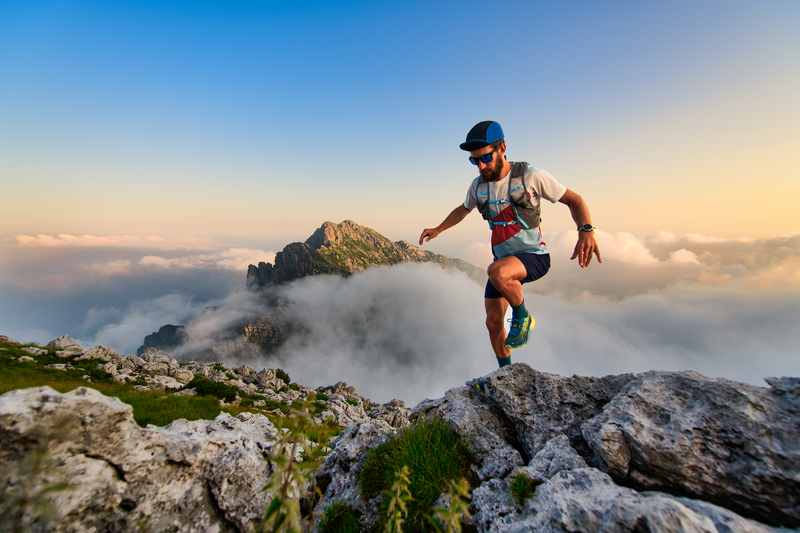
95% of researchers rate our articles as excellent or good
Learn more about the work of our research integrity team to safeguard the quality of each article we publish.
Find out more
REVIEW article
Front. Ecol. Evol. , 15 June 2021
Sec. Biogeography and Macroecology
Volume 9 - 2021 | https://doi.org/10.3389/fevo.2021.689192
This article is part of the Research Topic Predicting and Managing Climate-Driven Range Shifts in Plants View all 12 articles
Climate warming is predicted to shift species’ ranges as previously uninhabitable environments just beyond the leading range edges become suitable habitat and trailing range edges become increasingly unsuitable. Understanding which aspects of the environment and species traits mediate these range shifts is critical for understanding species’ possible redistributions under global change, yet we have a limited understanding of the ecological and evolutionary responses underlying population spread or extinction at species’ range edges. Within plant populations, shifts in flowering phenology have been one of the strongest and most consistent responses to climate change, and are likely to play an important role in mediating population dynamics within and beyond species’ ranges. However, the role of phenological shifts, and particularly phenological plasticity, in species’ range shifts remains relatively unstudied. Here, we synthesize literature on phenology, plasticity, and adaptation to suggest ways in which phenological responses to climate may vary across species’ ranges and review the empirical evidence for and against these hypotheses. We then outline how phenological plasticity could facilitate or hinder persistence and potential consequences of phenological plasticity in range expansions, including phenological cues, shifts in correlated traits, altered species interactions, and effects on gene flow. Finally, we suggest future avenues for research, such as characterizing reaction norms for phenology across a species’ range and in beyond-the-range transplant experiments. Given the prevalence and magnitude of phenological shifts, future work should carefully dissect its costs and benefits for population persistence, and incorporate phenological plasticity into models predicting species’ persistence and geographic range shifts under climate change.
Species’ geographic distributions are shifting due to climate change, but we often do not know the mechanisms underlying species’ range shifts (Gaston, 2009) (see Glossary for bolded terms). Species may initially respond to novel environmental conditions via plastic responses (Ghalambor et al., 2007), either due to shifting climate conditions in situ or by encountering novel environmental conditions during range expansion. Distributional limits and range contractions should then occur where plasticity fails to enable establishment and/or long-term persistence (Pigliucci, 2001). One of the most commonly observed plastic responses to climate change is shifts in phenology, or the timing of life-cycle events. Given its prevalence, phenological plasticity is likely to play a critical role in shaping species’ responses to ongoing climate change (Münzbergová et al., 2017). Therefore, phenological plasticity will likely influence spatial dynamics of persistence, range expansion, or local extirpation, but the consequences of phenological plasticity at species’ range limits and beyond the range edge are relatively unstudied (Ensing and Eckert, 2019).
A fundamental question in range shifts is whether phenological plasticity can facilitate population spread and contribute to population persistence under novel environmental conditions (Richter et al., 2012; Urban et al., 2016). Most studies assume that phenological plasticity will promote establishment, population persistence, and population growth, but phenological plasticity could either facilitate or hinder population persistence in novel conditions. Specifically, phenological plasticity could “pre-adapt” populations to conditions beyond the leading range edge (i.e., the expanding or colonizing margin of a species’ distribution) or act to stabilize populations at the trailing range edge if plasticity shifts the phenotype in the same direction that would be favored in the novel environment (i.e., adaptive phenotypic plasticity (Ghalambor et al., 2007; Soularue and Kremer, 2012). This is most often considered with respect to changes in mean climate conditions, but adaptive plasticity may also serve a critical role in enabling population persistence under increasingly variable environmental conditions with climate change (IPCC, 2014). Alternatively, phenological plasticity could be maladaptive, such as advancing flowering that risks damage from exposure to frost (Cooper et al., 2019). Maladaptive phenological plasticity would reduce fitness in novel environments and contribute to range contractions (Ghalambor et al., 2007; Ensing and Eckert, 2019). Maladaptive plasticity may be particularly likely in the context of climate change if environmental cues or species interactions become disrupted. For example, mismatches with chilling requirements or mutualists during range expansions could cause historically adaptive patterns of phenological plasticity to become maladaptive in novel environments (Valladares et al., 2014; Uelmen et al., 2016).
An added complexity is the potential for populations across a species’ range to differ in the magnitude or even perhaps direction of phenological plasticity. Because edge populations often experience more extreme or variable environments than central populations (Chevin and Lande, 2011; Lázaro-Nogal et al., 2015; but see Volis et al., 1998; Mägi et al., 2011), they may have evolved greater phenological plasticity than central populations to better track the window of suitable conditions from year to year. As such, leading edge populations (i.e., poleward or higher latitude or elevation) may be poised to expand into novel environmental conditions beyond the contemporary range edge (Hargreaves and Eckert, 2019). Trailing edge populations (i.e., equatorial or lower latitude or elevation), meanwhile, could harbor phenological plasticity in response to temperature or adaptive genetic variation that could benefit migration of genotypes poleward within the historical range (Hampe and Petit, 2005). Furthermore, either leading or trailing edge populations could persist in situ via phenological plasticity to changing climate conditions. Alternatively, edge populations may actually exhibit more canalized phenology, limiting plastic responses to environmental change, due to local adaptation to harsh environmental conditions (e.g., cold temperatures or drought) or, in the case of leading edge populations, shorter growing seasons (Gugger et al., 2015). Despite these conflicting theories, we have little empirical evidence for how patterns of phenological plasticity vary across species ranges (Eckert et al., 2008) or about the consequences of phenological plasticity in range shifts.
Finally, phenological plasticity can have consequences for longer-term persistence beyond the range edge, but studies rarely consider the costs and benefits of plasticity in range expansion beyond initial establishment. For example, phenological plasticity may have reproductive or demographic costs (Colautti et al., 2010; Reed et al., 2010) or involve correlated shifts in other life history traits such as resource acquisition (Sheth and Angert, 2016) or other phenological stages like germination or fruiting (Haggerty and Galloway, 2011). In addition, phenological plasticity likely affects biotic interactions (Benning et al., 2019) and patterns of gene flow (Weis, 2015) in ways that could either impede or facilitate persistence and adaptation to novel conditions. Each of these consequences may cause any benefits of phenological plasticity during range shifts to be ephemeral, but they are rarely considered despite their potential importance in determining whether phenological plasticity could enable not only expansion but persistence beyond the range edge.
Given the growing recognition of the role that phenological plasticity plays in range limits (Griffith and Watson, 2005; Morin et al., 2008; Wadgymar et al., 2015; Chapman et al., 2017), more forecasts of species range shifts under climate change that incorporate phenology are likely to appear. Now is a timely moment to synthesize the evidence for variation in phenological plasticity across and beyond species’ ranges, identify potential consequences of phenological plasticity in range shifts, and identify future directions for incorporating phenological plasticity into forecasts of species ranges under climate change.
We first review the evidence for geographic patterns in phenology across species’ ranges to test specific hypotheses related to the role of phenology in climate-mediated range shifts. We focus on evaluating the evidence for how plasticity and local adaptation shape geographic clines in phenology (H1) as well as whether the magnitude of phenological plasticity varies across species’ ranges (H2). We also address the evidence for two other interesting but less-studied hypotheses: the potential for phenological plasticity to either stabilize range edges or contribute to range shifts (H3) and variation in adaptive potential in phenology across species’ ranges (H4). Literature review: We searched ISI Web of Science on December 1, 2020, for studies that compared variation in phenology across a species’ geographic range (see Supplementary Appendices S1–S3 for methods and data). Specifically, we searched for empirical studies that explicitly compared at least one phenological variable between two or more populations that differed in range position, such as across latitudinal or elevation gradients. This requirement potentially excluded studies examining phenology at multiple sites that did not test for geographic differences in phenology and long-term studies from single localities (see Wolkovich et al., 2012; Thackeray et al., 2016; Tansey et al., 2017; Chmura et al., 2019; Piao et al., 2019 for syntheses of long-term studies). This yielded 107 studies, including data for a total of 300 phenological variables across 234 plants (45 unique forb and 44 unique tree species) 53 insects (43 species), 7 mammal (5 species), 5 bird (3 species), and 1 amphibian species. For each study, we evaluated results for each phenological variable (hereafter “phenophases”) examined across two or more populations of a given species in a given dataset (hereafter “cases”). In 117 cases, phenology was examined observationally in natural populations, while in 183 cases phenology was examined under experimental settings (Figure 1). The studies reviewed had a mean population number of 20.73 (median = 8; range = 2–240; values exclude one study with thousands of sites (Roy et al., 2015; Supplementary Appendix 4 Figure A1). The majority of latitudinal studies were at a continental or country-wide scale (>1000 km), while elevational studies spanned hundreds of meters. We grouped phenophases into four categories: emergence (23 cases), growth and development (155 cases), reproduction (97 cases), and senescence/autumn phenology (25 cases) (see Supplementary Appendix 1 Table A1 for phenophase definitions).
Figure 1. Frequency of each type of study conducted across each phenophase, including emergence (red), growth and development (green), reproduction (blue), and senescence or autumn phenology (purple). Studies were considered observational or experimental, the latter including resurrection studies, reciprocal transplants, controlled environments (i.e., greenhouse or growth chambers), controlled crosses, common gardens, or beyond the range transplants. Note that values here represent cases, or separate datasets, such that a single publication could include multiple types of cases (e.g., data from natural populations, a reciprocal transplant experiment, and a controlled environment greenhouse experiment).
We used these studies to evaluate support for eight hypotheses related to the role of phenological plasticity in range shifts (Figure 2). In each subsequent section, we first describe the conceptual motivation for each hypothesis and then evaluate the fraction of relevant cases that support, do not support, or yield mixed evidence for that hypothesis. We note that this qualitative approach does not address the magnitude of particular patterns or statistically account for non-independence among cases due to publication or study system (Gurevitch et al., 2001), but is most flexible given the wide range of approaches and metrics reported in these papers which precludes quantitative comparison. The majority of studies identified temperature as the primary climatic factor varying across geographic gradients, but in the handful of cases that focused on precipitation (e.g., drought, snowmelt), we characterized patterns with respect to spatial precipitation gradients (e.g., Bender et al., 2002; Eckhart et al., 2004; Torres-Martínez et al., 2017). We therefore focus our hypotheses on geographic and associated temperature and precipitation gradients. We recognize that these hypotheses are necessarily simplistic and that many ecological and evolutionary factors (e.g., photoperiod, soil characteristics, microclimatic variation, species interactions, etc.) could influence phenological patterns across geographic gradients (see Section “Costs and Benefits of Phenological Plasticity Beyond the Range Edge”). However, these broad patterns should be most generalizable for making predictions about the role of phenology in geographic range shifts across a variety of species.
