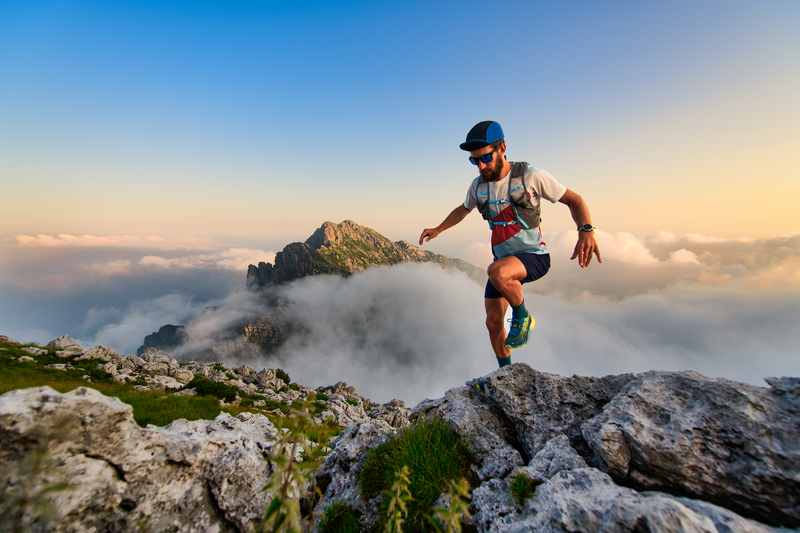
95% of researchers rate our articles as excellent or good
Learn more about the work of our research integrity team to safeguard the quality of each article we publish.
Find out more
REVIEW article
Front. Ecol. Evol. , 13 October 2021
Sec. Conservation and Restoration Ecology
Volume 9 - 2021 | https://doi.org/10.3389/fevo.2021.682349
This article is part of the Research Topic Nature-Based Solutions to Optimize Marine Ecosystems View all 8 articles
As the artificial defenses often required for urban and industrial development, such as seawalls, breakwaters, and bund walls, directly replace natural habitats, they may produce population fragmentation and a disruption of ecological connectivity, compromising the delivery of ecosystem services. Such problems have increasingly been addressed through “Working with Nature” (WwN) techniques, wherein natural features such as species and habitats are included as additional functional components within the design of built infrastructure. There now exists a convincing body of empirical evidence that WwN techniques can enhance the structural integrity of coastal works, and at the same time promote biodiversity and ecosystem services. While these benefits have often been achieved through modification of the hard surfaces of the coastal defense structures themselves, the desired ecological and engineering goals may often demand the creation of new soft substrates from sediment. Here we discuss the design considerations for creating new sediment habitats in the intertidal zone within new coastal infrastructure works. We focus on the sediment control structures required to satisfy the physiological and ecological requirements of seagrass and mangroves – two keystone intertidal species that are common candidates for restoration – and illustrate the concepts by discussing the case study of soft habitat creation within a major multi-commodity port.
It is broadly recognized that the degree of degradation of coastal ecosystems is such that protection alone is no longer sufficient to guarantee the ongoing provision of their essential services (Abelson et al., 2016; Ounanian et al., 2018). It has been argued that restoring or creating novel complex benthic ecosystems, and the services they provide, by increasing the abundance of keystone habitat-forming species, will be essential for meeting future human demographic challenges (Duarte et al., 2020). As such, so-called “Working with Nature” (WwN) techniques have gained traction over recent decades as fundamental tools in conservation, and significant progress has been made to seat the efforts to create novel or restored ecosystems within broader ecological theory (Palmer et al., 2016; Statton et al., 2018). For example, the modification of otherwise flat concrete surfaces of seawalls, greatly increasing the availability of ecological niches and substrate, has been used to encourage the settlement of native marine flora and fauna and increase biodiversity on seawalls (Perkol-Finkel et al., 2018; Strain et al., 2018, 2020). WwN approaches have also been used to control so-called “soft” or sediment habitats, such as the use of melaleuca fences to reduce wave energy and increase sediment accretion for mangrove restoration attempts (Van Cuong et al., 2015). Related concepts to WwN, often used somewhat interchangeably in the literature for purposeful efforts to restore or rehabilitate natural ecosystems, include building with nature, ecological engineering, ecoengineering, and reconciliation ecology. In addition, many of the techniques applied in WwN projects of coastal ecosystem restoration have been used as components for achieving other end goals, such as for increasing natural capital (Cziesielski et al., 2021), building green infrastructure (Ruckelshaus et al., 2016; Silva et al., 2017), and for promoting Ecosystem Based Adaptation (Hale et al., 2009; Sierra-Correa and Kintz, 2015).
While many habitat restoration and rehabilitation projects have been implemented at both experimental and large scale with ecosystem function as the primary focus, the opportunity to achieve ecological goals may also appear within the context of works of coastal engineering. In these cases, natural or restored habitats are incorporated as functional elements within the design of the structure, commonly to augment coastal defenses (Sutton-Grier et al., 2018). Indeed, these natural elements can prove more cost effective than traditional construction techniques, even before quantifying the ecosystem services (Narayan et al., 2016). The possibility to leverage positive ecological outcomes within coastal development projects represents a promising opportunity for coastal ecosystem conservation, and there is a growing body of empirical evidence that the implementation of WwN approaches within coastal works can provide both ecological and operational benefits. The habitat restoration techniques developed with protection as the primary function, however, may also be highly relevant for projects in industrial settings whose goal is conservation alone. Several marine examples now exist that demonstrate how biodiversity and ecosystem services may be maintained within urban and engineered habitats (Mitsch and Jørgensen, 2003; Hobbs et al., 2013; Perring et al., 2013; Firth et al., 2016). Coastal restoration initiatives involving oysters, seagrass, saltmarsh and mangroves have already demonstrated quantifiable improvements in water quality, carbon uptake and coastline stability (Lotze et al., 2006; Das and Vincent, 2009; Taillardat et al., 2018).
The design of WwN projects must commence from a clear identification of the sought ecological and functional outcomes, and how these two independent variables interact. For example, which of the physical functions that the eco-engineered habitat will provide are essential (e.g., coastal defense, conservation of an endangered species), and which are just desirable (e.g., improved water quality, recreational use)? Is the ecological goal “restoration” – recovery of the native ecosystem (Gann et al., 2019) – or “rehabilitation” – recovery only of some ecosystem services (Miller and Bestelmeyer, 2016; Zimmer, 2018)? The choice of WwN strategy should then be determined with reference to this possibly complex metric. While ecoengineering projects have commonly been driven from the conservation perspective (Dafforn et al., 2015; Mayer-Pinto et al., 2017), there is now sufficient evidence that such techniques can achieve both conservation and construction benefits when employed in civil/industrial projects in the coastal zone, such as coastal defense (protection of infrastructure on erosive coastlines), but also bunded walls for containing reclaimed land or stormwater, wharves and marinas. And hence represent viable alternatives to traditional construction techniques (Gittman et al., 2014; Narayan et al., 2016; Smallegan et al., 2016; Vuik et al., 2016; Smith et al., 2017; Morris et al., 2018). The reasons for the documented ecoengineering failures commonly stem from an incomplete appreciation of the physiological constraints and ecological traits of the target species (e.g., Samson and Rollon, 2008).
While a successfully restored ecosystem may, eventually, provide equivalent services to a conserved one, the practical challenges for implementing rehabilitation and conservation are divergent. Fundamentally, rehabilitation involves a purposeful change in the actual ecological function of a site, necessarily confronting the moral dilemma of ranking species importance. This choice is often relatively straight-forward – as in the case of highly degraded ecosystems – but not always. In practice, the task of engineering complex, functional ecosystems, potentially from a highly degraded state, begets both a sophisticated and broad understanding of the ecosystem ecology, and the resources necessary to implement, and monitor, the determined solutions.
The introduction of new hard structures in the coastal zone has commonly been associated with negative impacts on habitat quality that reach beyond the project’s immediate footprint, by altering the near field patterns of sedimentation/erosion, providing a beachhead for invasive species, and interrupting connectivity of the native populations (Chapman, 2003; Chapman and Bulleri, 2003; Browne and Chapman, 2014). The new artificial habitats created are likely to give rise to depauperate ecosystems characterized by low biodiversity and dominated by invasive species (Bulleri and Airoldi, 2005; Glasby et al., 2007; Dafforn et al., 2015; O’Shaughnessy et al., 2019). The prevalence of non-native taxa on hard coastal defenses in urban areas may be related to their proximity to known invasion hubs such as marinas and shipping facilities (Seebens et al., 2013), to high rates of disturbance (Bulleri and Airoldi, 2005), and/or to relatively low levels of predation by mobile consumers (Simkanin, 2013). The resulting degradation of ecosystem services brings quantifiable knock-on costs for society, and as a result, a significant degree of the research focus has been placed upon the hard surface itself – how the substrate may best be modified to sustain a native ecosystem (e.g., Perkol-Finkel et al., 2018).
The sought operational or ecological goals may, however, involve modification of the landscape adjacent to the hard surface. In particular, habitat restoration that involves manipulating the coastal bathymetry has often been used to arrest coastal recession. This has been referred to as, among other names, “soft ecological engineering” (Temmerman et al., 2013; Narayan et al., 2016) and is embodied in the “living shoreline” approach developed in the 1960s (Garbisch and Garbisch, 1994), where careful terraforming of degraded shores allowed colonization by saltmarsh, and the subsequent development of resilient dunes. Rehabilitated mangrove, seagrass, coral and oyster habitats have been demonstrated to provide coastal protection that is as effective as traditional techniques (Narayan et al., 2016). As these species are highly immersion sensitive, significant terraforming may be required to ensure that the substrate lies at a suitable elevation. In the case of sediment substrate, this may be achieved by modifying the hydrodynamic conditions to encourage natural sedimentation (e.g., Winterwerp et al., 2020), or by the deliberate placing of sediments from another source (Yozzo et al., 2004; Baptist et al., 2019). In particular, the potential exists for dredged material that is presently destined to land reclamation to be usefully repurposed for conservation. In either case, the construction of secondary hard structures, such as permeable dams, breakwaters or groynes, or modification of the primary structure, such as adding crenulations, may be required to prevent erosion prior to consolidation of the placed sediment. While successful examples exist of creating sediment habitats either intentionally or unintentionally (Sheridan, 2004; Osland et al., 2012), the design of structures to use for sediment management remains non-trivial and careful consideration of local conditions remains vital for a successful restoration.
In section “Key Concepts for Restoration of Soft Intertidal Habitats” we review the design considerations for the restoration or creation of intertidal mangrove and seagrass habitats, using natural or repurposed sediment to achieve a suitable substrate. The section firstly discusses the requirements for placed or accreted sediments to not resuspend, and then the physiological and ecological conditions that must be considered for ecosystems based on mangroves or seagrass to develop on the new sediment substrate. The concepts are exemplified in section “Case Study: “Living Seawall” Options for an Industrial Port” with reference to an industry-driven coastal rehabilitation project in a major industrial port.
