- 1Dipartimento di Scienze della Terra, Sapienza Università di Roma, Rome, Italy
- 2Evolutionary Studies Institute (ESI) and School of Geosciences, University of the Witwatersrand, Johannesburg, South Africa
- 3Associazione Paleontologica e Paleoartistica Italiana (APPI), Parma, Italy
Pareiasaurs (Amniota, Parareptilia) were characterized by a global distribution during the Permian period, forming an important component of middle (Capitanian) and late Permian (Lopingian) terrestrial tetrapod biodiversity. This clade represents an early evolution of sizes over a ton, playing a fundamental role in the structure of middle and late Permian biodiversity and ecosystems. Despite their important ecological role and relative abundance around the world, our general knowledge of the biology of these extinct tetrapods is still quite limited. In this contribution we provide a possible in vivo reconstruction of the largest individual of the species Scutosaurus karpinskii and a volumetric body mass estimate for the taxon, considering that body size is one of the most important biological aspects of organisms. The body mass of Scutosaurus was calculated using a 3D photogrammetric model of the complete mounted skeleton PIN 2005/1537 from the Sokolki locality, Arkhangelsk Region, Russia, on exhibit at the Borissiak Paleontological Institute, Russian Academy of Sciences (Moscow). By applying three different densities for living tissues of 0.99, 1, and 1.15 kg/1,000 cm3 to reconstructed “slim,” “average” and “fat” 3D models we obtain average body masses, respectively, of 1,060, 1,160, and 1,330 kg, with a total range varying from a minimum of one ton to a maximum of 1.46 tons. Choosing the average model as the most plausible reconstruction and close to the natural condition, we consider a body mass estimate of 1,160 kg as the most robust value for Scutosaurus, a value compatible with that of a large terrestrial adult black rhino and domestic cow. This contribution demonstrates that barrel-shaped herbivores, subsisting on a high-fiber diet and with a body mass exceeding a ton, had already evolved in the upper Palaeozoic among parareptiles, shedding new light on the structure of the first modern terrestrial ecosystems.
Introduction
Pareiasaurs comprise a monophyletic group of herbivorous parareptiles, which lived during the middle and the late Permian (Boonstra, 1969; Lee, 1993, 1994, 1997a,b) comprising at least 22 species (Liu and Bever, 2018; Van den Brandt, 2020; Van den Brandt et al., 2020), were relatively abundant and had a global distribution during the Permian period, thus forming an important component of middle (Capitanian) and late Permian (Lopingian) terrestrial tetrapod biodiversity. They represent an early evolution of sizes over a ton and made up an important component of middle and late Permian biodiversity and ecosystems (Lee, 1997a; Day, 2013; Day et al., 2015, 2018). Along with the Dinocephalia and caseids (Romano and Rubidge, 2019a; Rubidge et al., 2019; Day and Rubidge, 2020), pareiasaurs were the only other amniote group that attained massive size in the middle Permian (up to 3 m long). The pareiasaur clade achieved a high level of diversity and a world-wide distribution by the late Permian, before their ultimate demise at the Permo-Triassic boundary.
Pareiasaurs are classified as members of the clade Parareptilia, a group of basal amniotes with no living representatives (Tsuji and Muller, 2009). Members of this group were distributed world-wide and were characterized by a “cheek” flange produced by an expanded quadratojugal bone on the side of the skull, for which the group is named (Owen, 1876), cranial ornamentation comprising bosses, pitting and ridges and a unique protruding boss on the angular bone of the lower jaw (Owen, 1876; Seeley, 1888, 1892). All members possess labio-lingually flattened (leaf-shaped) teeth specialized for herbivory (Lee, 1993, 1994, 1997a,b) and dermal body ossification (osteoderms or scutes) distributed in different patterns on different species (Boonstra, 1934a; Lee, 1994, 1997a; Scheyer and Sander, 2009; Boyarinova et al., 2019).
The majority of early pareiasaur material stemmed from South Africa and Russia (e.g., Seeley, 1888, 1892; Broom, 1912, 1913, 1914, 1924; Watson, 1914; Amalitsky, 1922; Haughton and Boonstra, 1929; Hartmann-Weinberg, 1930, 1937; Boonstra, 1934b) where in the late 1800’s and early 1900’s a plethora of pareiasaur species were named. This generated an inflated number of species and historic taxonomic confusion which hampered understanding of this important group of tetrapods for most of the twentieth century until the work of Lee which sorted out many taxonomic issues, reducing the number of recognized species from 49 to 17 (Lee, 1994, 1997a).
Presently, almost half of all pareiasaur genera are found in southern Africa (eight genera, see below), followed by Russia (four genera, see below) and China (also four genera: Honania, Young, 1979; Xu et al., 2015; Shihtienfenia, Young and Yeh, 1963; Benton, 2016; Sanchuansaurus, Gao, 1989; Benton, 2016; Elginia wuyongae, Liu and Bever, 2018). One species each are found in Zambia (Pareiasuchus nasicornis, Haughton and Boonstra, 1929), Tanzania [Pareiasaurus(?) haughtoni, von Huene, 1944; Maisch and Matzke, 2019], Niger (Bunostegos, Sidor et al., 2003; Tsuji et al., 2013; Turner et al., 2015), Morocco (Arganaceras, Jalil and Janvier, 2005), Brazil (Provelosaurus, Araujo, 1985; Cisneros et al., 2005), and Scotland (Elginia mirabilis, Newton, 1893).
Pareiasaurs follow three different morphotypes or shape models (Scheyer and Sander, 2009): (1) large, sparsely armored forms, to (2) medium to large forms with more armor coverage, to (3) small, very heavily armored dwarf pareiasaurs.
In South Africa, the pareiasaurs of the middle Permian Tapinocephalus Assemblage Zone (AZ) (Lee, 1997a; Day and Rubidge, 2020) are all large basal forms (Bradysaurus, Embrithosaurus, and Nochelesaurus) and have restricted dermal armor comprising a narrow band of isolated osteoderms above the vertebral column (Watson, 1914; Haughton and Boonstra, 1929; Lee, 1997a; Van den Brandt et al., 2021a,b). In the overlying late Permian Endothiodon, Cistecephalus, and Daptocephalus AZs (Day and Smith, 2020; Smith, 2020; Viglietti, 2020) are medium-to large-sized pareiasaurs which have isolated osteoderms covering the entire body (Pareiasaurus and Pareiasuchus), and derived dwarf pareiasaurs with united osteoderms forming a carapace over the entire body (Nanopareia, Anthodon, and Pumiliopareia) (Watson, 1914; Lee, 1997a).
In South Africa, the three middle Permian pareiasaur genera were all large animals (3 m body length) and all went extinct at the end of the Capitanian, to be replaced by a new, local pareiasaurian fauna comprising predominately small to medium sized forms and the large Pareiasaurus. Across all terrestrial vertebrate groups, including pareiasaurs, the taxa that went extinct at the end-Capitanian and end Permian, were mostly large animals.
Russian Pareiasaurs
After South Africa, Russia is the next most prolific region for pareiasaur remains. In Russia, the different pareiasaurian taxa are readily identifiable, morphologically distinct, and have differing body sizes. Currently four Russian pareiasaur species are recognized. The large and derived Scutosaurus karpinskii (2.5–3 m long) was the first named Russian pareiasaur (Amalitsky, 1922), followed by the medium-sized, more primitive Deltavjatia rossica (1.5–2 m long) (Hartmann-Weinberg, 1937), the large Proelginia permiana (Hartmann-Weinberg, 1937; Ivakhnenko et al., 1997; Ivakhnenko, 2008) and the small, derived Obirkovia gladiator (<1 m long) (Bulanov and Yashina, 2005). The large Scutosaurus is late Permian in age and the medium-sized Deltavjatia is middle Permian in age (Benton et al., 2012; Arefiev et al., 2015). As a result of their distinct and differing morphology and obvious size differences, the taxonomy of the Russian pareiasaurs is well-established, apart from the placement of Proelginia permiana (Hartmann-Weinberg, 1937, holotype PIN 156/1), which Lee (1996, 1997a) synonymised with Scutosaurus. Ivakhnenko (2008), A Sennikov and E Boyarinova (personal communications) interpret Proelginia permiana as a separate species from Scutosaurus karpinskii.
Deltavjatia has been very well-studied and is currently probably the best known Russian pareiasaur. Tsuji (2010, 2013) published updated cranial and postcranial descriptions and phylogenetic analyses for Deltavjatia, and due to the preservation of number of different sized (i.e., aged) skulls, this is the only pareiasaur for which cranial ontogenetic growth studies have been published (Tsuji, 2010, 2013). Deltavjatia is also renowned for the exceptional preservation of about 20 complete or nearly complete specimens, from the Kotel’nich locality, Vjatka River, Kirov Region, which has facilitated ecological lifestyle and taphonomic scenario studies of these animals (Benton et al., 2012). Obirikovia gladiator, although small and distinct, with long conical cranial bosses, is the least known Russian taxon, represented by only a fragment of the quadratojugal and a few osteoderms (Bulanov and Yashina, 2005).
