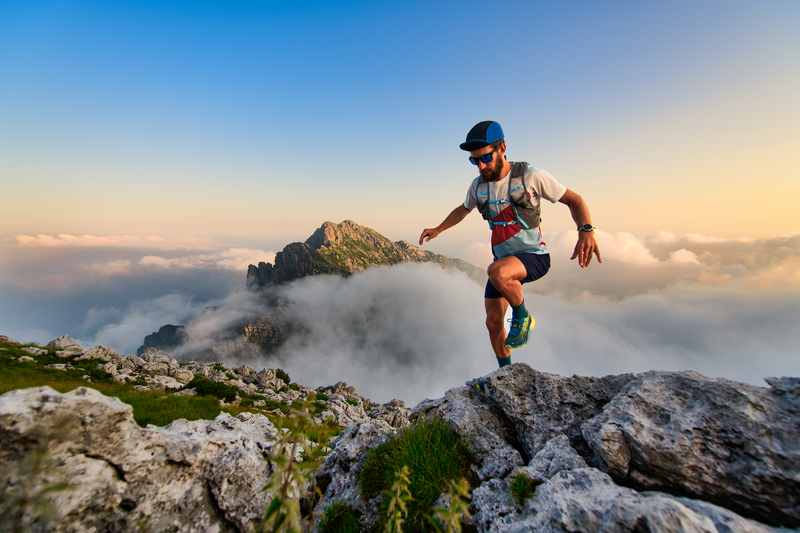
95% of researchers rate our articles as excellent or good
Learn more about the work of our research integrity team to safeguard the quality of each article we publish.
Find out more
ORIGINAL RESEARCH article
Front. Ecol. Evol. , 27 July 2021
Sec. Paleontology
Volume 9 - 2021 | https://doi.org/10.3389/fevo.2021.674151
This article is part of the Research Topic Origin and Early Evolution of Amniotes View all 16 articles
Relationships between the complexity of the cranial sutures and the inferred ecology of dicynodont synapsids are explored. Simple complexity indices based on degree of sutural interdigitation were calculated for 70 anomodont species and indicate that the naso-frontal sutures of Cistecephalidae, a clade inferred to be dedicated fossors based on aspects of postcranial morphology, are substantially more complex than those of other dicynodonts. The elevated complexity of the naso-frontal suture in this clade is interpreted as being related to compressive forces sustained during burrowing, paralleling the condition in some other fossorial vertebrate groups (e.g., amphisbaenians). The most highly interdigitated sutures in the cistecephalid skull are those oriented transversely to its long axis, which would experience the greatest longitudinal stresses from contact with the substrate. Although it is uncertain to what degree cistecephalid burrowing was based on scratch vs. head-lift digging, it is argued that the head played an important role during locomotion in this group. Increased sutural complexity, rather than cranial fusion, as an adaptation to resisting compressive forces during burrowing may be related to indeterminate growth in dicynodonts.
Anomodonts were the most ecologically diverse group of herbivorous synapsids in the Permo-Triassic, spanning an impressive size range (mature skull length from 4 to ∼100 cm) and occupying a breadth of niches, including small arboreal forms, mid-sized rooters, and massive, graviportal browsers (Cruickshank, 1978; King, 1990; Surkov and Benton, 2008; Fröbisch and Reisz, 2009). The majority of anomodont species richness and specimen abundance is held in its subclade Dicynodontia, a group characterized by a usually edentulous premaxillary beak and the presence of caniniform processes (usually housing tusks) on the maxilla (King, 1988). The earliest known dicynodonts appear in the fossil record in the mid-Permian (Rubidge and Day, 2020) and soon become the most abundant vertebrates in terrestrial ecosystems (Day et al., 2018). Although dicynodonts survived the end-Permian mass extinction and ranged through to the Late Triassic, their diversity was decimated, with their post-extinction radiation limited to a single clade of large-bodied taxa (Kammerer et al., 2013; Sulej and Niedźwiedzki, 2019).
Many unusual and specialized taxa can be found amongst the expansive Permian diversity of dicynodonts, including the deep-jawed endothiodontids with their secondarily expanded tooth row, massive-skulled geikiids with their exaggerated nasal bosses, and the enigmatic, short-skulled Lanthanostegus, which may have had stereoscopic vision (Latimer et al., 1995; Modesto et al., 2002; Maisch and Gebauer, 2005). The most highly specialized Permian dicynodonts, however, belong to the family Cistecephalidae. The type genus Cistecephalus was one of the first dicynodonts to be described from the fossil-rich late Permian strata of the South African Karoo Basin (Owen, 1876), and is usually one of the most abundant taxa at sites where it is found (making it a useful index fossil; Smith, 2020). With large, forward-facing orbits, a short, narrow snout, and an extremely broad interorbital region giving the skull a distinctly “boxy” appearance (hence the genus name, meaning “box head”), Cistecephalus has one of the most distinctive crania among dicynodonts. The oddity of its skull morphology inspired many decades of debate as to the ecology of this taxon, with proposals including fully or semi-aquatic (e.g., Broom, 1948; Brink, 1950), fossorial, and even scansorial/arboreal (e.g., Keyser, 1973). Cluver (1978) was the first to reconstruct the complete skeleton of Cistecephalus, recognizing a suite of derived features (e.g., robust humerus with massively expanded condyles, elongate olecranon process of the ulna, broadened manus, mobile pes) indicating that this genus was highly adapted to a fossorial lifestyle, similar to modern moles. Comparable adaptations had earlier been recognized in the closely related genera Kawingasaurus (Cox, 1972) and Cistecephaloides (Cluver, 1974), and the “cistecephalids as diggers” hypothesis is now generally accepted among paleontologists (see, e.g., Angielczyk and Kammerer, 2018).
Resolution of the question of cistecephalid ecology at a broad level has thankfully not meant the cessation of research on functional specializations in the group, and recent studies have augmented the morphological evidence for cistecephalid fossoriality with data from long bone microstructure (Nasterlack et al., 2012) and CT-assisted reconstructions of endocranial anatomy (Laaß, 2014; Laaß and Kaestner, 2017). Furthermore, discoveries of new cistecephalid taxa in Gondwanan basins outside of the Main Karoo have begun to provide data on the assembly of their highly specialized morphology, based on species showing a mosaic of the derived features seen in Cistecephalus and Kawingasaurus and the more generalized characters common to emydopoid dicynodonts (e.g., Lungmus et al., 2015; Kammerer et al., 2016; Angielczyk et al., 2019).
Here, I provide novel insights into cistecephalid cranial function using data from a previously understudied facet of their anatomy: cranial sutures. Study of dicynodont sutures and description of their variation has a lengthy history (Owen, 1876), and descriptions of the internal structure and complexity of dicynodont sutures go back to the earliest serial sections made of dicynodont skulls (Sollas and Sollas, 1914, 1916; Olson, 1944). Early studies such as these were largely limited to basic descriptive anatomy, albeit with occasional, brief comments on functional implications (e.g., Agnew, 1958). More recently, however, some workers have begun to examine dicynodont sutural structure in an explicitly functional context. In their study on the paleobiology of the iconic Early Triassic “disaster taxon” Lystrosaurus, King and Cluver (1990) noted a “zone of weakness” at the premaxillary-nasal contact, which they interpreted as a “shock-absorber” during biting/rooting. In a series of papers, Jasinoski et al. (2009, 2010a,b, 2014) re-examined the function of the premaxillary-nasal suture in Lystrosaurus in extensive detail, as part of broader studies of cranial function and sutural anatomy in that taxon and the Permian cryptodont Oudenodon. They concluded that the straight scarf suture between the premaxilla and nasal in Lystrosaurus primarily served to decrease stress and strain during biting, but may also have dampened forces incurred through rooting/grubbing behavior. Using finite element analysis (FEA), Jasinoski et al. (2010a) also reconstructed areas and orientations of dominant strain on the skulls of Lystrosaurus and Oudenodon during biting, relating these to observed sutural morphologies in these areas.
