- 1The Peregrine Fund, Boise, ID, United States
- 2Endangered Wildlife Trust, Glen Austin, South Africa
- 3U.S. Geological Survey, Boise, ID, United States
Energy infrastructure, particularly for wind power, is rapidly expanding in Africa, creating the potential for conflict with at-risk wildlife populations. Raptor populations are especially susceptible to negative impacts of fatalities from wind energy because individuals tend to be long-lived and reproduce slowly. A major determinant of risk of collision between flying birds and wind turbines is the altitude above ground at which a bird flies. We examine 18,710 observations of flying raptors recorded in southern Africa and we evaluate, for 49 species, the frequency with which they were observed to fly at the general height of a wind turbine rotor-swept zone (50–150 m). Threatened species, especially vultures, were more likely to be observed at turbine height than were other species, suggesting that these raptors are most likely to be affected by wind power development across southern Africa. Our results highlight that threatened raptor species, particularly vultures, might be especially impacted by expanded wind energy infrastructure across southern Africa.
Introduction
Globally, wind energy has expanded substantially in past decades (Wiser et al., 2011; Energy Information Agency, 2020; Global Wind Energy Council, 2020). Particularly in Africa, the quantity of energy generated from wind power has increased, with potential for expansion across the continent (Mentis et al., 2015; Mas’ud et al., 2017; Kazimierczuk, 2019). The Global Wind Energy Council (2020) predicted that wind energy production in Africa will more than double over the period from 2018 to 2023.
There are considerable ecological challenges to the expansion of wind energy (Katzner et al., 2019). Among the most prominent of these is wildlife fatality from collisions with turbines (Arnett et al., 2008; Smallwood and Thelander, 2008; Loss et al., 2013). Even if animals can avoid collision by adjusting their use of space, such avoidance of turbines results in a functional loss of habitat (Larsen and Guillemette, 2007; Diehl, 2013; Marques et al., 2020).
Raptors (Iriarte et al., 2019; McClure et al., 2019) are of particular conservation concern because they are more threatened and declining than other groups of birds (McClure and Rolek, 2020). Unfortunately, compared to other bird taxa, raptors are especially susceptible to negative impacts of wind energy because they are often victims of fatal collisions (Thaxter et al., 2017). These birds tend to be long-lived and reproduce slowly (Newton, 1998; Madders and Whitfield, 2006; Smith and Dwyer, 2016; Watson et al., 2018), thus survival rates tend to drive population trajectories (Newton, 1979; Sæther and Bakke, 2000). Indeed, collision at wind turbines creates population-level risk for species as diverse as Egyptian Vultures (Carrete et al., 2009) and Red Kites (Schaub, 2012).
Africa is a hotspot of threatened and declining raptors (McClure et al., 2018b). Accipitrid vultures are among the most threatened groups of birds on the planet (Buechley and Şekercioğlu, 2016; Ogada et al., 2016; McClure and Rolek, 2020), but there are also a suite of other declining species throughout Africa (McClure et al., 2018b). Within South Africa, diurnal raptors in the families Accipitriformes and Falconiformes are the group of birds most often killed by wind turbines (Perold et al., 2020).
Whether a given turbine affects a flying animal depends on that animal’s use of three dimensional space. Indeed, flight altitude is an important determinant of collision risk (Band et al., 2007; Furness et al., 2013; Khosravifard et al., 2020). For example, Poessel et al. (2018) demonstrated that Critically Endangered California Condors (Gymnogyps californianus) more frequently flew at altitudes of wind turbine blades at specific times of the day and the year. Thus their flight behavior modulates their risk of collision with wind turbines (Poessel et al., 2018). Examining flight altitude is frequently used to lend inference into risk of collision with wind turbines (Katzner et al., 2012; Johnston A. et al., 2014; Ainley et al., 2015; Péron et al., 2017; Tikkanen et al., 2018).
Perhaps the most accurate method of determining flight altitude is via high-resolution global positioning system (GPS; Schaub et al., 2020) or barometric altimeters (Cleasby et al., 2015; but see Péron et al., 2020). However, a more commonly used method to determine flight altitude, especially in relation to turbine height, is visual estimation by human observers (Osborn et al., 1998; Larsen and Guillemette, 2007; Rothery et al., 2009; Smallwood et al., 2009; Dahl et al., 2013; Johnston A. et al., 2014; Johnston N. et al., 2014; Ainley et al., 2015). In fact, a recent review demonstrated that; of methods to discern bird flight altitude, visual methods were by far the most often used (Largey et al., 2021).
Here, we analyze thousands of observations of raptors flying in southern Africa. We calculate the proportion of observations in which each species was seen at the height of a typical wind turbine and evaluate which taxa are more often observed at turbine height. We also test whether threatened species are more often observed at turbine height than non-threatened species, and if scavenging vultures were more often observed at turbine height than were other raptors.