Scutosaurus
Based on several virtually complete specimens excavated in the early 1900’s from North Dvina (Amalitsky, 1922), Amalitsky named four pareiasaur species (misspelling the genus name): Pareiosaurus karpinskii, based on PIN 2005/1532; Pareiosaurus tuberculatus, based on PIN 2005/1533; Pareiosaurus horridus, based on PIN 2005/1535; and Pareiosaurus elegans, based on PIN 2005/1538 (Ivakhnenko et al., 1997). Hartmann-Weinberg (1930) considered these four Russian taxa as all belonging to one species (Scutosaurus karpinskii) and erected the genus Scutosaurus to accommodate them. Kuhn (1969) and Lee (1994, 1996, 1997a) agreed with the single species interpretation of Hartmann-Weinberg (1930) for Scutosaurus karpinskii (that it includes P. tuberculatus, P. horridus, and P. elegans). Ivakhnenko (1987) reinstated Scutosaurus tuberculatus and erected Scutosaurus itilensis based on PIN 3919/1, which Lee (1996, 1997a) interprets as a junior synonym for Scutosaurus karpinskii. Lee (1996:80) explained his reasoning for supporting the single species interpretation of Scutosaurus, based on his observations of the four holotypes of Amalitsky, PIN 3919, and several dozen more specimens, all of which show no clear differences between them. According to Tsuji (2013) and Benton (2016) Scutosaurus represents one of the most morphologically derived taxa within the pareiasaurian clade.
Presently, the four original holotypes of Amalitsky (1922) are included in the incredible display of seven adult mounted articulated Scutosaurus specimens at the PIN in Moscow (PIN 2005/1532, PIN 2005/1533, PIN 2005/1534, PIN 2005/1535, PIN 2005/1536, PIN 2005/1537, and PIN 2005/1538) and an additional mounted juvenile specimen (PIN 2005/1578).
Despite the importance of the well-preserved Scutosaurus specimens to determine early amniote body weights, currently no detailed body mass estimate for this genus is available. The evolution of body size over time is a very crucial aspect in a macro-evolutionary context, considering the central importance of body mass in several biological aspects including general physiology, ecology, metabolism, preferential diet, home range and trophic requirements, growth rate, locomotion, life span, and fecundity (see Millar and Zammuto, 1983; LaBarbera, 1989; Martin and Palumbi, 1993; Calder, 1996; Davidowitz and Nijhout, 2004; Gillooly et al., 2006; Fisher et al., 2011; Campione and Evans, 2012; Clauss et al., 2013). In the field of paleontology and macroevolution, it is pivotal to understand how huge body masses evolved for the first time, particularly in the evolution of herbivores which developed long intestinal tracts to enable digestion of celluloses and hemicelluloses (Reisz and Sues, 2000; Lombardo, 2008; Hong et al., 2011; Romano and Nicosia, 2014, 2015; Romano, 2017a), and as a deterrent to large contemporary predators (see Sinclair et al., 2003). In this context, obtaining a plausible and refined estimate of Scutosaurus karpinskii body mass will shed light on the evolution of body sizes in late Paleozoic tetrapods.
In this contribution we present a volumetric body mass estimate of Scutosaurus determined using a 3D photogrammetric model of the complete mounted skeleton PIN 2005/1537 from the Sokolki locality, Arkhangelsk Region, Russia (Figure 1), which is on exhibit at the Borissiak Paleontological Institute, Russian Academy of Sciences (Moscow).
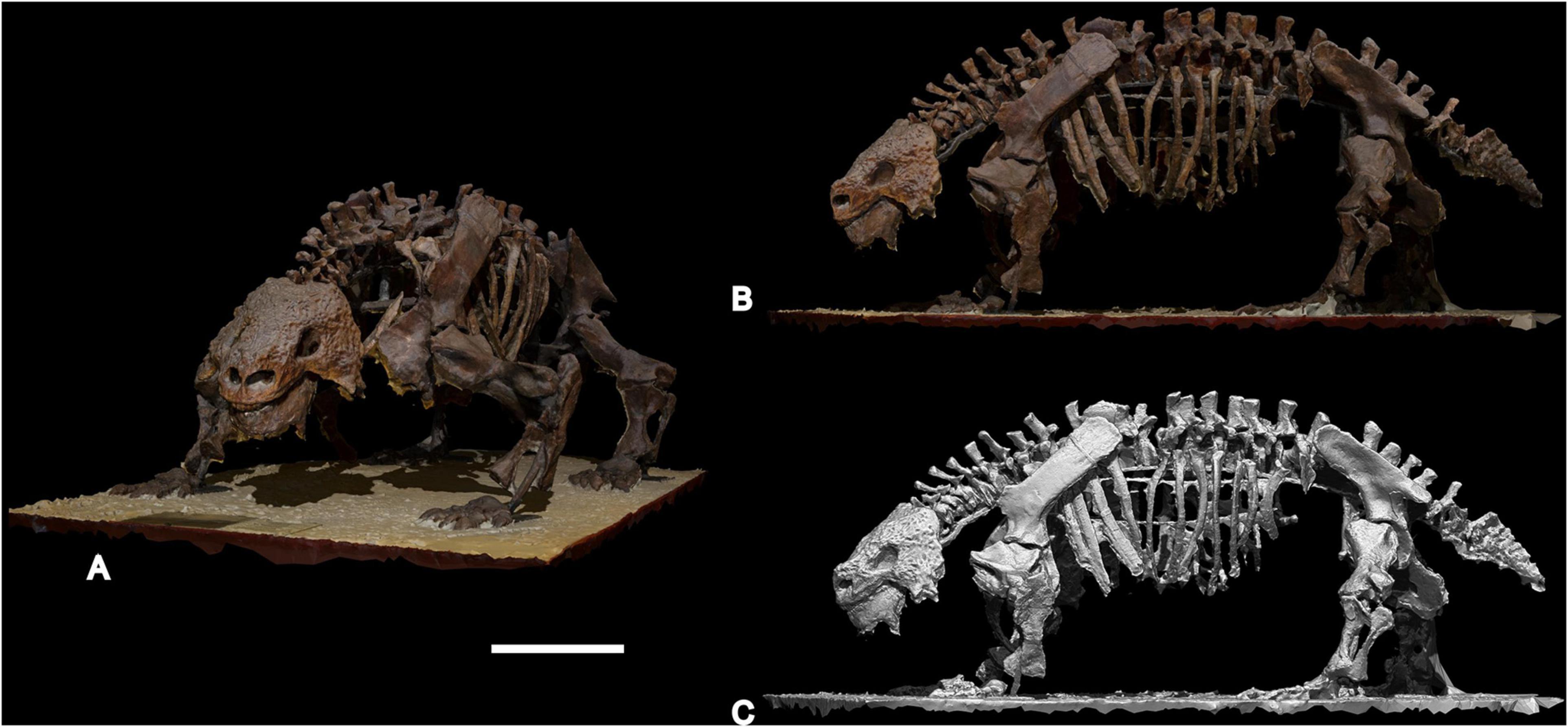
Figure 1. 3D photogrammetric model of almost complete Scutosaurus karpinskii skeleton PIN 2005/1537 from the Sokolki locality, Arkhangelsk Region (Russia), on exhibit at the Borissiak Paleontological Institute, Russian Academy of Sciences, Moscow, Russia. (A,B) 3D model with original texture; (C) 3D solid model. Scale bar equal to 50 cm.
In addition to the body mass estimation we also present an in vivo artistic reconstruction of Scutosaurus karpinskii on the base of the “average model.”
Materials and Methods
In vivo restoration and body mass estimate of Scutosaurus karpinskii is based on the almost complete mounted skeleton PIN 2005/1537 from the Sokolki locality, Arkhangelsk Region (Russia), on exhibit at the Borissiak Paleontological Institute, Russian Academy of Sciences, Moscow, Russia. Firstly a 3D photogrammetric model of the skeleton was generated by taking 180 photographs around the specimen with a 24 Megapixel Canon EOS 750D (18 mm focal length). Then a high definition model was calculated using the software Agisoft Metashape Standard Edition, version 1.5.0 (Educational License, 64 bit) (Figure 1). Agisoft enables automatic generation of DSMs/DTMs from still images, point clouds, polygonal models, georeferenced true orthomosaics, and textured. High resolution Digital Photogrammetry is based on Multi View Stereo (MVS; Seitz et al., 2006) algorithms and structure from Motion (SfM) (Ullman, 1979); close-range photography results in an accuracy of up to 1 mm in the calculated models.
The obtained photogrammetric model was exported as “Ply” files and uploaded in ZBrush, software for digital sculpting and painting, which enables isolation of individual bones in the skeleton, compensation for distortion and, when necessary, to modify the posture of the animal (Romano and Rubidge, 2019a; see Romano and Manucci, 2019). Following the procedure proposed by Romano and Manucci (2019) and Romano and Rubidge (2019a), to produce a realistic range of weight estimations we used the 3D sculpture software to obtain three different reconstructions of Scutosaurus, adding different masses of soft tissue around the reconstructed skeleton (Figures 2, 3). The first is a body reconstruction following the contour of the skeleton, indicated as “slim model” (Figure 2B). The second model, termed “average model” is what we consider the most likely reconstruction of the animal in life, with the most probable amount of fleshy material (Figures 2C, 3). The third reconstruction is generated by adding an excess of soft tissue mass around the skeleton, and is termed “fat model” (Figure 2D).