The growth and morphology of cranial sutures are related to a combination of genetic and epigenetic factors (Kopher and Mao, 2003; Slater et al., 2008). Cranial sutures are complex, plastic structures, often remaining patent late into ontogeny (Lenton et al., 2005; Marilao et al., 2020) and capable of alteration related to forces experienced by the skull. Sutures are capable of greater absorption of energy than solid bone (Jaslow, 1990), and different sutural configurations confer varying benefits/tradeoffs as regard minimizing the effects of stress and strain (Herring and Ochareon, 2005; Jasinoski et al., 2010c). One common sutural morphology in tetrapods is interdigitation, in which the edges between adjacent bones bear alternating processes forming complex interconnections. Foundational work on the mechanical properties of interdigitated sutures by Herring (1972; see also Herring, 1974, 2008; Herring and Mucci, 1991; Herring and Teng, 2000) and Jaslow (1990; see also Jaslow and Biewener, 1995; Jaslow and Lanier, 2001) has demonstrated that this morphology confers increased resistance to compressive strain. As a notable example of how this relates to behavior, Jaslow (1989) showed that sutural interdigitation is sexually dimorphic in wild sheep, with greater complexity present in males that sustain extreme compressive forces during agonistic head-butting (although quasi-static loading from the weight of the horns may also play a role; Herring, 2008).
Although dicynodont functional morphology is relatively well-studied (at least compared to that of coeval Permo-Triassic tetrapods), particularly as concerns feeding (e.g., Cox, 1959, 1998; Crompton and Hotton, 1967; Cluver, 1970; King, 1981; Renaut, 2001; Maisch, 2003; Angielczyk, 2004; Surkov and Benton, 2008; Cox and Angielczyk, 2015; Angielczyk et al., 2018), only a few studies have seriously explored the role of sutural morphology in this system (Jasinoski et al., 2009, 2010a,b). Furthermore, these studies have been limited to two particularly abundant genera (Lystrosaurus and Oudenodon) for which destructive sampling (sectioning) of cranial elements was possible; dicynodont sutural variation has yet to be analyzed in a broadly comparative systematic framework. Here, I present such a study, focusing on the naso-frontal suture. This suture was chosen for several reasons: (1). It is usually well-exposed on the skull, even in incompletely prepared specimens (allowing for an extensive sample size); (2). It is exposed largely in a single orientation (dorsal view of the skull) permitting quantitative study based on specimen photographs in orthogonal views; (3). It divides the snout from the rest of the skull roof, giving it potential importance in uniting distinct morphofunctional regions of the cranium; and (4). In the course of collecting data on dicynodont anatomy for previous studies (e.g., Kammerer et al., 2011), I anecdotally noted remarkable variation in the shape and complexity of this suture worth analyzing. Cistecephalids are particularly notable in this regard, usually showing highly interdigitated naso-frontal sutures. Complex sutures in Cistecephalus have been recognized for some time (e.g., Keyser, 1973), but whether they actually vary significantly from those of other dicynodonts and the functional implications of such variation have not yet been examined.
414 specimens representing 70 anomodont species were analyzed, including representatives from all major subclades. Four of the five currently recognized cistecephalid species were sampled; unfortunately, no specimens of the Indian taxon Sauroscaptor tharavati (Kammerer et al., 2016) preserve clear sutures in the snout region, so that species was excluded. Sampling density varied between taxa based on their abundance in collections and typical preservation regime; 21 of the sampled taxa are singletons (either because the taxon is only known from the holotype, or only a single specimen preserved measurable sutures). The largest sample is of the South African dicynodont Oudenodon bainii (with 55 specimens); other taxa with extensive sampling are also among the highest-abundance dicynodonts from the Karoo Basin (Diictodon feliceps, 45 specimens; Cistecephalus microrhinus, 36; Aulacephalodon bainii, 25; Lystrosaurus murrayi, 23; Pristerodon mackayi, 21; Tropidostoma dubium, 18; Dicynodon lacerticeps, 17).
Specimens from the following institutions were studied: AM, Albany Museum, Makhanda, South Africa; AMNH, American Museum of Natural History, New York, United States; BP, Evolutionary Studies Institute, University of the Witwatersrand, Johannesburg, South Africa; BSPG, Bayerische Staatssammlung für Paläontologie und Geologie, Munich, Germany; CGS, Council for Geosciences, Pretoria, South Africa; CM, Carnegie Museum of Natural History, Pittsburgh, United States; FMNH, Field Museum of Natural History, Chicago, United States; GPIT, Paläontologische Sammlung der Universität Tübingen, Tübingen, Germany; GSN, Geological Survey of Namibia, Windhoek, Namibia; IVPP, Institute of Vertebrate Paleontology and Paleoanthropology, Beijing, China; KPM, Vyatka Paleontological Museum, Kirov, Russia; LL, Manchester Museum, Manchester, United Kingdom; MB, Museum für Naturkunde, Berlin, Germany; MGB, Museu Guido Borgamanero, Mata, Brazil; MMK, McGregor Museum, Kimberley, South Africa; NHCC, Natural Heritage Conservation Commission, Lusaka, Zambia; NHMUK, The Natural History Museum, London, United Kingdom; NHMW, Naturhistorisches Museum Wien, Vienna, Austria; NMC/NMQR, National Museum, Bloemfontein, South Africa; PIN, Paleontological Institute of the Russian Academy of Sciences, Moscow, Russia; RC, Rubidge Collection, Wellwood Farm, Graaff-Reinet, South Africa; RGM, Naturalis, Leiden, The Netherlands; SAM, Iziko: The South African Museum, Cape Town, South Africa; TM, Ditsong: The National Museum of Natural History, Pretoria, South Africa; UCMP, University of California Museum of Paleontology, Berkeley, United States; UFRGS, Universidade Federal do Rio Grande do Sul, Porto Alegre, Brazil; UMZC, University Museum of Zoology, Cambridge, United Kingdom; UNIPAMPA, Universidade Federal do Pampa, São Gabriel, Brazil; UNLaR, Universidad Nacional de La Rioja, La Rioja, Argentina; USNM, National Museum of Natural History, Washington, D.C., United States.
Digital photographs (taken by the author) of skulls in dorsal view were imported into ImageJ 1.41o, with measurements calibrated using scale bars in the original photos. A simple interdigitation index (Rafferty and Herring, 1999) for the naso-frontal suture was calculated as the ratio of two measurements (Figure 1): (1). the shortest length between the origin of the naso-frontal suture at the cranial midline and the terminus of this suture at its contact with the prefrontal suture (measured using the “Straight Lines” tool), and (2). the complete path length between these points (measured using the “Freehand Lines” tool). Whenever possible, both the left and right naso-frontal sutures were measured on each skull, with the mean of these taken as the data point for the specimen. A total of 385 specimens had sutures that could be measured on both sides. Due to specimen incompleteness, 16 specimens could only be measured for the left and 13 specimens only for the right naso-frontal suture. Data for all specimens is available as Supplementary Data 1.
Figure 1. Illustration of the measurement protocol used in calculating the sutural complexity index. Straight black line (1) indicates least path length from the origin (o) to the terminus (t) of the left naso-frontal suture in dorsal view. Red line (2) shows total path length of the suture between these points. Sutural complexity index represents 1÷2. Scale bar represents 1 cm.
Analyses were performed in RStudio (RStudio Team, 2020). Clustering was performed on a data set of species-level means using Jenks natural breaks classification via the “setjenksBreaks” function in the R package BAMMtools (Rabosky et al., 2014), with optimal cluster number determined to be three using the elbow method. Natural breaks visualization was performed using the “plotJenks” function in the R package GmAMisc (Alberti, 2021).
A chart of mean naso-frontal interdigitation indices for all sampled taxa is shown in Figure 2. The lowest values (near 1.00, indicating sutures that are effectively straight) are those of non-dicynodont anomodonts (with the exception of Ulemica, which had a relatively high interdigitation index of 2.09). The four cistecephalids occupy the right-hand edge of the chart and exhibit what are by far the highest interdigitation values (> 3.50). These taxa all exhibit intensely interdigitated naso-frontal sutures (Figures 3A,B,D,E). The highest value outside of Cistecephalidae is Diictodon feliceps, in which the naso-frontal suture is usually also interdigitated (Figure 3G), but to a markedly lesser degree. Diictodon specimens also often exhibit an anterior process of the frontals, but this is a comparably minor addition to the path length of the suture. Dicynodontoids, which usually lack notable interdigitation of the naso-frontal suture, often also bear anterior frontal processes (e.g., Figure 3I), but their sutural complexity indices are generally low (Figure 2).
