between responses at different stages (31% of all species reorganize to different communities from adults to recruits) (Figure 4) reveal potential for change that cannot be fully anticipated from results presented here. A degree of coherence in niche shifts across the three life-history stages does not diminish the importance of inter-specific competition in influencing community dynamics, but it does indicate that species will be competing with different combinations of species.
Sensitivities quantified in this study show the basis for shifting forest distributions identified by Sharma et al. (2021). If warming has raised temperatures above those where tree populations recruited decades to centuries ago, then conditional recruitment should be occurring today in colder portions of the range and thus show less positive temperature responses than adults. We see this tendency for some species in the northeastern and northwestern TSHE-THPL (Supplementary Figure S1), indicated by brown shades for the species in Figure 2b (recruitment) than in Figure 2a (adults). These are also the communities tending to show a poleward shift in recruitment from adults (Sharma et al., 2021). The fact that recruitment rates for southwestern PSME-PICO are shifted to wetter (lower moisture deficit) parts of the range from adults (deep green for moist and brown for moisture deficit in Figure 2b) is consistent with a shift in regeneration to north and west of current populations (Sharma et al., 2021). The finding also provides evidence that the dieback-prone interior West (Allen et al., 2010) is already suffering from inadequate recruitment. Clearly, the devastating diebacks in this region are just the beginning of transformations that will critically depend on fecundity and seedling recruitment.
Across all species, fecundity exhibits the largest sensitivity to temperature and the lowest sensitivity to moisture deficit (Figure 1). High temperature generally increases fecundity in eastern forests (Supplementary Figure S2) and at least partially explains the fecundity hotspot in the warm southeastern North America (Clark et al., 2021). High recruitment rates are associated with younger stands compared to adult abundance (Figure 2), which could be caused by the increased seed availability where there are young and fast-growing trees (Clark et al., 2021). The fact that fecundity exhibits different responses to environment from those of adults and recruitment rate (Figures 1, 3) highlights the importance of including seed production in understanding climate-driven migration (Sharma et al., 2021). These niche differences interpreted in parameter space (Ba, C, and Bf) provide an alternative view of climate effects to distribution ranges (Lenoir et al., 2009; Zhu et al., 2011; Bell et al., 2014; Fei et al., 2017; Sharma et al., 2021).
Consistent responses to CEC suggest an important role for fertility gradients. The association of western TSHE-THPL, PSME-PICO communities with high CEC characterizes adult stages, but not seedling recruitments (Figure 2). Eastern communities span a range of CEC levels, but recruits are more strongly associated with lower CEC levels (deeper browns for ARCU-PRSE and QUNI-ILOP in Figure 2b). Positive responses to CEC can come with macroelements calcium, magnesium, and potassium that are essential for plant growth (Brady et al., 2008). On the other hand, vegetative growth promoted on fertile sites can reduce light availability and intensify canopy and understory light limitation on recruitment ( Hubbell, 1999; Walters and Reich, 2000; Clark et al., 2012, 2014; Käber, 2021). Similarly, clay and sand fractions determine nutrient and moisture retention, which affects plant growth and potentially influences fecundity through the partition between growth and reproduction. Furthermore, the dominance of positive interactions between moisture deficit and local moisture class is consistent with topographic mediation of climate, particularly for recruits. This positive interaction means that local moisture has an increasingly positive effect the greater the climate deficit (Seyednasrollah and Clark, 2020). The high sensitivity of this interaction for recruitment, particularly in PIPO-ABCO and ACSA-PIST, is consistent with high seedling sensitivity to water availability compared to that of adults (Ibáñez et al., 2007; Dobrowski et al., 2015; Kueppers et al., 2017).
The conditional treatment of recruitment allows for potentially large differences in adult and recruitment habitats, so the similarities and differences found here are both meaningful. An unconditional analysis of recruitment would find high similarity between adults and recruits simply due to the fact that recruits will generally only be abundant where there are adults. These locations share the same covariate values, so recruitment differences from adults are limited to differences in their relative abundances across a species range, but constrained by the fact that the range itself is basically the same for both. Moreover, it would not account for the effects of adults on recruitment. By allowing for variation in adult abundance and then isolating environmental effects (given the adult effects), we could expect to find recruitment sensitivities that diverge from adults. We do in fact find substantial differences (Figures 1, 2).