Figure 2. Conceptual diagram illustrating hypotheses for the potential role of phenological plasticity in range shifts under climate change. (H1) Each line indicates the slope of phenological responses across a geographic gradient spanning (a,c) the trailing (equatorial/low elevation) to leading (poleward/high elevation) edge or (b) an associated environmental gradient wherein trailing edge populations experience warmer, drier conditions while leading edge populations experience colder, wetter conditions. (H1a) Phenology demonstrates a geographic cline in wild populations; later onset of spring and colder environments at high latitude and elevation should result in delayed emergence, slower growth and development, and delayed reproduction (solid line), and earlier senescence (dashed line) in leading relative to trailing edge populations. (H1b) Phenology demonstrates adaptive plasticity, such that individual populations grown in common conditions respond to environmental variation (i.e., reaction norm) by altering phenology in a way that is consistent with geographic clines in H1a (note that warmer populations are on the left side of the axis, consistent with the trailing edge). (H1c) Phenology demonstrates co-gradient genetic variation (solid line), such that populations grown in common environments demonstrate genetic differences mirroring geographic clines (vs. counter-gradient genetic variation; dotted line). (Expectations for spring phenology shown.) (H2) Each line represents the reaction norm for a single population (solid = central; trailing = dotted; dashed = leading) grown under contrasting environmental conditions (warm/dry vs. cool/wet) (e.g., in common gardens or climate manipulations). (H2a) The magnitude of phenological plasticity (i.e., responsiveness to environmental variation) is greater in populations from range edges vs. centers, and (H2b) from leading edges vs. trailing edges. (H3) Boxes show the range shift of a population in space. Phenological plasticity may allow populations to suitably shift their phenology to persist in situ (i.e., “range stability,” light gray boxes) or to expand beyond the range edge (white box). Alternatively, local adaptation in phenology can drive range shifts by causing populations to track suitable climate for a given phenology in space (black boxes). (H4a) Adaptive potential in phenology (i.e., genetic variance or heritability) is greater in populations from range centers vs. edges, and (H4b) from leading vs. trailing edges.
We first addressed general geographic patterns in phenology across species’ ranges and their contribution from plasticity and genetic adaptation. Later onset of spring and colder environments at high latitude and elevation should result in delayed emergence, slower growth and development, delayed reproduction, and earlier senescence in nature (Figure 2: H1a). However, these natural clines may be driven by some combination of phenological plasticity and/or genetic adaptation of local populations. For example, single populations may exhibit plasticity in phenological traits that mimic natural gradients; this would be consistent with adaptive plasticity in which individual populations respond to environmental variation by altering phenology to match that observed in local populations (Figure 2: H1b) (Soularue and Kremer, 2012; Ensing and Eckert, 2019). Similarly, populations across the range may have genetically based differences in phenology when grown in common environmental conditions. Co-gradient genetic variation occurs if genetic differences mimic the natural cline, whereas genetic differences that oppose the natural cline (“counter-gradient genetic variation,” sensu Conover, 1990; Conover and Schultz, 1995) can evolve to either minimize changes in traits across the natural gradient or compensate for maladaptive plasticity (Figure 2: H1c). For example, populations in colder climates may evolve more rapid development to compensate for shorter growing seasons (Eckhart et al., 2004; Conover et al., 2009).
We first evaluated the hypothesis that populations across a geographic gradient should vary in phenology such that leading edge populations have delayed emergence, growth, and reproduction and earlier senescence relative to trailing edge populations (H1a). To test this hypothesis, we scored whether phenology demonstrated a geographic cline (used interchangeably with “natural cline”) in naturally occurring populations and whether this cline was in the expected direction (e.g., a positive slope of spring reproductive phenology with elevation or latitude). Of the 294 cases for which we could evaluate phenological differences across a natural gradient, the vast majority reported significant clines in phenology (84%). We note that publication bias could play into this finding, as studies that do not detect a geographic cline might not be published. Of these, 23% were in an unexpected direction (e.g., earlier emergence in poleward or higher elevation populations; Figure 3: H1a). We also examined patterns in plant vs. animal taxa separately. Trends for variation in phenology across geographic gradients were similar (Supplementary Appendix 4 Figures A2a, A3a), with 60% of plant and 62% of animal studies detecting expected geographic clines in phenology. However, the number of plant cases (n = 228) far exceeded animal cases (n = 66), and few animal studies address the remaining hypotheses (Supplementary Appendix 4 Figures A2, A3). We additionally examined whether annual vs. perennial plants differed in their responses; patterns were qualitatively similar, although the vast majority of studies were conducted using perennials (n = 185 perennials vs. 35 annuals) (Supplementary Appendix 4 Figure A4).
Figure 3. Percent of cases supporting each of the hypotheses outlined for (left to right) all phenophases combined, growth and development, emergence, reproduction, and senescence/autumn phenology. Values represent the number of cases for or against each hypothesis out of the total number of cases that addressed that hypothesis. (H1a) We examine whether the leading edge (i.e., higher latitude or elevation) populations demonstrate delayed spring phenology (growth and development, emergence, and reproduction) and earlier autumn phenology relative to trailing edge (i.e., lower latitude or elevation) populations. “Expected” slopes indicate that phenology follows this pattern; “unexpected” indicates the reverse; “none” indicates no pattern across a geographic gradient. (H1b) We examine whether phenology demonstrates adaptive phenotypic plasticity (i.e., phenological plasticity is in the same direction as the geographic cline and so shifts phenotypes in the direction that would be adaptive in the novel environment), maladaptive plasticity (i.e., phenological plasticity is not consistent with the geographic cline), or mixed evidence. (H1c) We examine whether phenology demonstrates co-gradient genetic clines (i.e., many populations grown in a common environment demonstrate phenological plasticity consistent with the geographic and phenotypic cline), a counter-gradient genetic cline (“counter”), or mixed evidence. For (H2), we examine whether (H2a) edge versus central and (H2b) leading vs. trailing edge populations (or neither) demonstrate greater phenological plasticity, respectively. (H3) is not shown on this figure due to low sample size. For (H4), we examine whether (H4a) edge vs. central and (H4b) leading vs. trailing edge populations (or neither) demonstrate lower genetic variation in phenological traits, respectively.
We next examined whether plasticity in phenological traits was consistent with the direction of the natural cline in phenology (H1b). For example, if reproductive phenology is delayed in higher latitude populations experiencing colder climates, then we would expect single populations to also exhibit delayed reproduction when grown in higher latitudes or colder temperatures. Assuming that locally expressed phenology is adaptive in that environment, such patterns would indicate adaptive plasticity (Ensing and Eckert, 2019). We evaluated this hypothesis by comparing the slope of phenological plasticity across the natural cline to the slope across an environmental gradient. We most often used observational patterns of the natural cline because this data was more frequently available; however, when possible we assessed whether plasticity was adaptive by comparing patterns of population differentiation under common environments. We identified 107 cases that quantified phenological plasticity for one or more populations, either in transplant and/or climate manipulation experiments. Of these, the majority (78%) documented plasticity in the same direction as natural geographic clines (this pattern was again similar in plant and animal taxa; Supplementary Appendix 2 Figures A2, A3), suggesting that phenological plasticity may often be in a direction that facilitates the expression of local phenologies under novel environmental conditions (Figure 3: H1b). However, 16% of cases documented phenological plasticity in the opposite direction of geographic clines, consistent with maladaptive phenology that could contribute to range contractions, and an additional 6% found mixed patterns with some but not all populations exhibiting maladaptive plasticity.
Finally, we looked for evidence of co- vs. counter-gradient patterns of genetic variation in phenology in studies that compared two or more populations in a common environment (H1c; note that the studies used to test H1c are a subsample of those used to test H1b). If phenological plasticity is adaptive, we would predict either no genetic differentiation (i.e., perfect plasticity) or co-gradient genetic variation in which evolution has favored phenological traits in the same direction as environmental effects across species’ ranges. Alternatively, counter-gradient genetic variation could evolve in response to growing season constraints (e.g., later spring, earlier fall, colder temperatures). In this case, leading edge populations, which we predicted would demonstrate delayed emergence, growth, and reproduction and earlier senescence in nature, would instead express more rapid emergence, growth, and reproduction and delayed senescence when grown under common environmental conditions. Of the 56 cases that tested for genetically based differences in phenology, the majority (66%) were consistent with co-gradient genetic variation, whereas 27% were consistent with counter-gradient genetic variation and 7% found mixed results with more variable patterns of genetic differentiation (Figure 3: H1c). Interestingly, most instances of co-gradient variation were detected for phenological traits related to reproduction (72%, or 13/18 cases) whereas counter-gradient variation was equally as likely as co-gradient variation for phenological traits related to growth and development (54%, or 14/26 cases). Although sample sizes for particular phenophases are limited, this is broadly consistent with prior evidence for counter-gradient genetic variation in growth and development (Angilletta, 2009; Conover et al., 2009).
Across geographic gradients, environmental conditions at range margins can prime edge populations to either persist in place or shift the species’ range poleward (Lenoir et al., 2008). Edge populations, particularly at the leading edge, are often assumed to experience harsher and more temporally heterogeneous environments, which could affect plastic responses and the selective pressures leading to local adaptation (Chevin and Lande, 2011) (although we note that edge populations do not necessarily occur in harsher or less stable habitats (Granado-Yela et al., 2013), and climate may impose novel stresses on rear edge habitats (Hampe and Petit, 2005). Additionally, rates of warming relative to historical climate have been more pronounced in high relative to low elevation/latitude environments over the past 50 years (Root et al., 2003; McGuire et al., 2012). For these reasons, we might expect that populations along geographic gradients show differences in the magnitude of phenological plasticity that reflect variation in climatic conditions (Valladares et al., 2014). Specifically, we examined the hypotheses that edge populations would show greater phenological plasticity than central populations (Figure 2: H2a), and similarly that leading edge populations would be more plastic than trailing edge populations (Figure 2: H2b). Alternatively, leading edge populations could be less plastic than trailing edge or central populations if extreme environmental conditions impose stronger selection on phenological and other traits, potentially leading to genetic canalization (Angert et al., 2011; Sheth and Angert, 2014), or if edge habitat is more suitable.
We identified 114 cases that compared phenological plasticity in central vs. edge populations and 113 that compared leading vs. trailing edge populations. We used the authors’ designations for central, leading, or trailing populations. Contrary to our hypotheses, most studies failed to find consistent and statistically significant differences in the magnitude of phenological plasticity across species ranges. Central and edge populations often did not differ in their phenological plasticity (53%, or 60/114 cases), and this pattern was consistent across different phenophases (Figure 3: H2a). (Although in animals, 73% of cases (11/15) detected greater plasticity in edge populations, while evidence among plants was more mixed; Supplementary Appendix 4 Figures A2b, A3b). However, when differences were detected, edge populations were more plastic than central populations in 41% of cases (47/114) whereas central populations were most plastic in only 6% of cases. Additionally, leading and trailing edges often did not differ in phenological plasticity (39%, or 44/113 cases). Leading edges were more plastic than trailing edges in only 27% of cases (20/113) and trailing edges were actually more plastic than leading edges in 35% of cases (39/113 cases) (Figure 3: H2b). Perennial plants in particular were more likely to demonstrate greater plasticity in their trailing edge populations, especially for growth and development (57% of cases, or 27/47; Supplementary Appendix 4 Figure A4). This pattern differed between plant and animal taxa: trailing edges were slightly more likely to demonstrate greater plasticity than leading edges in plants (38%, or 37/98 cases; although plants were just as likely to demonstrate no differences in plasticity between leading and trailing edges), while leading edges more often demonstrated higher plasticity than trailing edges in animals (60%, or 9/15 cases; Supplementary Appendix 4 Figures A2c, A3c).