Figure 2. Mean interdigitation index of the naso-frontal suture plotted for sampled anomodont species. Cistecephalids highlighted in black. Dotted lines indicate Jenks natural breaks separating the data into optimized clusters. Taxa: 1. Patranomodon nyaphulii. 2. Otsheria netzvetajevi. 3. Lystrosaurus youngi. 4. Myosaurus gracilis. 5. Rhadiodromus mariae. 6. Katumbia parringtoni. 7. “Kannemeyeria” latirostris. 8. Elph borealis. 9. Shansiodon wangi. 10. Kitchinganomodon crassus. 11. Lystrosaurus declivis. 12. Idelesaurus tataricus. 13. Lystrosaurus hedini. 14. Delectosaurus arefjevi. 15. Dicynodontoides nowacki. 16. Interpresosaurus blomi. 17. Brachyprosopus broomi. 18. Endothiodon tolani. 19. Jimusaria sinkianensis. 20. Endothiodon bathystoma. 21. Lystrosaurus maccaigi. 22. Lystrosaurus murrayi. 23. Kannemeyeria simocephalus. 24. Abajudon kaayai. 25. Tetragonias njalilus. 26. Aulacephalodon bainii. 27. Geikia locusticeps. 28. Odontocyclops whaitsi. 29. Stahleckeria potens. 30. Dinanomodon gilli. 31. Daptocephalus leoniceps. 32. Syops vanhoepeni. 33. Euptychognathus bathyrhynchus. 34. Dolichuranus primaevus. 35. Compsodon helmoedi. 36. Dicynodon lacerticeps. 37. Vivaxosaurus trautscholdi. 38. Thliptosaurus imperforatus. 39. Rastodon procurvidens. 40. Rhachiocephalus magnus. 41. Delectosaurus berezhanensis. 42. Peramodon amalitzkii. 43. Pelanomodon moschops. 44. Dinodontosaurus tener. 45. Daqingshanodon limbus. 46. Lystrosaurus curvatus. 47. Dinodontosaurus brevirostris. 48. Jachaleria candelariensis. 49. Bulbasaurus phylloxyron. 50. Oudenodon bainii. 51. Dicynodontoides recurvidens. 52. Oudenodon luangwanensis. 53. Prosictodon dubei. 54. Emydops arctatus. 55. Emydorhinus sciuroides. 56. Ulemica efremovi. 57. Australobarbarus kotelnitschi. 58. Pristerodon mackayi. 59. Eosimops newtoni. 60. Tropidostoma dubium. 61. Eodicynodon oosthuizeni. 62. Digalodon rubidgei. 63. Keyseria benjamini. 64. Basilodon woodwardi. 65. Robertia broomiana. 66. Diictodon feliceps. 67. Cistecephaloides boonstrai. 68. Kawingasaurus fossilis. 69. Cistecephalus microrhinus. 70. Kembawacela kitchingi.
Figure 3. Comparison of naso-frontal sutures (highlighted in red) in select dicynodonts. (A) Cistecephalus microrhinus (BP/1/33). (B) Cistecephalus microrhinus (CGS R30). (C) Cistecephalus microrhinus, aberrant specimen (CGS RMS410). (D) Kembawacela kitchingi (NHCC LB14). (E) Cistecephaloides boonstrai (SAM-PK-6243). (F) Myosaurus gracilis (BP/1/4262). (G) Diictodon feliceps (SAM-PK-K7730). (H) Delectosaurus arefjevi (PIN 4644/1). (I) Dinanomodon gilli (SAM-PK-K10618). Specimens in (A–E) are cistecephalids (inferred to be fossorial). (F) is a member of the sister-clade to Cistecephalidae (Myosauridae), whose ecology is uncertain. (G) is a known burrower (skeletons found in burrow casts), but lacks specializations indicating that it was primarily fossorial in ecology. (H) and (I) are large, presumed fully surface-dwelling taxa. Scale bars represent 1 cm.
Application of the Jenks natural breaks algorithm to the data demonstrates that cistecephalids form a discrete data class separated from other known anomodonts by their elevated naso-frontal sutural complexity. The three means classes separated by Jenks natural breaks consist of: (1). a large (n = 51) group of anomodont species with minimal naso-frontal interdigitation (1.01–1.83); (2). a smaller (n = 15) group of primarily small-bodied dicynodont species with moderate naso-frontal interdigitation (1.95–2.95); and (3). a group consisting solely of the cistecephalid species in the data (n = 4), with high naso-frontal interdigitation (3.65–4.65). Class 2 (those with moderate interdigitation) includes one taxon known to have lived in burrows, Diictodon feliceps (a taxon whose fossils have been found preserved inside burrow casts; Smith, 1987; Smith et al., 2021). Class 1 (low interdigitation) also contains one taxon (Lystrosaurus curvatus) that has been found preserved in a burrow cast (Botha-Brink, 2017). Enforcing additional (suboptimal) breaks results in the partition of classes 1 and 2, but in all cases cistecephalids remain a separate and discrete class (see Supplementary Data 2).
Potential sexual dimorphism has been noted in the literature for Cistecephalus microrhinus (Nasterlack et al., 2012), based on the presence/absence of supraorbital ridges in specimens greater than 5 cm in skull length. Possible sexual variability in sutural morphology is difficult to evaluate for this taxon, however. Only seven C. microrhinus specimens in the data set showed definite, well-developed supraorbital ridges (BP/1/496, CGS R154, NMQR 1671, SAM-PK-K11187, UCMP 42802, UMZC T403, and USNM 22942). In many of the larger (> 5 cm) specimens without ridges, though, breakage or erosion of the skull roof makes their biological (rather than taphonomic) absence uncertain, so the ridge-less specimens do not form a useful comparative sample. With this said, it is worth noting that the ridge-bearing specimens all fall within the range of variation present in the ridge-less sample (naso-frontal interdigitation indices for specimens with supraorbital ridges: 2.78–5.66; total range of variation for C. microrhinus: 1.88–6.62; although see Discussion for caveats on the low-complexity outliers). Thus, while at present there is no indication that the presence of ridges is associated with differences in sutural morphology, a larger sample of undamaged, well-prepared Cistecephalus crania is needed to test this in greater detail.
The quantitative analysis herein focused on the naso-frontal suture, both for its functional significance and its relative ease of measurement. Although it was not possible to analyze the sutural complexity of all cranial sutures across the same phylogenetic breadth, some qualitative observations on cistecephalid sutures in general are warranted. As observed by Jasinoski et al. (2010a) for Lystrosaurus and Oudenodon, a variety of sutural types are present in the skull of Cistecephalus, including scarf sutures between the maxilla, jugal, and squamosal and butt-ended sutures between the parietals. However, a greater number of sutures in Cistecephalus appear interdigitated than in other dicynodonts, and interdigitated sutures in Cistecephalus appear to generally be more complex than those of other dicynodonts.
To illustrate this, Figure 4 shows comparisons between ventral sutures of the cranium in Cistecephalus microrhinus and Dicynodon lacerticeps. The Cistecephalus specimen (BSPG 1932-I-502) is somewhat overprepared, showing sutural morphology below the actual bone surface, but comparisons with intact Cistecephalus specimens and comparably overprepared specimens of other dicynodont groups indicate that the general trends on display here hold (Kammerer, pers. obs.; the greater complexity of the Cistecephalus sutures below the surface indicate that the interdigitation indices calculated based on surface structure are likely an underestimate, and future work analyzing this complexity in 3D using CT-data would be fruitful). Particularly notable are the longitudinally oriented interdigitations along sutures running transverse to the long axis of the skull (e.g., the maxillary-palatine, palatine-pterygoid, pterygoid-parabasisphenoid, and parabasisphenoid-basioccipital sutures), which are clearly more elongate and complex than the comparable sutures in Dicynodon.
Figure 4. Comparisons of sutural complexity on the ventral surface of the skull in two dicynodonts. (A,B) Photograph and interpretive drawing of Cistecephalus microrhinus (BSPG 1932-I-502). (C,D) Photograph and interpretive drawing of Dicynodon lacerticeps (SAM-PK-K7011). Boxes to the right show magnified views of select sutural boundaries from the interpretive drawings, with Cistecephalus sutures shown in the left column and Dicynodon sutures in the right. ec, ectopterygoid; ju, jugal; mx, maxilla; pbs, parabasisphenoid; pl, palatine; pmx, premaxilla; pt, pterygoid. Gray regions in interpretive drawings indicate matrix. Scale bars represent 1 cm.