Materials and Methods
We evaluated flight altitudes from observation records stored in the Global Raptor Impact Network (GRIN) database (sourced 2 September, 2020). GRIN is a collaborative information platform designed to support raptor ecology and conservation by collecting, storing, analyzing, and distributing information about raptors (McClure et al., 2021a).1 The majority of the data within the GRIN database were collected using one of two smartphone applications [hereafter, apps; either the GRIN mobile app (2020–present), or its deprecated predecessor the African Raptor Observations app (2012–2020)]. These apps allow users to record georeferenced observations of raptors including the species, whether the bird is flying, and at what estimated altitude. Most of the users of these apps are raptor biologists.
Altitude above ground was recorded by users of the GRIN and African Raptor Observations apps into categories of 0, 1–20, 20–50, 50–150, 150–500, and >500 m. Users were given references for each altitude category as ground level, low tree, tall tree, turbine risk, low altitude, and high altitude, respectively. We assumed that using such categories lessens some of the error associated with estimating altitude.
Users can log their confidence in each species identification on a scale of 0–100%; for this analysis, we only analyzed observations with 100% confidence in identification. We also only considered observations of raptors flying. We considered data from all seasons for each species for which there were ≥25 flight observations within southern (≤−17.5° latitude) Africa. For each of those species, we calculated the category in which they were most often observed (i.e., the mode) and the proportion of observations at turbine height (50–150 m).
We used logistic regression to test whether the proportion of observations approximately at the height of the rotor-swept zone of modern turbines (50–150 m) was greater for species designated as threatened with extinction on the International Union for the Conservation of Nature’s (IUCN) Red List of Threatened Species (BirdLife International, 2021). We categorized species as threatened when listed by IUCN as Vulnerable, Endangered, or Critically Endangered (IUCN Standards and Petitions Subcommittee, 2019). The response variable for this regression included two vectors, one containing the number of observations at turbine height and the other containing the number of observations outside of turbine height. The explanatory variable was binary with 1 indicating a threatened species and 0 indicating a non-threatened species. To analyze whether vultures were observed more at turbine height than other raptors, we performed another similar logistic regression where we coded the explanatory variable as 1 when the species was a vulture and a 0 otherwise. We performed analysis using the glm() function in R (R Core Team, 2019) with a binomial distribution.
Results
Within our study area, there were 111,024 observations of raptors; 62,911 observations were collected using the apps; and 19,061 were of flying raptors. Of these, we analyzed the 18,710 observations that had 100% confidence in identification and were of the 49 species with ≥25 observations (Figures 1,2). These were collected by 73 users, although one (author AB) collected a plurality of the data (39%). Proportions of observations at turbine height ranged from 0.49 for the Lappet-faced Vulture (Torgos tracheliotos) to 0.02 for the Barn Owl (Tyto alba; Figure 2 and Supplementary Tables 1,2). Nine (18%) species, including five vulture and three eagle species, had the mode of their observations at turbine height, and one species had a mode above turbine height (Figure 2 and Supplementary Tables 1,2).
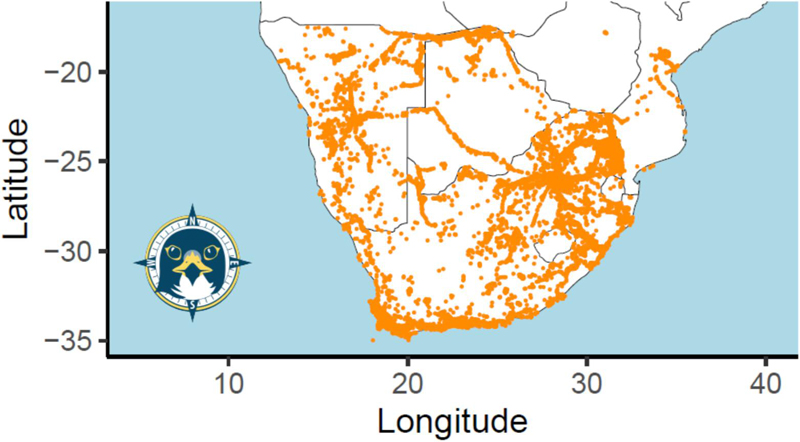
Figure 1. Locations of observations of flying raptors (orange points) in southern Africa within the Global Raptor Impact Network database.