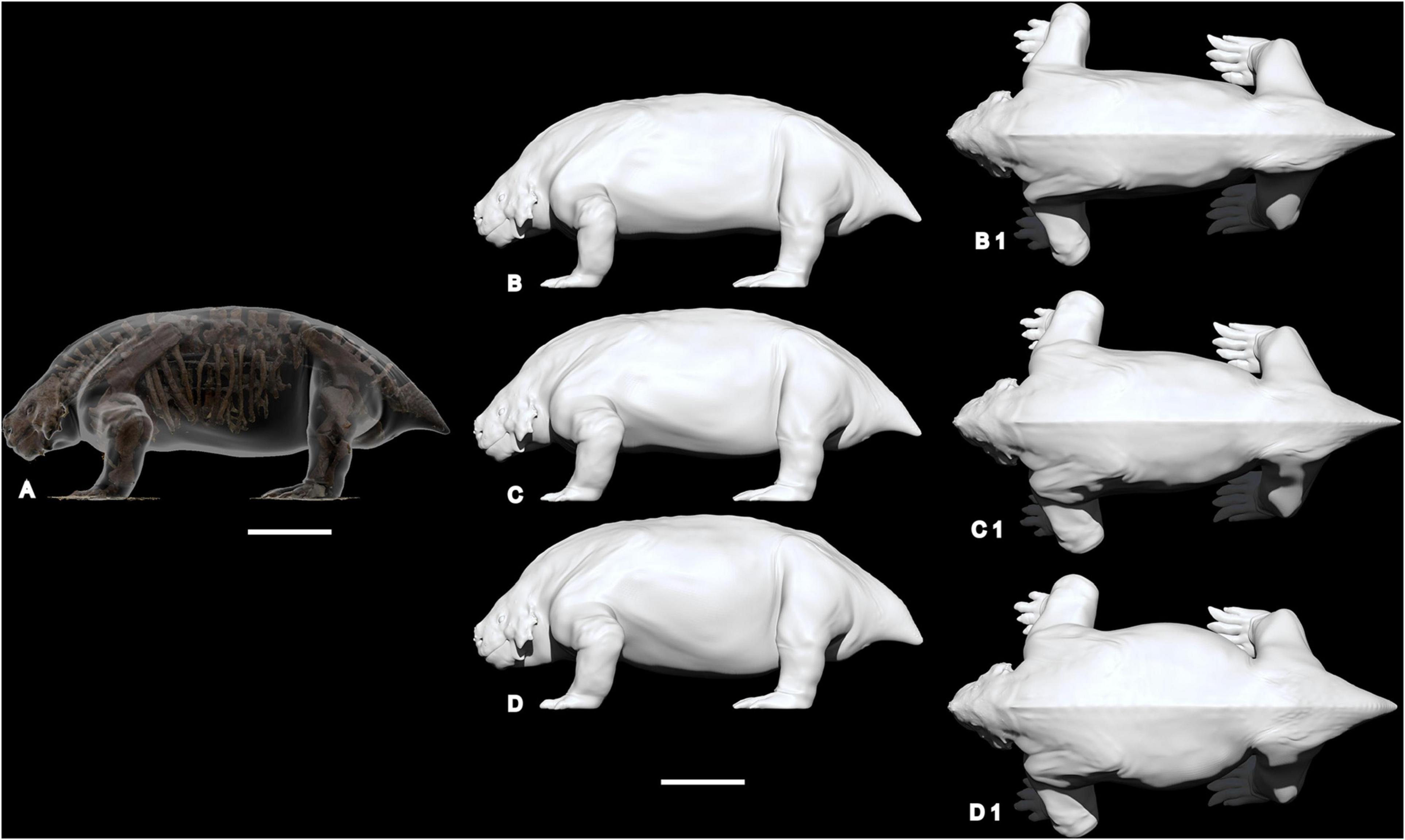
Figure 2. Three different reconstructions of Scutosaurus, adding different masses of soft tissue around the reconstructed skeleton. (A) digital sculpture in transparency around the original skeleton; (B,B1) “slim model” in lateral and dorsal views; (C,C1) “average model” in lateral and dorsal view; (D,D1) “fat model” in lateral and dorsal view. Scale bar equal to 50 cm.
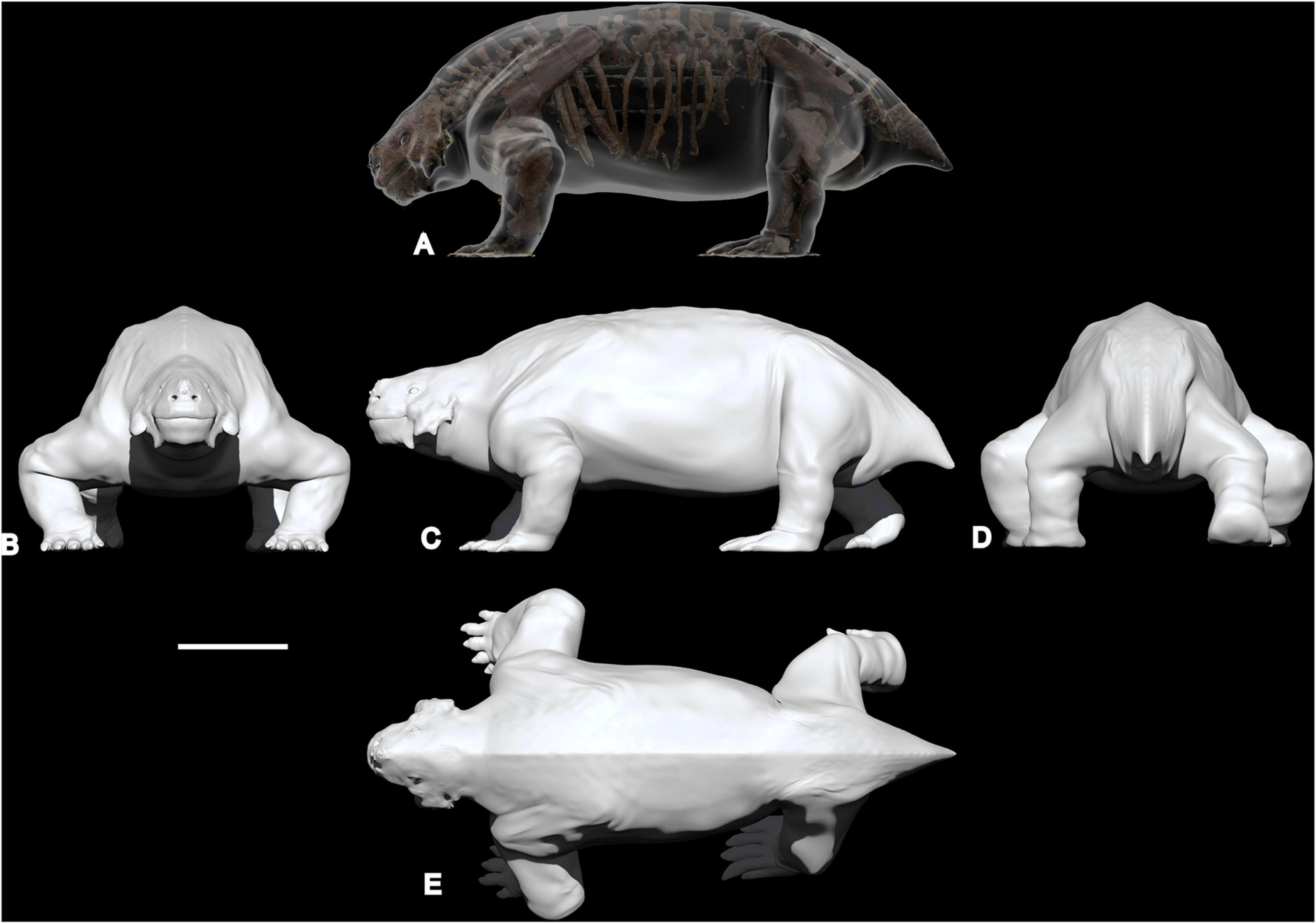
Figure 3. Digital sculpture of the “average model” in transparency around the original skeleton (A), frontal view (B), lateral view (C), posterior view (D), and dorsal view (E). Scale bar equal to 50 cm.
The three models were than imported in the software 3D Studio Max to calculate the total surface area and volume of the scaled digital sculptures (see Romano and Manucci, 2019; Romano and Rubidge, 2019a; Romano et al., 2021). The next step was to apply a specific density to the living tissue to obtain an estimate of the body mass from the obtained volume. In particular, Larramendi (2016) proposed a specific average body gravity in extinct proboscideans of 0.99 to 1.01; in several contributions (e.g., Alexander, 1985, 1989; Gunga et al., 1995; Henderson, 1999; Hurlburt, 1999; Hutchinson et al., 2007; Bates et al., 2009, 2015; Romano et al., 2021) the density of water, i.e., 1 kg/1,000 cm3, has been used as the most plausible value to calculate body mass in extinct vertebrates. According to Gunga et al. (2007), rhinoceros has the highest density of land mammals, equal to 1.15 kg/1,000 cm3. Considering this range of values for the density of living tissue in land vertebrates, we applied the three densities of 0.99, 1, and 1.15 kg/1,000 cm3 to the volumes in the three different reconstructed models of Scutosaurus, following the same procedure proposed by Romano et al. (2021).
For completeness we also calculated the possible body mass of Scutosaurus using classic regression formulas based on the circumference of the femur and humerus, in particular the formulas proposed by Anderson et al. (1985) and Campione and Evans (2012). Anderson et al. (1985) propose the formula W = 0.078⋅Ch+F2.73 ± 0.09, where CH+F is the sum of the humerus and femur circumferences, calculated on the basis of a large mammal dataset from the Mountain Zebra National Park and the Kruger National Park of South Africa. Campione and Evans (2012) propose the universal formula LogBM = 2.754.logCh+F − 1.097, on the basis of a large dataset incorporating non-avian reptiles and mammals.
Results
The reconstruction of the appearance of Scutosaurus in the three proposed variants is shown in Figure 2. The “slim model” was reconstructed following the outline of the skeleton (Figure 2B), hence not including additional muscle mass or soft tissue. The digitally sculptured “slim model” once imported and scaled in 3D Studio Max using different bone measures, returned a total surface area for the model of 8.25 m2, and a total volume of 1.01 m3. Application of the densities for the living tissues selected for the present work to this volume, equal to 0.99, 1, and 1.15 kg/1,000 cm3, the respective resultant body mass estimates were 1,000, 1,010, and 1,160 kg, with an average value of 1,060 kg.