Rising temperatures, combined with an increased drought severity in many parts of U.S. forests, point to the urgent need to identify effective conservation strategies to maintain stability of forest ecosystems. The shifts from adults to fecundity are more severe than that from adults to recruits (Figure 4B), with a re-coalescence of communities as recruitment for many species revert to niche spaces that are similar to those of adults. Framed in a forest management context, while the fecundity stage may enable climate change-induced disruption of adult to recruit linkages, it may also afford opportunities to develop and implement conservation practices (Nagel et al., 2017) (e.g., silvicultural systems aligned with fecundity dynamics) that take advantage of this disruption to favor particular demographic trajectories across species to meet management objectives.
In summary, the relationships between three life history stages and climate-habitat interactions provide the underlying sensitivities needed to interpret changes in forest recruitment that are happening now and likely to shape future forests. The capacity to examine these stages independently and, in the case of recruitment, conditionally, provides new insight. Collectively, these results highlight species differences in the contribution to community reorganization and how it diverges between adults, seed production, and seedling recruitment. The three life history stages can each contribute to forest change in different ways.
Data Availability Statement
The original contributions presented in the study are included in the article/Supplementary Material, further inquiries can be directed to the corresponding author/s.
Author Contributions
JSC designed the study and compiled the MASTIF and wrote the MSTIF model and software. TQ and JSC performed the statistical analysis and wrote the first draft of the manuscript. All authors contributed to the FIA data organization and contributed to manuscript revision, read, and approved the submitted version.
Funding
This project has been funded by the National Science Foundation DEB-1754443, the Belmont Forum (1854976), NASA (AIST16-0052, AIST18-0063), and the Programme d'Investissement d'Avenir under project FORBIC (18-MPGA-0004) (Make Our Planet Great Again).
Conflict of Interest
The authors declare that the research was conducted in the absence of any commercial or financial relationships that could be construed as a potential conflict of interest.
Publisher's Note
All claims expressed in this article are solely those of the authors and do not necessarily represent those of their affiliated organizations, or those of the publisher, the editors and the reviewers. Any product that may be evaluated in this article, or claim that may be made by its manufacturer, is not guaranteed or endorsed by the publisher.
Supplementary Material
The Supplementary Material for this article can be found online at: https://www.frontiersin.org/articles/10.3389/fevo.2021.719141/full#supplementary-material
References
Abatzoglou, J. T., Dobrowski, S. Z., Parks, S. A., and Hegewisch, K. C. (2018). Terraclimate, a high-resolution global dataset of monthly climate and climatic water balance from 1958–2015. Sci. Data 5, 170191. doi: 10.1038/sdata.2017.191
Allen, C. D., Macalady, A. K., Chenchouni, H., Bachelet, D., McDowell, N., Vennetier, M., et al. (2010). A global overview of drought and heat-induced tree mortality reveals emerging climate change risks for forests. For. Ecol. Manag. 259, 660–684. doi: 10.1016/j.foreco.2009.09.001
Austin, M. P. (2002). Spatial prediction of species distribution: an interface between ecological theory and statistical modelling. Ecol. Modell. 157, 101–118. doi: 10.1016/S0304-3800(02)00205-3
Bell, D. M., Bradford, J. B., and Lauenroth, W. K. (2014). Early indicators of change: divergent climate envelopes between tree life stages imply range shifts in the western united states. Glob. Ecol. Biogeogr. 23, 168–180. doi: 10.1111/geb.12109
Brady, N. C., Weil, R. R., and Weil, R. R. (2008). The Nature and Properties of Soils, Vol. 13. Upper Saddle River, NJ: Prentice Hall.