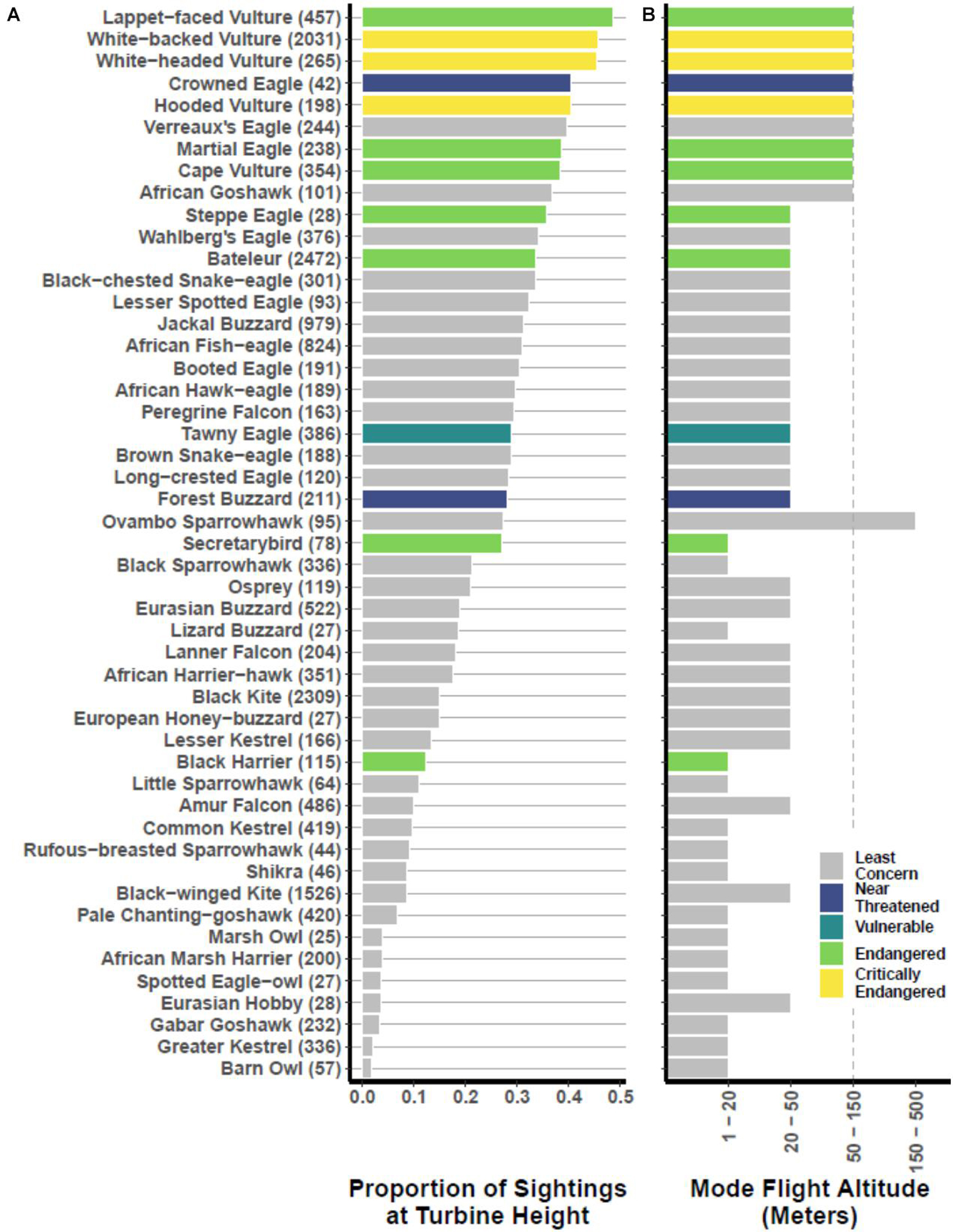
Figure 2. (A) Proportion of observations of African raptor species that were flying at turbine height (50–150 m). Sample sizes are listed parenthetically. (B) The flight altitude category in which each species was most recorded (mode). Vertical dashed line represents maximum of the range of turbine heights. Colors represent the conservation status of each species. See Supplementary Table 1 for scientific names.
Of the 49 species, 11 met our criteria for ‘threatened’; five of these were vultures. The nine species with the highest proportions of observations at turbine height also had modes at turbine height and six of these species were threatened (Figure 2 and Supplementary Tables 1,2). Threatened species (β = 0.97, SE = 0.03, p < 0.01) and vultures (β = 1.08, SE = 0.03, p < 0.01) were observed proportionally more at turbine height than non-threatened species and non-vultures, respectively.
Discussion
Our results provide initial insight into the set of raptor species that may be affected by wind power development within southern Africa. However, if the two-dimensional distribution of birds and turbines do not overlap, then collision risk is essentially zero. Some studies have identified areas of potential conflict and for potential prioritization for conservation by examining the spatial overlap of potential wind power development with two-dimensional species ranges (Santangeli et al., 2018, 2019). That said, within a species distribution, collisions with wind turbines occur in three-dimensions such that a bird must be within both the horizontal and vertical planes of a rotor-swept zone. We therefore interpret our results under the assumption that species seen more often at rotor-swept zone height are at increased risk of collision. Our results thus add perspective to two-dimensional distributional studies by highlighting which species behavior might influence their demographic risk from wind power development within their southern African range.
Populations of vultures are declining drastically across Africa (Ogada et al., 2016), and these species were among those for which the greatest proportion of observations were at turbine height. When African vultures are evaluated for conservation purposes, wind energy is only rarely considered a threat (BirdLife International, 2021). In fact, of the 11 threatened species we considered, the IUCN Red List (BirdLife International, 2021) identifies renewable energy as a threat for only one vulture and two eagles (Cape Vulture Gyps coprotheres, Steppe Eagle Aquila nipalensis, and Crowned Eagle Stephanoaetus coronatus). However, the Multi-species Action Plan to Conserve African-Eurasian Vultures (Botha et al., 2017), prominently lists collisions with wind turbines as a threat to vultures. It is possible that the Red List underestimates the threat wind power poses to raptors in Africa (Santangeli et al., 2018), or it is possible that wind energy is such a novel threat that it has not yet been incorporated into these assessments.