The design of effective secondary control structures to manage coastal sediment requires an understanding of their dynamics. The sediment composition of coastal habitats is in constant flux. Depending on the kinetic or turbulent energy of the flow, bottom sediment is continually being exchanged with the water column via suspension and deposition, sustained differences between these leading to net erosion or accretion of coastal sediments (Wolanski and Elliott, 2016). These differences may be orders of magnitude less than the rates of suspension and deposition, and as such relatively small changes in hydrodynamic conditions, or in the suspended sediment load, can flip conditions between eroding and accreting (Winterwerp et al., 2020). The suspension of settled particulate matter occurs when the upward component of the drag forces exerted upon the particle surface exceeds gravity. As the strength of the drag forces depends on the flow speed and particle surface area, while gravity scales with particle volume, the flow required for suspension to occur tends to increase with particle size. As such, for any given hydrodynamic conditions, only a part of the sediment size spectrum will be suspended (McCave, 1984), but this fraction of the sediment load entrained within the flow may be deposited far from the source, once the drag forces have lessened sufficiently.
Clearly then the primary strategy for encouraging sedimentation is to maintain the bottom shear stresses exerted by the flow upon the sediment below the critical value that produces suspension for the local sediment size class. While this simply involves sheltering the coast from sources of hydrodynamic energy, the way to achieve this is site dependent, and will depend upon the source of the energy. In the coastal ocean the bottom flows effecting erosion are associated with waves or currents. The sediment control measures needed to produce a stable habitat depend upon the importance of each of these sources of coastal erosion.
Ocean waves typically intersect the coast at an angle close to perpendicular. As a result, when waves are the dominant source of hydrodynamic energy, barriers placed parallel to the coast that reflect or absorb the wave energy – such as breakwaters or sills – can be used to create sheltered conditions inshore that will be favorable to sedimentation. By reducing incident energy and promoting sedimentation, breakwaters have been shown to stabilize intertidal sediments (Currin et al., 2010; Scyphers et al., 2011), improve mudflat stability, and encourage salt marsh (Chowdhury et al., 2019) and seagrass development (Sharma et al., 2016). The artificial barriers themselves may be designed following WwN principles, such as artificial oyster breakwater reefs whose primary function remains coastal protection (Temmerman et al., 2013).
Ocean currents, on the other hand, largely flow tangent to the coastline. As a result, when currents are the predominant source of hydrodynamic energy, the construction of obstacles perpendicular to the shore is required to encourage sedimentation. The rock groynes ubiquitous on urban sandy coastlines are designed to retain locally suspended sand and interrupt along-shore sediment transport (Kraus et al., 1995). These littoral groynes are typically subject to persistent long-shore currents and produce strongly asymmetrical patterns of sedimentation. However, as seen below, an array of groynes subject to tidal flow can establish a relatively homogeneous zone of weak flow. Groynes are commonly associated with negative ecosystem impacts, both locally on the substrate (Dafforn et al., 2015) and far-field alterations to the sedimentation regime (Walker et al., 2008; Fanini et al., 2009). Groynes have been little used in the context of intertidal habitat creation, but examples are more common for terrestrial soft habitats, where protection from alongshore erosion has involved the use of solid structures that eventually become buried within the sediment (Nordstrom, 2014). The use of “resistant cores” of geotextiles, gabions or sand fences has been investigated for beach and dune stabilization (d’Angremond et al., 1993; Kraus and McDougal, 1996; Cooper and Lemckert, 2012). Once the solid defense structures become assimilated within the sediment matrix, many of the negative ecological consequences associated with introducing hard artificial substrates are removed (Nordstrom, 2014). The significant, and often problematic, distortion of the natural coastal sediment pathways associated with coastal groyne arrays vanishes if these can be designed to become and/or remain buried.
Permeable dams may combine both of these elements, being comprised of a network of parallel and perpendicular permeable barriers to flow, to create a series of partially enclosed “sedimentation basins” in which accretion is favored. A key characteristic of this technique is the barriers permeability, which acts to dissipate rather than reflect energy. As discussed earlier, interaction of waves and currents with solid rock constructions may result in near-field erosion (Fredsøe and Sumer, 2002). Permeable dams have proven to be a highly successful strategy to encourage natural sedimentation of eroded coastlines (Winterwerp et al., 2020). While in low energy environments they can be implemented relatively quickly and cheaply from lightweight materials, more energetic conditions may mandate rock construction, at significantly greater cost.
Finally, the hydrodynamic energy climate may be sufficiently benign to obviate additional structures, as has often been the case for the “living shoreline” approach (Bilkovic et al., 2016). Sufficiently low levels of wind and tidal energy generally occur only in narrow, microtidal estuaries. Alternatively, on coastline subject to higher energy but where secondary hard structures would be undesirable, the lost sediment may simply be replaced periodically, at high cost, through “shore nourishment” (Hamm et al., 2002; Paul, 2018).
While a consideration of the source and intensity of littoral bottom velocities guides the choice of sediment control structures, it should be borne in mind that the sediment dynamics contain significant uncertainty, and that this uncertainty should be incorporated within the project planning. The critical velocity for suspension is not only a function of particle size, but also depends in a complex way upon its electrochemical properties, the level of consolidation, and biological processes such as bioturbation (Wolanski and Elliott, 2016), and hence is best estimated empirically at the site. As a result, beneficial placement of dredged materials in the intertidal zone is often preceded with small-scale field trials (Bolam and Whomersley, 2003). Complex interactions between flow, sediment and coastal geometry are also possible, and as such the effect of the barriers on the coastal circulation and sediment transport pathways should be assessed. Groynes in the surf zone can enhance rip formation and the export of sand (Nielsen and Gordon, 2020), and create local seabed scouring (Winterwerp et al., 2013), while breakwaters for protection from wave energy may also induce erosional currents to form in their vicinity (Fredsøe and Sumer, 2002). When natural sedimentation is to be encouraged, the subsequent interruption of the existing sediment pathways must be assessed to ensure that problems of excessive erosion are not simply exported downstream. While such issues can be investigated numerically, monitoring and readiness to implement adjustments have been key characteristics of successful sediment control projects (Bilkovic et al., 2016).
The decision on how, and even whether, to intervene in the coastal sedimentation regime is a consequence of the sought ecological, functional and legal outcomes. The project goals should guide the choice of restoration species, and in turn, the need to alter the coastal elevation. Keystone, habitat forming species are an obvious target for restoration or novel ecosystem creation, and as such are commonly employed in ecoengineering projects of shoreline stabilization. These include reef-forming invertebrates such as corals (Ferrario et al., 2014), oysters (Scyphers et al., 2011), mussels and worms (Moody, 2012), intertidal vegetation such as mangroves and saltmarsh (Kumara et al., 2010; Gittman et al., 2014) or subtidal vegetation, such as seagrass or kelp (Dubi and Tørum, 1995; Ondiviela et al., 2014). The choice is based on which species provide the desired ecosystem services, and on whether their critical physiological and ecological requirements can reasonably be met (e.g., Ng et al., 2015; Ferrario et al., 2016; Perkol-Finkel et al., 2018). For the case of new intertidal sediment habitats of interest here, the target keystone species would typically be salt marsh, mangroves or seagrass. The living shoreline approach provides extensive documentation of salt marsh habitat creation (Bilkovic et al., 2016). In the following we concentrate instead on the key considerations for producing mangrove and seagrass habitats in the intertidal zone, summarised in Table 1.
Table 1. Summary of key considerations for creating new sediment substrates destined for mangrove and seagrass habitats.
While sustained mangrove loss worldwide has contributed to shoreline erosion and the loss of ecosystem services (Duarte et al., 2020), mangroves have increasingly been used for enhancing coastal protection against numerous hazards, such as cyclones and tsunamis. Hogarth (2015) reports that wind speeds can be reduced by more than half, ocean waves attenuated by up to 66%, and storm surges by 40–50 cm/km after passing through 100 m of mangrove forest. By damping the flow, mangroves encourage sedimentation, contributing to reduced turbidity, erosion prevention and the excessive degradation of coastlines (Schaffelke et al., 2005). There exist in excess of thirty published studies of mangrove rehabilitation projects, ten of which occurred within a coastal defense context (Morris et al., 2018). Mangrove rehabilitation and restoration projects tend to vary in the effort placed into plantation techniques (Chan, 1996; Matsui et al., 2012; Cheong et al., 2013; Lai et al., 2015; Mayer-Pinto et al., 2017; Chow, 2018) versus substrate preparation (Lewis, 2005; Lee et al., 2019; Lewis et al., 2019; Suman, 2019). The latter have achieved success by allowing recruitment to occur naturally, and simply ensuring that the habitat experiences natural tidal flushing and connectivity to a region with abundant propagule supply. Natural recruitment on suitably conditioned substrates has shown greater success than planted monocultures (Djamaluddin, 2008), and the multi-species, natural regeneration at Pasir Ris, has led to faster tree growth and biomass accumulation than at other mangrove rehabilitation sites in Singapore (Friess, 2017).
The ability of mangroves to tolerate extremely stressful abiotic conditions is due, in part, to key adaptations – such as salt-excreting glands, aerial or prop roots, and pneumatophores – and to intraspecific positive feedbacks – such as neighboring trees’ leaky roots oxygenating soils and buffering high sulfide concentrations (McKee et al., 1988). Mangroves generally exhibit a remarkable tolerance to a wide range of salinities, with optimal physiological function and growth of seedlings being possible for salinities from 3 to 27 ppm. For both congeneric (Ball and Pidsley, 1995) and sympatric (Cardona-Olarte et al., 2006) mangrove seedlings, growth rates are adversely affected beyond this range. Increasing salt tolerance occurs at the expense of higher nutritional demands, and results in lower maximal growth rates than occurs at low salinities (Ball, 1988, 2002). There is evidence that salinity fluctuations may provide a greater physiological stress for seedlings, than exposure to a higher, but constant, salinity level.
Mangroves demonstrate the capacity to successfully recruit to a wide variety of soil classes, from coarse sands to fine cohesive sediments (Krauss et al., 2008). Mangrove establishment, however, can be limited by some aspects of soil chemistry. High sediment sulfide concentrations may adversely affect both growth and survival of some mangrove seedlings at low light (Krauss et al., 2008). Low reduction potential and high salinity have been implicated in examples of poor mangrove regeneration success (Samson and Rollon, 2008). Partly, this is because most coastal wetland plants engineer the substrate to ameliorate harmful conditions, an effect that increases with wetland plant density (e.g., Howes et al., 1986; Gedan and Silliman, 2009; Aquino-Thomas and Proffitt, 2014). An important consequence of this from the restoration perspective is that seedlings are likely to exhibit positive, not negative, density dependence because of the facilitative effects of neighbors on ameliorating anoxic soil conditions.