Phenological plasticity has most often been discussed in the context of tracking shifting climate conditions in time, as an alternative to range shifts which track shifting climate conditions in space. In this view, phenological plasticity should stabilize historical range boundaries, whereas local adaptation of phenology should facilitate poleward range shifts (Colautti and Barrett, 2013). In other words, maintaining a historically adaptive phenology may best facilitate expansion of leading edge genotypes beyond the historical range edge as long as conditions there track those in the historical range edge (Figure 2: H3) (Hargreaves and Eckert, 2019). However, the potential for phenological plasticity to facilitate range shifts has received less consideration, but may be important if migration rates do not perfectly track climate change or conditions beyond the range favor new phenology.
Testing whether expanding populations can persist beyond their current range edge, and the importance of phenological plasticity or local adaptation in this process, requires transplant experiments testing performance beyond the current range limit (Hargreaves et al., 2014). However, we found only four studies (spanning three species) that examined (only reproductive) phenology in transplants beyond the range edge (Eckhart et al., 2004; Wadgymar et al., 2015; Benning et al., 2019; Hargreaves and Eckert, 2019). Two of these showed that leading-edge genotypes expressed adaptive phenology, and had greater fitness, than central or trailing-edge genotypes when transplanted beyond the range (Wadgymar et al., 2015; Hargreaves and Eckert, 2019). However, only one study found that adaptive phenological plasticity benefitted fitness beyond the range edge (Wadgymar et al., 2015). In contrast, studies with Clarkia xantiana suggest that maladaptive phenology limits performance in beyond-the-range transplants (Eckhart et al., 2004; Benning et al., 2019). Interestingly, none of these studies detected greater phenological plasticity in edge populations relative to central populations.
In addition to plasticity in phenology, populations may also adapt to novel climatic conditions through evolutionary changes in phenology. The magnitude of genetic variation, the material for evolutionary change, in phenology will limit species’ ability to adapt to novel environmental conditions either in situ or during range shifts. Populations may harbor different levels of genetic variation in phenological traits (Pironon et al., 2017), and theory predicts that edge populations might exhibit lower genetic variation than central populations for three reasons. First, edge populations are often more isolated than central populations (Hengeveld and Haeck, 1982; Brown, 1984; Leonardi et al., 2012). Second, edge populations are often less abundant, and smaller population sizes can result in decreases in vital rates toward the range edge (Sexton et al., 2009). Third, edge populations presumably persist in less optimal environmental conditions than central populations, assuming that a species’ range represents its ecological niche. These three conditions - isolation, smaller populations sizes, and marginality - are predicted to decrease genetic diversity within a population (Lawton, 1993). We therefore predicted that edge populations harbor less genetic variation than central populations (H4a), potentially limiting range expansions under climate change (Figure 2: H4).
Seven studies in our dataset reported genetic variation in 18 total phenological traits for two or more populations spanning a geographic gradient (and almost all studies used perennial plants; Supplementary Appendix 4 Figure A4) (De Kort et al., 2016; Evans et al., 2016; Sheth and Angert, 2016; Firmat et al., 2017; Lustenhouwer et al., 2018; Bemmels and Anderson, 2019; Wenden et al., 2020). Of those, edge populations demonstrated lower genetic variation than central populations in 67% of cases (12/18), and the remaining cases failed to find consistent or statistically significant differences (Figure 3: H4a). Interestingly, no cases reported lower genetic variation in central populations.
Patterns of genetic variation could also differ between leading and trailing edge populations, with greater climate variability and/or gene flow from the center of the range perhaps maintaining greater genetic variation in phenology at leading range edges compared to trailing range edges (Davis and Shaw, 2001; H4b). Alternatively, trailing edge populations often have longer and more stable histories (i.e., persistence during interglacial periods) than leading edge populations and often respond to different environmental factors and so may exhibit unique genetic variation that could benefit species’ persistence and range shifts under future climates (Hampe and Petit, 2005; Rehm et al., 2015). Of the 18 cases addressing genetic variation in leading vs. trailing edge populations, genetic variation in phenological traits was lowest in leading edge populations in 50% of cases (9/18) and in trailing edge populations in 22% of cases (4/18). Thus, we find some evidence for lower adaptive potential in phenology in edge populations, and particularly leading edge populations, which would further suggest the importance of plasticity in mediating phenological shifts. However, we caution that this is based on a small number of studies and in roughly one third of cases populations did not show clear patterns in adaptive potential across geographic gradients.
Taken together, we found surprisingly mixed evidence for hypothesized patterns in phenological plasticity across species’ ranges. Phenology often demonstrated a geographic cline in the studies reviewed here, though in a quarter of cases it was in an unexpected direction (Figure 3: H1a). In these cases, leading edge populations demonstrated earlier emergence, growth and development, reproduction, and/or later senescence than trailing edge populations. We might find these patterns in cases where other phenological cues (e.g., photoperiod; see below) are important or if species’ ranges are fragmented (such that central populations are isolated). Most studies detected adaptive plasticity, indicating that phenological plasticity may often be in a direction that facilitates the expression of adaptive phenologies under novel environmental conditions. Given that the direction of phenological plasticity will determine the initial success of a population experiencing novel environmental conditions (Ghalambor et al., 2007), such adaptive plasticity will likely facilitate population persistence in situ as well as enable edge populations to expand into novel environmental conditions beyond the range edge. However, despite this general trend, we note that some cases found either direct evidence of maladaptive plasticity (16%) and/or counter-gradient genetic variation (37%), suggesting that environmental effects on phenology may actually decrease fitness and contribute to range contractions in a minority of species. Similarly, in a review of population vs. genetic differentiation, Stamp and Hadfield (2020) found that 20% of traits exhibit maladaptive plasticity. This pattern of mostly adaptive, but some maladaptive, plasticity in phenology under climate change is broadly consistent with observations that leading-edge range expansions are more common than trailing-edge range contractions in terrestrial species (Sunday et al., 2012).
Despite strong support for adaptive clines in phenology, we did not detect clear trends in either the magnitude of phenological plasticity or adaptive potential in phenology between edge vs. central populations. The only conclusion we are able to make based on our literature review is that central populations do not generally have greater phenological plasticity or lower adaptive potential than edge populations. Otherwise, we found very mixed support for either greater phenological plasticity and lower adaptive potential at range edges, or no consistent geographic trends in the magnitude of plasticity or adaptive potential. If plasticity and adaptive potential do not generally show predictable geographic clines, then that would suggest that the spatial pattern of persistence and range shifts may be more idiosyncratic, depending on the dynamics of local populations.
Additionally, we actually found more studies detecting greater phenological plasticity and adaptive potential in trailing edge populations than in leading edge populations (although this pattern was only true for plants). Although this is in contrast to our hypotheses, there are several possible reasons for these patterns. First, leading edge populations often experience a narrow set of harsh environmental conditions; cold, short growing seasons at high latitudes or elevations can select for canalized phenology under strict time constraints (Gugger et al., 2015). As poleward populations already have a compressed growing season, they may lack the plasticity to further advance their phenology (Clark et al., 2014). In this case, leading edge range limits might be set by an inability to complete reproduction (Morin et al., 2007; Chuine, 2010). Further, greater plasticity at trailing edges could allow populations to adjust their phenology and persist in place as local environments shift (e.g., glacial refugia during the Quaternary; Petit et al., 2003), potentially preventing range contractions at the trailing edge (Figure 2: H3). Finally, trailing edge populations may maintain greater genetic variation due to their more stable demographic histories, high levels of genetic differentiation, and local adaptation between populations (Petit et al., 2003; Hampe and Petit, 2005), which could potentially provide a source of species-level genetic diversity that would promote persistence under novel conditions. If trailing edge (or central) populations have more genetic variation than leading edge populations, as we found some support for in our review, then gene flow from these populations could provide crucial genetic material for range expansions (see Hampe and Petit, 2005 for a review of the importance of rear edge populations).
Ultimately, range shifts will also require population persistence beyond the range edge. As described, we only found four studies that assessed phenology in transplants beyond the range edge. Of these, two included a climate manipulation designed to test how range edge populations respond to climate change (Wadgymar et al., 2015; Hargreaves and Eckert, 2019). Overall, results for fitness beyond the range edge were mixed. Evidence in Chamaecrista fasciculata suggests that local adaptation to contemporary conditions in leading edge populations combined with gene flow from trailing edge populations of alleles that are adaptive under warming could facilitate range expansions (Wadgymar et al., 2015). However, although northern populations of Rhinanthus minor also performed best beyond the range edge, this was due to increased fecundity under warming rather than earlier flowering (Hargreaves and Eckert, 2019). In contrast to these two studies, two subspecies of Clarkia xantiana demonstrated later phenology at and beyond their range edges, resulting in low fitness beyond the range edge (Eckhart et al., 2004; Benning et al., 2019). However, C. xantiana did flower earlier in dry seasons, suggesting that phenological plasticity over time (rather than across space) in response to variable environmental conditions could enhance performance. Altogether, the limited number of studies and variability in these results highlights a need for reciprocal transplant experiments that test for local adaptation or plasticity in phenological traits and their effects on fitness and population persistence, which will ultimately determine successful colonization beyond the range edge.
Climate-mediated range shifts will require not only initial establishment beyond the range edge, but consideration of other factors influencing long-term persistence in novel environments. Below, we outline several additional ways in which phenological plasticity may mediate ecological and evolutionary dynamics that govern population persistence beyond the range edge.
Variation in phenological responses to climate is in part due to variation in the environmental cues that regulate phenological plasticity. For example, plasticity may be driven by temperature, photoperiod, winter chilling, growing season length, or soil conditions, and the seasonal windows in which these cues influence phenology may also differ across species’ ranges and with novel climate change (Frei et al., 2014; Körner et al., 2016; Cooper et al., 2019; Wenden et al., 2020). Here we outline potential constraints on the role of phenological plasticity in facilitating range shifts. Understanding how multiple environmental variables interact to drive phenological plasticity, instead of just temperature, across geographic gradients will be necessary to predict range shifts and develop effective adaptation strategies (e.g., assisted migration).
Phenology may be responsive to photoperiod rather than (or in addition to) temperature. As climate warming pushes phenology to new seasonal limits both at the early and late end of the growing season, photoperiod might constrain warming-induced phenological shifts (Richardson et al., 2018). For example, shorter photoperiods during early phenophases such as bud break can dampen phenological advances (Körner and Basler, 2010; Meng et al., 2021) and slow development (Fu et al., 2019). This could prevent plants from emerging too early and risking exposure to harsh conditions like frost (Flynn and Wolkovich, 2018). Additionally, Picea from high elevations exhibit greater sensitivity to photoperiod (Körner et al., 2016), suggesting potential variation in photoperiod responses across species’ ranges. Since phenological responses to photoperiod and its interaction with temperature are not as well-studied as temperature alone (Meng et al., 2021), future studies may need to examine how photoperiod might limit phenological advances and potential shifts in species’ distributions.