The number of existing wind power facilities across Africa is unlikely to be large enough to be threatening most raptor populations at a large scale. That said, despite the lack of a current threat from existing wind turbines, our approach is useful because it identifies species that might be at risk if such infrastructure expands into their habitat. Horizon scanning—i.e., systematically searching for potential threats and opportunities—is important (Sutherland and Woodroof, 2009) and our evaluation of flight altitude adds to prior work on this subject (Santangeli et al., 2018, 2019). The fact that threatened species were observed more often at turbine height suggests that expansion of wind power across southern Africa might expose more of these African raptor species to increased mortality.
Many factors determine the flight altitude of a bird at any given moment. Such factors include topography, vegetation, weather, wind conditions, and time of day or year (Lanzone et al., 2012; Poessel et al., 2018; Tikkanen et al., 2018; Duerr et al., 2019). Despite its utility as a first analysis of risk, our analyses ignore many of these factors. Future research, therefore, could discern other patterns in our dataset that would provide further inference into raptor flight behavior.
Site-specific factors also influence a bird’s risk of collision within a wind power facility (de Lucas et al., 2008). Indeed, certain turbines present more collision risk than others (McClure et al., 2021b), even under low bird densities (de Lucas et al., 2012). Weak relationships between pre-construction abundance and post-construction mortality at wind power facilities make it difficult to predict where collision risk will be greatest (Ferrer et al., 2012). The flight paths of Griffon Vultures through a wind power facility in Spain matched predominant wind flows (de Lucas et al., 2012). Thus, flight altitude is but one factor of the many that determine the collision risk of birds with wind turbines (Marques et al., 2014).
Any study is subject to some error, especially when using human observers. Our methodology minimized errors by relying to a large part on data collection by raptor biologists and by classifying observations into broad altitudinal ranges. Human ability to identify raptors declines with distance (McClure et al., 2018a), but we avoid this problem to some extent by only including data in which the user was 100% confident in identification. Detection probability also decreases with distance (Berthiaume et al., 2009; Nolte et al., 2016; McClure et al., 2018a), meaning that many species probably spend more time at greater altitudes than our results suggest. Importantly, large birds are easier to detect than smaller ones at greater distances (Nolte et al., 2016). Because threatened species were mostly vultures and eagles, which are relatively large, it is possible that they are observed more often at turbine height than other species because they are more detectable at that altitude. Because of these constraints, we interpret our results in the context of these limitations and for bird flight within the range of human sight. Our work says little about high altitude bird flight that is difficult for humans to observe from the ground.
Despite these potential sources of error, our results match what one would expect a priori based on the biology of many focal species. For example, kestrels and harriers are known to fly or hover close to the ground, whereas vultures and eagles are soaring birds that fly at greater altitude (Ferguson-Lees and Christie, 2001). Barn Owls (Tyto alba) were observed the least at turbine height and were most often observed flying at low altitude (1–20 m). It is possible this is partly due to the inability of observers to see far at night when owls are active. However, Barn Owls most often forage by quartering low (1.5–4.5 m; Marti et al., 2020) over open areas (König and Weick, 2008; Marti et al., 2020). This species is also a frequent victim of vehicle collision (Gomes et al., 2009; Boves and Belthoff, 2012; Arnold et al., 2019), reflecting their low-flying behavior. We were surprised that the Ovambo Sparrowhawk (Accipiter ovampensis), a relatively small woodland-dwelling accipiter (Ferguson-Lees and Christie, 2001), had the highest mode flight altitude. However, this species sometimes forages by stooping from high over the canopy (Kemp and Kemp, 1975; Kemp and Kirwan, 2020). Ferguson-Lees and Christie (2001, p. 576), state that this species “stoops at prey from up to 150 m.” Thus, past observations of these species support our results.
There is a paucity of quantitative work examining the flight altitudes of our focal species. Indeed, we are aware of a single study that we can use to quantitatively check our results. In South Africa, Murgatroyd et al. (2021) used GPS tracking to demonstrate that Verreaux’s Eagles (Aquila verreauxii) fly at turbine height (defined as ≤200 m) 68 ± 4% of the time. This is consistent with the data from GRIN, in which Verreaux’s Eagles were seen flying at or below turbine height 73% of the time. Thus, although error estimation by human observers is imprecise, the data for this species are consistent with existing GPS data. Given the prevalence of studies using visual estimation of flight altitude (Largey et al., 2021), a fruitful avenue for future work would therefore be to compare our observations with results from GPS telemetry.
This is the first study to examine the flight altitudes of so many species across such a large region. As the number of users of the GRIN app rises, future work will expand the current analysis to a global scale. Although Africa is certainly a hotspot of raptor diversity and conservation need, other areas including South America and South and Southeast Asia are also priorities (McClure et al., 2018b). Study of flight altitude of raptors in those understudied regions, whether via the GRIN app or otherwise, would contribute to understanding of the potential risk these birds may face from wind energy development. This may be especially important given the height of wind turbines is projected to grow with time, and already some turbines have blades that extend above the 150 m we considered here. Works such as this are an important component of efforts to encourage compatibility of energy development with conservation of biodiversity.