Structural aspects of the substrate can also condition propagule establishment. Avicennia alba seedlings, for example, must be able to establish an initial root anchor during an inundation-free period that can withstand its own buoyancy and the drag forces experienced during the following tidal inundation (Balke et al., 2011). As such, for similar tidal flows, germination on cohesive muddy sediments may enhance seedling success rates compared to loose sand. More generally, substrate instability may impair the growth and natural succession of mangroves. Excess sediment accretion may also cause die-off and smothering, while too little sediment input can deprive mangroves of sufficient material to build soils in which to grow, or to direct erosion of the roots (Woodroffe et al., 2016).
More than the composition of the substrate, elevation has proven to be the most critical factor determining the success of mangrove restoration efforts. It is of primary importance that the tidal inundation time experienced by the mangrove seedlings lies within their physiological limits (Ellison, 2000; Lewis, 2005). The slow growth rate and high mortality of Rhizophora spp. seedlings observed in some mangrove restoration projects in the Philippines has been linked to their inappropriate placement in low intertidal mudflat and seagrass habitats (Primavera and Esteban, 2008).
Historically, mangrove rehabilitation projects have involved the planting of seedlings or saplings as monocultures, and there now exists substantial empirical evidence on the optimal planting techniques (Chan, 1996; Field, 1999; Matsui et al., 2012; Chow, 2018). Firstly, there is evidence to suggest that replanted, naturally sprouted, seedlings have higher rates of success than those sourced from plantations (Kamali and Hashim, 2011). Secondly, while seedlings have commonly been planted evenly spaced, based on the logic that this minimizes competition and maximizes yield, the underlying assumption of negative density dependence for juvenile mangroves – that they prefer to be spaced rather than clumped – may not be correct (Bakrin Sofawi et al., 2017). A restoration project using Rhizophora mucronata in Puttalam Lagoon found no evidence that increasing plant density evoked a trade-off with growth and survival of the planted trees (Kumara et al., 2010). On the contrary, the bare ground between seedlings can, in fact, hamper restoration by lowering the soil redox potential (Mossman et al., 2012). Denser clumping patterns, on the other hand, can promote sedimentation, share oxygen, reduce evaporation through shading, reduce predation, and promote colonization by other species (Kumara et al., 2010; Castellanos-Galindo et al., 2013), facilitating conspecifics as well as other associated species, and hence allowing for community development. Clumping of foundation species, such as oysters, mussels, and mangroves, is more common in stressful environments, where survivorship of individuals is higher in groups than when found alone. For instance, oxygen stress in mudflats is often alleviated through positive interactions among clumped marsh plants and mangrove saplings. Salt-marsh grasses and mangroves planted closely together benefit from oxygen leaking out of the roots of nearby plants (Howes et al., 1986; Gedan and Silliman, 2009) and plants in clumps can grow two to three times faster. Similarly, mangroves can reduce sedimentation and salinity stress for other mangroves or other foundation species such as oysters (Aquino-Thomas and Proffitt, 2014). Oyster reefs can, in turn, provide new habitat for mangroves to colonize, and augment the settlement of mangrove propagules (McClenachan et al., 2021). As such, by fostering beneficial interactions between neighboring conspecifics, the dense restoration of these intertidal keystone species can provide improved restoration success. More generally, purposefully incorporating positive interactions into designs of restoration projects and ecosystem recovery experiments could therefore increase community recovery and stability by orders of magnitude.
Another consideration is ensuring that the sediment is firm enough to minimize mixing and erosion in the surface layers (Balke et al., 2011). The typically vigorous physical mixing of the upper sediment layer on mudflats can be reduced when sediments are consolidated and cohesive. Unconsolidated sediments can be easily dislodged and resuspended. Balke et al. (2011) note that cohesive muddy sediments may give more support to the roots of mangroves than the loose sand used in their experiments, and that therefore the threshold for shoot length would be lower, and establishment of the seedlings possible in a shorter inundation free window.
Other factors influencing the establishment of mangroves includes the concentrations of sulfides in sediments which may adversely affect both growth and survival of mangrove seedlings at low light (Krauss et al., 2008). As such, when a sediment substrate is to be created from repurposed sediment, such as dredged sediment, treatment, mixing or blending may be required to lower sulfide concentrations following processes that are well established in coastal engineering (Burt, 1996).
Seagrass meadows deliver a range of ecosystem services from the provisioning, regulating and cultural categories (Barbier et al., 2011; Nordlund et al., 2017). They function as important nursery and foraging habitat for fish, shellfish (Jackson et al., 2001; de la Torre-Castro et al., 2008; Warren et al., 2010) and grazers (Ganter, 2000; Scott et al., 2020). They are also thought to oxygenate sediments, provide shoreline stabilization and protect from erosion (Koch et al., 2009), and are natural hotspots for carbon sequestration and nutrient cycling (Kennedy et al., 2010). Seagrasses are considered a foundation species, providing habitat and enhancing ecosystem biodiversity (Scott et al., 2020). They are also an important sentinel of system health, due to their sensitivity to both water quality and physical disturbances.
Whilst the conclusion from seagrass restoration efforts is that there is no “one solution fits all” approach, ecologically meaningful large-scale seagrass restoration is possible given enough scientific, community, and political support (Tan et al., 2020). Key components of successful seagrass restoration include understanding levels of connectivity between restoration locations and neighboring meadows to promote natural recovery, ensuring the complete life-cycle can occur within the restoration site, and assessing the genetic diversity of restored meadows and material used for restoration (Statton et al., 2018). How to ensure the return of ecological function (e.g., biogeochemical processes, trophic dynamics, nursery habitat) following seagrass restoration remains an outstanding research question. Emerging techniques showing high success rates include Buoy-Deployed Seeding (BuDS) (Pickerell et al., 2005), Dispenser Injection Seeding (Govers, 2018) and the use of nurseries (Tanner and Parham, 2010). Aquaculture of seagrass seeds, seedlings and plants is a promising source of planting units in restoration. The chances of transplant unit survival may be increased by artificial anchoring devices (Wendländer et al., 2019), or through positive biological interactions, such as improved water quality associated with coincident oyster reef restoration (Sharma et al., 2016).
The suitable conditions for seagrass growth are generally well established and include light availability (Duarte, 1991; Ralph et al., 2007), hydrodynamic environment (Fonseca and Kenworthy, 1987; Schanz and Asmus, 2003), substratum (Erftemeijer and Middelburg, 1993; van Katwijk and Wijgergangs, 2004), or nutrient availability (Touchette and Burkholder, 2000). Light for photosynthesis is a main requirement of seagrasses and therefore both water column transmissivity and depth will control the lower depth limit of seagrass (Dalla Via et al., 1998). Chartrand et al. (2016) arrived at a working light trigger value of 6 mol m–2.d–1 over a rolling 2-week average for the management of seagrasses. Physical, biological and chemical parameters that alter light availability (depth, storm events, epiphyte biomass, dissolved inorganic nitrogen and phosphorous, suspended chlorophyll concentration) are commonly listed as habitat requirements for seagrass colonization and growth. Such parameters have been used in predictive models of habitat suitability (Koch, 2001; Bos et al., 2005).
Whilst the lower depth limit for seagrass species tends to be determined by light availability – the ecological compensation depth – and predation (Schonbeck and Norton, 1980), it is the physiological tolerance to hydrodynamic and desiccation stresses that control the upper depth limit (Zaneveld, 1969; Schonbeck and Norton, 1978). Resuspension of sediments by wave energy can also strongly influence the light climate of the water column (Koch, 2001). High wave and current exposure can also reduce vegetative (rhizome) spreading, inhibit seedling colonization and decrease the accumulation of fine sediments and organic matter (Fonseca et al., 1983). Seagrass beds therefore tend to be in sheltered locations with limited fetch, or areas where long gently sloping shorelines dampen wave energy (Moore, 1963). A spontaneous unplanned establishment of a small seagrass meadow near a reclaimed shoreline behind a breakwater has been linked to reduced wave exposure (Yaakub et al., 2014).
While low flow does tend to improve light availability due to reduced self-shading and reduced water turbidity (Fonseca and Bell, 1998), overly low velocities may increase sediment sulfide concentrations (Koch, 1999), and sufficiently reduce the diffusive exchange of carbon and nutrients into the leaves of the plant as to limit photosynthesis (Jones et al., 1999). Low current velocities do convey some advantages, such as a reduction in self shading (leaves of plant more erect) and reduced sediment re-suspension and erosion, each contributing to greater light availability (Fonseca and Bell, 1998).
Seagrass growth may also be limited by the physical and geochemical processes associated with sediment type, and not by the grain size per se. For example, Barko and Smart (1983) suggest that the growth of seagrass is limited to sediments containing less than 5% organic matter. Grain size, wave exposure and current velocities will all influence the mobility of the sediment at a particular location (Soulsby, 1997). In a study by Collins et al. (2010), the sediment in unvegetated areas linked to anchoring and mooring disturbance were less cohesive, contained less organic material and had a lower silt fraction than surrounding habitats where seagrass was present.
Whilst seagrasses can maintain a positive carbon balance across a wide range of salinities, they do not thrive equally well at all salinities. Studies have shown that survival, growth and reproduction are impaired by extreme salinities (Agustí et al., 1994). For example, Nejrup and Pedersen (2008) found that the optimum salinity for Z. marina was between 10 and 25 ppm, in terms of shoot mortality and elongation rates. There are also interactions between salinity and other environmental factors. In higher salinity environments, seagrass requires sediments which are more oxygenated (coarser) and in which sulfide levels can be reduced via higher porewater advection rates (Fine and Tchernov, 2007).
Although debated, in general seagrasses growing in sandy or organic sediments are regarded as N-limited, and those in carbonate sediments as P-limited (Touchette and Burkholder, 2000). Kaldy (2009) identified that seagrass C:N:P was about 400:20:1, suggesting they are phosphorous limited, however, carbonate dissolution from seagrass organic acids may meet seagrass phosphorous requirements (Berkenhagen and Ebeling, 2010).
The proximity of the major multi-commodity Port of Gladstone, Australia, to the Great Barrier Reef World Heritage Area (Figure 1) provides unique operational constraints, including the requirement for land disposal of all capital dredge material. As is the case for most ports, Gladstone’s coast has been heavily modified from its natural state, including extensive reclamation of intertidal mud flats and clearing of mangrove-lined foreshores (Harris, 2009). As such, the Gladstone Port Corporation have demonstrated interest in using WwN concepts to achieve better environmental, social and economic outcomes within their reclamation and dredging activities. The possibility to use dredge material to create new habitat contiguous to the port’s Western Basin Reclamation Area (WBRA) is discussed here. The WBRA is located in the northern portion of the harbor (Figure 1), adjacent to the large intertidal mudflat of the Western Basin that contains sparse seagrass and mature multi-specific mangrove forest on its landward edge. Options are considered for creating a “living seawall” of new soft intertidal habitat adjacent to the western bund wall of the WBRA.
Figure 1. (A) The location of the Port of Gladstone, indicated by the box, relative to the Coral Sea, at the southern extent of the Great Barrier Reef World Heritage Area; (B) the location of the Western Bason Reclamation Area, whose western limit is considered for the “living seawall,” is shown within the Port of Gladstone.