Previous work in dicynodonts (Jasinoski et al., 2010a) associated sutural interdigitation (e.g., the premaxillary-nasal suture of Oudenodon) with compressive strain incurred while biting. While bite forces doubtless played some role in shaping cistecephalid sutures, there is reason to suspect that additional influences are at play here. Cox (1998, p. 372) studied jaw mechanics across a wide variety of dicynodont taxa, concluding that the feeding system of cistecephalids was “almost identical” to that of other small emydopoids such as Emydops and Myosaurus. If strain incurred during feeding is the dominant factor underlying sutural complexity in cistecephalids, we would expect the interdigitation indices of Emydops and Myosaurus to be comparable to them. Myosaurus in particular, as the taxon usually recovered as most closely related to cistecephalids among emydopoids (e.g., Kammerer et al., 2016; Angielczyk et al., 2019), should show similar sutural morphology, yet it has one of the lowest interdigitation indices in Dicynodontia (Figures 2, 3F). The marginally more powerful bites of cistecephalids (inferred based on larger areas of insertion for jaw musculature; Cox, 1998) are unlikely to account for this difference (particularly as clades with substantially greater areas of attachment for jaw musculature, such as cryptodonts and dicynodontoids, also have markedly lower interdigitation indices). Instead, it is likely that this morphology is tied to external forces, related to the highly specialized lifestyle of cistecephalids.
Extreme sutural interdigitation as an adaptation to a fossorial lifestyle was first recognized by Gans (1960, 1974, 1978) in his studies of amphisbaenian reptiles. The majority of amphisbaenians are legless and burrow by compressing sediment using powerful movements of the head (Gans, 1973; Navas et al., 2004; Kazi and Hipsley, 2018). Amphisbaenian skulls are generally divided into two major morphofunctional components, an anterior (pre-orbital) “spade” and a posterior (post-orbital) “handle” region, divided dorsally at the deeply interdigitated fronto-parietal suture (Gans and Montero, 2008). The complex interdigitations at this junction help to reduce the intense compressive stresses incurred by the snout during burrowing (Gans, 1960). Although less extreme, complexly interdigitated sutures of the skull roof are also present in the elongate Permo-Carboniferous recumbirostran Brachydectes, which has also been interpreted as a head-first burrower (Bolt and Wassersug, 1975; Pardo and Anderson, 2016). However, this specialization is not present in all vermiform burrowing tetrapods, with little interdigitation of the dorsal cranial sutures present in caecilians (Kleinteich et al., 2012), blind snakes (Rieppel et al., 2009), or legless skinks (Rieppel, 1981). In non-vermiform dedicated fossors such as moles, the skull is often extensively fused such that the sutures have been obliterated during ontogeny (Goswami and Prochel, 2007).
Mammalian fossors are generally divided into three categories: “scratch diggers” (e.g., true moles), “chisel-tooth diggers” (e.g., most mole rats), and “head-lift diggers” (e.g., marsupial and golden moles), based on their primary mode of substrate alteration (Hildebrand, 1985). However, there can be extensive overlap between these categories: tuco-tucos (Ctenomys) use both scratch and chisel-tooth digging in constructing burrows (Echeverría et al., 2017), and all head-lift diggers also use scratch or chisel-tooth digging to aid in moving sediment while burrowing (Borghi et al., 2002). Head-lift diggers use the head as a wedge, incurring compressive forces from contact with the sediment, and often have the surface of the head covered in thickened, hairless integumentary pads inferred to alleviate stresses from this activity (Wake, 1993). Convergence in overall skull shape between the major categories of fossorial mammals has been used to infer digging behavior in extinct rodents (Samuels and Van Valkenburgh, 2009), but has not been applied to the vastly different non-mammalian configuration of the cistecephalid skull. Based on gross morphology (Angielczyk et al., 2019) and analyses of manual element proportions (Kümmell and Frey, 2012), cistecephalids have been reconstructed as scratch-diggers, primarily using their expanded, flattened manus and unguals to move sediment. However, numerous authors (e.g., Cox, 1972; Cluver, 1974; Nasterlack et al., 2012) have also noted the distinctly “wedge-shaped” appearance of the cistecephalid snout, suggesting that the skull also played some role in burrowing.
If the skull of cistecephalids was in any way used as a wedge during burrowing, even if not to the degree of extant head-lift diggers, it would incur compressive strain. Intense interdigitation of the sutures in this clade is here suggested to represent a plastic response from the bones to such forces, with this sutural structure increasingly conferring resistance through the animal’s life (Jaslow, 1990). Given that the animal would have been locomoting head-first through sediment, it makes sense that it is mostly the transverse sutures which show extreme interdigitation, as they are most subject to longitudinally oriented compression of the skull. It should be noted, however, that recent fossorial mammals mostly lack interdigitated sutures and instead alleviate this issue through a combination of calvarial fusion, skull pneumatization, and (in the case of head-lift diggers) soft-tissue integumentary shields. Given that the cistecephalid body morph is more similar, overall, to that of extant mammalian fossors (all of which are, to varying degrees, convergent on the postcranial anatomy originated by cistecephalids) than reptilian fossors (in which a trend toward vermiform locomotion dominates), why then did they not also converge in their cranial specializations? The difference may lie in growth patterns.
Mammals are generally characterized by determinate growth, in which the animal ceases skeletal growth upon reaching maturity (Mumby et al., 2015). By contrast, many ectothermic vertebrates exhibit indeterminate growth, in which growth continues after sexual maturity, sometimes for the duration of the animal’s lifespan (Armstrong et al., 2017). As is the case for many aspects of their paleobiology, non-mammalian synapsid growth appears to show a mixture of traits seen in extant ectothermic and endothermic tetrapods. Osteohistological studies suggest that early synapsids (“pelycosaurs”) had an indeterminate growth strategy (Sánchez-Villagra, 2010), whereas later, more mammal-like synapsids (e.g., mammaliamorph cynodonts) had determinate growth (Chinsamy and Hurum, 2006). Non-cynodont therapsids such as dicynodonts generally show rapid early growth slowing markedly into maturity (Botha-Brink and Angielczyk, 2010), but can be considered indeterminate overall (Ray et al., 2009). Although fused elements can still grow (as an example within the study clade, several braincase/occipital elements in dicynodonts are usually fused into a single periotic bone early in development, which nevertheless increases in absolute size during ontogeny; Kammerer et al., 2015), the growth potential of elements with closed sutures is reduced (Delashaw et al., 1989), so it is possible that indeterminate growth in this clade conflicted with the trend toward broad fusion across the cranium seen in fossorial mammals.
The two dicynodont taxa (Diictodon feliceps and Lystrosaurus curvatus) known to have occupied burrows based on fossil evidence (Smith, 1987; Botha-Brink, 2017) have naso-frontal interdigitation indices markedly lower than those of cistecephalids. Based on Jenks natural breaks classification, Diictodon falls into class 2 (moderately interdigitated) for mean complexity, while L. curvatus is a member of class 1 (minimally interdigitated) (Figure 2). This variation can probably be attributed to differences in lifestyle between these taxa and cistecephalids. The combination of general postcranial anatomical (Cluver, 1978), osteohistological (Nasterlack et al., 2012), endocranial (Laaß, 2014), and cranial sutural data (this paper) are all consistent with a dedicated fossorial lifestyle for cistecephalids (i.e., their locomotion was for the most part occurring in the subterranean realm). By contrast, the known burrows of Diictodon and Lystrosaurus appear to represent dens (also potentially brood chambers in the case of Diictodon; Smith et al., 2021). If this is the case, these animals would have been foraging primarily at the surface and using the burrow as a refuge.