Clark, J. S., Andrus, R., Aubry-Kientz, M., Bergeron, Y., Bogdziewicz, M., Bragg, D. C., et al. (2021). Continent-wide tree fecundity driven by indirect climate effects. Nat. Commun. 12:1242. doi: 10.1038/s41467-020-20836-3
Clark, J. S., Bell, D. M., Hersh, M. H., and Nichols, L. (2011). Climate change vulnerability of forest biodiversity: climate and competition tracking of demographic rates. Glob. Chang Biol. 17, 1834–1849. doi: 10.1111/j.1365-2486.2010.02380.x
Clark, J. S., Bell, D. M., Kwit, M., Stine, A., Vierra, B., and Zhu, K. (2012). Individual-scale inference to anticipate climate-change vulnerability of biodiversity. Philos. Trans. R. Soc. B Biol. Sci. 367, 236–246. doi: 10.1098/rstb.2011.0183
Clark, J. S., Bell, D. M., Kwit, M. C., and Zhu, K. (2014). Competition-interaction landscapes for the joint response of forests to climate change. Glob. Chang Biol. 20, 1979–1991. doi: 10.1111/gcb.12425
Clark, J. S., Iverson, L., Woodall, C. W., Allen, C. D., Bell, D. M., Bragg, D. C., et al. (2016). The impacts of increasing drought on forest dynamics, structure, and biodiversity in the united states. Glob. Chang Biol. 22, 2329–2352. doi: 10.1111/gcb.13160
Clark, J. S., Macklin, E., and Wood, L. (1998). Stages and spatial scales of recruitment limitation in southern appalachian forests. Ecol. Monogr. 68, 213–235. doi: 10.1890/0012-9615(1998)068[0213:SASSOR]2.0.CO;2
Clark, J. S., Nemergut, D., Seyednasrollah, B., Turner, P. J., and Zhang, S. (2017). Generalized joint attribute modeling for biodiversity analysis: median-zero, multivariate, multifarious data. Ecol. Monogr. 87, 34–56. doi: 10.1002/ecm.1241
Clark, J. S., Nunez, C., and Tomasek, B. (2019). Foodwebs based on unreliable foundations: spatiotemporal masting merged with consumer movement, storage, and diet. Ecol. Monogr. 89:e01381. doi: 10.1002/ecm.1381
Crimmins, S. M., Dobrowski, S. Z., Greenberg, J. A., Abatzoglou, J. T., and Mynsberge, A. R. (2011). Changes in climatic water balance drive downhill shifts in plant species' optimum elevations. Science 331, 324–327. doi: 10.1126/science.1199040
Davis, K. T., Dobrowski, S. Z., Higuera, P. E., Holden, Z. A., Veblen, T. T., Rother, M. T., et al. (2019). Wildfires and climate change push low-elevation forests across a critical climate threshold for tree regeneration. Proc. Natl. Acad. Sci. U.S.A. 116, 6193–6198. doi: 10.1073/pnas.1815107116
Dawson, A., Paciorek, C. J., Goring, S. J., Jackson, S. T., McLachlan, J. S., and Williams, J. W. (2019). Quantifying trends and uncertainty in prehistoric forest composition in the upper midwestern united states. Ecology 100:e02856. doi: 10.1002/ecy.2856
Dobrowski, S. Z., Swanson, A. K., Abatzoglou, J. T., Holden, Z. A., Safford, H. D., Schwartz, M. K., et al. (2015). Forest structure and species traits mediate projected recruitment declines in western us tree species. Glob. Ecol. Biogeogr. 24, 917–927. doi: 10.1111/geb.12302
Engler, R., Randin, C. F., Vittoz, P., Czáka, T., Beniston, M., Zimmermann, N. E., et al. (2009). Predicting future distributions of mountain plants under climate change: does dispersal capacity matter? Ecography 32, 34–45. doi: 10.1111/j.1600-0587.2009.05789.x
Fei, S., Desprez, J. M., Potter, K. M., Jo, I., Knott, J. A., and Oswalt, C. M. (2017). Divergence of species responses to climate change. Sci. Adv. 3:e1603055. doi: 10.1126/sciadv.1603055
Freckleton, R., and Watkinson, A. (2002). Large-scale spatial dynamics of plants: metapopulations, regional ensembles and patchy populations. J. Ecol. 90, 419–434. doi: 10.1046/j.1365-2745.2002.00692.x
Fridley, J. D., and Wright, J. P. (2018). Temperature accelerates the rate fields become forests. Proc. Natl. Acad. Sci. U.S.A. 115, 4702–4706. doi: 10.1073/pnas.1716665115
García-Camacho, R., Iriondo, J. M., and Escudero, A. (2010). Seedling dynamics at elevation limits: complex interactions beyond seed and microsite limitations. Am. J. Bot. 97, 1791–1797. doi: 10.3732/ajb.1000248
Gray, A. N., Brandeis, T. J., Shaw, J. D., McWilliams, W. H., and Miles, P. (2012). “Forest inventory and analysis database of the united states of america (fia). Biodiver. Ecol. 4, 225–231. doi: 10.7809/b-e.00079
Grubb, P. J. (1977). The maintenance of species-richness in plant communities: the importance of the regeneration niche. Biol. Rev. 52, 107–145. doi: 10.1111/j.1469-185X.1977.tb01347.x
Hartigan, J. A., and Wong, M. A. (1979). A k-means clustering algorithm. J. R. Stat. Soc. 28, 100–108. doi: 10.2307/2346830
Hengl, T., Mendes de Jesus, J., Heuvelink, G. B. M., Ruiperez Gonzalez, M., Kilibarda, M., Blagotić, A., et al. (2017). Soilgrids250m: global gridded soil information based on machine learning. PLoS ONE 12:e0169748. doi: 10.1371/journal.pone.0169748
Hubbell, S. P., Foster, R. B., O'Brien, S. T., Harms, K. E., Condit, R., Wechsler, B, and Light-Gap disturbances, recruitment limitation, tree diversity in a neotropical forest. Science. (1999) 283:554–7. doi: 10.1126/science.283.5401.554.