The oblate geometry of the Port of Gladstone provides substantial protection of the foreshore from waves. The port is, however, macrotidal – dominantly semi-diurnal with a tidal range of 4.8 m – resulting in strong currents during flood and ebb exceeding 2 knots. The strong tidal currents, intense ship activity and input of fine sediment of terrestrial origin maintain the port’s waters turbid year-round (Conaghan, 1966). The creation of the reclamation area itself has decreased the fetch for the western shoreline, and hence further reduced incident wave energy. Increasing the distance for the tide to propagate into the southern portion of the Western Basin has, however, increased tidal currents, most significantly adjacent to the WBRA’s western bund wall. As such, the dominant source of erosional bottom stress for a novel sediment habitat located adjacent to the inner bund wall is tidal flow.
The risk of resuspension of placed sediment is greatest immediately following its placement. Even when consolidated sediments are placed, as for this example, a number of inundation cycles may be required for the consolidation of fine sediments. Over longer time-scales the vegetation itself will provide protection from erosion of the substrate. As outlined in section “Key Concepts for Restoration of Soft Intertidal Habitats,” various strategies exist for protecting the soft habitat from erosion during this initial phase. Continual nourishment can be ruled out on the bases of the resulting disturbance upon the establishment of the target ecosystem, the fact that it would equate to exporting dredge material back the harbor, and the expense. Given that wave energy upon this section of the bund wall is minimal, and that the tidal flow runs tangent to the bund wall, breakwaters placed parallel to the shore would not provide any protection from erosion. As such, an array of solid structures oriented perpendicular to the bund wall would be needed to create a low flow boundary layer that spans the width of the desired new habitat. The endemism of mangroves and seagrass makes them obvious first candidates to consider as the focus of restoration, as this assures a supply of natural propagules and implies broad suitability of the local environmental conditions. None-the-less, care is required to ensure that the physical and chemical conditions engineered of the novel sediment substrate are within the tolerances of the target species, as outlined in section “Key Concepts for Restoration of Soft Intertidal Habitats.” These are considered below. Given that the project has a purely conservation goal, with no coastal protection requirement, the choice of target species may consider the ecosystem services to be expected in each case, in addition to the likelihood of achieving a successful restoration.
Of the mangrove candidates for restoration, the highly successful colonizers Avicennia marina and Rhizophora stylosa are abundant within the Port of Gladstone, including the natural shore of the Western Basin (Saenger and Moverley, 1985; Houston et al., 2016). Both species produce abundant viviparous seeds capable of long-distance pelagic dispersal and able to anchor rapidly upon finding a suitable substrate. As a result, there is high likelihood that a suitably configured novel substrate could be rapidly colonized by A. marina and R. stylosa, without efforts in plantation or assisted recruitment. In either case – seagrass or mangrove restoration – measures to reduce tidal flows adjacent to the reclamation area bund wall are required to prevent sediment resuspension and improve the success rate of seedlings. The character of dredged sediments vary depending upon the source within the harbor, with capital dredge tending to be coarser, and maintenance spoils fine and cohesive (Wolanski and Elliott, 2016). While growth rates of seagrass and mangrove demonstrate some dependence on edaphic conditions as outlined above, both are observed to inhabit substrates from coarse sands to fine clays within the Port of Gladstone, providing some freedom to source the sediment to be repurposed for habitat creation. The larger grained sediment from capital dredge would be expected to show greater resistance to erosion, at the expense of possibly increased failure rate of seedlings due to dislodgement from the looser substrate. Nonetheless, the dependence of recruitment success upon sediment source would best be addressed empirically prior to a final decision.
The seagrass meadows in the Port of Gladstone and surroundings have been regularly mapped and monitored for over a decade (Thomas et al., 2010; Smith et al., 2021). The mono and multi-specific meadows of the species Halophila spinulosa, Halophila ovalis, Halophila decipiens, Halodule uninervis, and Zostera muelleri subsp. capricornii, span a range of environmental gradients (salinity, depth, wave and current exposure, turbidity) and vary in terms of configuration (patch size, species composition) and disturbance regime (including human pressures). Maximum Entropy (MaxEnt) modeling (Phillips and Dudík, 2008) has been used to infer the habitat dependencies of the local seagrass populations, and genetic techniques to infer population connectivity between local meadows (Jackson et al., 2020). The fact that Z. muelleri is present historically within the Western Basin makes it a logical candidate as a keystone species for the created habitat.
As such, more than the source of sediment, the elevation of the engineered substrate is the main factor that depends sensitively upon the choice of rehabilitation habitat. The reported maximum inundation times for A. marina and R. stylosa are approximately 400 min per day (Duke, 2006; Houston et al., 2016), which for Gladstone’s tidal regime provides a minimum elevation of the novel substrate of 70 cm above MSL. Local examples of both species are found commonly at this elevation throughout the Port of Gladstone region, and occasionally on substrates as low as 50 cm above sea level. On the other hand, the optimal depth for the target intertidal seagrass Z. marina is set by light availability, desiccation and hydrodynamic stress. Within the Port of Gladstone these conditions are generally met from depths of 80 cm above MSL. The hydrodynamic stress is unlikely to be limiting, given that Zostera (though notably for Zostera marina) have been observed to withstand velocities up to 180 cm.s–1 (Koch, 2001), which is well above the level at which significant sediment resuspension would occur.
For either target habitat, without secondary defenses the sediment placed up to the required elevation adjacent to the seawall risks rapid resuspension by tidal stress. The optimal geometry for the array of structures required to protect the placed sediments within this setting was investigated using numerical modeling, the technical details of which are provided as Supplementary Material. Designs were sought that prevent erosion across the area to be restored at all substrate heights and phases of the tide. Such a constraint is necessary when natural sedimentation is desired, and in the case of placed sediments suggests that recovery should occur following events of erosion. Thus, despite the significant difference in substrate elevation required for mangrove and seagrass habitats, the choice between these two habitats does not influence the geometry of the network of secondary sediment control structures. As peak tidal currents occur at mid-tide, the need to ensure that the flow control structures extend sufficiently above MSL to avoid being overtopped during periods of strong flow is a design constrain that is common to both habitat choices. The solid structures are required to withstand not only the substantial hydraulic stresses exerted by the peak tidal flows, in particular at their seaward extent, but also unbalanced static loadings during the placement of sediments, suggesting the use of solid structural elements such as rock groynes.
Groynes generally produce a region of reduced tangential flow for a distance downstream that exceeds their length (Kraus et al., 1995). For regulatory reasons the maximum groyne extension from the seawall is limited to 20 m. Given the desirability of minimizing the number and height of the groynes, the numerical modeling focused upon determining the minimum spacing and minimum height of 20 m long groynes required to establish a permanent continuous accreting boundary layer adjacent to the seawall when exposed to tidal flows. In the absence of groynes, simulated peak tidal flows along the face of the bund wall exceeded 1.2 m.s–1 (Figure 2). By simulating the inclusion groynes of varying spacing and height, it was found that an inter-groyne spacing of 150 m, and a height of 70 cm above MSL, was sufficient to establish the desired low flow conditions (Figure 2). With such a design for the groyne array, repurposed sediment added between the groynes would be expected to resist erosion by tidal currents, and hence allow the target species to establish, independently of the target substrate elevation. In the case of mangrove habitat, the rock groynes would be completely assimilated within the placed sediment, and subsequent natural accretion would allow them to eventually be incorporated within the mangrove ecosystem. For seagrass, however, the groynes of this height would extend 1.5 m above the habitat substrate. This would not be expected to have a negative impact upon the seagrass, that have been reported to thrive in the lee of breakwaters (Yaakub et al., 2014), and the exposed groyne surface can be designed following well established ecological engineering principles, to encourage oyster recruitment (Scyphers et al., 2011).
Figure 2. The maximum tidal speeds (in units of m.s− 1) of the numerical simulations of tidal flow adjacent to a section of the western bund wall of the Western Basin Reclamation Area are shown without (left) and with (right) the inclusion of a series of subtidal groynes. The series of groynes reduce the maximum tidal bottom stresses sufficiently to guarantee that sediments placed for the purpose of habitat creation are not resuspended, while still being sufficiently low that, in the case of a mangrove habitat, the groynes would become assimilated within the sediment.
The numerical modeling considers the interaction of the tidal flow with the array of groynes, but not the non-linear interactions between flow and sediment. Changes in the bottom depth due to accretion/erosion will, in turn, change the local patterns of flow. In nature, this process leads to the formation of tidal channels that may be highly dynamic, and the formation of such a tidal channel abutting the groynes is likely to occur. When the space between groynes is entirely filled with repurposed sediment, as would occur for mangrove habitat, erosion due to primary tidal flows is prevented, as inundation of the substrate occurs only during weak flows at high tide. The formation of erosional channels in the sediment driven by tidal percolation of the receding tide is, nonetheless, possible and may be intensified adjacent to the groynes. While such processes can be simulated numerically with coupled sediment-hydrodynamic models, empirical studies and monitoring remain essential to address problems of excessive secondary erosion as they arise.
The rehabilitation or creation of coastal habitats through reuse or accretion of sediments is likely to continue apace, driven by mainstream recognition of the urgent need to recover the services provided by the coastal marine ecosystem. Although land reclamation has been practiced for millennia with socio-economic aims (Winterwerp et al., 2020), over previous decades new strategies have been developed with an ecosystem focus. Ecological engineering approaches for protection of eroded coastlines or coastal infrastructure commonly seek to facilitate the virtuous cycle between sedimentation and vegetation in the intertidal zone. However, “soft” substrates may also be created by the placement of waste sediments, such as dredge spoils. While the focus shifts from encouraging natural sedimentation to preventing erosion of the placed sediment, the sediment control techniques are common. In all but protected coastlines, some matrix of hard structures may be required, at least transiently, to guarantee consolidation of the substrate and its ecosystem.
The choice of which hard elements to use within a novel intertidal sediment habitat requires careful consideration of the source of erosional energy. Where coastal erosion is associated with wave energy, correctly configured barriers placed parallel to the coast can ensure protected, accretion-favorable conditions in their lee. In sufficiently low energy conditions these barriers may be lightweight, and eventually assimilated completely within the new substrate. Otherwise, when solid constructions such as breakwaters are required, these can be designed to achieve other conservation outcomes, such as by encouraging oyster recruitment.
But when the main source of incident energy is due to currents, such as may happen within macrotidal estuaries, elements perpendicular to the coast are typically needed to prevent coastal erosion during periods of inundation. Groyne-type structures have been little used in the context of habitat creation, presumably due to the fact that wave action is more often the limiting factor for coastal sedimentation, but also due to the negative ecological consequences that have often derived from the introduction of hard, reflective structures in the coastal zone. However, when successful restoration hinges on substrate stability, the use of groynes within the sediment matrix may be indicated.
While these considerations should guide the geometry and construction of sediment control structures for novel soft habitats, the potential complexities of the interactions between sediment, flow, and coastline, counsel for prior empirical research and posterior monitoring and correction activities to be budgeted for. For placed sediments, monitoring is especially necessary during the initial phase of sediment consolidation. Structures placed to dissipate hydrodynamic energy may concentrate part of that energy into currents, creating localized erosion. The readjustment of currents and sediment loads due to the introduction of obstacles to the flow may also result in a non-beneficial change in the patterns of sediment transport. Even when hard elements are buried immediately within placed sediment, erosional gullies may form at their interface with the sediment.