The “average model” was reconstructed using extant land tetrapods such as hippos and rhinos as a reference and as an indication of muscle mass and soft tissues, is shown in Figures 2C, 3. In our opinion this model represents the most likely proportions closest to the natural condition. The model imported and scaled in 3D Studio Max is characterized by a total surface area of 8.5 m2 and a volume of 1.11 m3. By applying the three densities to this volume the obtained body mass are 1,090, 1,110, and 1,280 kg respectively, with an average value of 1,160 kg.
The “fat model” was sculptured using an extremely barrel-shaped ribcage layout (as found in some reconstructions of Bradysaurus (Lee, 1994, 1997a) and digitally adding excess muscle mass and soft tissue to the photogrammetric model of the skeleton (Figure 2D). The model, scaled and imported in 3D Studio Max, has a total surface are of 9.04 m2 and a volume of 1.27 m3. The application of the three densities for living tissues to this volume yielded a body mass of, respectively, 1,260, 1,270, and 1,460 kg, with an average value of 1,330 kg.
The presence of extremely robust limb bones in Scutosaurus has led to the hypothesis that the classic regression formulas based on stylopods could lead to an overestimation of the body mass, as already found in large therapsids (Romano and Manucci, 2019; Romano and Rubidge, 2019a) and dwarf island elephants (Romano et al., 2021). By applying the formula of Anderson et al. (1985) to the circumference of the left femur (296 mm) and left humerus (247 mm) in Scutosaurus specimen PIN 2005/1537 we obtain a minimum value of 1,294 kg and a maximum value of 4,020 kg, with an average value of 2,657 kg. Application of this body mass to the volume of the average model equal to 1.11 m3, we obtain a density of 2.39 for living tissues i.e., slightly more than the density of sandstone. By applying to the same average volume the maximum value of 4,020 kg calculated by using the formula of Anderson et al. (1985), the density of Scutosaurus in life would have been more than the average density of diamond (i.e., 3.62).
The formula provided by Campione and Evans (2012) returned an average body mass of 2,720 kg, with a minimum of 2,040 kg and a maximum of 3,499 kg considering the ±25% error. By applying these body masses to the average volume of the reconstructed Scutosaurus, the density varies from a minimum of 2.16 (about the density of the concrete) up to 3.15 (slightly greater than granite and marble).
Figure 4 shows the box plots with the estimate of the body mass of the three reconstructed morphs (using the three different densities) and the regression formulae of Anderson et al. (1985) and Campione and Evans (2012). As shown in the graph, the largest range is given by the formula of Anderson et al. (1985) (Figure 4C), covering almost the entire spectrum of body mass obtained with the other methods. The weight range obtained using the formula of Campione and Evans (2012) is more restricted but still consistent, ranging from a minimum of 2,040 kg to a maximum of 3,499 kg (Figure 4B). In contrast the range obtained using the volumetric method is much narrower (Figure 4A), as already observed in other studies (Romano and Manucci, 2019; Romano and Rubidge, 2019a), with only a slight overlap between the maximum volumetric values and the minimum values obtained with the method of Anderson et al. (1985). According to the present volumetric study, the body mass of an adult Scutosaurus karpinskii could likely vary from a minimum of one ton to a maximum of 1.46 tons with an average value, considered to be the closest to the natural condition, of 1,160 kg.
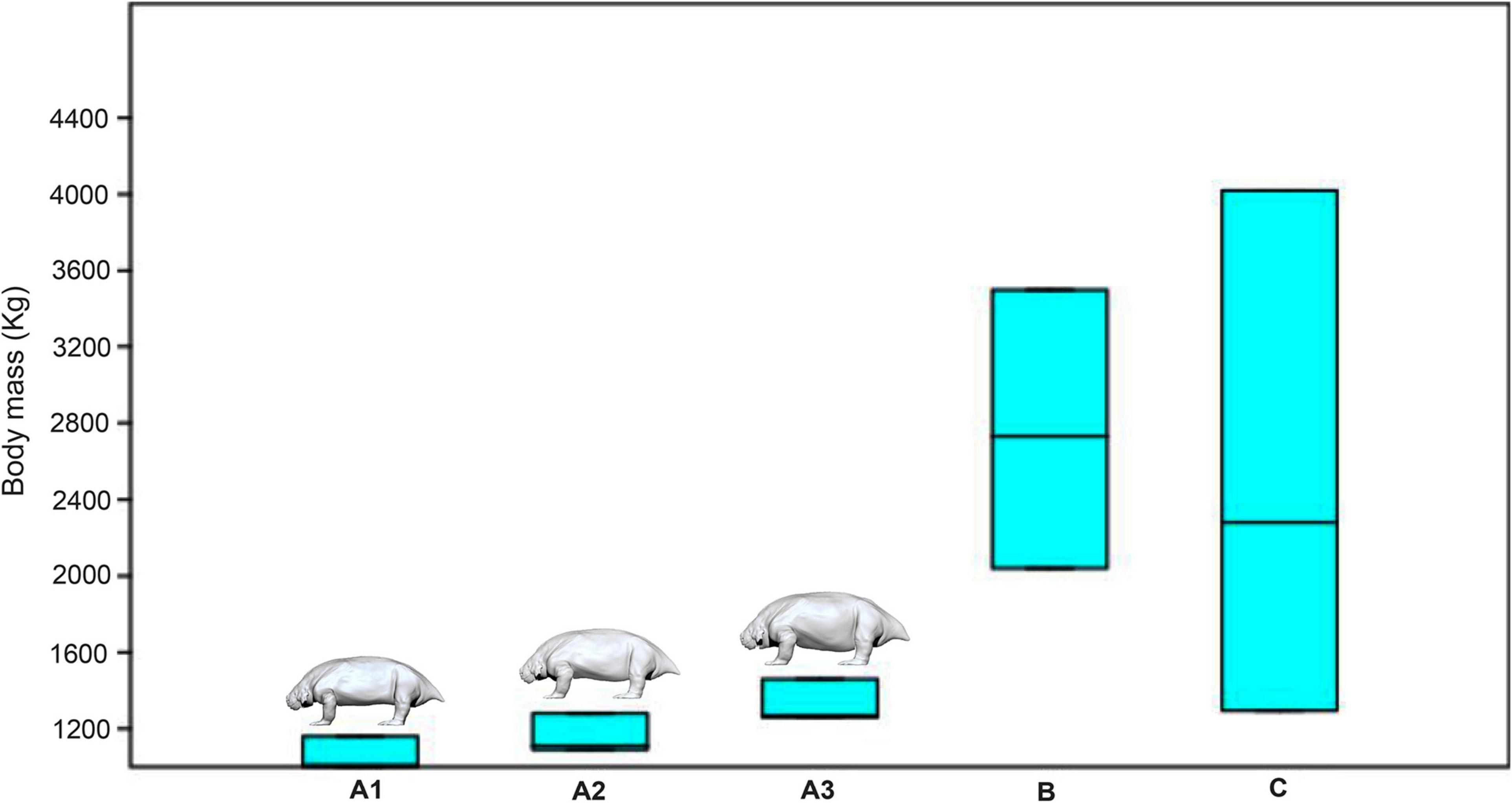
Figure 4. Box plot showing the range of body mass estimate in the “Slim model” (A1), “Average model” (A2), and “Fat model” (A3), in comparison with the range of weight calculated with the formula by Campione and Evans (2012) (B) and Anderson et al. (1985) (C).
Discussion and Conclusion
Pareiasaurs had a global distribution and have been described from several countries with the majority of species found in southern Africa, Russia, and China, and a few species from Niger, Morocco, Brazil, Scotland and Germany. It is increasingly apparent that they were an important component of Permian terrestrial ecosystems and biodiversity, not only in terms of numbers of specimens but also because of their large body size at that time. Despite this, relatively little is known about the palaeobiology of middle and late Permian species and how they achieved global distribution. South Africa has the most diverse pareiasaurian fauna in terms of number of species, and also has the oldest and most primitive members of the group, from the middle Permian Tapinocephalus Assemblage Zone of the Beaufort Group (Van den Brandt, 2020; Van den Brandt et al., 2021b).
The first pareiasaurs to appear are the three middle Permian genera from South Africa (Bradysaurus, Embrithosaurus, and Nochelesaurus) and they are all large forms, 2.5–3 m in body length, similar in size (and presumably weight) to Scutosaurus. Deltavjatia is the only other middle Permian pareiasaur and is medium-sized at about 2 m in length. The primitive condition for pareiasaurs, as shown by their abrupt first occurrences of multiple genera in South Africa, is therefore large. This is surprising and interesting as their closest relatives are much smaller animals (procolophonoids and “nycteroleters”) (Tsuji and Muller, 2009) and may indicate an extremely rapid initial size increase for pareiasaurs likely connected to the origin of herbivory, as already found in edaphosaurid synapsids (Brocklehurst and Brink, 2017). Over time, pareiasaur species tended to decrease in size since in the late Permian almost all members of the group are small to medium-sized forms. Only Scutosaurus in Russia and Pareiasaurus in South Africa are large members of the group in the late Permian. As part of his Ph.D. Lee (1994) produced an updated and extremely detailed cranial re-description for Scutosaurus karpinskii, using several adult and juvenile specimens. Lee also analyzed the hypothesized two sexually dimorphic body types (Hartmann-Weinberg, 1937; Lee, 1994:55–60) and found two definitive morphs based on size, justifying this on the basis of different sexes rather than different species. Lee (1994, 1997a) produced the most recent diagnosis, a comparative list of important cranial and postcranial features, and the first phylogenetic analysis for Scutosaurus. Lee (1996, 1997a) also produced a full body skeletal restoration of Scutosaurus karpinskii, based on the holotype PIN 2005/1532.