Hutchinson, G. (1957). “Concluding remarks. Population studies: animal ecology and demography,” in Cold Spring Harbor Symposia on Quantitative Biology (New Haven, CT), 415–427.
Ibanez, I., Clark, J. S., and Dietze, M. C. (2009). Estimating colonization potential of migrant tree species. Glob. Chang Biol. 15, 1173–1188. doi: 10.1111/j.1365-2486.2008.01777.x
Ibanez, I., Clark, J. S., Dietze, M. C., Feeley, K., Hersh, M., LaDeau, S., et al. (2006). Predicting biodiversity change: Outside the climate envelope, beyond the species-area curve. Ecology 87, 1896–1906. doi: 10.1890/0012-9658(2006)87[1896:PBCOTC]2.0.CO;2
Ibáñez, I., Clark, J. S., LaDeau, S., and Lambers, J. H. R. (2007). Exploiting temporal variability to understand tree recruitment response to climate change. Ecol. Monogr. 77, 163–177. doi: 10.1890/06-1097
Iverson, L. R., and Prasad, A. M. (1998). Predicting abundance of 80 tree species following climate change in the eastern united states. Ecol. Monogr. 68, 465–485. doi: 10.1890/0012-9615(1998)068[0465:PAOTSF]2.0.CO;2
Iverson, L. R., Prasad, A. M., Matthews, S. N., and Peters, M. (2008). Estimating potential habitat for 134 eastern us tree species under six climate scenarios. For. Ecol. Manag. 254, 390–406. doi: 10.1016/j.foreco.2007.07.023
Käber, Y., Meyer, P., Stillhard, J., De Lombaerde, E., Zell, J., Stadelmann, G., et al. (2021). Tree recruitment is determined by stand structure and shade tolerance with uncertain role of climate and water relations. Ecol. Evol. 11, 12182–12203. doi: 10.1002/ece3.7984
Karger, D. N., Conrad, O., Böhner, J., Kawohl, T., Kreft, H., Soria-Auza, R. W., et al. (2017). Climatologies at high resolution for the earth's land surface areas. Sci. Data 4:170122. doi: 10.1038/sdata.2017.122
Kueppers, L. M., Conlisk, E., Castanha, C., Moyes, A. B., Germino, M. J., de Valpine, P., et al. (2017). Warming and provenance limit tree recruitment across and beyond the elevation range of subalpine forest. Glob. Chang Biol. 23, 2383–2395. doi: 10.1111/gcb.13561
Lenoir, J., Gégout, J.-C., Guisan, A., Vittoz, P., Wohlgemuth, T., Zimmermann, N. E., et al. (2010). Going against the flow: potential mechanisms for unexpected downslope range shifts in a warming climate. Ecography 33, 295–303. doi: 10.1111/j.1600-0587.2010.06279.x
Lenoir, J., Gégout, J.-C., Pierrat, J.-C., Bontemps, J.-D., and Dhôte, J.-F. (2009). Differences between tree species seedling and adult altitudinal distribution in mountain forests during the recent warm period (1986–2006). Ecography 32, 765–777. doi: 10.1111/j.1600-0587.2009.05791.x
Nagel, L. M., Palik, B. J., Battaglia, M. A., D'Amato, A. W., Guldin, J. M., Swanston, C. W., et al. (2017). Adaptive silviculture for climate change: a national experiment in manager-scientist partnerships to apply an adaptation framework. J. For. 115, 167–178. doi: 10.5849/jof.16-039
Pearson, R. G., and Dawson, T. P. (2003). Predicting the impacts of climate change on the distribution of species: are bioclimate envelope models useful? Glob. Ecol. Biogeogr. 12, 361–371. doi: 10.1046/j.1466-822X.2003.00042.x
R Core Team (2020). R: A Language and Environment for Statistical Computing. Vienna: R Foundation for Statistical Computing.