The location and type of substrate are, of course, ultimately determined by the target restoration ecosystem. We have reviewed the key physiological and ecological considerations for two common intertidal sediment biomes – mangroves and seagrass. Project success hinges not simply upon correctly identifying the needed minimal environmental conditions for the chosen keystone species, but upon the ability to implement measures to ensure these. The latter implies a significant effort in monitoring to ensure that the implemented strategy is proving the minimal requirements of the target species.
In the case considered of the construction of a “living seawall” using dredge spoils to create mangrove and seagrass habitat adjacent to a bund wall within the Port of Gladstone, low wave energy and strong tidal flows argue for the use of rock groynes to ensure that placed recycled sediments resist erosion during inundation. Even though the elevation of the novel substrate would vary by 1.5 m depending on whether the target biome is seagrass or mangrove, the design of the sediment control structures would be largely the same in either case, involving groynes that extend to approximately 70 cm above MSL to shield the novel substrate from peak tidal flows on both ebb and flows.
This example also highlights the opportunity to engage industrial users of the coastal zone in conservation efforts. By reconciling ecological goals with economic realities, WwN and related approaches are providing tools to achieve coastal rehabilitation at scale. There is now sufficient practical experience to have confidence that the dredge material produced as an unavoidable consequence of modern port operation can be repurposed to achieve positive outcomes for ecosystem services in the intertidal, even without a coastal defense objective.
CA lead the redaction of the manuscript and performed the numerical modeling. RM performed the literature review. GD contributed to the living seawall designs. EJ lead and coordinated the research and co-wrote the manuscript. All authors contributed to the article and approved the submitted version.
This work was supported by working with Nature Investigations for Seawall Designs in the Port of Gladstone – Gladstone Ports Corporation OS19308956.
The authors declare that this study received funding from the Gladstone Ports Corporation, an Australian Government Owned Corporation. The funder provided the technical details for the case study, and is the employer of author GD.
The remaining authors declare that the research was conducted in the absence of any commercial or financial relationships that could be construed as a potential conflict of interest.
All claims expressed in this article are solely those of the authors and do not necessarily represent those of their affiliated organizations, or those of the publisher, the editors and the reviewers. Any product that may be evaluated in this article, or claim that may be made by its manufacturer, is not guaranteed or endorsed by the publisher.
The authors would like to thank Chris Gillies from The Nature Conservancy, and Judith Wake and Wayne Houston from CQUniversity for contributing to discussions on feasibility and habitat requirements.
The Supplementary Material for this article can be found online at: https://www.frontiersin.org/articles/10.3389/fevo.2021.682349/full#supplementary-material
Abelson, A., Halpern, B. S., Reed, D. C., Orth, R. J., Kendrick, G. A., Beck, M. W., et al. (2016). Upgrading marine ecosystem restoration using ecological-social concepts. BioScience 66, 156–163. doi: 10.1093/biosci/biv171
Agustí, S., Enríquez, S., Frost-Christensen, H., Sand-Jensen, K., and Duarte, C. M. (1994). Light harvesting among photosynthetic organisms. Funct. Ecol. 8, 273–279. doi: 10.2307/2389911
Aquino-Thomas, J., and Proffitt, C. E. (2014). Oysters Crassostrea virginica on red mangrove Rhizophora mangle prop roots: facilitation of one foundation species by another. Mar. Ecol. Prog. Series 503, 177–194. doi: 10.3354/meps10742
Bakrin Sofawi, A., Rozainah, M. Z., Normaniza, O., and Roslan, H. (2017). Mangrove rehabilitation on Carey Island, Malaysia: an evaluation of replanting techniques and sediment properties. Mar. Biol. Res. 13, 390–401. doi: 10.1080/17451000.2016.1267365
Balke, T., Bouma, T. J., Horstman, E. M., Webb, E. L., Erftemeijer, P. L., and Herman, P. M. (2011). Windows of opportunity: thresholds to mangrove seedling establishment on tidal flats. Mar. Ecol. Prog. Series 440, 1–9. doi: 10.3354/meps09364
Ball, M., and Pidsley, S. (1995). Growth responses to salinity in relation to distribution of two mangrove species, Sonneratia alba and lanceolata, S., in northern Australia. Funct. Ecol. 9, 77–85. doi: 10.2307/2390093
Ball, M. C. (1988). Salinity tolerance in the mangroves Aegiceras corniculatum and Avicennia marina. I. water use in relation to growth, carbon partitioning, and salt balance. Funct. Plant Biol. 15, 447–464. doi: 10.1071/pp9880447
Ball, M. C. (2002). Interactive effects of salinity and irradiance on growth: implications for mangrove forest structure along salinity gradients. Trees 16, 126–139. doi: 10.1007/s00468-002-0169-3
Baptist, M. J., Gerkema, T., Van Prooijen, B. C., Van Maren, D. S., Van Regteren, M., Schulz, K., et al. (2019). Beneficial use of dredged sediment to enhance salt marsh development by applying a ‘Mud Motor’. Ecol. Eng. 127, 312–323. doi: 10.1016/j.ecoleng.2018.11.019
Barbier, E. B., Hacker, S. D., Kennedy, C., Koch, E. W., Stier, A. C., and Silliman, B. R. (2011). The value of estuarine and coastal ecosystem services. Ecol. Monographs 81, 169–193.
Barko, J., and Smart, R. (1983). Effects of organic matter additions to sediment on the growth of aquatic plants. J. Ecol. 71, 161–175. doi: 10.2307/2259969
Berkenhagen, J., and Ebeling, M. W. (2010). Links between fishery and the work of johann heinrich von thünen, eponym of the newly founded federal research institute. J. Appl. Ichthyol. 26, 14–18. doi: 10.1111/j.1439-0426.2010.01442.x
Bilkovic, D. M., Mitchell, M., Mason, P., and Duhring, K. (2016). The role of living shorelines as estuarine habitat conservation strategies. Coastal Manag. 44, 161–174. doi: 10.1080/08920753.2016.1160201
Bolam, S., and Whomersley, P. (2003). Invertebrate recolonization of fine-grained beneficial use schemes: an example from the southeast coast of England. J. Coastal Conserv. 9, 159–169. doi: 10.1652/1400-0350(2003)009[0159:irofbu]2.0.co;2
Bos, A. R., Dankers, N., Groeneweg, A. H., Hermus, D. C. R., Jager, Z., de Jong, D. J., et al. (2005). Eelgrass (Zostera marina L.) in the Western Wadden Sea: Monitoring, Habitat Suitability Model, Transplantations and Communication. San Francisco, CA: VLIZ Special Publication.
Browne, M. A., and Chapman, M. G. (2014). Mitigating against the loss of species by adding artificial intertidal pools to existing seawalls. Mar. Ecol. Prog. Series 497, 119–129. doi: 10.3354/meps10596
Bulleri, F., and Airoldi, L. (2005). Artificial marine structures facilitate the spread of a non-indigenous green alga, Codium fragile ssp. tomentosoides, in the north Adriatic Sea. J. Appl. Ecol. 42, 1063–1072. doi: 10.1111/j.1365-2664.2005.01096.x
Burt, T. (1996). Guidelines for the Beneficial use of Dredged Material. SR 488. Oxon: HR Wallingford.
Cardona-Olarte, P., Twilley, R. R., Krauss, K. W., and Rivera-Monroy, V. (2006). Responses of neotropical mangrove seedlings grown in monoculture and mixed culture under treatments of hydroperiod and salinity. Hydrobiologia 569, 325–341. doi: 10.1007/s10750-006-0140-1
Castellanos-Galindo, G. A., Krumme, U., Rubio, E. A., and Saint-Paul, U. (2013). Spatial variability of mangrove fish assemblage composition in the tropical eastern Pacific Ocean. Rev. Fish Biol. Fish. 23, 69–86. doi: 10.1007/s11160-012-9276-4
Chan, H. (1996). Mangrove Reforestation in Peninsular Malaysia: a Case Study of Matang. Restoration of Mangrove Ecosystems. Japan: International Society for Mangrove Ecosystems, Okinawa.
Chapman, M. G. (2003). Paucity of mobile species on constructed seawalls: effects of urbanization on biodiversity. Mar. Ecol. Prog. Series 264, 21–29. doi: 10.3354/meps264021
Chapman, M. G., and Bulleri, F. (2003). Intertidal seawalls—new features of landscape in intertidal environments. Landscape Urban Plan. 62, 159–172. doi: 10.1016/s0169-2046(02)00148-2
Chartrand, K. M., Bryant, C. V., Carter, A. B., Ralph, P. J., and Rasheed, M. A. (2016). Light thresholds to prevent dredging impacts on the great barrier reef seagrass, Zostera muelleri ssp. capricorni. Front. Mar. Sci. 3:106. doi: 10.3389/fmars.2016.00106
Cheong, S.-M., Silliman, B., Wong, P. P., Van Wesenbeeck, B., Kim, C.-K., and Guannel, G. J. N. (2013). Coastal adaptation with ecological engineering. Nat. Climate Change 3, 787–791. doi: 10.1038/nclimate1854
Chow, J. (2018). Mangrove management for climate change adaptation and sustainable development in coastal zones. J. Sustainable Forestry 37, 139–156. doi: 10.1080/10549811.2017.1339615
Chowdhury, M. S. N., Wijsman, J. W. M., Hossain, M. S., Ysebaert, T., and Smaal, A. C. (2019). A verified habitat suitability model for the intertidal rock oyster, Saccostrea cucullata. PLoS One 14:e0217688. doi: 10.1371/journal.pone.0217688
Collins, K. J., Suonpää, A. M., and Mallinson, J. J. (2010). The impacts of anchoring and mooring in seagrass, Studland Bay, Dorset, UK. Underwater Technol. Int. J. Soc. Underwater 29, 117–123. doi: 10.3723/ut.29.117
Conaghan, P. J. (1966). Sediments and Sedimentary Processes in Gladstone Harbour, Queensland. Brisbane: University of Queensland Press.
Cooper, J. A. G., and Lemckert, C. (2012). Extreme sea-level rise and adaptation options for coastal resort cities: a qualitative assessment from the Gold Coast, Australia. Ocean Coastal Manag. 64, 1–14. doi: 10.1016/j.ocecoaman.2012.04.001
Currin, C. A., Chappell, W. S., and Deaton, A. (2010). “Developing alternative shoreline armoring strategies: the living shoreline approach in North Carolina,” in Proceedings of the Puget Sound Shorelines and the Impacts of Armoring—Proceedings of a State of the Science Workshop, May 2009, eds H. Shipman, M. N. Dethier, G. Gelfenbaum, K. L. Fresh, and R. S. Dinicola. (Virginia, VA: U.S. Geological Survey).