In addition to photoperiod, winter chilling and temperatures, growth season length, and exposure to harsh soil conditions can limit phenological responses. Similarly to photoperiod constraints, winter chilling requirements are also hypothesized to limit early development (Vitasse et al., 2014). As temperatures rise, chilling requirements can be either only partially met or met later in time (Fu et al., 2015). For these reasons, species that are not constrained by chilling requirements, such as pedunculate oak (Quercus robur), often demonstrate greater phenological sensitivity to temperature than species that are limited by chilling, such as European beech (Fagus sylvatica) (Wenden et al., 2020). Similarly, phenology can respond to winter warming as much as spring warming, but its effects are understudied. Species in the United States and United Kingdom, for instance, delay flowering in warmer winters but advance flowering in warmer springs (Cook et al., 2012), and early-flowering native prairie forbs advance flowering in response to warming winters as much as warming springs (Zettlemoyer et al., 2021). At leading range edges, growing season length constraints may also limit the degree to which phenology can shift (e.g., risk of frost at either end of the growing season; de Valpine and Harte, 2001; Inouye, 2008; Munguía-Rosas et al., 2011; Pardee et al., 2019). Finally, changes in soil moisture (and other soil factors like depth or fertility; Blume et al., 2016) can also affect phenology, though evidence for the direction of this effect is mixed as drought can either delay (Adams et al., 2015) or advance phenology (Giménez-Benavides et al., 2006; Gugger et al., 2015). Moreover, interactions between warming and drought are particularly likely to matter in water-limited regions, such as might occur at trailing range edges in low-elevation (Giménez-Benavides et al., 2006) or Mediterranean environments (Hänel and Tielbörger, 2015). Altogether, because abiotic factors other than spring temperature can influence phenology, range shifts are likely to be a balancing act between phenological plasticity in response to temperature and costs or limitations associated with responses to other facets of the environment.
The timing of development and reproduction is an important aspect of life history, and advancing phenology in response to climate change may lead to correlated shifts in other life history or resource-use traits that could constrain lifetime fitness or even limit evolutionary responses (Etterson and Shaw, 2001). For example, earlier reproduction is often correlated with smaller size at reproduction (Bale et al., 2002; Franks and Weis, 2008; Colautti et al., 2010) and a switch from more resource conservative to more resource acquisitive traits (e.g., greater specific leaf area, higher nitrogen content; Wright et al., 2004; Sheth and Angert, 2016). Indeed, there is increasing evidence that advancing phenology under climate change is leading to more compressed life-cycles and faster life-history strategies (Dingle et al., 1990; Berner and Blanckenhorn, 2006; Välimäki et al., 2013; Zettlemoyer et al., 2017; Peterson et al., 2020). However, the consequences of these multivariate trait shifts for absolute fitness appear to be mixed. Although earlier flowering is often correlated with higher reproductive success in plants (Munguía-Rosas et al., 2011; Lustenhouwer et al., 2018), other studies have found reduced reproductive success due to advancing or compressed life cycles under climate change (Burgess et al., 2007; Colautti et al., 2010; Haggerty and Galloway, 2011; Rafferty et al., 2016). Such changes in correlated traits could also have important implications for the longer-term persistence and evolutionary potential of populations (Cotto et al., 2019). On the one hand, faster life histories and reduced generation times should facilitate rapid adaptation to environmental change. On the other hand, correlated shifts across multiple traits may increase the potential for antagonistic genetic correlations to constrain evolutionary responses (Etterson and Shaw, 2001; Chevin, 2013).
Although here we have focused on the timing of reproduction, due to its emphasis in the literature, it is important to note that shifts in early phenological events such as emergence can also modify the timing of subsequent phenophases (Donohue, 2002). Different phenophases can vary in their plasticity or environmental cues (Mediavilla and Escudero, 2009; Buckley et al., 2015; Wadgymar et al., 2015), but phenological shifts are rarely studied in the context of the entire life cycle of an organism and early phenophases such as germination or emergence are particularly understudied (Figure 1). Future studies should focus on shifts in early phenophases as well as correlated phenological traits across ontogeny. Ultimately, understanding the traits that potentially trade-off with flowering time (both other phenophases and correlated traits) will be crucial to predicting population responses and range shifts under climate change.
Not only can climate limit species’ distributions and range shifts, but biotic interactions are also likely to shape geographic range edges. In particular, competition, predation, and hybridization could constrain range expansions, while mutualisms could extend or limit range expansion (Louthan et al., 2015). Below we highlight a few examples of potential mismatched species interactions that could arise due to altered phenology across geographic gradients (see Louthan et al. (2015) for a review on the role of species interactions in determining species’ geographic range limits and Visser and Gienapp. (2019) for a review of phenological mismatches).
First, altered phenology could affect species’ likelihood of experiencing predation and herbivory beyond the range edge. For example, in Clarkia xantiana ssp. xantiana, delayed phenology beyond the range edge dramatically increases the probability of fatal herbivory, maintaining this range limit (Benning et al., 2019). This interaction is further complicated by climate, as phenology is further delayed in wet years, resulting in 25% higher herbivory beyond the range edge in wet relative to drier years (Benning et al., 2019). In contrast, phenological escape from herbivores and granivores has been shown to facilitate range shifts in several plant taxa, particularly for non-native species invading new ranges (Mlynarek et al., 2017). For insect populations expanding poleward, such escape of potential food sources would likely lead to extirpation (Cahill et al., 2013).
Perhaps more widely considered, climate-driven phenological mismatches between host plants and pollinators are likely to affect persistence beyond range edges. While demographic costs of such mismatches have been considered (Visser and Gienapp., 2019), few studies examine spatial variation in synchrony between species in the context of climate change and range shifts. However, phenological synchrony between species can vary across geographic gradients. For instance, in southern areas, the butterfly Anthocharis cardamines is well-synchronized with its local hosts because they demonstrate similar advancements in spring phenology in response to warming. In the north, on the other hand, relative phenology between the butterfly and host plants is shifting with warming, mostly due to more limited phenological plasticity in A. cardamines (aligning with the prediction that climate change will lead to weaker phenological advancements in consumers relative to resources; Renner and Zohner, 2018). This scenario again points to the potential importance of trailing edge populations as a source of potentially adaptive phenology during poleward expansion (Posledovich et al., 2018). Not only can climate change affect the synchrony of pollinators, but pollination efficacy can also vary with warming (Rafferty and Ives, 2012). Analyses of pollination synchrony and success over geographic gradients will be necessary to determine whether populations that shift poleward as a result of phenological plasticity can persist in the context of novel environments and commensurate shifts in species interactions.
Phenological mismatches constraining range shifts could also occur within a given trophic level. Phenological shifts will vary from year-to-year and across space depending on local environmental conditions, and the relative importance of the different variables that might affect phenology (e.g., temperature, photoperiod, precipitation) varies among species. For instance, flowering phenology shifts at different rates across elevations in the southwestern United States, advancing at lower elevations but not necessarily at higher elevations (Rafferty et al., 2020). These divergent responses can disrupt species interactions across trophic levels, as described above, but can also reshuffle communities (CaraDonna et al., 2014) and disrupt important interactions within a species such as gene flow between subpopulations or access to mates (see below). More specifically, synchronous flowering with other community members can increase pollinator visitation (Burkle et al., 2013). However, we have a limited understanding of the biological consequences of interspecific phenological synchrony (Zohner et al., 2018), and no studies to our knowledge have investigated interspecific phenological synchrony across species’ ranges or beyond the edge.
Although many traits may be important in mediating species’ climate tolerances, shifts in reproductive phenology are unique in their potential to directly disrupt patterns of gene flow across a species’ range (Levin, 2009; Franks, 2015). Differences in flowering time have been shown to generate assortative mating within populations (Weis and Kossler, 2004) as well as mediate gene flow between populations (Antonovics, 2006). Thus, climate-mediated shifts in phenology could have profound impacts on patterns of genetic variation within and among populations, ultimately affecting the adaptive potential of populations in response to climate change.
Within populations, several studies have found more variable and less synchronized reproductive phenology in response to climate change, perhaps reflecting differences among individuals in phenological plasticity (CaraDonna et al., 2014; Hall et al., 2018; Zohner et al., 2018). Such reductions in reproductive synchrony within populations are likely to decrease opportunities for outcrossing, limit within-population genetic variation, and decrease reproductive success (Augspurger, 1981; Giménez-Benavides et al., 2006). For example, Hall et al. (2018) found that the fitness benefits of earlier flowering under advancing snowmelt were at least partially counteracted by costs associated with decreased reproductive synchrony. However, most studies of phenological shifts have emphasized population-level averages and simple metrics (i.e., day of first flower) rather than detailed individual-level data on phenological distributions needed to quantify shifts in synchrony under climate change.
Among populations, phenology can be a critical driver of patterns of gene flow. For example, gradients in the timing of snowmelt, and thus flowering phenology, have been shown to be significant predictors of genetic isolation in several alpine plants (Hirao and Kudo, 2004; Yamagishi et al., 2005). In the context of climate change, phenological plasticity may disrupt these patterns of historical gene flow. For example, advancing flowering phenology actually led to greater separation of peak flowering, and reduced potential for gene flow among populations along elevational gradients in Trillium erectum (Rivest et al., 2021). Alternatively, adaptive phenological plasticity could facilitate the spread of warm-adapted alleles by enabling trailing-edge genotypes to successfully migrate and interbreed with populations throughout the species’ range, contributing to greater genetic variation and adaptive potential (Ensing and Eckert, 2019). On the other hand, expanding leading-edge populations could potentially be limited by “swamping” gene flow from central populations (Kirkpatrick and Barton, 1997) or reduced genetic diversity following colonization (e.g., founder events; Sjölund et al., 2019) that constrain the ability of range-edge populations to adapt to novel environmental conditions. For example, there is some evidence that adaptive genetic differentiation is weaker along steeper environmental elevational gradients vs. more gradual latitudinal gradients due to gene flow (Bachmann et al., 2020). The extent to which phenological plasticity will shape gene flow, and thus adaptation and range shifts under climate change, is still very much an open question.
We have reviewed and outlined different routes by which phenological plasticity could facilitate or hinder range shifts. However, no studies have demonstrated that phenological plasticity facilitates range shifts and persistence beyond contemporary range edges, despite the common assumption that phenological plasticity facilitates population growth and spread (McLean et al., 2016). Recognizing how phenological plasticity could facilitate range shifts, and particularly expansions at the leading edge, is an important first step to designing empirical studies to test these processes. We envision several approaches for testing the role of phenological plasticity in range shifts: (1) filling in gaps in our knowledge; (2) incorporation and evaluation of phenology in improving models of species distributions, (3) examining phenological responses to experimental manipulations of climate change across geographic gradients including (i) genetic variation in phenological traits and (ii) phenological synchrony across and within trophic levels; (4) beyond the edge transplants in nature; and (5) greater consideration of the role of trailing populations in range shifts. We address each of these points below.