Burrows of Diictodon are highly stereotyped (a helical tunnel emerging into an expanded terminal living chamber; Smith, 1987) and indicate that they were dug from the surface, without substantial subterranean elaboration or extension following their initial construction. The one robustly identified Lystrosaurus burrow cast consists of a simple, expanded terminal chamber (Botha-Brink, 2017). Another possible Lystrosaurus burrow (containing definite Lystrosaurus skeletal remains, although these have alternatively been interpreted as prey left by a carnivorous burrow-maker; Modesto and Botha-Brink, 2010) consists of a similar terminal chamber connected to the surface by a straight, low-angle ramp. Such burrows may have remained stable and been occupied for long periods, and these taxa may have spent only a small portion of their time actively digging. Of the two, Diictodon is more frequently found in burrows and does exhibit some (albeit minor compared to cistecephalids) postcranial adaptations for scratch-digging (Ray and Chinsamy, 2003). Notably, this taxon also has the highest naso-frontal interdigitation index outside of Cistecephalidae, suggesting that it was also experiencing compressive stresses on the skull, even if not as consistently and/or intensely as cistecephalids. Other dicynodonts in class 2 mostly consist of small-bodied taxa similar to Diictodon in general morphology (including other pylaecephalids, Emydops, and Pristerodon), and their elevated interdigitation indices (relative to class 1 anomodonts) could be evidence for some burrowing behavior in these taxa as well. Although it is important not to over-attribute variation in complex structures such as sutures to a single source (considering that feeding behaviors and even potentially agonistic behaviors can also be important influences on suture shape), the higher complexity of the class 2 dicynodonts relative to otherwise similar taxa in class 1 (e.g., Compsodon, Elph, Myosaurus) does beg explanation, and burrowing (if only for den construction) should be considered as a possibility.
One unusual specimen of Cistecephalus (CGS RMS410; Figure 3C) represents a notable outlier in the data. With an interdigitation index of only 1.88, it lies well outside the main range of variation (3.31–6.62) for this taxon. A second outlier (BP/1/496; interdigitation index = 0.278) is also below this range, but in this specimen the naso-frontal suture was traced in black ink following its initial preparation, which may not have captured the true complexity of the underlying structure. The suture in CGS RMS410, by contrast, is unmarked, well-preserved, and well-exposed on the skull, such that no latter-day alterations can be invoked to explain its low complexity. Although not quantified, it should be noted that the other exposed sutures on the skull also show minimal interdigitation (contrasting with that seen in, e.g., BSPG 1932-I-502). Nasterlack et al. (2012) referred CGS RMS410 to the species Cistecephalus microrhinus, and this referral is upheld here. Other than in its sutural morphology, this specimen accords perfectly with other C. microrhinus specimens of comparable size; in gross morphology it is nearly indistinguishable from a specimen such as BP/1/33 (Figure 3A). As such, taxonomic distinction is unlikely to explain the low amount of interdigitation in this individual. Finally, while this specimen is relatively large (basal skull length 6.70 cm), it is not at the maximum size for C. microrhinus, so its condition cannot be attributed to occupying an extreme in the growth history of the taxon.
In the absence of clear taphonomic, taxonomic, or ontogenetic explanations for the low sutural complexity of CGS RMS410, some speculation as to possible life history factors is warranted. Although fossorial behavior in cistecephalids is well-supported based on morphological evidence, nothing is known about other aspects of the life history and behavior in this clade. One particularly notable gap in our knowledge concerns the structure of cistecephalid burrows and the potential for cistecephalid sociality; there are no published examples of a cistecephalid specimen within a burrow cast. The best fossil record of dicynodont burrows is that of Diictodon, which demonstrate that this taxon produced Daimonelix-style helical burrows with an elongate terminal chamber that could house up to two adult individuals (Smith, 1987; Ray and Chinsamy, 2003). It is unknown whether Cistecephalus had a comparable, lesser, or greater degree of sociality; although highly abundant in the strata where they occur (Smith, 2020), Cistecephalus fossils are usually disarticulated (with only the skulls typically recovered), making life association of this material highly uncertain. Modern burrowing mammals run the gamut from completely solitary (e.g., the mole, Talpa) to maintaining large, multigenerational colonies with complex social structure (e.g., prairie dogs, Cynomys) to eusocial (e.g., the naked mole rat, Heterocephalus) (Jarvis, 1981; Loy et al., 1994; Johnson and Collinge, 2004). Cistecephalids could conceivably have occupied any part of this spectrum.
If the intense interdigitation of cistecephalid sutures developed as a result of compressive forces exerted on the skull, as argued here, this suggests that CGS RMS410 was, for some reason, not experiencing those forces during its life. The locality producing this specimen (the farm Vogelfontein, near Fraserburg in the Northern Cape Province) yields other C. microrhinus specimens (e.g., CGS RMS413) showing the typical naso-frontal morphology for the species, suggesting that local differences in substrate (i.e., looser, sandier soil) are not the cause. At present, we can only speculate, but several possibilities are worth considering. Could this individual have occupied an existing burrow (as known to have been done by some other Permo-Triassic tetrapods; Fernandez et al., 2013) and been foraging primarily at the surface? Many extant rodents use burrows primarily as protection/den sites and do most of their foraging on the surface (Heffner et al., 1994), although this would seem to conflict with the extensive postcranial specializations of cistecephalids for fossorial life. Alternatively, could it be that this individual was somehow incapacitated and cared for by a conspecific? There is no skeletal evidence of pathology in CGS RMS410, although this is not proof that the animal was operating at normal capacity. A final possibility to consider would be eusocial cistecephalids, with CGS RMS410 representing a member of a non-working (and thus, non-digging) caste. However, this is unlikely, given the large sample known for this species and the absence of any other comparably low-complexity individuals in the record. For now, this is a mystery, and demonstrates how much there is left to learn about the paleobiology of even the best-studied and most abundant early synapsids. In the case of cistecephalids, many of the specimens in museums were collected with limited taphonomic context, prior to field-recognition of burrow casts, so it is hoped that renewed fieldwork at classic localities (Smith, 2020) will improve our understanding of how these remarkable animals actually lived.
The original contributions presented in the study are included in the article/Supplementary Material, further inquiries can be directed to the corresponding author.
CK photographed the specimens, analyzed the data, produced the figures, and wrote the manuscript.
The photographic data utilized in this study was collected over the course of numerous collections visits, some which were supported by NSF DEB 0608415 from the National Science Foundation and DFG KA 3144-1 from the Deutsche Forschungsgemeinschaft.
The author declares that the research was conducted in the absence of any commercial or financial relationships that could be construed as a potential conflict of interest.
All claims expressed in this article are solely those of the authors and do not necessarily represent those of their affiliated organizations, or those of the publisher, the editors and the reviewers. Any product that may be evaluated in this article, or claim that may be made by its manufacturer, is not guaranteed or endorsed by the publisher.
Sincere thanks to the many collections managers and curators who facilitated access to the anomodont fossils used in this study: Billy de Klerk (AM), Carl Mehling (AMNH), Sifelani Jirah (BP/ESI), Oliver Rauhut (BSPG), Nonhlanhla Mchunu (CGS), Bill Simpson (FMNH), Ingmar Werneburg (GPIT), Helke Mocke (GSN), Li Jinling (IVPP), Kenneth Angielczyk (NHCC specimens currently under study at the FMNH), Paul Barrett (NHMUK), Ursula Göhlich (NHMW), Elize Butler (NMC/NMQR), Valeriy Golubev and the late Mikhail Ivakhnenko (PIN), Robert and Marion Rubidge (RC), Zaituna Skosan (SAM), Heidi Fourie (TM), Pat Holroyd (UCMP), Cesar Schultz (UFRGS), Mat Lowe (UMZC), Felipe Pinheiro (UNIPAMPA), Matt Carrano (USNM). Thanks to Elizabeth Gray for a helpful discussion of clustering methodologies and the three reviewers (JM and two anonymous) for their aid in improving the manuscript. Thanks also to the special issue editors JF, Mark MacDougall, Michel Laurin, Sean Modesto, and Robert Reisz for their invitation to contribute to this selection of papers on the early evolutionary history of amniotes.
The Supplementary Material for this article can be found online at: https://www.frontiersin.org/articles/10.3389/fevo.2021.674151/full#supplementary-material
Supplementary Data 1 | Spreadsheet of measurements, specimen means, and species means for the 70 sampled anomodont species (.xlsx).
Supplementary Data 2 | Additional plots for the Jenks natural breaks classification of species means (.pdf).
Agnew, J. D. (1958). Cranio-osteological studies in Dicynodon grimbeeki with special reference to the sphenethmoid region and cranial kinesis. Palaeontol. Afr. 6, 77–107.
Alberti, G. (2021). GmAMisc: ‘Gianmarco Alberti’ Miscellaneous. R Package Version 1.2.0. Available online at: https://CRAN.R-project.org/package=GmAMisc (accessed June 7, 2021).