Data Availability Statement
The original contributions presented in the study are included in the article/Supplementary Material, further inquiries can be directed to the corresponding author.
Ethics Statement
Ethical review and approval was not required for the animal study because we did not handle any animals.
Author Contributions
CM devised the study, performed analysis, and wrote the first draft. LD managed the data. All authors provided substantial input in interpretation of results and edited the manuscript.
Funding
The M. J. Murdock Charitable Trust and the author’s institutions funded this study.
Conflict of Interest
The authors declare that the research was conducted in the absence of any commercial or financial relationships that could be construed as a potential conflict of interest.
Publisher’s Note
All claims expressed in this article are solely those of the authors and do not necessarily represent those of their affiliated organizations, or those of the publisher, the editors and the reviewers. Any product that may be evaluated in this article, or claim that may be made by its manufacturer, is not guaranteed or endorsed by the publisher.
Acknowledgments
We thank Rob Davies and Habitat Info, Ltd., for building and maintaining the African Raptor DataBank and the GRIN app. We especially thank the users of the GRIN and African Raptor Observations apps. Any use of trade, firm, or product names is for descriptive purposes only and does not imply endorsement by the U.S. Government.
Supplementary Material
The Supplementary Material for this article can be found online at: https://www.frontiersin.org/articles/10.3389/fevo.2021.667384/full#supplementary-material
Footnotes
References
Ainley, D. G., Porzig, E., Zajanc, D., and Spear, L. B. (2015). Seabird flight behavior and height in response to altered wind strength and direction. Mar. Ornithol. 43, 25–36.
Arnett, E. B., Brown, W. K., Erickson, W. P., Fiedler, J. K., Hamilton, B. L., Henry, T. H., et al. (2008). Patterns of bat fatalities at wind energy facilities in North America. J. Wildl. Manage. 72, 61–78. doi: 10.2193/2007-221
Arnold, E. M., Hanser, S. E., Regan, T., Thompson, J., Lowe, M., Kociolek, A., et al. (2019). Spatial, road geometric and biotic factors associated with Barn Owl mortality along an interstate highway. IBIS 161, 147–161. doi: 10.1111/ibi.12593
Band, W., Madders, M., and Whitfield, D. P. (2007). “Developing field and analytical methods to assess avian collision risk at wind farms,” in Birds and Wind Farms: risk Assessment and Mitigation, eds M. Lucas, G. F. E. Janss, and M. Ferrer (Madrid: Quercus), 259–275.
Berthiaume, É., Bélisle, M., and Savard, J.-P. (2009). Incorporating detectability into analyses of population trends based on hawk counts: a double-observer approach. Condor 111, 43–58. doi: 10.1525/cond.2009.080081
BirdLife International (2021). IUCN Red List for birds. Available Online at: http://www.birdlife.org (accessed on 12 January, 2021).
Botha, A., Andevski, J., Bowden, C. G. R., Gudka, M., Safford, R., and Williams, N. P. (2017). Multi-species action plan to conserve African-Eurasian Vultures (Vultures MSAP):CMS Raptors MOU Technical Publication No. 4. CMS Technical Series No. 33. Abu Dhabi, United Arab Emirates: Coordinating Unit of the CMS Raptors MOU.
Boves, T. J., and Belthoff, J. R. (2012). Roadway mortality of barn owls in Idaho, USA. J. Wildl. Manage. 76, 1381–1392. doi: 10.1002/jwmg.378
Buechley, E. R., and Şekercioğlu, ÇH. (2016). The avian scavenger crisis: looming extinctions, trophic cascades, and loss of critical ecosystem functions. Biol. Conserv. 198, 220–228. doi: 10.1016/j.biocon.2016.04.001
Carrete, M., Sánchez-Zapata, J. A., Benítez, J. R., Lobón, M., and Donázar, J. A. (2009). Large scale risk-assessment of wind-farms on population viability of a globally endangered long-lived raptor. Biol. Conserv. 142, 2954–2961. doi: 10.1016/j.biocon.2009.07.027
Cleasby, I. R., Wakefield, E. D., Bearhop, S., Bodey, T. W., Votier, S. C., and Hamer, K. C. (2015). Three-dimensional tracking of a wide-ranging marine predator: flight heights and vulnerability to offshore wind farms. J. Appl. Ecol. 52, 1474–1482. doi: 10.1111/1365-2664.12529