Pareiasaurs were some of the largest terrestrial tetrapods to have evolved a herbivorous diet in middle and late Permian ecosystems (Ivakhnenko, 1987, 2001; Sennikov, 1995, 1996; Lee, 1997a, 2000; Reisz and Sues, 2000; Boitsova et al., 2019). As stressed by Boitsova et al. (2019), despite the evident importance of this clade for the understanding of the first terrestrial ecosystems of the “modern” type and the abundance of finds in various countries, general knowledge about the biology of these animals is still limited. Within this framework, we determined the body mass of Scutosaurus. Size and body mass are of the most important biological aspects for organisms, and influence various vital aspects such as life span, general ecology, fecundity, physiology, metabolism, growth rate, locomotion, preferential diet and many others. In terms of macro-evolution it is important to understand when large size and body masses were first achieved by terrestrial vertebrates, and in this context the importance of a sound mass estimate for pareiasaurs is essential.
Using the high definition 3D photogrammetric model of the almost complete mounted skeleton PIN 2005/1537 from the Sokolki locality, we developed a 3D in vivo restoration of Scutosaurus karpinskii, resulting in a stocky animal with a large barrel-shaped rib cage (Figure 5). This conformation, typical of herbivorous tetrapods, is required to accommodate a large and long intestinal tract for digestion of celluloses and hemicelluloses (see Reisz and Sues, 2000; Lombardo, 2008; Hong et al., 2011; Romano, 2017a). In terms of physiology, two main models have been proposed in the literature as an explanation of the evolution of large body size in herbivorous lineages (see Brocklehurst and Brink, 2017): the abundance packet size hypothesis (Olsen, 2015) and the Jarman–Bell principle (Geist, 1974). According to the abundance packet size hypothesis diet transition is subsequent to change in body size, thus with the emergence of herbivory in larger taxa (see Brocklehurst and Brink, 2017). The model predicts a selective pressure toward a more abundant food source like plant material, since taxa with larger body sizes are characterized by greater energy requirements (Olsen, 2015). According to the Jarman–Bell principle, small animals are characterized by higher metabolic energy requirements with respect to their body size when compared to larger animals, even if the latter need greater absolute energy (Geist, 1974). Since low quality plant material such as leaves is more abundant than higher quality material (e.g., fruits, roots), the Jarman–Bell principle predicts an evolution of large body size in herbivorous clades. The above mentioned rapid initial size increase for pareiasaurs probably indicates that the Jarman–Bell principle provides a better explanation for the evolution of large body sizes in this clade, as already stressed for the evolution of large size in edaphosaurids by Brocklehurst and Brink (2017).
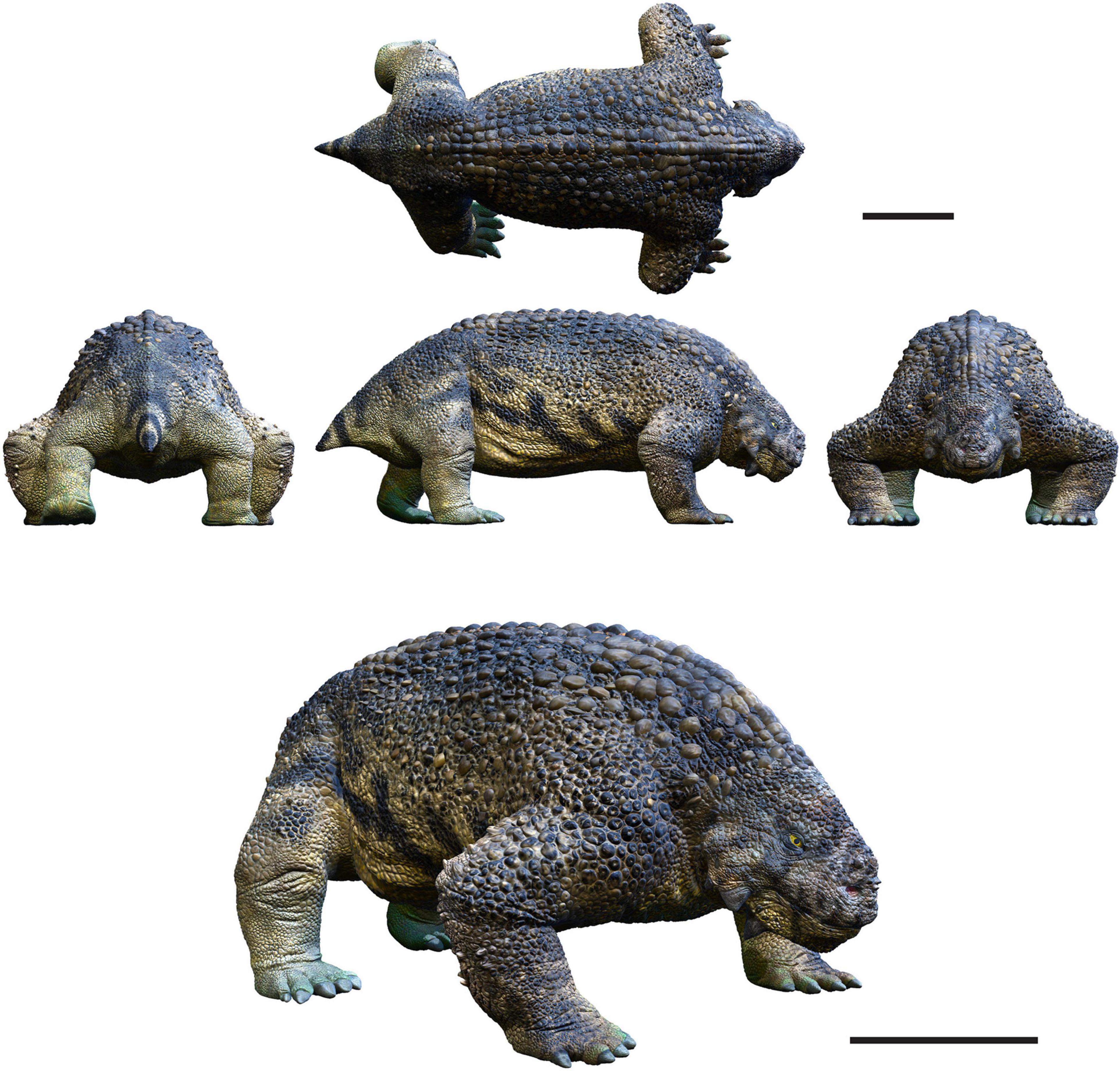
Figure 5. Artistic in vivo restoration of Scutosaurus karpinskii based on the 3D solid “average model” sculptured around the specimen PIN 2005/1537 from the Sokolki locality.
Following published literature we reconstructed Scutosaurus as having a traditional sprawling posture in both front and hind limbs as is the case in most pareiasaurs (Haughton and Boonstra, 1930; Boonstra, 1932; Lee, 1997a). Turner et al. (2015) discussed the forelimb posture of Bunostegos as implied by the degree of offset between the planes of the proximal and distal expansions of the humerus. They noted that most pareiasaurs have a value around 45 degrees of offset, inferring a sprawling posture, and proposed a more upright (and less sprawling) posture for Bunostegos since the humerus has proximal and distal expansions that are untwisted and are in line with each other (flat). In Scutosaurus the humeral torsion is 45 degrees (Lee, 1994, 1997a), implying a sprawling fore-limb posture (Lee, 1997a: 241, Figure 3B).
Haughton and Boonstra (1930) considered a relatively low or shallow femoral head expansion to represent the primitive condition and indicate a more horizontal femur orientation and a sprawling hind limb posture as in Bradysaurus, Embrithosaurus, and Nochelesaurus, which measured about 40 degrees relative to the substrate (Van den Brandt, 2020; Van den Brandt et al., 2021a,b). In Scutosaurus the femoral head expansion is also low (Turner et al., 2015, character 120) indicating a sprawling hind limb posture. However, the fore- and hind- limb posture has no effect on the total volume of the reconstruction, and thus does not affect the estimate of the body mass calculated in this paper.
Following the procedure proposed by Romano et al. (2021) we applied the living tissue densities of 0.99, 1, and 1.15 kg/1,000 cm3 to each of the three reconstructions. Lower densities, ranging from 0.8 to 0.85 kg/1,000 cm3, have been proposed in the literature for tetrapods characterized by an intense pneumatization of the postcranial skeleton, such as prosauropods and sauropods (Wedel, 2003, 2005; Gunga et al., 2008), but are not applicable to pareiasaurs. By applying the three densities to the “slim,” “average” and “fat model” we obtain an average body mass, respectively, of 1,060, 1,160, and 1,330 kg, with a total range for the estimated body weight from a minimum of one ton to a maximum of 1.46 tons. Taking into consideration the relative proportions between skeleton and soft parts in extant tetrapods, we consider the “average model” (Figure 3) as the most plausible reconstruction and close to the natural condition. We thus consider the most robust body mass estimate for Scutosaurus to be 1,160 kg, which is comparable to that of an adult black rhino or a large domestic cow.