Angielczyk, K. D. (2004). Phylogenetic evidence for and implications of a dual origin of propaliny in anomodont therapsids (Synapsida). Paleobiology 30, 268–296. doi: 10.1666/0094-8373(2004)030<0268:pefaio>2.0.co;2
Angielczyk, K. D., Benoit, J., and Rubidge, B. S. (2019). A new tusked cistecephalid dicynodont (Therapsida, Anomodontia) from the upper Permian Upper Madumabisa Mudstone Formation, Luangwa Basin, Zambia. Pap. Palaeontol. 7, 405–446. doi: 10.1002/spp2.1286
Angielczyk, K. D., Hancox, P. J., and Nabavizadeh, A. (2018). A redescription of the Triassic kannemeyeriiform dicynodont Sangusaurus (Therapsida, Anomodontia), with an analysis of its feeding system. Soc. Vertebr. Paleontol. Mem. 17, 189–227. doi: 10.1080/02724634.2017.1395885
Angielczyk, K. D., and Kammerer, C. F. (2018). “Non-mammalian synapsids: the deep roots of the mammalian family tree,” in Handbook of Zoology: Mammalia, eds R. Asher and F. Zachos (Berlin: DeGruyer), 117–198. doi: 10.1515/9783110341553-005
Armstrong, D. P., Keevil, M. G., Rollinson, N., and Brooks, R. J. (2017). Subtle individual variation in indeterminate growth leads to major variation in survival and lifetime reproductive output in a long-lived reptile. Funct. Ecol. 32, 752–761. doi: 10.1111/1365-2435.13014
Bolt, J. R., and Wassersug, R. J. (1975). Functional morphology of the skull in Lysorophus: a snake-like Paleozoic amphibian (Lepospondyli). Paleobiology 1, 320–332. doi: 10.1017/s0094837300002566
Borghi, C. E., Giannoni, S. M., and Roig, V. G. (2002). Eye reduction in subterranean mammals and eye protective behavior in Ctenomys. J. Neotrop. Mammal. 9, 123–134.
Botha-Brink, J., and Angielczyk, K. D. (2010). Do extraordinarily high growth rates in Permo-Triassic dicynodonts (Therapsida, Anomodontia) explain their success before and after the end-Permian extinction? Zool. J. Linn. Soc. 160, 341–365. doi: 10.1111/j.1096-3642.2009.00601.x
Botha-Brink, J. (2017). Burrowing in Lystrosaurus: preadaptation to a postextinction environment? J. Vertebr. Paleontol. 37:e1356080. doi: 10.1080/02724634.2017.1365080
Brink, R. (1950). On a new species of Cistecephalus Owen. Ann. Mag. Nat. Hist. 3, 985–997. doi: 10.1080/00222935008656104
Broom, R. (1948). A contribution to our knowledge of the vertebrates of the Karroo Beds of South Africa. Trans. R. Soc. Edinburgh 61, 577–629. doi: 10.1017/s0080456800004865
Chinsamy, A., and Hurum, J. H. (2006). Bone microstructure and growth patterns of early mammals. Acta Palaeontol. Pol. 51, 325–338.
Cluver, M. A. (1970). The palate and mandible in some specimens of Dicynodon testudirostris Broom & Haughton (Reptilia, Therapsida). Ann. S. Afr. Mus. 56, 133–153.
Cluver, M. A. (1974). The skull and mandible of a new cistecephalid dicynodont. Ann. S. Afr. Mus. 67, 7–23.
Cluver, M. A. (1978). The skeleton of the mammal-like reptile Cistecephalus with evidence for a fossorial mode of life. Ann. S. Afr. Mus. 76, 213–246.
Cox, C. B. (1959). On the anatomy of a new dicynodont genus with evidence of the position of the tympanum. Proc. Zool. Soc. Lond. 132, 321–367. doi: 10.1111/j.1469-7998.1959.tb05526.x
Cox, C. B. (1972). “A new digging dicynodont from the upper Permian of Tanzania,” in Studies in Vertebrate Evolution, eds K. A. Joysey and T. S. Kemp (Edinburgh: Oliver and Boyd), 173–189.
Cox, C. B. (1998). The jaw function and adaptive radiation of the dicynodont mammal-like reptiles of the karoo basin of South Africa. Zool. J. Linn. Soc. 122, 349–384. doi: 10.1111/j.1096-3642.1998.tb02534.x
Cox, C. B., and Angielczyk, K. D. (2015). A new endothiodont dicynodont (Therapsida, Anomodontia) from the Permian Ruhuhu formation (Songea Group) of Tanzania and its feeding system. J. Vertebr. Paleontol. 35:e935388. doi: 10.1080/02724634.2014.935388
Crompton, A. W., and Hotton, N. III (1967). Functional morphology of the masticatory apparatus of two dicynodonts (Reptilia, Therapsida). Postilla 109, 1–51.
Cruickshank, A. R. I. (1978). Feeding adaptations in Triassic dicynodonts. Palaeontol. Afr. 21, 121–132.
Day, M. O., Benson, R. B., Kammerer, C. F., and Rubidge, B. S. (2018). Evolutionary rates of mid-Permian tetrapods from South Africa and the role of temporal resolution in turnover reconstruction. Paleobiology 44, 347–367. doi: 10.1017/pab.2018.17
Delashaw, J. B., Persing, J. A., Broaddus, W. C., and Jane, J. A. (1989). Cranial vault growth in craniosynostosis. J. Neurosurg. 70, 159–165. doi: 10.3171/jns.1989.70.2.0159
Echeverría, A. I., Becerra, F., Buezas, G. N., and Vassallo, A. I. (2017). Bite it forwards…bite it better? Incisor procumbency and mechanical advantage in the chisel-tooth and scratch-digger genus Ctenomys (Caviomorpha, Rodentia). Zoology 125, 53–68. doi: 10.1016/j.zool.2017.08.003
Fernandez, V., Abdala, F., Carlson, K. J., Cook, D. C., Rubidge, B. S., Yates, A., et al. (2013). Synchrotron reveals early Triassic odd couple: injured amphibian and aestivating therapsid share burrow. PLoS One 8:e64978. doi: 10.1371/journal.pone.0064978
Fröbisch, J., and Reisz, R. R. (2009). The late Permian herbivore Suminia and the early evolution of arboreality in terrestrial vertebrate ecosystems. Proc. R. Soc. B 276, 3611–3618. doi: 10.1098/rspb.2009.0911
Gans, C. A. (1960). Studies on amphisbaenids (Amphisbaenia, Reptilia). 1. A taxonomic revision of the Trogonophinae, and a functional interpretation of the amphisbaenid adaptive pattern. Bull. Am. Mus. Nat. Hist. 119, 129–203.
Gans, C. A. (1973). Locomotion and burrowing in limbless vertebrates. Nature 242, 414–415. doi: 10.1038/242414a0
Gans, C. A. (1974). Biomechanics: An Approach to Vertebrate Biology. Philadelphia, PA: J. B. Lippincott Co.
Gans, C. A. (1978). The characteristics and affinities of the Amphisbaenia. Trans. Zool. Soc. Lond. 34, 347–416. doi: 10.1111/j.1096-3642.1978.tb00376.x
Gans, C. A., and Montero, R. (2008). “An atlas of amphisbaenian skull anatomy,” in Biology of the Reptilia, eds C. A. Gans, A. S. Gaunt, and K. Adler (Ithaca, NY: Society for the Study of Amphibians and Reptiles), 621–738.