Dahl, E. L., May, R., Hoel, P. L., Bevanger, K., Pedersen, H. C., Røskaft, E., et al. (2013). White-tailed eagles (Haliaeetus albicilla) at the Smøla wind-power plant, central Norway, lack behavioral flight responses to wind turbines. Wildl. Soc. Bull. 37, 66–74. doi: 10.1002/wsb.258
de Lucas, M., Ferrer, M., and Janss, G. F. E. (2012). Using Wind Tunnels to Predict Bird Mortality in Wind Farms: the Case of Griffon Vultures. PLoS One 7:e48092. doi: 10.1371/journal.pone.0048092
de Lucas, M., Janss, G. F. E., Whitfield, D. P., and Ferrer, M. (2008). Collision fatality of raptors in wind farms does not depend on raptor abundance. J. Appl. Ecol. 45, 1695–1703. doi: 10.1111/j.1365-2664.2008.01549.x
Diehl, R. H. (2013). The airspace is habitat. Trends Ecol. Evol. 28, 377–379. doi: 10.1016/j.tree.2013.02.015
Duerr, A. E., Miller, T. A., Dunn, L., Bell, D. A., Bloom, P. H., Fisher, R. N., et al. (2019). Topographic drivers of flight altitude over large spatial and temporal scales. Auk 136, 1–11. doi: 10.1093/auk/ukz002
Energy Information Agency (2020). Electricity data browser. Net generation from wind by state by sector, annual back to 2001. Washington: U.S. Energy Information Administration
Ferguson-Lees, J., and Christie, D. A. (2001). Raptors of the World. Boston, Massachusetts, USA: Houghton Mifflin. doi: 10.1111/j.1557-9263.2006.00078_1.x
Ferrer, M., de Lucas, M., Janss, G. F. E., Casado, E., Muñoz, A. R., Bechard, M. J., et al. (2012). Weak relationship between risk assessment studies and recorded mortality in wind farms. J. Appl. Ecol. 49, 38–46. doi: 10.1111/j.1365-2664.2011.02054.x
Furness, R. W., Wade, H. M., and Masden, E. A. (2013). Assessing vulnerability of marine bird populations to offshore wind farms. J. Environ. Manage. 119, 56–66. doi: 10.1016/j.jenvman.2013.01.025
Global Wind Energy Council (2020). GWEC Global Wind Report 2019. Brussels, Belgium: Global Wind Energy Council.
Gomes, L., Grilo, C., Silva, C., and Mira, A. (2009). Identification methods and deterministic factors of owl roadkill hotspot locations in Mediterranean landscapes. Ecol. Res. 24, 355–370. doi: 10.1007/s11284-008-0515-z
Iriarte, J. A., Rivas-Fuenzalida, T., and Jaksic, F. M. (2019). Las Aves Rapaces De Chile. Santiago, Chile: Ocho Libros.
IUCN Standards and Petitions Subcommittee (2019). Guidelines for Using the IUCN Red List Categories and Criteria. Version 13. Available Online at: http://www.iucnredlist.org/documents/RedListGuidelines.pdf (accessed September 17, 2021).
Johnston, A., Cook, A. S. C. P., Wright, L. J., Humphreys, E. M., and Burton, N. H. K. (2014). Modelling flight heights of marine birds to more accurately assess collision risk with offshore wind turbines. J. Appl. Ecol. 51, 31–41. doi: 10.1111/1365-2664.12191
Johnston, N. N., Bradley, J. E., and Otter, K. A. (2014). Increased flight altitudes among migrating golden eagles suggest turbine avoidance at a rocky mountain wind installation. PLoS One 9:e93030. doi: 10.1371/journal.pone.0093030
Katzner, T. E., Brandes, D., Miller, T., Lanzone, M., Tremblay, J. A., Mulvihill, R., et al. (2012). Topography drives migratory flight altitude of golden eagles: implications for on-shore wind energy development. J. Appl. Ecol. 49, 1178–1186. doi: 10.1111/j.1365-2664.2012.02185.x
Katzner, T. E., Nelson, D. M., Diffendorfer, J. E., Duerr, A. E., Campbell, C. J., Leslie, D., et al. (2019). Wind energy: an ecological challenge. Science 366, 1206–1207.
Kazimierczuk, A. H. (2019). Wind energy in Kenya: a status and policy framework review. Renew. Sustain. Energy Rev. 107, 434–445. doi: 10.1016/j.rser.2018.12.061
Kemp, A. C., and Kemp, M. I. (1975). Observations on the breeding biology of the Ovambo Sparrowhawk, Accipiter ovampensis Accipitridae). Ann. Transvaal Mus. 29, 185–190.
Kemp, A. C., and Kirwan, G. M. (2020). “Ovambo Sparrowhawk (Accipiter ovampensis), version 1.0,” in Birds of the World, eds J. del Hoyo, A. Elliott, J. Sargatal, D. A. Christie, and E. de Juana (Ithaca, NY: Cornell Lab of Ornithology).