Cziesielski, M. J., Duarte, C. M., Aalismail, N., Al-Hafedh, Y., Anton, A., Baalkhuyur, F., et al. (2021). Investing in blue natural capital to secure a future for the red sea ecosystems. Front. Mar. Sci. 7:603722. doi: 10.3389/fmars.2020.603722
Dafforn, K. A., Glasby, T. M., Airoldi, L., Rivero, N. K., Mayer-Pinto, M., and Johnston, E. L. (2015). Marine urbanization: an ecological framework for designing multifunctional artificial structures. Front. Ecol. Environ. 13:82–90. doi: 10.1890/140050
Dalla Via, J., Sturmbauer, C., Schonweger, G., Sotz, E., Mathekowitsch, S., Stifter, M., et al. (1998). Light gradients and meadow structure in Posidonia oceanica: ecomorphological and functional correlates. Mar. Ecology-Prog. Series 163, 267–278. doi: 10.3354/meps171267
d’Angremond, K., van den Berg, E. J., and de Jager, J. H. (1993). Use and behavior of gabions in coastal protection. Coastal Eng. 1, 1748–1757.
Das, S., and Vincent, J. R. (2009). Mangroves protected villages and reduced death toll during Indian super cyclone. Proc. Natl. Acad. Sci. U S A. 106, 7357–7360. doi: 10.1073/pnas.0810440106
de la Torre-Castro, M., Björk, M., Eklöf, J., and Rönnbäck, P. (2008). Seagrass importance in food provisioning services: fish stomach content as a link between seagrass meadows and local fisheries. Western Indian Ocean J. Mar. Sci. 7, 95–110.
Djamaluddin, R. (2008). Completion of the Project on Cost-effective Mangrove Rehabilitation Focusing on Restoration of Hydrology. Project report. London: The Rufford Small Grants Foundation.
Duarte, C. M. (1991). Allometric scaling of seagrass form and productivity. Mar. Ecol. Prog. Series Oldendorf 77, 289–300. doi: 10.3354/meps077289
Duarte, C. M., Agusti, S., Barbier, E., Britten, G. L., Castilla, J. C., et al. (2020). Rebuilding marine life. Nature 580, 39–51. doi: 10.1038/s41586-020-2146-7
Duke, N. C. (2006). Australia’s Mangroves: the Authoritative Guide to Australia’s Mangrove Plants. Brisbane, QLD: University of Queensland
Ellison, A. M. (2000). Mangrove restoration: do we know enough? Restoration Ecol. 8, 219–229. doi: 10.1046/j.1526-100x.2000.80033.x
Erftemeijer, P. L., and Middelburg, J. J. (1993). Sediment-nutrient interactions in tropical seagrass beds: a comparison between a terrigenous and a carbonate sedimentary environment in South Sulawesi (Indonesia). Mar. Ecol. Prog. Series 102, 187–198. doi: 10.3354/meps095187
Fanini, L., Marchetti, G. M., Scapini, F., and Defeo, O. (2009). Effects of beach nourishment and groynes building on population and community descriptors of mobile arthropodofauna. Ecol. Indicators 9, 167–178. doi: 10.1016/j.ecolind.2008.03.004
Ferrario, F., Beck, M. W., Mayer Pinto, C., Storlazzi, D., Micheli, F., Shepard, C. C., et al. (2014). The effectiveness of coral reefs for coastal hazard risk reduction and adaptation. Bioscience 5, 1–9. doi: 10.1007/978-94-007-3008-3_1
Ferrario, F., Iveša, L., Jaklin, A., Perkol-Finkel, S., and Airoldi, L. (2016). The overlooked role of biotic factors in controlli, the ecological performance of artificial marine habitats. J. Appl. Ecol. 53, 16–24. doi: 10.1111/1365-2664.12533
Field, C. (1999). Rehabilitation of mangrove ecosystems: an overview. Mar. Pollut. Bull. 37, 383–392. doi: 10.1016/s0025-326x(99)00106-x
Fine, M., and Tchernov, D. (2007). Scleractinian coral species survive and recover from decalcification. Science 315:1811. doi: 10.1126/science.1137094
Firth, L. B., Browne, K. A., Knights, A. M., Hawkins, S. J., and Nash, R. (2016). Eco-engineered rock pools: a concrete solution to biodiversity loss and urban sprawl in the marine environment. Environ. Res. Lett. 11:094015. doi: 10.1088/1748-9326/11/9/094015
Fonseca, M. S., and Bell, S. S. (1998). Influence of physical setting on seagrass landscapes near Beaufort, North Carolina, USA. Mar. Ecol. Prog. Series 171, 109–121. doi: 10.3354/meps171109
Fonseca, M. S., and Kenworthy, W. J. (1987). Effects of current on photosynthesis and distribution of seagrasses. Aquatic Botany 27, 59–78. doi: 10.1016/0304-3770(87)90086-6
Fonseca, M. S., Zieman, J. C., Thayer, G. W., and Fisher, J. S. (1983). The role of current velocity in structuring seagrass meadows. Estuarine Coastal Shelf Sci. 17, 367–380. doi: 10.1016/0272-7714(83)90123-3
Fredsøe, J., and Sumer, B. M. (2002). The Mechanics of Scour in the Marine Environment. Singapore: World Scientific Publishing Company.
Friess, D. A. (2017). Mangrove rehabilitation along urban coastlines: a Singapore case study. Regional Stud. Mar. Sci. 16, 279–289. doi: 10.1016/j.rsma.2017.09.013
Gann, G. D., Mcdonald, T., Walder, B., Aronson, J., Nelson, C. R., Jonson, J., et al. (2019). International principles and standards for the practice of ecological restoration. Restoration Ecol. 27, S1–S46.
Ganter, B. (2000). Seagrass (Zostera spp.) as food for brent geese (Branta bernicla): an overview. Helgoland Mar. Res. 54, 63–70. doi: 10.1007/s101520050003
Garbisch, E. W., and Garbisch, J. L. (1994). Control of upland bank erosion through tidal marsh construction on restored shores: application in the Maryland portion of Chesapeake Bay. Environ. Manag. 18, 677–691. doi: 10.1007/bf02394633
Gedan, K. B., and Silliman, B. R. (2009). Using facilitation theory to enhance mangrove restoration. AMBIO: J. Hum. Environ. 38, 109–109. doi: 10.1579/0044-7447-38.2.109
Gittman, R. K., Popowich, A. M., Bruno, J. F., and Peterson, C. H. (2014). Marshes with and without sills protect estuarine shorelines from erosion better than bulkheads during a Category 1 hurricane. Ocean Coastal Manag. 102, 94–102. doi: 10.1016/j.ocecoaman.2014.09.016
Glasby, T. M., Connell, S. D., Holloway, M. G., and Hewitt, C. L. (2007). Nonindigenous biota on artificial structures: could habitat creation facilitate biological invasions? Mar. Biol. 151, 887–895. doi: 10.1007/s00227-006-0552-5
Govers, L. L. (2018). “Set-backs and successes: seagrass restoration in the Dutch Wadden Sea,” in Paper Presented to World Seagrass Conference (Singapore).
Hale, L. Z., Meliane, I., Davidson, S., Sandwith, T., Beck, M., Hoekstra, J., et al. (2009). Ecosystem-based adaptation in marine and coastal ecosystems. Renewable Resources J. 25, 21–28.
Hamm, L., Capobianco, M., Dette, H. H., Lechuga, A., Spanhoff, R., et al. (2002). A summary of European experience with shore nourishment. Coastal Eng. 47, 237–264. doi: 10.1016/s0378-3839(02)00127-8
Harris, J. (2009). The Vanished Mud Flats Around Gladstone: Reflecting on Events and Happenings on Gladstone’s Mud Flats before the Industrial Development. Gladstone, QLD: Gladstone Regional Council.
Hobbs, R. J., Higgs, E. S., and Hall, C. M. (2013). Novel ecosystems: Intervening in the New Ecological World Order. Hoboken, NJ: Wiley Blackwell.
Houston, W., Charlesworth, D., and Black, L. (2016). Mangrove Condition in Relation to Reference Sites at Fisherman’s Landing, Port Curtis, Spring 2016: Report to Gladstone Ports Corporation. Rockhampton: School of Medical and Applied Sciences, CQUniversity.
Howes, B., Dacey, J., and Goehringer, D. (1986). Factors controlling the growth form of Spartina alterniflora: feedbacks between above-ground production, sediment oxidation, nitrogen and salinity. J. Ecol. 74, 881–898. doi: 10.2307/2260404
Jackson, E. L., Rowden, A. A., Attrill, M. J., Bossey, S. J., and Jones, M. B. (2001). The importance of seagrass beds as a habitat for fishery species. Oceanography Mar. Biol. Annual Rev. 39, 269–304.
Jackson, E. L., Smith, T. M., York, P. H., Nielsen, J., Irving, A. D., and Sherman, C. D. (2020). An assessment of the seascape genetic structure and hydrodynamic connectivity for subtropical seagrass restoration. Restoration Ecol. 29, 1–11. doi: 10.1016/j.ecolind.2017.12.029
Jones, J. I., Young, J. O., Haynes, G. M., Moss, B., Eaton, J. W., and Hardwick, K. J. (1999). Do submerged aquatic plants influence their periphyton to enhance the growth and reproduction of invertebrate mutualists? Oecologia 120, 463–474. doi: 10.1007/s004420050879
Kaldy, J. E. (2009). “Water column and sediment nutrients as limits to growth of Zostera marina and Thalassia testudinum,” in Seagrasses and Protective Criteria: A Review and Assessment of Research Status, ed. W. G. Nelson (Oregon, OR: United States Environmental Protection Agency, Newport).
Kamali, B., and Hashim, R. (2011). Mangrove restoration without planting. Ecol. Eng. 37, 387–391. doi: 10.1016/j.ecoleng.2010.11.025
Kennedy, H., Beggins, J., Duarte, C. M., Fourquean, J. W., Holmer, M., Marbá, N., et al. (2010). Seagrass sediments as a global carbon sink: isotopic constraints. Global Biogeochem. Cycles 24, 1–8.
Koch, E. (2001). Beyond light: Physical, geological, and geochemical parameters as possible submersed aquatic vegetation habitat requirements. Estuaries Coasts 24, 1–17. doi: 10.2307/1352808
Koch, E. W. (1999). Sediment resuspension in a shallow Thalassia testudinum banks ex König bed. Aquatic Botany 65, 269–280. doi: 10.1016/s0304-3770(99)00045-5
Koch, E. W., Barbier, E. B., Silliman, B. R., Reed, D. J., Perillo, G. M. E., et al. (2009). Non-linearity in ecosystem services: temporal and spatial variability in coastal protection. Front. Ecol. Environ. 7:29–37. doi: 10.1890/080126
Kraus, N. C., Hanson, H., and Blomgren, S. H. (1995). Modern functional design of groin systems. Coastal Eng. 1, 1327–1342.
Kraus, N. C., and McDougal, W. G. (1996). The effects of seawalls on the beach: Part I, an updated literature review. J. Coastal Res. 12, 691–701.