Goswami, A., and Prochel, J. (2007). Ontogenetic morphology and allometry of the cranium in the common European mole (Talpa europaea). J. Mammal. 88, 667–677. doi: 10.1644/06-mamm-a-315r.1
Heffner, R. S., Heffner, H. E., Contos, C., and Kearns, D. (1994). Hearing in prairie dogs: transition between surface and subterranean rodents. Hear. Res. 73, 185–189. doi: 10.1016/0378-5955(94)90233-x
Herring, S. W. (1972). Sutures—a tool in functional cranial analysis. Acta Anat. 83, 222–247. doi: 10.1159/000143860
Herring, S. W. (1974). A biometric study of suture fusion and skull growth in peccaries. Anat. Embryol. 146, 167–180. doi: 10.1007/bf00315593
Herring, S. W. (2008). “Mechanical influences on suture development and patency,” in Craniofacial Sutures. Development, Disease and Treatment, ed. D. P. Rice (Basel: Karger), 41–56. doi: 10.1159/000115031
Herring, S. W., and Mucci, R. J. (1991). In vivo strain in cranial sutures: the zygomatic arch. J. Morphol. 207, 225–239. doi: 10.1002/jmor.1052070302
Herring, S. W., and Ochareon, P. (2005). Bone—special problems of the craniofacial region. Orthod. Craniofac. Res. 8, 174–182. doi: 10.1111/j.1601-6343.2005.00328.x
Herring, S. W., and Teng, S. (2000). Strain in the braincase and its sutures during function. Am. J. Phys. Anthropol. 112, 575–593. doi: 10.1002/1096-8644(200008)112:4<575::aid-ajpa10>3.0.co;2-0
Hildebrand, M. (1985). “Digging in quadrupeds,” in Functional Vertebrate Morphology, eds M. Hildebrand, D. M. Bramble, K. F. Liem, and D. B. Wake (Cambridge, MA: Harvard University Press), 89–109. doi: 10.4159/harvard.9780674184404.c6
Jarvis, J. U. M. (1981). Eusociality in a mammal: cooperative breeding in naked mole-rat colonies. Science 212, 571–573. doi: 10.1126/science.7209555
Jasinoski, S. C., Cluver, M. A., Chinsamy, A., and Reddy, B. D. (2014). “Anatomical plasticity in the snout of Lystrosaurus,” in Early Evolutionary History of the Synapsida, eds C. F. Kammerer, K. D. Angielczyk, and J. Fröbisch (Berlin: DeGruyter), 139–149. doi: 10.1007/978-94-007-6841-3_8
Jasinoski, S. C., Rayfield, E. J., and Chinsamy, A. (2009). Comparative feeding biomechanics of Lystrosaurus and the generalized dicynodont Oudenodon. Anat. Rec. 292, 862–874.
Jasinoski, S. C., Rayfield, E. J., and Chinsamy, A. (2010a). Functional implications of dicynodont cranial suture morphology. J. Morphol. 271, 705–728.
Jasinoski, S. C., Rayfield, E. J., and Chinsamy, A. (2010b). Mechanics of the scarf premaxilla-nasal suture in the snout of Lystrosaurus. J. Vertebr. Paleontol. 30, 1283–1288. doi: 10.1080/02724634.2010.483556
Jasinoski, S. C., Reddy, B. D., Louw, K. K., and Chinsamy, A. (2010c). Mechanics of cranial sutures using the finite element method. J. Biomech. 43, 3104–3111. doi: 10.1016/j.jbiomech.2010.08.007
Jaslow, C. A. (1989). Sexual dimorphism of cranial suture complexity in wild sheep (Ovis orientalis). Zool. J. Linn. Soc. 95, 273–284. doi: 10.1111/j.1096-3642.1989.tb02312.x
Jaslow, C. R. (1990). Mechanical properties of cranial sutures. J. Biomech. 23, 313–321. doi: 10.1016/0021-9290(90)90059-c
Jaslow, C. R., and Biewener, A. A. (1995). Strain patterns in the horncores, cranial bones and sutures of goats (Capra hircus) during impact loading. J. Zool. 235, 193–210. doi: 10.1111/j.1469-7998.1995.tb05137.x
Jaslow, C. R., and Lanier, B. (2001). Changes in rat cranial suture morphology. Rhodes J. Biol. Sci. 48, 14–27.
Johnson, W. C., and Collinge, S. K. (2004). Landscape effects on black-tailed prairie dog colonies. Biol. Conserv. 115, 487–497. doi: 10.1016/s0006-3207(03)00165-4
Kammerer, C. F., Angielczyk, K. D., and Fröbisch, J. (2011). A comprehensive taxonomic revision of Dicynodon (Therapsida, Anomodontia), and its implications for dicynodont phylogeny, biogeography, and biostratigraphy. Soc. Vertebr. Paleontol. Mem. 11, 1–158. doi: 10.1080/02724634.2011.627074
Kammerer, C. F., Angielczyk, K. D., and Fröbisch, J. (2015). Redescription of the geikiid Pelanomodon (Therapsida, Dicynodontia), with a reconsideration of ‘Propelanomodon’. J. Vertebr. Paleontol. 36:e1030408. doi: 10.1080/02724634.2015.1030408
Kammerer, C. F., Bandyopadhyay, S., and Ray, T. (2016). A new taxon of cistecephalid dicynodont from the upper Permian Kundaram Formation of India. Pap. Palaeontol. 2, 569–584. doi: 10.1002/spp2.1055
Kammerer, C. F., Fröbisch, J., and Angielczyk, K. D. (2013). On the validity and phylogenetic position of Eubrachiosaurus browni, a kannemeyeriiform dicynodont (Anomodontia) from Triassic North America. PLoS One 8:e64203. doi: 10.1371/journal.pone.0064203
Kazi, S., and Hipsley, C. A. (2018). Conserved evolution of skull shape in Caribbean head-first burrowing worm lizards (Squamata: Amphisbaenia). Biol. J. Linn. Soc. 125, 14–29. doi: 10.1093/biolinnean/bly086
Keyser, A. W. (1973). A preliminary study of the type area of the Cistecephalus Zone of the Beaufort Series, and a revision of the anomodont family Cistecephalidae. Geol. Surv. S. Afr. Mem. 61, 1–72.
King, G. M. (1981). The functional anatomy of a Permian dicynodont. Phil. Trans. R. Soc. Lond. B 291, 243–322. doi: 10.1098/rstb.1981.0001
King, G. M. (1988). Handbuch der Paläoherpetologie. Teil 17C: Anomodontia. Stuttgart: Gustav Fischer Verlag.
King, G. M., and Cluver, M. A. (1990). The aquatic Lystrosaurus: an alternative lifestyle. Hist. Biol. 4, 323–341.
Kleinteich, T., Maddin, H. C., Herzen, J., Beckmann, F., and Summers, A. P. (2012). Is solid always best? Cranial performance in solid and fenestrated caecilian skulls. J. Exp. Biol. 215, 833–844. doi: 10.1242/jeb.065979
Kopher, R. A., and Mao, J. J. (2003). Suture growth modulated by the oscillatory component of micromechanical strain. J. Bone Miner. Res. 18, 521–528. doi: 10.1359/jbmr.2003.18.3.521
Kümmell, S. B., and Frey, E. (2012). What digits tell us about digging, running and climbing in recent and fossil Synapsida. Fundamental 20, 117–119.
Laaß, M. (2014). Bone-conduction hearing and seismic sensitivity of the late Permian anomodont Kawingasaurus fossilis. J. Morphol. 276, 121–143. doi: 10.1002/jmor.20325
Laaß, M., and Kaestner, A. (2017). Evidence for convergent evolution of a neocortex-like structure in a late Permian therapsid. J. Morphol. 278, 1033–1057. doi: 10.1002/jmor.20712
Latimer, E. M., Gow, C. E., and Rubidge, B. S. (1995). Dentition and feeding niche of Endothiodon (Synapsida; Anomodontia). Palaeontol. Afr. 32, 75–82.
Lenton, K. A., Nacamuli, R. P., Wan, D. C., Helms, J. A., and Longaker, M. T. (2005). Cranial suture biology. Curr. Top. Dev. Biol. 66, 287–328.
Loy, A., Beolchini, F., Martullo, S., and Capanna, E. (1994). Territorial behaviour of Talpa romana in an olivegrove habitat in central Italy. Ital. J. Zool. 61, 207–211. doi: 10.1080/11250009409355887
Lungmus, J. K., Angielczyk, K. D., Sidor, C. A., Nesbitt, S. J., Smith, R. M., Steyer, J.-S., et al. (2015). A new cistecephalid dicynodont (Therapsida, Anomodontia) from the mid-Zambezi basin (Zambia) and its fossorial adaptations. J. Vertebr. Paleontol. Programs Abstr. 2015:169.
Maisch, M. W. (2003). Lower jaw morphology and jaw adductor musculature of the giant Permian dicynodont Rhachiocephalus Seeley, 1898 (Therapsida) from the late Permian of Tanzania. Geol. Paleontol. 37, 89–106.