Rosvall, M., and Bergstrom, C. T. (2010). Mapping change in large networks. PLoS ONE 5:e8694. doi: 10.1371/journal.pone.0008694
Serra-Diaz, J. M., Franklin, J., Dillon, W. W., Syphard, A. D., Davis, F. W., and Meentemeyer, R. K. (2016). California forests show early indications of both range shifts and local persistence under climate change. Glob. Ecol. Biogeogr. 25, 164–175. doi: 10.1111/geb.12396
Seyednasrollah, B., and Clark, J. S. (2020). Where resource-acquisitive species are located: the role of habitat heterogeneity. Geophys. Res. Lett. 47:e2020GL087626. doi: 10.1029/2020GL087626
Sharma, S., Andrus, R., Bergeron, Y., Bogdziewicz, M., Bragg, D. C., Brockway, D., et al. (2021). North american tree migration tracking climate change in the west, slowed by reproduction in the east.
Swab, R. M., Regan, H. M., Keith, D. A., Regan, T. J., and Ooi, M. K. J. (2012). Niche models tell half the story: spatial context and life-history traits influence species responses to global change. J. Biogeogr. 39, 1266–1277. doi: 10.1111/j.1365-2699.2012.02690.x
Thomas, C. D., Cameron, A., Green, R. E., Bakkenes, M., Beaumont, L. J., Collingham, Y. C., et al. (2004). Extinction risk from climate change. Nature 427, 145–148. doi: 10.1038/nature02121
Walck, J. L., Hidayati, S. N., Dixon, K. W., Thompson, K., and Poschlod, P. (2011). Climate change and plant regeneration from seed. Glob. Chang Biol. 17, 2145–2161. doi: 10.1111/j.1365-2486.2010.02368.x
Walters, M. B., and Reich, P. B. (2000). Seed size, nitrogen supply, and growth rate affect tree seedling survival in deep shade. Ecology 81, 1887–1901. doi: 10.1890/0012-9658(2000)081[1887:SSNSAG]2.0.CO;2
Williams, J. W., and Jackson, S. T. (2007). Novel climates, no-analog communities, and ecological surprises. Front. Ecol. Environ. 5, 475–482. doi: 10.1890/070037
Woodall, C., Oswalt, C., Westfall, J., Perry, C., Nelson, M., and Finley, A. (2009). An indicator of tree migration in forests of the eastern united states. For. Ecol. Manag. 257, 1434–1444. doi: 10.1016/j.foreco.2008.12.013
Woudenberg, S. W., Conkling, B. L., O'Connell, B. M., LaPoint, E. B., Turner, J. A., and Waddell, K. L. (2010). The forest inventory and analysis database: database description and users manual version 4.0 for phase 2. General Technical Report RMRS-GTR-245. Fort Collins, CO: US Department of Agriculture, Forest Service, Rocky Mountain Research Station.
Zhu, K., Woodall, C. W., and Clark, J. S. (2011). Failure to migrate: lack of tree range expansion in response to climate change. Glob. Chang Biol. 18, 1042–1052. doi: 10.1111/j.1365-2486.2011.02571.x
Zhu, K., Woodall, C. W., Ghosh, S., Gelfand, A. E., and Clark, J. S. (2014). Dual impacts of climate change: forest migration and turnover through life history. Glob. Chang Biol. 20, 251–264. doi: 10.1111/gcb.12382
Keywords: climate change, regeneration niche, tree migration, life-history stages, seed production
Citation: Qiu T, Sharma S, Woodall CW and Clark JS (2021) Niche Shifts From Trees to Fecundity to Recruitment That Determine Species Response to Climate Change. Front. Ecol. Evol. 9:719141. doi: 10.3389/fevo.2021.719141
Received: 01 June 2021; Accepted: 08 November 2021;
Published: 23 December 2021.
Edited by:
Marta Benito Garzon, INRAE Nouvelle-Aquitaine Bordeaux, FranceReviewed by:
Zehao Shen, Peking University, ChinaStefan Siebert, North-West University, South Africa
Copyright © 2021 Qiu, Sharma, Woodall and Clark. This is an open-access article distributed under the terms of the Creative Commons Attribution License (CC BY). The use, distribution or reproduction in other forums is permitted, provided the original author(s) and the copyright owner(s) are credited and that the original publication in this journal is cited, in accordance with accepted academic practice. No use, distribution or reproduction is permitted which does not comply with these terms.
*Correspondence: Tong Qiu, dG9uZy5xaXUmI3gwMDA0MDtkdWtlLmVkdQ==