Khosravifard, S., Skidmore, A. K., Naimi, B., Venus, V., Muñoz, A. R., and Toxopeus, A. G. (2020). Identifying Birds’ Collision Risk with Wind Turbines Using a Multidimensional Utilization Distribution Method. Wildl. Soc. Bull. 44, 191–199. doi: 10.1002/wsb.1056
Lanzone, M. J., Miller, T. A., Turk, P., Brandes, D., Halverson, C., Maisonneuve, C., et al. (2012). Flight responses by a migratory soaring raptor to changing meteorological conditions. Biol. Lett. 8, 710–713. doi: 10.1098/rsbl.2012.0359
Largey, N., Cook, A. S. C. P., Thaxter, C. B., MCluskie, A., Stokke, B. G., Wilson, B., et al. (2021). Methods to quantify avian airspace use in relation to wind energy development. IBIS 163, 747–764. doi: 10.1111/ibi.12913
Larsen, J. K., and Guillemette, M. (2007). Effects of wind turbines on flight behaviour of wintering common eiders: implications for habitat use and collision risk. J. Appl. Ecol. 44, 516–522. doi: 10.1111/j.1365-2664.2007.01303.x
Loss, S. R., Will, T., and Marra, P. P. (2013). Estimates of bird collision mortality at wind facilities in the contiguous United States. Biol. Conserv. 168, 201–209.
Madders, M., and Whitfield, D. P. (2006). Upland raptors and the assessment of wind farm impacts. IBIS 148, 43–56. doi: 10.1111/j.1474-919X.2006.00506.x
Marques, A. T., Batalha, H., Rodrigues, S., Costa, H., Pereira, M. J. R., Fonseca, C., et al. (2014). Understanding bird collisions at wind farms: an updated review on the causes and possible mitigation strategies. Biol. Conserv. 179, 40–52. doi: 10.1016/j.biocon.2014.08.017
Marques, A. T., Santos, C. D., Hanssen, F., Muñoz, A. R., Onrubia, A., Wikelski, M., et al. (2020). Wind turbines cause functional habitat loss for migratory soaring birds. J. Anim. Ecol. 89, 93–103. doi: 10.1111/1365-2656.12961
Marti, C. D., Poole, A. F., Bevier, L. R., Bruce, M. D., Christie, D. A., Kirwan, G. M., et al. (2020). “Barn Owl (Tyto alba), version 1.0,” in Birds of the World, ed. S. M. Billerman (Ithaca, NY: Cornell Lab of Ornithology), doi: 10.2173/bow.brnowl.01
Mas’ud, A. A., Wirba, A. V., Ardila-Rey, J. A., Albarracín, R., Muhammad-Sukki, F., Duque, ÁJ., et al. (2017). Wind power potentials in Cameroon and Nigeria: lessons from South Africa. Energies 10, 1–19. doi: 10.3390/en10040443
McClure, C. J. W., and Rolek, B. W. (2020). Relative conservation status of bird orders with special attention to raptors. Front. Ecol. Evol. 8:593941. doi: 10.3389/fevo.2020.593941
McClure, C. J. W., Martinson, L., and Allison, T. D. (2018a). Automated monitoring for birds in flight: proof of concept with eagles at a wind power facility. Biol. Conserv. 224, 26–33. doi: 10.1016/j.biocon.2018.04.041
McClure, C. J. W., Westrip, J. R. S., Johnson, J. A., Schulwitz, S. E., Virani, M. Z., Davies, R., et al. (2018b). State of the world’s raptors: distributions, threats, and conservation recommendations. Biol. Conserv. 227, 390–402. doi: 10.1016/j.biocon.2018.08.012
McClure, C. J. W., Schulwitz, S. E., Anderson, D. L., Robinson, B. W., Mojica, E. K., Therrien, J.-F., et al. (2019). Commentary: defining raptors and birds of prey. J. Raptor Res. 53, 419–430.
McClure, C. J. W., Anderson, D. L., Belthoff, J. R., Botha, A., Buechley, E. R., Buij, R., et al. (2021a). Commentary: the past, present, and future of the Global Raptor Impact Network. J. Raptor Res. (in press).
McClure, C. J. W., Rolek, B. W., Braham, M. A., Miller, T. A., Duerr, A. E., Mccabe, J. D., et al. (2021b). Eagles enter rotor-swept zones of wind turbines at rates that vary per turbine. Ecol. Evol. 11, 11267–11274. doi: 10.1002/ece3.7911
Mentis, D., Hermann, S., Howells, M., Welsch, M., and Siyal, S. H. (2015). Assessing the technical wind energy potential in Africa a GIS-based approach. Renew. Energy 83, 110–125. doi: 10.1016/j.renene.2015.03.072
Murgatroyd, M., Bouten, W., and Amar, A. (2021). A predictive model for improving placement of wind turbines to minimise collision risk potential for a large soaring raptor. J. Appl. Ecol. 58, 857–868. doi: 10.1111/1365-2664.13799
Newton, I. (1979). Population Ecology of Raptors. Vermillion, South Dakota, USA: Buteo Books, doi: 10.2307/4081
Nolte, E. G., Bart, J., Pauli, B. P., Kaltenecker, G. S., and Heath, J. A. (2016). Detectability of migrating raptors and its effect on bias and precision of trend estimates. Avian Conserv. Ecol. 11:9. doi: 10.5751/ACE-00894-110209
Ogada, D., Shaw, P., Beyers, R. L., Buij, R., Murn, C., Thiollay, J. M., et al. (2016). Another continental vulture crisis: africa’s vultures collapsing toward extinction. Conserv. Lett. 9, 89–97. doi: 10.1111/conl.12182
Osborn, R. G., Dieter, C. D., Higgins, K. F., and Usgaard, R. E. (1998). Bird flight characteristics near wind turbines in Minnesota. Am. Midl. Nat. 139, 29–38.