We identified several gaps in our current knowledge. First, relatively few studies address the role of phenophases beyond growth/development and reproduction. Emergence and senescence could also influence range shifts, but combined they only accounted for 14% of the studies reviewed here. Given that each of these phenophases bracket species’ life cycles and/or growing seasons, and therefore may impose the outer limits on how much phenology can shift, understanding their role will be critical to predicting overall phenological plasticity and fitness during range shifts. Emergence and senescence, as well as measurements of the start, peak, and end of the growing season, could benefit from increased use of remote sensing data across species’ geographic ranges (see Piao et al., 2019 for a review on the use of remote sensing data for phenological observations). Second, while Europe and North America accounted for 282 cases, only five studies came from Asia [three from Japan (Ishizuka et al., 2015; Sakurai and Takahasi, 2016; Jeong et al., 2020), one from China (Tang et al., 2017), one from India (Datta et al., 2017)], one from Mali (LaBarbera and Lacey, 2018), and one from Chile (Bull-Hereñu and Arroyo, 2009). Although this is likely partially because many studies address poleward shifts toward colder climates, biasing results toward the northern hemisphere, tropical alpine species face similar climate threats but their responses to climate change are relatively understudied (Telwala et al., 2013).
Models offer a potentially powerful approach to test the role of phenology in driving or constraining species’ range shifts. Several studies have used species’ phenological traits to develop models of their geographic distribution, suggesting an important role for phenology in determining range limits (e.g., Chuine and Beaubien, 2001; Morin et al., 2008; Chapman et al., 2014; Duputié et al., 2015), and some of these have included variation in phenology across populations or geographic gradients (Chapman et al., 2017; Gauzere et al., 2020). Such models provide a fruitful avenue to explore the potential consequences of variation in phenological plasticity and/or genetic variation in phenology across geographic gradients. In particular, studies that compare the predictive accuracy of models based on alternative assumptions about the role of plasticity would be insightful. However, ultimately we need empirical data to parameterize and test these models, and we envision three empirical approaches for testing the potential role of phenological plasticity in range shifts.
To test whether leading or trailing edges have greater phenological plasticity and whether that plasticity can facilitate both expansion and persistence beyond the range edge, we need (1) experiments combining phenological measurements across geographic gradients with manipulations of climate change as well as (2) transplants beyond contemporary range edges. Across a geographic gradient, phenological plasticity can enable persistence in situ, expansion to similar climatic conditions within the historical range, or expansion to newly suitable locations beyond the historical range. For these scenarios, we need to understand how trailing, central, and leading edge populations vary in phenological plasticity in response to environmental conditions that vary with geography. Additionally, genetic variation among populations from across these gradients will influence how well populations can adapt or respond plastically to different environments, but very few studies examine adaptive potential in phenology across geographic gradients. Similarly, few studies examine phenological synchrony across geographic gradients, but interactions across trophic levels (e.g., with pollinators and food sources), within trophic levels (e.g., competition or facilitation among co-flowering species), or within the same species (e.g., access to mates) will affect the fitness consequences of phenological shifts and thus whether populations can persist.
Climate warming will generally result in a shift of the favorable conditions for growth and reproduction poleward and upward in elevation. Studies that transplant individuals into new parts of the historical range that also impose a climate manipulation mimicking future environmental conditions offer critical insights into how populations throughout the range may respond and migrate during forecasted climate change. Taking this a step farther, transplants beyond current range edges represent the most powerful experimental approach for examining whether populations can actually persist in a new location. Beyond-the-edge transplants, which only represented four studies reviewed here, allow us to examine whether edge populations are primed for expansion or if the negative effects of small population size, genetic drift, or inbreeding might thwart colonization beyond the edge. They could also differentiate between two potential mechanisms of range expansion: phenological plasticity vs. local adaptation in phenology (Figure 2: H3). Yet even beyond-the-range transplants have often omitted several crucial parts to understanding range shifts: early life-history stages, multiple generations and multiple populations from various locations from within the species’ contemporary range (Hargreaves and Eckert, 2019). The latter in particular may become increasingly important if trailing edge populations are better-suited to the warmer temperatures expected under future conditions (Grady et al., 2011), indicating their potential in range shifts and species conservation.
Indeed, our review points to the possibility for trailing edge populations to demonstrate greater phenological plasticity and genetic variation in phenological traits than leading edge populations. If this pattern is generally true and individuals from trailing edge populations demonstrate sufficient phenological plasticity or adaptive potential, they could play an important role in stabilizing species’ trailing edges and/or dispersing potentially adaptive alleles poleward that could enable adaptation to novel habitats and climates (Gibson and Reed, 2008; Wadgymar et al., 2015). However, we know relatively little about how trailing edge populations might contribute to range shifts, suggesting that individuals from trailing edges should be included in studies examining species’ responses to climate change such as transplants along geographic and environmental gradients where we can estimate genetic variation under simulated future climates. For example, genetic variation in both germination and flowering phenology was highest in populations of Boechera stricta transplanted upslope and decreased in populations transplanted downslope, suggesting that range shifts may be facilitated by increased genetic variation (Bemmels and Anderson, 2019). Similarly, in Chamaecrista fasciculata, colonists from the trailing edge benefitted leading edge populations by introducing adaptive alleles (Wadgymar et al., 2015). These scenarios point to the possibility that trailing edge genotypes could be used to help mitigate extinction risk through assisted range expansion (Loss et al., 2011), although the benefits and goals of this approach are debated (Vitt et al., 2010; Hällfors et al., 2017). Overall, trailing edge populations may have enough genetic variation and/or plasticity to increase the chance that individuals can expand and persist beyond their current range limits, but trailing edge populations remain understudied and the performance and demography of trailing edge populations under novel environmental conditions remains an open area for research.
As climate change progresses, phenological plasticity is likely to play an important role in mediating population persistence and species’ range shifts. Our review of the literature suggests that phenotypic plasticity will often be in an adaptive direction that allows populations to match local phenotypes, and that trailing and central populations may be critical sources for phenological plasticity and genetic variation in phenology relative to leading edge populations. However, it remains difficult to make generalizations about the role of phenological plasticity in range shifts due to lack of empirical data. Importantly, we identify several gaps in our current understanding including relatively few studies that investigate phenophases related to emergence or senescence, and few studies that compare either patterns of adaptive potential in phenology across geographic gradients or the role of phenology in mediating range shifts. In particular, we highlight beyond-the-range transplant experiments that quantify phenological plasticity for multiple populations as being particularly fruitful. Shifting phenology may also influence longer-term ecological and evolutionary dynamics of populations during range shifts, such as correlated life history shifts, mismatched species interactions, and patterns of gene flow, that remain relatively understudied. Given that shifts in phenology have been one of the most consistent and dramatic biological responses to climate change, we urge future research to carefully dissect its causes and consequences for species range shifts.
MZ and MP conceived the manuscript and conducted the literature review. MZ wrote the first draft of the manuscript and both authors contributed to revisions. Both authors contributed to the article and approved the submitted version.
The authors declare that the research was conducted in the absence of any commercial or financial relationships that could be construed as a potential conflict of interest.
The Supplementary Material for this article can be found online at: https://www.frontiersin.org/articles/10.3389/fevo.2021.689192/full#supplementary-material
Adaptive plasticity: Environmental responses that shift a phenotype in the same direction that would be favored (i.e., have higher fitness) in that environment; or, in the absence of fitness data, inferred as the direction that would match the locally observed phenotype in that environment.
Central population: Populations located within the core of a species’ current geographic distribution.
Co-gradient genetic variation: Genetically based differences in the phenotype expressed by populations in a common environment that mimic the natural (geographic) cline.
Counter-gradient genetic variation: Genetically based differences in the phenotype expressed by populations in a common environment that oppose the natural (geographic) cline.
Geographic cline: Variation in a phenotype expressed in wild populations across a geographic (e.g., latitude or elevation) gradient.
Leading edge population: Populations residing at the current leading range edge; here, more poleward or higher elevation populations.
Leading range edge: The margin of a species’ distribution that is predicted to become more climatically suitable under climate change, potentially facilitating expansion beyond the historical range edge.
Maladaptive plasticity: Environmental responses that shift a phenotype away from the optimal phenotype in that environment; or, in the absence of fitness data, inferred as the direction away from the locally observed phenotype in that environment.
Phenological plasticity: A non-genetic shift in the timing of life-history events (here, “phenophases”) in response to change(s) in the (a)biotic environment.
Trailing edge population: Populations residing at the current trailing range edge; here, more equatorial or lower elevation populations.
Trailing range edge: The margin of a species’ distribution that is predicted to become less climatically suitable under climate change, potentially leading to local extirpation and contraction of the historical range edge.
Range shift: Change in the geographic distribution of a species due to range contractions and/or expansions beyond historical range edges.
Reaction norm: The relationship between an environmental variable and the phenotype expressed by a given genotype or population; the pattern of phenotypic plasticity.
Adams, H. D., Collins, A. D., Briggs, S. P., Vennetier, M., Dickman, L. T., Sevanto, S. A., et al. (2015). Experimental drought and heat can delay phenological development and reduce foliar and shoot growth in semiarid trees. Glob. Chang. Biol. 21, 4210–4220. doi: 10.1111/gcb.13030
Angert, A. L., Sheth, S. N., and Paul, J. R. (2011). Incorporating population-level variation in thermal performance into predictions of geographic range shifts. Integr. Comp. Biol. 51, 733–750. doi: 10.1093/icb/icr048
Angilletta, M. J. (2009). Thermal Adaptation: A Theoretical and Empirical Synthesis. Oxford: Oxford University Press.
Antonovics, J. (2006). Evolution in closely adjacent plant populations. X. Long-term persistence of prereproductive isolation at a mine boundary. Heredity 97, 33–37. doi: 10.1038/sj.hdy.6800835
Augspurger, C. K. (1981). Reproductive synchrony of a tropical shrub: experimental studies of effects of pollinators and seed predators on Hybanthus prunifolius (Violaceae). Biotropica 15, 257–267.
Bachmann, J. C., van Rensburg, A. J., Cortazar-Chinarro, M., Laurila, A., and Van Buskirk, J. (2020). Gene flow limits adaptation along steep environmental gradients. Am. Nat. 195, e67–e86.
Bale, J. S., Masters, G. J., Hodkinson, I. D., Amwack, C., Martijn Bezemer, T., Brown, V. K., et al. (2002). Herbivory in global climate change research: direct effects of rising temperature on insect herbivores. Glob. Chang. Ecol. 8, 1–16. doi: 10.1046/j.1365-2486.2002.00451.x
Bemmels, J. B., and Anderson, J. T. (2019). Climate change shifts natural selection and the adaptive potential of the perennial forb Boechera stricta in the Rocky Mountains. Evolution 73, 2247–2262. doi: 10.1111/evo.13854
Bender, M. H., Baskin, J. M., and Baskin, C. C. (2002). Phenology and common garden and reciprocal transplant studies of Polymnia canadensis (Asteraceae), a monocarpic species of the North American Temperate Deciduous Forest. Plant Ecol. 161, 15–39.