Krauss, K. W., Lovelock, C. E., McKee, K. L., López-Hoffman, L., Ewe, S. M., and Sousa, W. P. (2008). Environmental drivers in mangrove establishment and early development: a review. Aquatic Botany 89, 105–127. doi: 10.1016/j.aquabot.2007.12.014
Kumara, M. P., Jayatissa, L. P., Krauss, K. W., Phillips, D. H., and Huxham, M. (2010). High mangrove density enhances surface accretion, surface elevation change, and tree survival in coastal areas susceptible to sea-level rise. Oecologia 164, 545–553. doi: 10.1007/s00442-010-1705-2
Lai, S., Loke, L. H., Hilton, M. J., Bouma, T. J., and Todd, P. A. (2015). The effects of urbanisation on coastal habitats and the potential for ecological engineering: a Singapore case study. Ocean Coastal Manag. 103, 78–85. doi: 10.1016/j.ocecoaman.2014.11.006
Lee, S. Y., Hamilton, S., Barbier, E. B., Primavera, J., and Lewis, R. R. (2019). Better restoration policies are needed to conserve mangrove ecosystems. Nat. Ecol. Evol. 3, 870–872. doi: 10.1038/s41559-019-0861-y
Lewis, R. R. III, Brown, B. M., and Flynn, L. L. (2019). Methods and Criteria for Successful Mangrove Forest Rehabilitation. Coastal Wetlands. Amsterdam: Elsevier.
Lewis, R. R. (2005). Ecological engineering for successful management and restoration of mangrove forests. Ecol. Eng. 24, 403–418. doi: 10.1016/j.ecoleng.2004.10.003
Lotze, H., Lenihan, H. S., Bourque, B. J., Bradbury, R. H., Cooke, R., and Kay, M. C. (2006). Depletion, degradation, and recovery potential of estuaries and coastal seas. Science 312, 1806–1809. doi: 10.1126/science.1128035
Matsui, N., Morimune, K., Meepol, W., and Chukwamdee, J. J. F. (2012). Ten year evaluation of carbon stock in mangrove plantation reforested from an abandoned shrimp pond. Forests 3, 431–444. doi: 10.3390/f3020431
Mayer-Pinto, M., Johnston, E. L., Bugnot, A. B., Glasby, T. M., Airoldi, L., Mitchell, A., et al. (2017). Building ‘blue’: an eco-engineering framework for foreshore developments. J. Environ. Manage. 189, 109–114. doi: 10.1016/j.jenvman.2016.12.039
McCave, I. N. (1984). Erosion, transport and deposition of fine-grained marine sediments. Geol. Soc. London Special Publications 15, 35–69. doi: 10.1144/gsl.sp.1984.015.01.03
McClenachan, G., Witt, M., and Walters, L. J. (2021). Replacement of oyster reefs by mangroves: unexpected climate-driven ecosystem shifts. Global Change Biol. 27, 1226–1238. doi: 10.1111/gcb.15494
McKee, K. L., Mendelssohn, I. A., and Hester, M. W. (1988). Reexamination of pore water sulfide concentrations and redox potentials near the aerial roots of Rhizophora mangle and Avicennia germinans. Am. J. Botany 75, 1352–1359. doi: 10.2307/2444458
Miller, J. R., and Bestelmeyer, B. T. (2016). What’s wrong with novel ecosystems, really? Restoration Ecol. 24, 577–582. doi: 10.1111/rec.12378
Mitsch, W. J., and Jørgensen, S. E. (2003). Ecological engineering: a field whose time has come. Ecol. Eng. 20, 363–377. doi: 10.1016/j.ecoleng.2003.05.001
Moody, J. A. (2012). The Relationship between the Ribbed Mussel (Geukensia demissa) and Salt Marsh Shoreline Erosion. New Brunswick, NJ: Rutgers The State University of New Jersey-New Brunswick.
Moore, D. R. (1963). Distribution of the seagrass Thalassia in the United States. Bull. Mar. Sci. 13, 329–342.
Morris, R. L., Konlechner, T. M., Ghisalberti, M., and Swearer, S. E. (2018). From grey to green: efficacy of eco-engineering solutions for nature-based coastal defence. Global Change Biol. 24, 1827–1842. doi: 10.1111/gcb.14063
Mossman, H. L., Brown, M. J., Davy, A. J., and Grant, A. (2012). Constraints on salt marsh development following managed coastal realignment: dispersal limitation or environmental tolerance? Restoration Ecol. 20, 65–75. doi: 10.1111/j.1526-100x.2010.00745.x
Narayan, S., Beck, M. W., Reguero, B. G., Losada, I. J., Van Wesenbeeck, B., Pontee, N., et al. (2016). The effectiveness, costs and coastal protection benefits of natural and nature-based defences. PLoS One 11:e0154735. doi: 10.1371/journal.pone.0154735
Nejrup, L. B., and Pedersen, M. F. (2008). Effects of salinity and water temperature on the ecological performance of Zostera marina. Aquatic Botany 88, 239–246. doi: 10.1016/j.aquabot.2007.10.006
Ng, C. S. L., Lim, S. C., Ong, J. Y., Teo, L. M. S., Chou, L. M., et al. (2015). Enhancing the biodiversity of coastal defence structures: transplantation of nursery-reared reef biota onto intertidal seawalls. Ecol. Eng. 82, 480–486. doi: 10.1016/j.ecoleng.2015.05.016
Nielsen, L., and Gordon, A. (2020). Submerged groynes for beach stabilisation. Coastal Engineering Proceedings.
Nordlund, L. M., Jackson, E. L., Nakaoka, M., Samper-Villarreal, J., Beca-Carretero, P., and Creed, J. C. (2017). Seagrass ecosystem services–what’s next? Mar. Pollution Bull. 134, 145–151. doi: 10.1016/j.marpolbul.2017.09.014
Nordstrom, K. F. (2014). Living with shore protection structures: a review. Estuarine Coastal Shelf Sci. 150, 11–23. doi: 10.1016/j.ecss.2013.11.003
Ondiviela, B., Losada, I. J., Lara, J. L., Maza, M., Galván, C., Bouma, T. J., et al. (2014). The role of seagrasses in coastal protection in a changing climate. Coastal Eng. 87, 158–168. doi: 10.1016/j.coastaleng.2013.11.005
O’Shaughnessy, K., Hawkins, S. J., Evans, A. J., Hanley, M. E., Lunt, P., Thompson, R. C., et al. (2019). Design catalogue for eco-engineering of coastal artificial structures: a multifunctional approach for stakeholders and end-users. Urban Ecosystems 23, 431–443. doi: 10.1007/s11252-019-00924-z
Osland, M. J., Spivak, A. C., Nestlerode, J. A., Lessmann, J. M., Almario, A. E., Heitmuller, P. T., et al. (2012). Ecosystem development after mangrove wetland creation: plant–soil change across a 20-year chronosequence. Ecosystems 15, 848–866. doi: 10.1007/s10021-012-9551-1
Ounanian, K., Carballo-Cárdenas, E., van Tatenhove, J. P., Delaney, A., Papadopoulou, K. N., and Smith, C. J. (2018). Governing marine ecosystem restoration: the role of discourses and uncertainties. Mar. Policy 96, 136–144. doi: 10.1016/j.marpol.2018.08.014
Palmer, M. A., Zedler, J. B., and Falk, D. A. (2016). “Ecological theory and restoration ecology,” in Foundations of Restoration Ecology, eds D. A. Falk, M. A. Palmer, and J. B. Zedler (Washington, DC: Island Press).
Paul, M. (2018). The protection of sandy shores–can we afford to ignore the contribution of seagrass? Mar. Pollut. Bull. 134, 152–159. doi: 10.1016/j.marpolbul.2017.08.012
Perkol-Finkel, S., Hadary, T., Rella, A., Shirazi, R., and Sella, I. (2018). Seascape architecture–incorporating ecological considerations in design of coastal and marine infrastructure. Ecol. Eng. 120, 645–654. doi: 10.1016/j.ecoleng.2017.06.051
Perring, M. P., Standish, R. J., and Hobbs, R. J. (2013). Incorporating novelty and novel ecosystems into restoration planning and practice in the 21st century. Ecol. Processes 2, 1–8. doi: 10.4324/9781315442884-1
Phillips, S. J., and Dudík, M. (2008). Modeling of species distributions with Maxent: new extensions and a comprehensive evaluation. Ecography 31, 161–175. doi: 10.1111/j.0906-7590.2008.5203.x
Pickerell, C. H., Schott, S., and Wyllie-Echeverria, S. (2005). Buoy-deployed seeding: demonstration of a new eelgrass (Zostera marina L.) planting method. Ecol. Eng. 25, 127–136. doi: 10.1016/j.ecoleng.2005.03.005
Primavera, J. H., and Esteban, J. M. A. (2008). A review of mangrove rehabilitation in the Philippines: successes, failures and future prospects. Wetlands Ecol. Manag. 16, 345–358. doi: 10.1007/s11273-008-9101-y
Ralph, P. J., Durako, M. J., Enriquez, S., Collier, C. J., and Doblin, M. A. (2007). Impact of light limitation on seagrasses. J. Exp. Mar. Biol. Ecol. 350, 176–193. doi: 10.1016/j.jembe.2007.06.017
Ruckelshaus, M. H., Guannel, G., Arkema, K., Verutes, G., Griffin, R., Guerry, A., et al. (2016). Evaluating the benefits of green infrastructure for coastal areas: location, location, location. Coastal Manag. 44, 504–516. doi: 10.1080/08920753.2016.1208882
Saenger, P., and Moverley, J. (1985). Vegetative phenology of mangroves along the Queensland coastline. Proc. Ecol. Soc. Australia 13, 257–265.