As stressed in the text we prefer the volumetric method for body mass estimate, since the classic regression formulas based on long bone dimensions can lead to substantial under- or overestimation of the body weight of extinct tetrapods (see Sellers et al., 2012; Bates et al., 2015; Brassey et al., 2015; Larramendi, 2016; Romano and Manucci, 2019; Romano and Rubidge, 2019a; Romano et al., 2021). It has been empirically shown that the discrepancy between estimates obtained from regression formulas are greater when applied to clades phylogenetically distant from those used to construct the dataset (Brassey, 2016; Romano and Manucci, 2019; Romano and Rubidge, 2019a), and in particular to taxa with a “primitive” sprawling posture characterized by excessively “overbuilt” long bones (sensu Romano, 2017b; Romano and Rubidge, 2019b). The latter applies directly to Scutosaurus which has extremely strong and stocky long bones in proportion to their length, most likely to withstand the great torsional efforts caused by the sprawling posture. In fact, by using the regression formulae proposed by Campione and Evans (2012) and Anderson et al. (1985) we obtain a substantially overestimated body mass for Scutosaurus, up to 235% with respect to the average value obtained with the volumetric method (Figure 4), and relative densities of living tissues that are too high and unnatural. This contribution therefore once again supports the use of volumetric methods for body mass estimation in instances where sufficiently complete mounted skeletons are available, underlining the problem of obtaining overestimated values by applying regression formulae on extremely robust long bones.
This contribution demonstrates that amongst parareptiles barrel-shaped herbivores, which had a body mass of more than a ton and subsisted on a high fiber diet, had already evolved in the upper Palaeozoic, shedding new light on the structure of the first modern terrestrial ecosystems.
Data Availability Statement
The original contributions presented in the study are included in the article, further inquiries can be directed to the corresponding author/s.
Author Contributions
MR conceived the study, analyzed the specimen first hand, calculated the body masses, and draft the manuscript. MV and BR contributed to drafting of the manuscript. FM made the 3D sculptures and the in vivo reconstruction of Scutosaurus. All authors contributed to the article and approved the submitted version.
Funding
We acknowledge the financial support provided by the “Progetto di Ateneo, Sapienza University of Rome,” titled “A multidisciplinary approach to the study of tetrapod footprints: the Gardetta Plateau (Piedmont, Western Alps, Italy) and Sezze (Latium, Central Italy) case studies” (protocol number: RM120172B8D6EAAF) granted to MR, and the financial support provided by the DST/NRF Centre of Excellence in Palaeosciences (CoE-Pal). Opinions expressed and conclusions arrived at, are those of the authors and are not necessarily to be attributed to the CoE.
Conflict of Interest
The authors declare that the research was conducted in the absence of any commercial or financial relationships that could be construed as a potential conflict of interest.
Acknowledgments
We would like to thank Valeriy Golubev and Elena Boyarinova of the Borissiak Paleontological Institute, Russian Academy of Sciences (Moscow), for access to collections and for kind additional assistance, including very helpful comments that greatly improved the manuscript.
References
Alexander, R. M. (1985). Mechanics of posture and gait of some large dinosaurs. Zool. J. Linnean Soc. 83, 1–25. doi: 10.1111/j.1096-3642.1985.tb00871.x
Alexander, R. M. (1989). Dynamics of Dinosaurs and Other Extinct Giants. New York, NY: Columbia University Press.
Amalitsky, V. (1922). Diagnoses of the new forms of vertebrates and plants from the Upper Permian on North Dvina. Bull. Acad. Sci. Russ. VI Seìrie 16, 329–340.
Anderson, J. E., Hall-Martin, A., and Russell, D. A. (1985). Long bone circumference and weight in mammals, birds and dinosaurs. J. Zool. 207, 53–61. doi: 10.1111/j.1469-7998.1985.tb04915.x
Araujo, D. C. F. (1985). Sobre Pareiasaurus americanus sp. nov., do Permiano Superior do Rio Grande do Sul, Brasil. I- Diagnose específica. An. Acad. Bras. Cienc. 57, 63–66.
Arefiev, M. P., Golubev, V. K., Balabanov, Y. P., Karasev, E. V., Minikh, A. V., Minikh, M. G., et al. (2015). Type and Reference Sections of the Permian–Triassic Continental Sequences of the East European Platform: Main Isotope, Magnetic, and Biotic Events. XVIII International Congress on the Carboniferous and Permian. Sukhona and Severnaya Dvina Rivers field trip. Moscow: Borissiak Paleontological Institute RAS, 104.
Bates, K. T., Falkingham, P. L., Breithaupt, B. H., Hodgetts, D., Sellers, W. I., and Manning, P. L. (2009). How big was ‘Big Al’? Quantifying the effect of soft tissue and osteological unknowns on mass predictions for Allosaurus (Dinosauria: theropoda). Palaeontol. Electron. 12(14A), 1A–33A.
Bates, K. T., Falkingham, P. L., Macaulay, S., Brassey, C., and Maidment, S. C. (2015). Downsizing a giant: re-evaluating Dreadnoughtus body mass. Biol. Lett. 11:20150215. doi: 10.1098/rsbl.2015.0215
Benton, M. J. (2016). The Chinese pareiasaurs. Zool. J. Linnean Soc. 177, 813–853. doi: 10.1111/zoj.12389
Benton, M. J., Newell, A. J., Khlyupin, A. Y., Shumov, I. S., Price, G. D., and Kurkin, A. A. (2012). Preservation of exceptional vertebrate assemblages in Middle Permian fluviolacustrine mudstones of Kotel’nich, Russia: stratigraphy, sedimentology, and taphonomy. Palaeogeogr. Palaeoclimatol. Palaeoecol. 319-320, 58–83. doi: 10.1016/j.palaeo.2012.01.005
Boitsova, E. A., Skutschas, P. P., Sennikov, A. G., Golubev, V. K., Masuytin, V. V., and Masuytina, O. A. (2019). Bone histology of two pareiasaurs from Russia (Deltavjatia rossica and Scutosaurus karpinskii) with implications for pareiasaurian palaeobiology. Biol. J. Linn. Soc. 128, 289–310.
Boonstra, L. D. (1932). Pareiasaurian studies. Part 8. The osteology and myology of the locomotor apparatus. B – fore limb. Annls. S. Afr. Mus. 28, 437–503.
Boonstra, L. D. (1934a). Pareiasaurian studies. Part 10. The dermal armour. Annls. S. Afr. Mus. 31, 39–48.
Boonstra, L. D. (1934b). Pareiasaurian studies. Part 9. The cranial osteology. Annls. S. Afr. Mus. 31, 1–38. doi: 10.1127/njgpa/217/2000/1
Boonstra, L. D. (1969). The fauna of the Tapinocephalus zone (Beaufort beds of the Karoo). Annls. S. Afr. Mus. 56, 1–73.
Boyarinova, E. I., Bulanov, V. V., and Golubev, V. K. (2019). Significance of osteoderms for systematics of the Late Permian pareiasaurs of Eastern Europe. Kolner For. Geol. Palaont. 23, 54–55.
Brassey, C. A. (2016). Body-mass estimation in paleontology: a review of volumetric techniques. Paleont. Soc. Pap. 22, 133–156. doi: 10.1017/scs.2017.12
Brassey, C. A., Maidment, S. C. R., and Barrett, P. M. (2015). Body mass estimates of an exceptionally complete Stegosaurus (Ornithischia: thyreophora): comparing volumetric and linear bivariate mass estimation methods. Biol. Lett. 11:20140984. doi: 10.1098/rsbl.2014.0984
Brocklehurst, N., and Brink, K. S. (2017). Selection towards larger body size in both herbivorous and carnivorous synapsids during the Carboniferous. Facets 2, 68–84. doi: 10.1139/facets-2016-0046
Broom, R. (1912). On a new species of Propappus, and on the pose of the pareiasaurian limbs. Annls. S. Afr. Mus. 7, 323–331.
Broom, R. (1913). On four new fossil reptiles from the Beaufort Series, South Africa. Records Albany Mus. 2, 397–401.
Broom, R. (1914). Further observations on the South African fossil reptiles. Am. Mus. J. 14, 139–143.
Broom, R. (1924). On some points in the structure of the pareiasaurian skull. Proc. Zool. Soc. Lond. 94, 499–508. doi: 10.1111/j.1096-3642.1924.tb01510.x
Bulanov, V. V., and Yashina, O. V. (2005). Elginiid pareiasaurs of Eastern Europe. Paleontol. J. 39, 428–432.
Campione, N. E., and Evans, D. C. (2012). A universal scaling relationship between body mass and proximal limb bone dimensions in quadrupedal terrestrial tetrapods. BMC Biol. 10:60. doi: 10.1186/1741-7007-10-60
Cisneros, J. C., Abdala, F., and Malabarba, M. C. (2005). Pareiasaurids from the Rio do Rasto formation, southern Brazil: biostratigraphic implications for Permian faunas of the Parana basin. Rev. Bras. Paleontol. 8, 13–24. doi: 10.4072/rbp.2005.1.02
Clauss, M., Steuer, P., Müller, D. W., Codron, D., and Hummel, J. (2013). Herbivory and body size: allometries of diet quality and gastrointestinal physiology, and implications for herbivore ecology and dinosaur gigantism. PLoS One 8:e68714. doi: 10.1371/journal.pone.0068714
Davidowitz, G., and Nijhout, H. F. (2004). The physiological basis of reactionnorms: the interaction among growth rate, the duration of growth and body size. Integr. Comp. Biol. 44, 443–449. doi: 10.1093/icb/44.6.443
Day, M. O. (2013). Middle Permian Continental Biodiversity Changes as Reflected in the Beaufort Group of South Africa: a bio-and Lithostratigraphic Review of the Eodicynodon, Tapinocephalus, and Pristerognathus Assemblage Zones. 1–387. Ph.D thesis, University of the Witwatersrand, Johannesburg.