Benning, J. W., Eckhart, V. M., Geber, M. A., and Moeller, D. A. (2019). Biotic interactions contribute to the geographic range limit of an annual plant: herbivory and phenology mediate fitness beyond a range margin. Am. Nat. 193, 786–797. doi: 10.1086/703187
Berner, D., and Blanckenhorn, W. U. (2006). Grasshopper ontogeny in relation to time constraints: adaptive divergence and stasis. J. Anim. Ecol. 75, 130–139. doi: 10.1111/j.1365-2656.2005.01028.x
Blume, H.-P., Brümmer, G. W., Fleige, H., Horn, R., Kandeler, E., Kögel-Knaber, E., et al. (2016). Scheffer/Schachtschabel Soil Science. Springer-Verlag Berlin Heidelberg. doi: 10.1007/978-3-642-30942-7_1
Brown, J. H. (1984). On the relationship between abundance and distribution of species. Am. Nat. 124, 255–279. doi: 10.1086/284267
Buckley, L. B., Nufio, C. R., Kirk, E. M., and Kingsolver, J. G. (2015). Elevational differences in developmental plasticity determine phenological responses of grasshoppers to recent climate warming. Proc. R. Soc. B. 282, 20150441. doi: 10.1098/rspb.2015.0441
Bull-Hereñu, K., and Arroyo, M. T. (2009). Phenological and morphological differentiation in annual Chaetanthera moenchioides (Asteraceae) over an aridity gradient. Plant Syst. Evol. 278, 159–167. doi: 10.1007/s00606-008-0126-8
Burgess, K. S., Etterson, J. R., and Galloway, L. F. (2007). Artificial selection shifts flowering phenology and other correlated traits in an autotetraploid herb. Heredity 99, 641–648. doi: 10.1038/sj.hdy.6801043
Burkle, L. A., Marlin, J. C., and Knight, T. M. (2013). Plant-pollinator interactions over 120 years: loss of species, co-occurrence, and function. Science 339, 1611–1615. doi: 10.1126/science.1232728
Cahill, A. E., Aiello-Lammens, M. E., Fisher-Reid, M. C., Hua, X., Karanewsky, C. J., Ryu, H. Y., et al. (2013). How does climate change cause extinction? Proc. R. Soc. B. 280:20121890.
CaraDonna, P. J., Iler, A. M., and Inouye, D. W. (2014). Shifts in flowering phenology reshape a subalpine plant community. Proc. Natl. Acad. Sci.U.S.A. 111, 4916–4921. doi: 10.1073/pnas.1323073111
Chapman, D. S., Haynes, T., Beal, S., Essl, F., and Bullock, J. M. (2014). Phenology predicts the native and invasive range limits of common ragweed. Glob. Change Biol. 21, 192–202. doi: 10.1111/gcb.12380
Chapman, D. S., Scalone, R., Štefanić, E., and Bullock, J. M. (2017). Mechanistic species distribution modeling reveals a niche shift during invasion. Ecology 98, 1671–1680. doi: 10.1002/ecy.1835
Chevin, L.-M. (2013). Genetic constraints on adaptation to a changing environment. Evolution 67, 708–721. doi: 10.1111/j.1558-5646.2012.01809.x
Chevin, L. −M., and Lande, R. (2011). Adaptation to marginal habitats by evolution of increased phenotypic plasticity. J. Evol. Biol 24, 1462–1476. doi: 10.1111/j.1420-9101.2011.02279.x
Chmura, H. E., Kharouba, H. M., Ashander, J., Ehlman, S. M., Rivest, E. B., and Yang, L. H. (2019). The mechanisms of phenology: the patterns and processes of phenological shifts. Ecol. Monogr. 89:c01337.
Chuine, I. (2010). Why does phenology drive species distribution? Phil. Trans. R. Soc. B. 365, 3149–3160. doi: 10.1098/rstb.2010.0142
Chuine, I., and Beaubien, E. G. (2001). Phenology is a major determinant of tree species’ range. Ecology Letters 4, 500–510. doi: 10.1046/j.1461-0248.2001.00261.x
Clark, J. S., Salk, C., Melillo, J., and Mohan, J. (2014). Tree phenology responses to winter chilling, spring warming, at north and south range limits. Funct. Ecol. 28, 1344–1355. doi: 10.1111/1365-2435.12309
Colautti, R. I., and Barrett, S. C. H. (2013). Rapid adaptation to climate facilitates range expansion of an invasive plant. Science 342, 364–366. doi: 10.1126/science.1242121
Colautti, R. I., Eckert, C. G., and Barrett, S. C. H. (2010). Evolutionary constraints on adaptive evolution in an invasive plant. Proc. R. Soc. B. 277, 1799–1806. doi: 10.1098/rspb.2009.2231
Conover, D. O. (1990). The relationship between capacity for growth and length of growing season - evidence for and implications of countergradient variation. Trans. Am. Fish. Soc. 119, 416–430. doi: 10.1577/1548-8659(1990)119<0416:trbcfg>2.3.co;2
Conover, D. O., Duffy, T. A., and Hice, L. A. (2009). The covariance between genetic and environmental influences across ecological gradients. Ann. N.Y. Acad. Sci. 1168, 100–129. doi: 10.1111/j.1749-6632.2009.04575.x
Conover, D. O., and Schultz, E. T. (1995). Phenotypic similarity and the evolutionary significance of countergradient variation. Trends Ecol. Evol. 10, 248–252. doi: 10.1016/s0169-5347(00)89081-3
Cook, B. I., Wolkovich, E. M., and Parmesan, C. (2012). Divergent responses to spring and winter warming drive community level flowering trends. Proc. Natl. Acad. Sci. U.S.A. 109, 9000–9005. doi: 10.1073/pnas.1118364109
Cooper, H. F., Grady, K. C., Cowan, J. A., Best, R. J., Allan, G. J., and Whitham, T. G. (2019). Genotypic variation in phenological plasticity: reciprocal common gardens reveal adaptive responses to warmer springs but not to fall frost. Glob. Chang. Biol. 25, 187–200. doi: 10.1111/gcb.14494
Cotto, O., Sandell, L., Chevin, L.-M., and Ronce, O. (2019). Maladaptive shifts in life history in a changing environment. Am. Nat. 194, 558–573. doi: 10.1086/702716
Datta, A., Kuhn, I., Ahmad, M., Michalski, S., and Auge, H. (2017). Processes affecting altitudinal distribution of invasive Ageratina adenophora in western Himalaya: the role of local adaptation and the importance of different life-cycle stages. PLoS One 12:e0187708. doi: 10.1371/journal.pone.0187708
Davis, M. B., and Shaw, R. G. (2001). Range shifts and adaptive responses to quaternary climate change. Science 292, 673–679. doi: 10.1126/science.292.5517.673
De Kort, H., Vander Mijnsbrugge, K., Vandepitte, K., Mergeay, J., Ovaskainen, O., and Honnay, O. (2016). Evolution, plasticity and evolving plasticity of phenology in the tree species Alnus glutinosa. J. Evol. Biol. 29, 253–264. doi: 10.1111/jeb.12777
de Valpine, P., and Harte, J. (2001). Plant responses to experimental warming in a montane meadow. Ecology 82, 637–648. doi: 10.1890/0012-9658(2001)082[0637:prtewi]2.0.co;2
Dingle, H., Mousseau, T. A., and Scott, S. M. (1990). Altitudinal variation in life cycle syndromes of California populations of the grasshopper, Melanoplus sanguinipes (F.). Oecologia 84, 199–206. doi: 10.1007/bf00318272
Donohue, K. (2002). Germination timing influences natural selection on life-history characteristics in Arabidopsis thaliana. Ecology 83, 1006–1016. doi: 10.1890/0012-9658(2002)083[1006:gtinso]2.0.co;2
Duputié, A., Rutschmann, A., Ronce, P., and Chuine, I. (2015). Phenological plasticity will not help all species adapt to climate change. Global Change Biol. 21, 3062–3073. doi: 10.1111/gcb.12914
Eckert, C., Samis, K., and Lougheed, S. (2008). Genetic variation across species’ geographical ranges: the central-marginal hypothesis and beyond. Mol. Ecol. 17, 1170–1188. doi: 10.1111/j.1365-294x.2007.03659.x
Eckhart, V. M., Geber, M. A., and McGuire, C. M. (2004). Experimental studies of adaptation in Clarkia xantiana. I. Sources of trait variation across a subspecies border. Evolution 58, 59–70. doi: 10.1111/j.0014-3820.2004.tb01573.x
Ensing, D. J., and Eckert, C. G. (2019). Interannual variation in season length is linked to strong co-gradient plasticity of phenology in a montane annual plant. New Phytol. 224, 1184–1200. doi: 10.1111/nph.16009
Etterson, J. R., and Shaw, R. G. (2001). Constraint to adaptive evolution in response to global warming. Science 294, 151–154. doi: 10.1126/science.1063656
Evans, L. M., Kaluthota, S., Pearce, D. W., Allan, G. J., Floate, K., Rood, S. B., et al. (2016). Bud phenology and growth are subject to divergent selection across a latitudinal gradient in Populus angustifolia and impact adaptation across the distributional range and associated arthropods. Ecol. Evol. 6, 4565–4581. doi: 10.1002/ece3.2222
Firmat, C., Delzon, S., Louvet, J.-M., Parmentier, J., and Kremer, A. (2017). Evolutionary dynamics of the leaf phenological cycle in an oak metapopulation along an elevation gradient. J. Evol. Biol. 30, 2116–2131. doi: 10.1111/jeb.13185
Flynn, D. F. B., and Wolkovich, E. (2018). Temperature and photoperiod drive spring phenology across all species in a temperate forest community. New Phytol. 219, 1353–1362. doi: 10.1111/nph.15232
Franks, S. J. (2015). The unique and multifaceted importance of the timing of flowering. Am. J. Bot. 102, 1401–1402. doi: 10.3732/ajb.1500234
Franks, S. J., and Weis, A. E. (2008). A change in climate causes rapid evolution of multiple life-history traits and their interactions in an annual plant. J. Evol. Bio. 21, 1321–1334. doi: 10.1111/j.1420-9101.2008.01566.x
Frei, E. R., Ghazoul, J., Matter, P., Heggli, M., and Pluess, A. R. (2014). Plant population differentiation and climate change: responses of grassland species along an elevational gradient. Glob. Chang. Biol. 20, 441–455. doi: 10.1111/gcb.12403
Fu, Y., Piao, S., Vitasse, Y., Zhao, H., De Boeck, H. J., Liu, Q., et al. (2015). Increased heat requirements for leaf flushing in temperature woody species over 1980-2012: effects of chilling, precipitation and insolation. Glob. Chang. Biol. 21, 2687–2697. doi: 10.1111/gcb.12863
Fu, Y., Piao, S., Zhou, X., Geng, X., Hao, F., Vitasse, Y., et al. (2019). Short photoperiod reduces the temperature sensitivity of leaf-out in saplings of Fagus sylvatica but not in horse chestnut. Glob. Chang. Biol. 25, 1696–1703. doi: 10.1111/gcb.14599
Gaston, J. J. (2009). Geographic range limits of species. Proc. R. Soc. B 276, 1391–1393. doi: 10.1098/rspb.2009.0100
Gauzere, J., Teuf, B., Davi, H., Chevin, L.-M., Caignard, T., Leys, B., et al. (2020). Where is the optimum? Predicting the variation of selection along climatic gradients and the adaptive value of plasticity. A case study on tree phenology. Evol. Lett. 4, 109–123. doi: 10.1002/evl3.160
Ghalambor, C. K., McKay, J. K., Carroll, S. P., and Reznick, D. N. (2007). Adaptive versus non-adaptive phenotypic plasticity and the potential for contemporary adaptation in new environments. Funct. Ecol. 21, 394–407. doi: 10.1111/j.1365-2435.2007.01283.x
Giménez-Benavides, L., Escudero, A., and Iriondo, J. M. (2006). Reproductive limits of a late-flowering high-mountain Mediterranean plant along an elevational climate gradient. New Phytol 173, 367–382. doi: 10.1111/j.1469-8137.2006.01932.x
Grady, K. C., Ferrier, S. M., Kolb, T. E., Hart, S. C., Allan, G. J., and Whitman, T. G. (2011). Genetic variation in productivity of foundation riparian species at the edge of their distribution: implications for restoration and assisted migration in a warming climate. Glob. Chang. Ecol. 17, 3724–3735. doi: 10.1111/j.1365-2486.2011.02524.x
Granado-Yela, C., Balaguer, L., García-Verdugo, C., and Méndez, M. (2013). Thriving at the limit: differential reproductive performance in range-edge populations of a Mediterranean sclerophyll (Olea europaea). Acta. Oecol. 52, 29–37. doi: 10.1016/j.actao.2013.07.002
Griffith, T. M., and Watson, M. A. (2005). Stress avoidance in a common annual: reproductive timing is important for local adaptation and geographic distribution. J. Evol. Biol. 18, 1601–1612. doi: 10.1111/j.1420-9101.2005.01021.x
Gugger, S., Kesselring, H., Stöcklin, J., and Hamann, E. (2015). Lower plasticity exhibited by high- versus mid-elevation species in their phenological responses to manipulated temperature and drought. Ann. Bot. 116, 953–962.