Maisch, M. W., and Gebauer, E. V. I. (2005). Reappraisal of Geikia locusticeps (Therapsida: Dicynodontia) from the upper Permian of Tanzania. Palaeontology 48, 309–324. doi: 10.1111/j.1475-4983.2005.00451.x
Marilao, L. M., Kulik, Z. T., and Sidor, C. A. (2020). Histology of the preparietal: a neomorphic cranial elements in dicynodont therapsids. J. Vertebr. Paleontol. 40:e1770775. doi: 10.1080/02724634.2020.1770775
Modesto, S. P., and Botha-Brink, J. (2010). A burrow cast with Lystrosaurus skeletal remains from the lower Triassic of South Africa. Palaios 25, 274–281. doi: 10.2110/palo.2009.p09-077r
Modesto, S. P., Rubidge, B. S., and Welman, J. (2002). A new dicynodont therapsid from the lowermost Beaufort Group, upper Permian of South Africa. Can. J. Earth Sci. 39, 1755–1765. doi: 10.1139/e02-091
Mumby, H. S., Chapman, S. N., Crawley, J. A. H., Mar, K. U., Htut, W., Soe, A. T., et al. (2015). Distinguishing between determinate and indeterminate growth in a long-lived mammal. BMC Evol. Biol. 15:214. doi: 10.1186/s12862-015-0487-x
Nasterlack, T., Canoville, A., and Chinsamy, A. (2012). New insights into the biology of the Permian genus Cistecephalus (Therapsida, Dicynodontia). J. Vertebr. Paleontol. 32, 1396–1410. doi: 10.1080/02724634.2012.697410
Navas, C. A., Antoniazzi, M. M., Carvalho, J. E., Chaui-Berlink, J. G., James, R. S., Jared, C., et al. (2004). Morphological and physiological specialization for digging in amphisbaenians, an ancient lineage of fossorial vertebrates. J. Exp. Biol. 207, 2433–2441. doi: 10.1242/jeb.01041
Olson, E. C. (1944). Origin of mammals based upon the cranial morphology of the therapsid suborders. Spec. Pap. Geol. Soc. Am. 55, 1–136. doi: 10.1130/spe55-p1
Owen, R. (1876). Descriptive and Illustrated Catalogue of the Fossil Reptilia of South Africa in the Collection of the British Museum. London: Taylor and Francis.
Pardo, J. D., and Anderson, J. S. (2016). Cranial morphology of the Carboniferous-Permian tetrapod Brachydectes newberryi (Lepospondyli, Lysorophia): new data from μCT. PLoS One 11:e0161823. doi: 10.1371/journal.pone.0161823
Rabosky, D. L., Grundler, M., Anderson, C., Title, P., Shi, J. J., Brown, J. W., et al. (2014). BAMMtools: an R package for the analysis of evolutionary dynamics on phylogenetic trees. Methods Ecol. Evol. 5, 701–707. doi: 10.1111/2041-210x.12199
Rafferty, K. L., and Herring, S. W. (1999). Craniofacial sutures: morphology, growth, and in vivo masticatory strains. J. Morphol. 242, 167–179. doi: 10.1002/(sici)1097-4687(199911)242:2<167::aid-jmor8>3.0.co;2-1
Ray, S., Bandyopadhyay, S., and Bhawal, D. (2009). Growth patterns as deduced from bone microstructure of some selected neotherapsids with special emphasis on dicynodonts: phylogenetic implications. Palaeoworld 18, 53–66. doi: 10.1016/j.palwor.2008.09.001
Ray, S., and Chinsamy, A. (2003). Functional aspects of the postcranial anatomy of the Permian dicynodont Diictodon and their ecological implications. Palaeontology 46, 151–183. doi: 10.1111/1475-4983.00292
Renaut, A. J. (2001). Dicynodont jaw mechanisms reconsidered: the Kannemeyeria (Anomodontia Therapsida) masticatory cycle. Asoc. Paleontol. Arg. Pub. Esp. 7, 167–170.
Rieppel, O. (1981). The skull and jaw adductor musculature in some burrowing scincomorph lizards of the genera Acontias, Typhlosaurus, and Feylinia. J. Zool. 195, 493–528. doi: 10.1111/j.1469-7998.1981.tb03480.x
Rieppel, O., Kley, N. J., and Maisano, J. A. (2009). Morphology of the skull of the white-nosed blindsnake, Liotyphlops albirostris (Scolecophidia: Anomalepididae). J. Morphol. 270, 536–557. doi: 10.1002/jmor.10703
Rubidge, B. S., and Day, M. O. (2020). Biostratigraphy of the Eodicynodon Assemblage Zone (Beaufort Group, Karoo Supergroup), South Africa. S. Afr. J. Geol. 123, 141–148. doi: 10.25131/sajg.123.0010
Samuels, J. X., and Van Valkenburgh, B. (2009). Craniodental adaptations for digging in extinct burrowing beavers. J. Vertebr. Paleontol. 29, 254–268. doi: 10.1080/02724634.2009.10010376
Sánchez-Villagra, M. R. (2010). Developmental palaeontology in synapsids: the fossil record of ontogeny in mammals and their closest relatives. Proc. R. Soc. B 277, 1139–1147. doi: 10.1098/rspb.2009.2005
Slater, B. J., Lenton, K. A., Kwan, M. D., Gupta, D. M., Wan, D. C., and Longaker, M. T. (2008). Cranial sutures: a brief review. Plast. Reconstr. Surg. 121, 170e–178e.
Smith, R. M. H. (1987). Helical burrow casts of therapsid origin from the beaufort group (Permian) of South Africa. Palaeogeogr. Palaeoclimatol. Palaeoecol. 60, 155–170. doi: 10.1016/0031-0182(87)90030-7
Smith, R. M. H. (2020). Biostratigraphy of the Cistecephalus Assemblage Zone (Beaufort Group, Karoo Supergroup), South Africa. S. Afr. J. Geol. 123, 181–190. doi: 10.25131/sajg.123.0013
Smith, R. M. H., Angielczyk, K. D., Benoit, J., and Fernandez, V. (2021). Neonate aggregation in the Permian dicynodont Diictodon (Therapsida, Anomodontia): evidence for a reproductive function for burrows? Palaeogeogr. Palaeoclimatol. Palaeoecol. 569:110311. doi: 10.1016/j.palaeo.2021.110311
Sollas, I. B. J., and Sollas, W. J. (1914). A study of the skull of Dicynodon by means of serial sections. Phil. Trans. R. Soc. Lond. B. 204, 201–225. doi: 10.1098/rstb.1914.0006
Sollas, I. B. J., and Sollas, W. J. (1916). On the structure of the dicynodont skull. Phil. Trans. R. Soc. Lond. B. 207, 531–539. doi: 10.1098/rstb.1916.0012
Sulej, T., and Niedźwiedzki, G. (2019). An elephant-sized late Triassic synapsid with erect limbs. Science 363, 78–80. doi: 10.1126/science.aal4853
Surkov, M. V., and Benton, M. J. (2008). Head kinematics and feeding adaptations of the Permian and Triassic dicynodonts. J. Vertebr. Paleontol. 28, 1120–1129. doi: 10.1671/0272-4634-28.4.1120
Keywords: Synapsida, Dicynodontia, Permian, Triassic, sutures, fossoriality, functional anatomy
Citation: Kammerer CF (2021) Elevated Cranial Sutural Complexity in Burrowing Dicynodonts. Front. Ecol. Evol. 9:674151. doi: 10.3389/fevo.2021.674151
Received: 28 February 2021; Accepted: 21 June 2021;
Published: 27 July 2021.
Edited by:
Jörg Fröbisch, Museum of Natural History Berlin (MfN), GermanyReviewed by:
Johannes Müller, Museum of Natural History Berlin (MfN), GermanyCopyright © 2021 Kammerer. This is an open-access article distributed under the terms of the Creative Commons Attribution License (CC BY). The use, distribution or reproduction in other forums is permitted, provided the original author(s) and the copyright owner(s) are credited and that the original publication in this journal is cited, in accordance with accepted academic practice. No use, distribution or reproduction is permitted which does not comply with these terms.
*Correspondence: Christian F. Kammerer, Y2hyaXN0aWFuLmthbW1lcmVyQG5hdHVyYWxzY2llbmNlcy5vcmc=
Disclaimer: All claims expressed in this article are solely those of the authors and do not necessarily represent those of their affiliated organizations, or those of the publisher, the editors and the reviewers. Any product that may be evaluated in this article or claim that may be made by its manufacturer is not guaranteed or endorsed by the publisher.
Research integrity at Frontiers
Learn more about the work of our research integrity team to safeguard the quality of each article we publish.