Samson, M. S., and Rollon, R. N. (2008). Growth performance of planted mangroves in the Philippines: revisiting forest management strategies. AMBIO: J. Hum. Environment 37, 234–240. doi: 10.1579/0044-7447(2008)37[234:gpopmi]2.0.co;2
Schaffelke, B., Mellors, J., and Duke, N. C. (2005). Water quality in the Great Barrier Reef region: responses of mangrove, seagrass and macroalgal communities. Mar. Pollut. Bull. 51, 279–296. doi: 10.1016/j.marpolbul.2004.10.025
Schanz, A., and Asmus, H. (2003). Impact of hydrodynamics on development and morphology of intertidal seagrasses in the Wadden Sea. Mar. Ecol. Prog. Series 261, 123–134. doi: 10.3354/meps261123
Schonbeck, M., and Norton, T. A. (1978). Factors controlling the upper limits of fucoid algae on the shore. J. Exp. Mar. Biol. Ecol. 31, 303–313. doi: 10.1016/0022-0981(78)90065-5
Schonbeck, M. W., and Norton, T. A. (1980). Factors controlling the lower limits of fucoid algae on the shore. J. Exp. Mar. Biol. Ecol. 43, 131–150. doi: 10.1016/0022-0981(80)90021-0
Scott, A. L., York, P. H., Rasheed, M. A., and Coasts, J. E. (2020). Herbivory has a major influence on structure and condition of a great barrier reef subtropical seagrass meadow. Estuaries Coasts 44, 506–521. doi: 10.1007/s12237-020-00868-0
Scyphers, S. B., Powers, S. P., Heck, K. L. Jr., and Byron, D. (2011). Oyster reefs as natural breakwaters mitigate shoreline loss and facilitate fisheries. PLoS One 6:e22396. doi: 10.1371/journal.pone.0022396
Seebens, H., Gastner, M., Blasius, B., and Courchamp, F. J. E. (2013). The risk of marine bioinvasion caused by global shipping. Ecol. Lett. 16, 782–790. doi: 10.1111/ele.12111
Sharma, S., Goff, J., Moody, R. M., Byron, D., Heck, K. L. Jr., Powers, S. P., et al. (2016). Do restored oyster reefs benefit seagrasses? an experimental study in the Northern Gulf of Mexico. Restoration Ecol. 24, 306–313. doi: 10.1111/rec.12329
Sheridan, P. (2004). Recovery of floral and faunal communities after placement of dredged material on seagrasses in Laguna Madre, Texas. Estuarine Coastal Shelf Sci. 59, 441–458. doi: 10.1016/j.ecss.2003.10.004
Sierra-Correa, P. C., and Kintz, J. R. C. (2015). Ecosystem-based adaptation for improving coastal planning for sea-level rise: a systematic review for mangrove coasts. Mar. Policy 51, 385–393. doi: 10.1016/j.marpol.2014.09.013
Silva, R., Lithgow, D., Esteves, L. S., Martínez, M. L., Moreno-Casasola, P., Martell, R., et al. (2017). Coastal risk mitigation by green infrastructure in Latin America. Proc. Institution Civil Engineers-Maritime Eng. 170, 39–54. doi: 10.1680/jmaen.2016.13
Simkanin, C. (2013). Marine bioinvasions in anthropogenic and natural habitats: an investigation of nonindigenous ascidians in British Columbia. Mar. Ecol. 33, 499–511.
Smallegan, S. M., Irish, J. L., Van Dongeren, A. R., and Den Bieman, J. P. (2016). Morphological response of a sandy barrier island with a buried seawall during Hurricane Sandy. Coastal Eng. 110, 102–110. doi: 10.1016/j.coastaleng.2016.01.005
Smith, C. S., Gittman, R. K., Neylan, I. P., Scyphers, S. B., Morton, J. P., Fodrie, F. J., et al. (2017). Hurricane damage along natural and hardened estuarine shorelines: using homeowner experiences to promote nature-based coastal protection. Mar. Policy 81, 350–358. doi: 10.1016/j.marpol.2017.04.013
Smith, T. M., Reason, C., and McKenna, S. (2021). 2021. Seagrasses in Port Curtis and Rodds Bay 2020 Annual Long-term monitoring. Centre for Tropical Water & Aquatic Ecosystem Research (TropWATER) Publication 21/16. Cairns, QLD: James Cook University.
Soulsby, R. (1997). Dynamics of marine sands: a manual for practical applications. Oceanographic Literature Rev. 9:947.
Statton, J., Dixon, K. W., Irving, A. D., Jackson, E. L., Kendrick, G. A., Orth, R. J., et al. (2018). Decline and Restoration Ecology of Australian Seagrasses. Seagrasses of. Australia: Springer.
Strain, E. M. A., Cumbo, V. R., Morris, R. L., Steinberg, P. D., and Bishop, M. J. (2020). Interacting effects of habitat structure and seeding with oysters on the intertidal biodiversity of seawalls. PLoS One 15:e0230807. doi: 10.1371/journal.pone.0230807
Strain, E. M. A., Morris, R. L., Coleman, R. A., Figueira, W. F., Steinberg, P. D., Johnston, E. L., et al. (2018). Increasing microhabitat complexity on seawalls can reduce fish predation on native oysters. Ecol. Eng. 120, 637–644. doi: 10.1016/j.ecoleng.2017.05.030
Suman, D. O. (2019). Mangrove Management: Challenges and Guidelines. Coastal Wetlands. Amsterdam: Elsevier.
Sutton-Grier, A. E., Gittman, R. K., Arkema, K. K., Bennett, R. O., Benoit, J., Blitch, S., et al. (2018). Investing in natural and nature-based infrastructure: building better along our coasts. Sustainability 10:523. doi: 10.3390/su10020523
Taillardat, P., Friess, D. A., and Lupascu, M. (2018). Mangrove blue carbon strategies for climate change mitigation are most effective at the national scale. Biol. Lett. 14:20180251.
Tan, Y. M., Dalby, O., Kendrick, G. A., Statton, J., Sinclair, E. A., Fraser, M. W., et al. (2020). Seagrass restoration is possible: Insights and lessons from Australia and New Zealand. Front. Mar. Sci. 7:617. doi: 10.3389/fmars.2020.00617
Tanner, C. E., and Parham, T. (2010). Growing Zostera marina (eelgrass) from seeds in land-based culture systems for use in restoration projects. Restoration Ecol. 18, 527–537. doi: 10.1111/j.1526-100x.2010.00693.x
Temmerman, S., Meire, P., Bouma, T. J., Herman, P. M., Ysebaert, T., and De Vriend, H. J. (2013). Ecosystem-based coastal defence in the face of global change. Nature 504, 79–83. doi: 10.1038/nature12859
Thomas, R., Unsworth, R. K. F., and Rasheed, M. A. (2010). Seagrasses of Port Curtis and Rodds Bay and Long Term Monitoring, November 2009. Cairns, QLD: DEEDI.
Touchette, B. W., and Burkholder, J. M. (2000). Review of nitrogen and phosphorus metabolism in seagrasses. J. Exp. Mar. Biol. Ecol. 250, 133–167. doi: 10.1016/s0022-0981(00)00195-7
Van Cuong, C., Brown, S., To, H. H., and Hockings, M. (2015). Using melaleuca fences as soft coastal engineering for mangrove restoration in kien giang, Vietnam. Ecol. Eng. 81, 256–265. doi: 10.1016/j.ecoleng.2015.04.031
van Katwijk, M. M., and Wijgergangs, L. J. M. (2004). Effects of locally varying exposure, sediment type and low-tide water cover on Zostera marina recruitment from seed. Aquatic Botany 80, 1–12. doi: 10.1016/j.aquabot.2004.04.003
Vuik, V., Jonkman, S. N., Borsje, B. W., and Suzuki, T. (2016). Nature-based flood protection: the efficiency of vegetated foreshores for reducing wave loads on coastal dikes. Coastal Eng. 116, 42–56. doi: 10.1016/j.coastaleng.2016.06.001
Walker, S. J., Schlacher, T. A., and Thompson, L. M. (2008). Habitat modification in a dynamic environment: the influence of a small artificial groyne on macrofaunal assemblages of a sandy beach. Estuarine Coastal Shelf Sci. 79, 24–34. doi: 10.1016/j.ecss.2008.03.011
Warren, M. A., Gregory, R. S., Laurel, B. J., and Snelgrove, P. V. R. (2010). Increasing density of juvenile Atlantic (Gadus morhua) and Greenland cod (G. ogac) in association with spatial expansion and recovery of eelgrass (Zostera marina) in a coastal nursery habitat. J. Exp. Mar. Biol. Ecol. 394, 154–160. doi: 10.1016/j.jembe.2010.08.011
Wendländer, N. S., Lange, T., Connolly, R. M., Kristensen, E., Pearson, R. M., Valdemarsen, T., et al. (2019). Assessing methods for restoring seagrass (Zostera muelleri) in Australia’s subtropical waters. Mar. Freshwater Res. 71, 996–1005. doi: 10.1071/mf19237
Winterwerp, J. C., Albers, T., Anthony, E. J., Friess, D. A., Mancheño, A. G., Moseley, K., et al. (2020). Managing erosion of mangrove-mud coasts with permeable dams–lessons learned. Ecol. Eng. 158:106078. doi: 10.1016/j.ecoleng.2020.106078
Winterwerp, J. C., Erftemeijer, P. L. A., Suryadiputra, N., Van Eijk, P., and Zhang, L. (2013). Defining eco-morphodynamic requirements for rehabilitating eroding mangrove-mud coasts. Wetlands 33, 515–526. doi: 10.1007/s13157-013-0409-x
Wolanski, E., and Elliott, M. (2016). “3 - Estuarine sediment dynamics,” in Estuarine Ecohydrology, 2nd Edn, eds E. Wolanski and M. Elliott (Boston, MA: Elsevier).
Woodroffe, C. D., Rogers, K., McKee, K. L., Lovelock, C. E., Mendelssohn, I., and Saintilan, N. (2016). Mangrove sedimentation and response to relative sea-level rise. Ann. Rev. Mar. Sci. 8, 243–266. doi: 10.1146/annurev-marine-122414-034025
Yaakub, S. M., McKenzie, L. J., Erftemeijer, P. L., Bouma, T., and Todd, P. A. (2014). Courage under fire: seagrass persistence adjacent to a highly urbanised city–state. Mar. Pollut. Bull. 83, 417–424. doi: 10.1016/j.marpolbul.2014.01.012
Yozzo, D. J., Wilber, P., and Will, R. J. (2004). Beneficial use of dredged material for habitat creation, enhancement, and restoration in New York–New Jersey Harbor. J. Environ. Manage. 73, 39–52. doi: 10.1016/j.jenvman.2004.05.008
Zaneveld, J. S. (1969). Factors controlling the delimitation of littoral benthic marine algal zonation. Am. Zool. 9, 367–391. doi: 10.1093/icb/9.2.367
Keywords: ecological engineering, mangrove restoration, seagrass restoration, Working with Nature, marine sediment
Citation: Aiken CM, Mulloy R, Dwane G and Jackson EL (2021) Working With Nature Approaches for the Creation of Soft Intertidal Habitats. Front. Ecol. Evol. 9:682349. doi: 10.3389/fevo.2021.682349
Received: 18 March 2021; Accepted: 08 September 2021;
Published: 13 October 2021.
Edited by:
Louise Firth, University of Plymouth, United KingdomReviewed by:
Alice Jessie Twomey, The University of Queensland, AustraliaCopyright © 2021 Aiken, Mulloy, Dwane and Jackson. This is an open-access article distributed under the terms of the Creative Commons Attribution License (CC BY). The use, distribution or reproduction in other forums is permitted, provided the original author(s) and the copyright owner(s) are credited and that the original publication in this journal is cited, in accordance with accepted academic practice. No use, distribution or reproduction is permitted which does not comply with these terms.
*Correspondence: Emma L. Jackson, ZW1tYS5qYWNrc29uQGNxdS5lZHUuYXU=
Disclaimer: All claims expressed in this article are solely those of the authors and do not necessarily represent those of their affiliated organizations, or those of the publisher, the editors and the reviewers. Any product that may be evaluated in this article or claim that may be made by its manufacturer is not guaranteed or endorsed by the publisher.
Research integrity at Frontiers
Learn more about the work of our research integrity team to safeguard the quality of each article we publish.