Gurevitch, J., Curtis, P., and Jones, M. H. (2001). Meta-analysis in ecology. Adv. Ecol. Res. 32, 199–247.
Haggerty, B. P., and Galloway, L. F. (2011). Response of individual components of reproductive phenology to growing season length in a monocarpic herb. J. Ecol. 99, 242–253. doi: 10.1111/j.1365-2745.2010.01744.x
Hall, E. S., Piedrahita, L. R., Kendziorski, G., Waddle, E., Doak, D. F., and Peterson, M. L. (2018). Climate and synchrony with conspecifics determine the effects of flowering phenology on reproductive success in Silene acaulis. Arc. Antarct. Alp. Res. 50:e1548866. doi: 10.1080/15230430.2018.1548866
Hällfors, M. H., Aikio, S., and Schulman, L. E. (2017). Quantifying the need and potential of assisted migration. Biol. Conserv. 205, 34–41. doi: 10.1016/j.biocon.2016.11.023
Hampe, A., and Petit, R. J. (2005). Conserving biodiversity under climate change: the rear edge matters. Ecol. Lett. 8, 461–467. doi: 10.1111/j.1461-0248.2005.00739.x
Hänel, S., and Tielbörger, K. (2015). Phenotypic response of plants to simulated climate change in a long-term rain-manipulation experiment: a multi-species study. Oecologia 177, 1015–1024. doi: 10.1007/s00442-015-3231-8
Hargreaves, A., and Eckert, C. G. (2019). Local adaptation primes cold-edge populations for range expansion but not warming-induced range shifts. Ecol. Lett. 22, 78–88. doi: 10.1111/ele.13169
Hargreaves, A., Samis, K. E., and Eckert, C. G. (2014). Are species’ range limits simply niche limits writ large? A review of transplant experiments beyond the range. Am. Nat. 183, 157–173. doi: 10.1086/674525
Hengeveld, R., and Haeck, J. (1982). The distribution of abundance. I. Measurements. J. Biogeogr. 9, 303–316. doi: 10.2307/2844717
Hirao, A. S., and Kudo, G. (2004). Landscape genetics of alpine-snowbed plants: comparisons along geographic and snowmelt gradients. Heredity 93, 290–298. doi: 10.1038/sj.hdy.6800503
Inouye, D. W. (2008). Effects of climate change on phenology, frost damage, and floral abundance of montane wildflowers. Ecology 89, 353–362. doi: 10.1890/06-2128.1
IPCC (2014). Climate Change 2014: Synthesis Report. Contribution of Working Groups I, II and III to the Fifth Assessment Report of the Intergovernmental Panel on Climate Change, eds P. K. Pachauri and L. A. Meyer (Geneva: IPCC).
Ishizuka, W., Ono, K., Hara, T., and Goto, S. (2015). Use of intraspecific variation in thermal responses for estimating an elevational cline in the timing of cold hardening in a sub-boreal conifer. Plant Biol. 17, 177–185. doi: 10.1111/plb.12214
Jeong, M.-S., Kim, H., and Lee, W.-S. (2020). Spatio-temporal variation in egg-laying dates of nestbox-breeding varied tits (Poecile varius) in response to spring pre-breeding period temperatures at long-term study sites in South Korea and Japan. J. For. Res. 25, 232–241. doi: 10.1080/13416979.2020.1779424
Körner, C., and Basler, D. (2010). Phenology under global warming. Science 327, 1461–1462. doi: 10.1126/science.1186473
Körner, C., Basler, D., Hoch, G., Kollas, C., Lenz, A., Randin, C. F., et al. (2016). Where, why and how? Explaining the low-temperature range limits of temperate tree species. J. Ecol. 104, 1076–1088. doi: 10.1111/1365-2745.12574
LaBarbera, K., and Lacey, E. A. (2018). Breeding season length and nest mortality drive cryptic life history variation in Dark-eyed Juncos (Juncos hyemalis) breeding across a ontane elevational gradient. Auk 135, 284–298. doi: 10.1642/auk-17-184.1
Lawton, J. H. (1993). Range, population abundance and conservation. Trends Evol. Ecol. 8, 409–413. doi: 10.1016/0169-5347(93)90043-o
Lázaro-Nogal, A., Matesanz, S., Godoy, A., Pérez-Trautman, F., Gianoli, E., and Valladares, F. (2015). Environmental heterogeneity leads to higher plasticity in dry-edge populations of a semi-arid Chilean shrub: insights in climate change responses. J. Ecol. 103, 338–350. doi: 10.1111/1365-2745.12372
Lenoir, J., Gégout, J. C., Marquet, P. A., de Ruffray, P., and Brisse, H. (2008). A significant upward shift in plant species optimum elevation during the 20th century. Science 320, 1768–1771. doi: 10.1126/science.1156831
Leonardi, S., Piovani, P., Scalfi, M., Piotti, A., Giannini, R., and Menozzi, P. (2012). Effects of habitat fragmentation on the genetic diversity and structure of peripheral populations of beech in central Italy. J. Hered. 103, 408–417. doi: 10.1093/jhered/ess004
Levin, D. A. (2009). Flowering-time plasticity facilitates niche shifts in adjacent populations. New Phytol. 183, 661–666. doi: 10.1111/j.1469-8137.2009.02889.x
Loss, S. R., Terwilliger, L. A., and Peterson, A. C. (2011). Assisted colonization: integrating conservation strategies in the face of climate change. Biol. Conserv. 144, 92–100. doi: 10.1016/j.biocon.2010.11.016
Louthan, A. M., Doak, D. F., and Angert, A. L. (2015). Where and when do species interactions set range limits? Trends Ecol. Evol. 30, 780–792. doi: 10.1016/j.tree.2015.09.011
Lustenhouwer, N., Wilschut, R. A., Williams, J. L., van der Putten, W. H., and Levine, J. M. (2018). Rapid evolution of phenology during range expansion with recent climate change. Glob. Chang. Biol. 24, e534–e544.
Mägi, M., Semchenko, M., Kalamees, R., and Zobel, K. (2011). Limited phenotypic plasticity in range-edge populations: a comparison of co-occurring populations of two Agrimonia species with different geographical distributions. Plant Bio. 13, 177–184. doi: 10.1111/j.1438-8677.2010.00342.x
McGuire, C. R., Nufio, C. R., Bowers, M. D., and Guralnick, R. P. (2012). Elevation-dependent temperature trends in the Rocky Mountain front range: changes over a 56- and 20-year record. PLoS One 7:e44370. doi: 10.1371/journal.pone.0044370
McLean, N., Lawson, C. R., Leech, D. I., and van de Pol, M. (2016). Predicting when climate-driven phenotypic change affects population dynamics. Ecol. Lett. 19, 595–608. doi: 10.1111/ele.12599
Mediavilla, S., and Escudero, A. (2009). Ontogenetic changes in leaf phenology of two co-occurring Mediterranean oaks differing in leaf life span. Ecol. Res. 24, 1083–1090. doi: 10.1007/s11284-009-0587-4
Meng, L., Zhou, Y., Gu, L., Richardson, A., Peñuelas, J., Fu, Y., et al. (2021). Photoperiod decelerates the advance of spring phenology of six deciduous tree species under climate warming. Glob. Chang. Biol. 27, 2914–2927. doi: 10.1111/gcb.15575
Mlynarek, J. J., Moffat, C. E., Edwards, S., Einfeldt, A. L., Heustis, A., Johns, R., et al. (2017). Enemy escape: a general phenomenon in a fragmented literature? Facets 2, 1015–1044. doi: 10.1139/facets-2017-0041
Morin, X., Auspurger, C., and Chuine, I. (2007). Process-based modeling of species’ distributions: what limits temperate tree species’ range boundaries? Ecology 88, 2280–2291. doi: 10.1890/06-1591.1
Morin, X., Viner, D., and Chuine, I. (2008). Tree species range shifts at a continental scale: new predictive insights from a process-based model. J. Ecol. 96, 784–794. doi: 10.1111/j.1365-2745.2008.01369.x
Munguía-Rosas, M. A., Ollerton, J., Parra-Tabla, V., and De Nova, J. A. (2011). Meta-analysis of phenotypic selection on flowering phenology suggests that early flowering plants are favoured. Ecol. Lett. 14, 511–521. doi: 10.1111/j.1461-0248.2011.01601.x
Münzbergová, Z., Hadincová, V., Skálová, H., and Vandvik, V. (2017). Genetic differentiation and plasticity interact along temperature and precipitation gradients to determine plant performance under climate change. J. Ecol. 105, 1358–1373. doi: 10.1111/1365-2745.12762
Pardee, G. L., Jensen, I. O., Inouye, D. W., and Irwin, R. E. (2019). The individual and combined effects of snowmelt timing and frost exposure on the reproductive success of montane forbs. J. Ecol. 107, 1970–1981. doi: 10.1111/1365-2745.13152
Peterson, M. L., Angert, A. L., and Kay, K. M. (2020). Experimental migration upward in elevation is associated with strong selection on life history traits. Ecol. Evol. 10, 612–625. doi: 10.1002/ece3.5710
Petit, R. J., Aguinagalde, I., de Beaulieu, J.-L., Bittkau, C., Brewer, S., Cheddadi, R., et al. (2003). Glacial refugia: hotspots but not melting pots of genetic diversity. Science 300, 1563–1565. doi: 10.1126/science.1083264
Piao, S., Liu, Q., Chen, A., Janssens, I. A., Fu, Y., Dai, J., et al. (2019). Plant phenology and global climate change: Current progresses and challenges. Glob. Chang. Biol. 25, 1922–1940. doi: 10.1111/gcb.14619
Pigliucci, M. (2001). Phenotypic Plasticity: Beyond Nature and Nurture. Baltimore, MD: Johns Hopkins University Press.