Day, M. O., Benson, R. B., Kammerer, C. F., and Rubidge, B. S. (2018). Evolutionary rates of mid-Permian tetrapods from South Africa and the role of temporal resolution in turnover reconstruction. Paleobiology 44, 347–367. doi: 10.1017/pab.2018.17
Day, M. O., Ramezani, J., Bowring, S. A., Sadler, P. M., Erwin, D. H., Abdala, F., et al. (2015). When and how did the terrestrial mid-Permian mass extinction occur? Evidence from the tetrapod record of the Karoo Basin, South Africa. Proc. R. Soc. B 282, 20150834. doi: 10.1098/rspb.2015.0834
Day, M. O., and Rubidge, B. S. (2020). Biostratigraphy of the tapinocephalus Assemblage Zone (Beaufort Group, Karoo Supergroup), South Africa. South Afr. J. Geol. 123, 149–164. doi: 10.25131/sajg.123.0012
Day, M. O., and Smith, R. M. H. (2020). Biostratigraphy of the Endothiodon Assemblage Zone (Beaufort Group, Karoo Supergroup), South Africa. South Afr. J. Geol. 123, 165–180. doi: 10.25131/sajg.123.0011
Fisher, J. T., Anholt, B., and Volpe, J. P. (2011). Body mass explains characteristic scales of habitat selection in terrestrial mammals. Ecol. Evol. 1, 517–528. doi: 10.1002/ece3.45
Gao, K. (1989). Pareiasaurs from the Upper Permian of north China. Can. J. Earth Sci. 26, 1234–1240. doi: 10.1139/e89-104
Geist, V. (1974). On the relationship of social evolution and ecology in ungulates. Am. Zool. 14, 205–220. doi: 10.1093/icb/14.1.205
Gillooly, J. F., Allen, A. P., and Charnov, E. L. (2006). Dinosaur fossils predict body temperatures. PLoS Biol. 4:e248. doi: 10.1371/journal.pbio.0040248
Gunga, H. C., Kirsch, K., Baartz, F., and ö, L. (1995). New data on the dimensions of Brachiosaurus brancai and their physiological implications. Naturwissenschaften 82, 190–192. doi: 10.1007/s001140050167
Gunga, H. C., Suthau, T., Bellmann, A., Friedrich, A., Schwanebeck, T., Stoinski, S., et al. (2007). Body mass estimations for Plateosaurus engelhardti using laser scanning and 3D reconstruction methods. Naturwissenschaften 94, 623–630. doi: 10.1007/s00114-007-0234-2
Gunga, H. C., Suthau, T., Bellmann, A., Stoinski, S., Friedrich, A., Trippel, T., et al. (2008). A new body mass estimation of Brachiosaurus brancai Janensch, 1914 mounted and exhibited at the Museum of Natural History (Berlin, Germany). Foss. Rec. 11, 33–38. doi: 10.1002/mmng.200700011
Hartmann-Weinberg, A. P. (1930). Zur Systermatik der Nord-Duna-Pareiasauridae. Palaontol. Z. 12, 47–59. doi: 10.1007/bf03045064
Haughton, S. H., and Boonstra, L. D. (1929). Pareiasaurian studies. Part I. An attempt at a classification of the pareiasauria based on skull features. Annls. S. Afr. Mus. 28, 79–87.
Haughton, S. H., and Boonstra, L. D. (1930). Pareiasaurian studies. Part 6. The osteology and myology of the locomotor apparatus. A – hind limb. Annls. S. Afr. Mus. 28, 296–366.
Henderson, D. M. (1999). Estimating the masses and centers of masses of extinct animals by 3-D mathematical slicing. Paleobiology 25, 88–106.
Hong, P. Y., Wheeler, E., Cann, I. K., and Mackie, R. I. (2011). Phylogenetic analysis of the fecal microbial community in herbivorous land and marine iguanas of the Galápagos Islands using 16S rRNA-based pyrosequencing. ISME J. 5, 1461–1470. doi: 10.1038/ismej.2011.33
Hurlburt, G. (1999). Comparison of body mass estimation techniques, using recent reptiles and the pelycosaur Edaphosaurus boanerges. J. Vertebr. Paleontol. 19, 338–350. doi: 10.1080/02724634.1999.10011145
Hutchinson, J. R., Ng-Thow-Hing, V., and Anderson, F. C. (2007). A 3D interactive method for estimating body segmental parameters in animals: application to the turning and running performance of Tyrannosauus rex. J. Theor. Biol. 246, 660–680. doi: 10.1016/j.jtbi.2007.01.023
Ivakhnenko, M. F. (1987). Permian parareptiles of the USSR. Trudy Paleontol. Inst. Akademii Nauk SSSR 223, 1–159.
Ivakhnenko, M. F. (2001). Tetrapods from the East European Placket, a Late Paleozoic natural territorial complex. Trudy Paleontol. Instituta Rossiiskoi Akademii Nauk 283, 1–200.
Ivakhnenko, M. F. (2008). “Subclass parareptilia,” in Fossil Vertebrates of Russia and Adjacent Countries. Fossil Reptiles and Birds. Part 1, eds M. F. Ivakhnenko and E. N. Kurochkin (Moscow: Geos), 48–100.
Ivakhnenko, M. F., Golubev, V. K., Gubin, Y. M., Kalandadze, N. N., Sennikov, A. G., and Rautian, A. S. (1997). Permian and Triassic Tetrapods of Eastern Europe. Moscow: Transactions of the Paleontological Institute of the Russian Academy of Sciences, 216.
Jalil, N.-E., and Janvier, P. (2005). Les pareiasaures (Amniota, Parareptilia) du Permien superieur du Bassin d’Argana, Maroc. Geodiversitas 27, 35–132.
Kuhn, O. (1969). Cotylosaria. Handbuch der Palaoherpetologie, Teil 6. Stuttgart: Gustav Fischer Verlag.
LaBarbera, M. (1989). Analyzing body size as a factor in ecology and evolution. Annu. Rev. Ecol. Evol. Syst. 20, 97–117. doi: 10.1146/annurev.es.20.110189.000525
Larramendi, A. (2016). Shoulder height, body mass, and shape of proboscideans. Acta Palaeontol. Pol. 61, 537–574.
Lee, M. S. (2000). “The Russian pareiasaurs,” in The Age of Dinosaurs in Russia and Mongolia, eds M. J. Benton, M. A. Shishkin, D. M. Unwin, and E. N. Kurochkin (Cambridge: Cambridge University Press), 78–85.
Lee, M. S. Y. (1993). The origin of the turtle body plan: bridging a famous morphological gap. Science 261, 1716–1720. doi: 10.1126/science.261.5129.1716
Lee, M. S. Y. (1994). Evolutionary Morphology of Pareisaurs. 1–392. Ph.D thesis, University of Cambridge, Cambridge.
Lee, M. S. Y. (1996). Correlated progression and the origin of turtles. Nature 379, 812–815. doi: 10.1038/379812a0
Lee, M. S. Y. (1997b). Pareiasaur phylogeny and the origin of turtles. Zool. J. Linnean Soc. 120, 197–280. doi: 10.1111/j.1096-3642.1997.tb01279.x
Lee, M. S. Y. (1997a). A taxonomic revision of pareiasaurian reptiles: implications for Permian terrestrial palaeoecology. Mod. Geol. 21, 231–298.
Liu, J., and Bever, G. S. (2018). The tetrapod fauna of the upper permian naobaogou formation of China: a new species of Elginia (Parareptilia, Pareiasauria). Pap. Palaeontol. 4:197209.
Lombardo, M. P. (2008). Access to mutualistic endosymbiotic microbes: an underappreciated benefit of group living. Behav. Ecol. Sociobiol. 62, 479–497. doi: 10.1007/s00265-007-0428-9
Maisch, M., and Matzke, A. T. (2019). Anthodon? haughtoni (v. Huene, 1944), a pareiasaurid (Parareptilia: Pareiasauria) from the Late Permian Usili Formation of Kingori, Ruhuhu Basin, Tanzania. Neues Jahrb Geol. Palaontol. Abh. 29, 197–204. doi: 10.1127/njgpa/2019/0796
Martin, A. P., and Palumbi, S. R. (1993). Body size, metabolic rate, generation time, and the molecular clock. Proc. Natl. Acad Sci. U.S.A. 90, 4087–4091. doi: 10.1073/pnas.90.9.4087
Millar, J. S., and Zammuto, R. M. (1983). Life histories of mammals: an analysis of life tables. Ecology 64, 631–635. doi: 10.2307/1937181
Newton, E. T. (1893). On some new reptiles from the Elgin sandstones. Philos. Trans. R. Soc. B 184, 431–503. doi: 10.1098/rstb.1893.0007
Olsen, A. M. (2015). Exceptional avian herbivores: multiple transitions toward herbivory in the bird order Anseriformes and its correlation with body mass. Ecol. Evol. 5, 5016–5032. doi: 10.1002/ece3.1787
Owen, R. (1876). Descriptive and Illustrated Catalogue of the Fossil Reptilia of South Africa in the Collection of the British Museum. London: British Museum (Natural History).