Pironon, S., Papuga, G., Villellas, J., Angert, A. L., García, M. B., and Thompson, J. D. (2017). Geographic variation in genetic and demographic performance: new insights from an old biogeographical paradigm. Biol. Rev. 92, 1877–1909. doi: 10.1111/brv.12313
Posledovich, D., Toftegaard, T., Wiklund, C., Ehrlen, J., and Gotthard, K. (2018). Phenological synchrony between a butterfly and its host plants: experimental test of the effects of spring temperature. J. Anim. Ecol. 87, 150–161. doi: 10.1111/1365-2656.12770
Rafferty, N. E., Bertelsen, C. D., and Bronstein, J. L. (2016). Later flowering is associated with a compressed flowering season and reduced reproductive output in an early season floral resource. Oikos 125, 821–828. doi: 10.1111/oik.02573
Rafferty, N. E., Diaz, J. M., and Bertelson, C. D. (2020). Changing climate drives divergent and nonlinear shifts in flowering phenology across elevations. Curr. Biol. 30, 432–441. doi: 10.1016/j.cub.2019.11.071
Rafferty, N. E., and Ives, A. R. (2012). Pollinator effectiveness varies with experimental shifts in flowering time. Ecology 93, 803–814. doi: 10.1890/11-0967.1
Reed, T. E., Waples, R. S., Schindler, D. E., Hard, J. J., and Kinnison, M. T. (2010). Phenotypic plasticity and population viability: the importance of environmental predictability. Proc. R. Soc. B. 277, 3391–3400. doi: 10.1098/rspb.2010.0771
Rehm, E. M., Olivas, P., Stroud, J., and Feeley, K. J. (2015). Losing your edge: climate change and the conservation value of range-edge populations. Ecol. Evol. 5, 4315–4326. doi: 10.1002/ece3.1645
Renner, S. S., and Zohner, C. M. (2018). Climate change and phenological mismatch in trophic interactions among plants, insects, and vertebrates. Annu. Rev. Ecol. Evol. Syst. 49, 165–182. doi: 10.1146/annurev-ecolsys-110617-062535
Richardson, A. D., Hufkens, K., Milliman, T., Aubrecht, D. M., Furze, M. E., Seyednasrollah, B., et al. (2018). Ecosystem warming extends vegetation activity but heightens vulnerability to cold temperatures. Nature 560, 368–371. doi: 10.1038/s41586-018-0399-1
Richter, S., Kipfer, T., Wohlgemuth, T., Cuerrero, C. C., Ghazoul, J., and Moser, B. (2012). Phenotypic plasticity facilitates resistance to climate change in a highly variable environment. Oecologia 169, 269–279. doi: 10.1007/s00442-011-2191-x
Rivest, S., Lajoie, G., Watts, D. A., and Vellend, M. (2021). Earlier spring reduces potential for gene flow via reduced flowering synchrony across an elevational gradient. Am. J. Bot. 108, 538–545. doi: 10.1002/ajb2.1627
Root, T. L., Price, J. T., Hall, K. R., Schneider, S. H., Rosenzweig, C., and Pounds, J. A. (2003). Fingerprints of global warming on wild animals and plants. Nature 421, 57–60. doi: 10.1038/nature01333
Roy, D. B., Oliver, T. H., Botham, M. S., Beckmann, B., Brereton, T., Dennis, R. L., et al. (2015). Similarities in butterfly emergence dates among populations suggest local adaptation to climate. Glob. Chang. Biol. 21, 3313–3322. doi: 10.1111/gcb.12920
Sakurai, A., and Takahasi, K. (2016). Flowering phenology and reproduction of the Solidago virgaurea L. complex along an elevational gradient on Mt. Norikura, central Japan. Plant Species Biol. 32, 270–278. doi: 10.1111/1442-1984.12153
Sexton, J. P., McIntyre, P. J., Angert, A. L., and Rice, K. J. (2009). Evolution and ecology of species range limits. Annu. Rev. Ecol. Evol. Syst. 40, 415–436. doi: 10.1146/annurev.ecolsys.110308.120317
Sheth, S. N., and Angert, A. L. (2014). The evolution of environmental tolerance and range size; a comparison of geographically restricted and widespread Mimulus. Evolution 68, 2917–2931. doi: 10.1111/evo.12494
Sheth, S. N., and Angert, A. L. (2016). Artificial selection reveals high genetic variation in phenology at the trailing edge of a species range. Am. Nat. 187, 182–193. doi: 10.1086/684440
Sjölund, M. J., González-Díaz, P., Moreno-Villena, J. J., and Jump, A. S. (2019). Gene flow at the leading range edge: the long-term consequences of isolation in European beech (Fagus sylvatica L. Kuhn). J. Biogeogr. 46, 2787–2799. doi: 10.1111/jbi.13701
Soularue, J.-P., and Kremer, A. (2012). Assortative mating and gene flow generate clinal phenological variation in trees. BMC Evol. Biol. 12:79. doi: 10.1186/1471-2148-12-79
Stamp, M. A., and Hadfield, J. D. (2020). The relative importance of plasticity versus genetic differentiation in explaining between population differences; a meta-analysis. Ecol. Lett. 23, 1432–1441.
Sunday, J. M., Bates, A. E., and Dulvy, N. K. (2012). Thermal tolerance and the global redistribution of animals. Nat. Clim. Chang. 2, 686–690. doi: 10.1038/nclimate1539
Tang, J., He, H., Chen, C., Fu, S., and Zue, F. (2017). Latitudinal cogradient variation of development time and growth rate and a negative latitudinal body weight cline in a widely distributed cabbage beetle. PLoS One 12:e0181030. doi: 10.1371/journal.pone.0181030
Tansey, C. J., Hadfield, J. D., and Phillimore, A. B. (2017). Estimating the ability of plants to plastically track temperature-mediated shifts in the spring phenological optimum. Glob. Chang. Biol. 23, 3321–3334. doi: 10.1111/gcb.13624
Telwala, Y., Brook, B. W., Manish, K., and Pandit, M. K. (2013). Climate-induced elevational range shifts and increase in plant species richness in a Himalayan biodiversity epicentre. PLoS One 8:e57103. doi: 10.1371/journal.pone.0181030
Thackeray, S. J., Henrys, P. A., Hemming, D., Bell, J. R., Botham, M. S., Burthe, S., et al. (2016). Phenological sensitivity to climate across taxa and trophic levels. Nature 535, 241–245.
Torres-Martínez, L., Weldy, P., Levy, M., and Emery, N. C. (2017). Spatiotemporal heterogeneity in precipitation patterns explain population-level germination strategies in an edaphic specialist. Ann. Bot. 119, 253–265. doi: 10.1093/aob/mcw161
Uelmen, J. A. Jr., Lindroth, R. L., Tobin, P. C., Reich, P. B., Schwartzberg, E. G., and Raffa, K. F. (2016). Effects of winter temperatures, spring degree-day accumulation, and insect population source on phenological synchrony between forest tent caterpillar and host trees. For. Ecol. Manag. 362, 241–250. doi: 10.1016/j.foreco.2015.11.045
Urban, M. C., Bodedi, G., Hendry, A. P., Mihoub, J.-B., Pe’er, G., Singer, A., et al. (2016). Improving the forecast for biodiversity under climate change. Science 353, aad8466.
Välimäki, P., Kivela, S. M., Maenpaa, M. I., and Tammaru, T. (2013). Latitudinal clines in alternative life histories in a geometrid moth. J. Evol. Biol. 26, 118–129. doi: 10.1111/jeb.12033
Valladares, F., Matesanz, S., Guilhaumon, F., Araújo, M. B., Balaguer, L., Benito-Garzón, M., et al. (2014). The effects of phenotypic plasticity and local adaptation on forecasts of species range shifts under climate change. Ecol. Lett. 17, 1351–1364. doi: 10.1111/ele.12348
Visser, M. E., and Gienapp, P. (2019). Evolutionary and demographic consequences of phenological mismatches. Nat. Ecol. Evol. 3, 879–885. doi: 10.1038/s41559-019-0880-8
Vitasse, Y., Lenz, A., Hoch, G., and Körner, C. (2014). Earlier leaf-out rather than difference in freezing resistance puts juvenile trees at greater risk of damage than adult trees. J. Ecol. 102, 981–988. doi: 10.1111/1365-2745.12251
Vitt, P., Havens, K., Kramer, A. T., Sollenberger, D., and Yates, E. (2010). Assisted migration of plants: changes in latitudes, changes in attitudes. Biol. Conserv. 143, 18–27. doi: 10.1016/j.biocon.2009.08.015
Volis, S., Mendlinger, S., Olsvig-Whittaker, L., Safriel, U. N., and Orlovsky, N. (1998). Phenotypic variation and stress resistance in core and peripheral populations of Hordeum spontaneum. Biod. Conserv. 7, 799–813. doi: 10.1023/a:1008844504010
Wadgymar, S. M., Cumming, M. N., and Weis, A. E. (2015). The success of assisted colonization and assisted gene flow depends on phenology. Glob. Chang. Biol. 21, 3786–3799. doi: 10.1111/gcb.12988
Weis, A. E. (2015). On the potential strength and consequences for nonrandom gene flow caused by local adaptation in flowering time. J. Evol. Biol. 28, 699–714. doi: 10.1111/jeb.12612
Weis, A. E., and Kossler, T. M. (2004). Genetic variation in flowering time induces phenological assortative mating: quantitative genetic methods applied to Brassica rapa. Am. J. Bot. 91, 825–836. doi: 10.3732/ajb.91.6.825
Wenden, B., Mariadassou, M., Chmielewski, F.-M., and Vitasse, Y. (2020). Shifts in the temperature-sensitive periods for spring phenology in European beech and pedunculate oak clones across latitudes and over recent decades. Glob. Chang. Biol. 26, 1808–1819. doi: 10.1111/gcb.14918
Wolkovich, E. M., Cook, B. I., Allen, J. M., Crimmins, T. M., Betancourt, J. L., Travers, S. E., et al. (2012). Warming experiments underpredict plant phenological responses to climate change. Nature 485, 494–497. doi: 10.1038/nature11014
Wright, I. J., Reich, P. B., Westoby, M., Ackerly, D. D., Baruch, Z., Bongers, F., et al. (2004). The worldwide leaf economics spectrum. Nature 428, 821–827.
Yamagishi, H., Allison, T. D., and Ohara, M. (2005). Effect of snowmelt timing on the genetic structure of an Erythronium grandiflorum population in an alpine environment. Ecol. Res. 20, 199–204. doi: 10.1007/s11284-004-0032-7
Zettlemoyer, M. A., Prendeville, H. R., and Galloway, L. F. (2017). The effect of a latitudinal temperature gradient on germination patterns. Int. J. Plant Sci. 178, 673–679. doi: 10.1086/694185
Zettlemoyer, M. A., Renaldi, K., Muzyka, M. D., and Lau, J. A. (2021). Extirpated prairie species demonstrate more variable phenological responses to warming than extant congeners. Am. J. Bot 108, 1–13. doi: 10.1002/ajb2.1684
Keywords: phenology, adaptive plasticity, co-gradient variation, geographic cline, range shift, counter-gradient variation, genetic cline, range expansion
Citation: Zettlemoyer MA and Peterson ML (2021) Does Phenological Plasticity Help or Hinder Range Shifts Under Climate Change? Front. Ecol. Evol. 9:689192. doi: 10.3389/fevo.2021.689192
Received: 31 March 2021; Accepted: 25 May 2021;
Published: 15 June 2021.
Edited by:
Marta Benito Garzon, INRAE Nouvelle-Aquitaine Bordeaux, FranceReviewed by:
Anne Duputié, Université de Lille, FranceCopyright © 2021 Zettlemoyer and Peterson. This is an open-access article distributed under the terms of the Creative Commons Attribution License (CC BY). The use, distribution or reproduction in other forums is permitted, provided the original author(s) and the copyright owner(s) are credited and that the original publication in this journal is cited, in accordance with accepted academic practice. No use, distribution or reproduction is permitted which does not comply with these terms.
*Correspondence: Meredith A. Zettlemoyer, bWVyZWRpdGguemV0dGxlbW95ZXIyNUB1Z2EuZWR1
†ORCID: Meredith A. Zettlemoyer, 0000-0002-8203-7207; Megan L. Peterson, 0000-0002-5010-2721
Disclaimer: All claims expressed in this article are solely those of the authors and do not necessarily represent those of their affiliated organizations, or those of the publisher, the editors and the reviewers. Any product that may be evaluated in this article or claim that may be made by its manufacturer is not guaranteed or endorsed by the publisher.
Research integrity at Frontiers
Learn more about the work of our research integrity team to safeguard the quality of each article we publish.