Reisz, R. R., and Sues, H. D. (2000). “Herbivory in late Paleozoic and Triassic terrestrial vertebrates,” in Evolution of Herbivory in Terrestrial Vertebrates, ed. H.-D. Sues (Cambridge: Cambridge University Press), 9–41. doi: 10.1017/CBO9780511549717.003
Romano, M. (2017a). Gut microbiota as a trigger of accelerated directional adaptive evolution: acquisition of herbivory in the context of extracellular vesicles, microRNAs and inter-kingdom crosstalk. Front. Microbiol. 8:721. doi: 10.3389/fmicb.2017.00721
Romano, M. (2017b). Long bone scaling of caseid synapsids: a combined morphometric and cladistic approach. Lethaia 50, 511–526. doi: 10.1111/let.12207
Romano, M., and Manucci, F. (2019). Resizing Lisowicia bojani: volumetric body mass estimate and 3D reconstruction of the giant Late Triassic dicynodont. Hist. Biol. 33, 474–479. doi: 10.1080/08912963.2019.1631819
Romano, M., Manucci, F., and Palombo, M. R. (2021). The smallest of the largest: new volumetric body mass estimate and in-vivo restoration of the dwarf elephant Palaeoloxodon ex gr. P. falconeri from Spinagallo Cave (Sicily). Hist. Biol. 33, 340–353. doi: 10.1080/08912963.2019.1617289
Romano, M., and Nicosia, U. (2014). Alierasaurus ronchii, gen. et sp. nov., a caseid from the Permian of Sardinia, Italy. J. Vertebr. Paleontol. 34, 900–913. doi: 10.1080/02724634.2014.837056
Romano, M., and Nicosia, U. (2015). Cladistic analysis of Caseidae (Caseasauria, Synapsida): using Gap-Weighting Method to include taxa based on poorly known specimens. Palaeontology 58, 1109–1130. doi: 10.1111/pala.12197
Romano, M., and Rubidge, B. (2019b). Long bone scaling in Captorhinidae: do limb bones scale according to elastic similarity in sprawling basal amniotes? Lethaia 52, 389–402. doi: 10.1111/let.12319
Romano, M., and Rubidge, B. S. (2019a). First 3D reconstruction and volumetric body mass estimate of the tapinocephalid dinocephalian Tapinocaninus pamelae (Synapsida: Therapsida). Hist. Biol. 33, 498–505. doi: 10.1080/08912963.2019.1640219
Rubidge, B. S., Govender, R., and Romano, M. (2019). The postcranial skeleton of the basal tapinocephalid dinocephalian Tapinocaninus pamelae (Synapsida: Therapsida) from the South African Karoo Supergroup. J. Syst. Palaeontol. 17, 1767–1789. doi: 10.1080/14772019.2018.1559244
Scheyer, T. M., and Sander, P. M. (2009). Bone microstructures and mode of skeletogenesis in osteoderms of three pareiasaur taxa from the Permian of South Africa. J. Evol. Biol 22, 1153–1162. doi: 10.1111/j.1420-9101.2009.01732.x
Seeley, H. G. (1888). Researches on the structure, organisation, and classification of the fossil Reptilia. II. On Pareiasaurus bombidens (Owen), and the significance of its affinities to amphibians, reptiles and mammals. Philos. Trans. R. Soc. B 179, 59–109. doi: 10.1098/rstb.1888.0003
Seeley, H. G. (1892). Researches on the structure, organisation, and classification of the fossil Reptilia. VII. Further observations on Pareiasaurus. Philos. Trans. R. Soc. B 183, 311–370. doi: 10.1098/rstb.1892.0008
Seitz, S. M., Curless, B., Diebel, J., Scharstein, D., and Szeliski, R. (2006). “A comparison and evaluation of multi-view stereo reconstruction algorithms,” in Proceedings of the 2006 IEEE Computer Society Conference on Computer Vision and Pattern Recognition (CVPR”06) 0–7695–2597-0/06,1,519528, (New York, NY).
Sellers, W. I., Hepworth-Bell, J., Falkingham, P. L., Bates, K. T., Brassey, C. A., Egerton, V. M., et al. (2012). Minimum convex hull mass estimations of complete mounted skeletons. Biol Lett. 8, 842–845. doi: 10.1098/rsbl.2012.0263
Sennikov, A. G. (1995). The early thecodonts of Eastern Europe. Trudy Paleontol. Instituta Rossiiskoi Akademii Nauk 263, 1–142.
Sennikov, A. G. (1996). Evolution of the Permian and Triassic tetrapod communities of Eastern Europe. Palaeogeogr. Palaeoclimatol. Palaeoecol. 120, 331–351. doi: 10.1016/0031-0182(95)00041-0
Sidor, C. A., Blackburn, D. C., and Gabo, B. (2003). The vertebrate fauna of the Upper Permian of Niger –II, Preliminary description of a new pareiasaur. Palaeontol. Africana 39, 45–52.
Sinclair, A. R. E., Mduma, S., and Brashares, J. S. (2003). Patterns of predation in a diverse predator-prey system. Nature 425, 288–290. 10.1038/nature01934
Smith, R. M. H. (2020). Biostratigraphy of the Cistecephalus Assemblage Zone (Beaufort Group, Karoo Supergroup), South Africa. S. Afr. J. Geol. 123, 181–190. doi: 10.25131/sajg.123.0013
Tsuji, L. A. (2010). Evolution, Morphology and Paleobiology of the Pareiasauria and their Relatives (Amniota: Parareptilia). 1–188. Ph.D thesis, Humboldt- University of Berlin, Berlin.
Tsuji, L. A. (2013). Anatomy, cranial ontogeny and phylogenetic relationships of the pareiasaur Deltavjatia rossicus from the Late Permian of central Russia. Earth Environ. Sci. Trans. Roy. Soc. Edinb. 104, 81–122. doi: 10.1017/s1755691013000492
Tsuji, L. A., and Muller, J. (2009). Assembling the history of the Parareptilia: phylogeny, diversification, and a new definition of the clade. Foss. Rec. 12, 71–81. doi: 10.1002/mmng.200800011
Tsuji, L. A., Sidor, C. A., Steyer, S. J., Smith, R. M. H., Tabor, N. J., and Ide, O. (2013). The vertebrate fauna of the upper permian of niger –VII. Cranial anatomy and relationships of Bunostegos akokanenis (Pareiasauria). J. Vertebr. Paleontol. 33, 747–763. doi: 10.1080/02724634.2013.739537
Turner, M. L., Tsuji, L. A., Ide, O., and Sidor, C. A. (2015). The vertebrate fauna of the Upper Permian of Niger –IX. The appendicular skeleton of Bunostegos akokanenis (Parareptilia: Pareiasauria). J. Vertebr. Paleontol 35, e994746. doi: 10.1080/02724634.2014.994746
Ullman, S. (1979). The interpretation of structure from motion. Proc. R. Soc. Lond. B Biol. Sci. 203, 405–426.
Van den Brandt, M. J. (2020). Morphology, Taxonomy, Phylogeny and Stratigraphic Ranges of South African Middle Permian Pareiasaurs. 565. Ph.D thesis, University of the Witwatersrand, Johannesburg.
Van den Brandt, M. J., Abdala, F., Benoit, J., Day, M. O., and Rubidge, B. S. (2021b). Taxonomy, phylogeny and stratigraphic ranges of middle Permian pareiasaurs from the Karoo Basin of South Africa. J. Syst. Palaeontol, in press.
Van den Brandt, M. J., Abdala, F., and Rubidge, B. S. (2020). Cranial morphology and phylogenetic relationships of the Middle Permian pareiasaur Embrithosaurus schwarzi from the Karoo Basin of South Africa. Zool. J. Linnean Soc. 188, 202–241.
Van den Brandt, M. J., Benoit, J., Abdala, F., and Rubidge, B. S. (2021a). Postcranial morphology of the South African middle Permian pareiasaurs from the Karoo Basin of South Africa. Palaeontolo. africana. 55, 1–9, Available online at: https://hdl.handle.net/10539/31290
Viglietti, P. A. (2020). Biostratigraphy of the Daptocepahlus Assemblage Zone (Beaufort Group, Karoo Supergroup), South Africa. S. Afr. J. Geol. 123, 191–206. doi: 10.25131/sajg.123.0014
von Huene, F. F. (1944). Pareiasaurierreste aus dem Ruhuhu-Gebiet. Palaeontol. Z 23, 386–410. doi: 10.1007/BF03160446
Wedel, M. J. (2003). Vertebral pneumaticity, air sacs, and the physiology of sauropod dinosaurs. Paleobiology 29, 243–255. doi: 10.1666/0094-8373(2003)029<0243:vpasat>2.0.co;2
Wedel, M. J. (2005). “Postcranial skeletal pneumaticity in sauropods and its implications for mass estimates,” in The Sauropods: evolution and Paleobiology, eds J. A. Wilson and K. Curry-Rogers (Berkeley, CA: University of California Press), 201–228. doi: 10.1525/9780520932333-010
Xu, L., Li, X. W., Jia, S. H., and Liu, J. (2015). The Jiyuan Tetrapod Fauna of the Upper Permian of China: New Pareiasaur Material and the Reestablishment of Honania complicidentata. Acta Palaeontol. Pol. 60, 689–700.
Keywords: Scutosaurus, pareiasaurs, Parareptilia, Palaeozoic tetrapods, Permian, body mass estimate
Citation: Romano M, Manucci F, Rubidge B and Van den Brandt MJ (2021) Volumetric Body Mass Estimate and in vivo Reconstruction of the Russian Pareiasaur Scutosaurus karpinskii. Front. Ecol. Evol. 9:692035. doi: 10.3389/fevo.2021.692035
Received: 07 April 2021; Accepted: 21 May 2021;
Published: 17 June 2021.
Edited by:
Mark Joseph MacDougall, Museum of Natural History Berlin (MfN), GermanyReviewed by:
Michael James Benton, University of Bristol, United KingdomNeil Brocklehurst, University of Oxford, United Kingdom
Copyright © 2021 Romano, Manucci, Rubidge and Van den Brandt. This is an open-access article distributed under the terms of the Creative Commons Attribution License (CC BY). The use, distribution or reproduction in other forums is permitted, provided the original author(s) and the copyright owner(s) are credited and that the original publication in this journal is cited, in accordance with accepted academic practice. No use, distribution or reproduction is permitted which does not comply with these terms.
*Correspondence: Marco Romano, bWFyY28ucm9tYW5vQHVuaXJvbWExLml0