Perold, V., Ralston-Paton, S., and Ryan, P. (2020). On a collision course? The large diversity of birds killed by wind turbines in South Africa. Ostrich 91, 228–239. doi: 10.2989/00306525.2020.1770889
Péron, G., Calabrese, J. M., Duriez, O., Fleming, C. H., García-Jiménez, R., Johnston, A., et al. (2020). The challenges of estimating the distribution of flight heights from telemetry or altimetry data. Anim. Biotelem. 8:5. doi: 10.1101/751271
Péron, G., Fleming, C. H., Duriez, O., Fluhr, J., Itty, C., Lambertucci, S., et al. (2017). The energy landscape predicts flight height and wind turbine collision hazard in three species of large soaring raptor. J. Appl. Ecol. 54, 1895–1906. doi: 10.1111/1365-2664.12909
Poessel, S. A., Brandt, J., Mendenhall, L., Braham, M. A., Lanzone, M. J., Mcgann, A. J., et al. (2018). Flight response to spatial and temporal correlates informs risk from wind turbines to the California Condor. Condor 120, 330–342. doi: 10.1650/CONDOR-17-100.1
R Core Team (2019). R: a language and environment for statistical computing. Vienna, Austria: R Foundation for Statistical Computing.
Rothery, P., Newton, I., and Little, B. (2009). Observations of seabirds at offshore wind turbines near blyth in northeast england. Bird Study 56, 1–14. doi: 10.1080/00063650802648093
Sæther, B.-E., and Bakke, Ø (2000). Avian life history variation and contribution of demographic traits to the population growth rate. Ecology 81, 642–653. doi: 10.1890/0012-96582000081
Santangeli, A., Butchart, S. H. M., Pogson, M., Hastings, A., Smith, P., Girardello, M., et al. (2018). Mapping the global potential exposure of soaring birds to terrestrial wind energy expansion. Ornis Fenn. 95, 1–14.
Santangeli, A., Girardello, M., Buechley, E., Botha, A., Di Minin, E., and Moilanen, A. (2019). Priority areas for conservation of Old World vultures. Conserv. Biol. 33, 1056–1065. doi: 10.1111/cobi.13282
Schaub, M. (2012). Spatial distribution of wind turbines is crucial for the survival of red kite populations. Biol. Conserv. 155, 111–118. doi: 10.1016/j.biocon.2012.06.021
Schaub, T., Klaassen, R. H. G., Bouten, W., Schlaich, A. E., and Koks, B. J. (2020). Collision risk of Montagu’s Harriers Circus pygargus with wind turbines derived from high-resolution GPS tracking. IBIS 162, 520–534. doi: 10.1111/ibi.12788
Smallwood, K. S., and Thelander, C. (2008). Bird mortality in the Altamont Pass Wind Resource Area, California. J. Wildl. Manage. 72, 215–223. doi: 10.2193/2007-032
Smallwood, K. S., Rugge, L., and Morrison, M. L. (2009). Influence of Behavior on Bird Mortality in Wind Energy Developments. J. Wildl. Manage. 73, 1082–1098. doi: 10.2193/2008-555
Smith, J. A., and Dwyer, J. F. (2016). Avian interactions with renewable energy infrastructure: an update. Condor 118, 411–423. doi: 10.1650/condor-15-61.1
Sutherland, W., and Woodroof, H. (2009). The need for environmental horizon scanning Articulo Revisión. Trends Ecol. Evol. 24, 523–527. doi: 10.1016/j.tree.2009.04.008
Thaxter, C. B., Buchanan, G. M., Carr, J., Butchart, S. H. M., Newbold, T., Green, R. E., et al. (2017). Bird and bat species’ global vulnerability to collision mortality at wind farms revealed through a trait-based assessment. Proc. R. Soc. B Biol. Sci. 284:20170829. doi: 10.1098/rspb.2017.0829
Tikkanen, H., Rytkönen, S., Karlin, O. P., Ollila, T., Pakanen, V. M., Tuohimaa, H., et al. (2018). Modelling golden eagle habitat selection and flight activity in their home ranges for safer wind farm planning. Environ. Impact Assess. Rev. 71, 120–131. doi: 10.1016/j.eiar.2018.04.006
Watson, R. T., Kolar, P. S., Ferrer, M., Nygård, T., Johnston, N., Hunt, W. G., et al. (2018). Raptor interactions with wind energy: case studies from around the world. J. Raptor Res. 52, 1–18. doi: 10.3356/JRR-16-100.1
Wiser, R., Yang, Z., Hand, M., Hohmeyer, O., Infield, D., Jensen, P. H., et al. (2011). “Wind Energy,” in IPCC Special Report on Renewable Energy Sources and Climate Change Mitigation, eds O. Edenhofer, R. Pichs-Madruga, Y. Sokona, K. Seyboth, P. Matschoss, S. Kadner, et al. (Cambridge, UK: Cambridge University Press).
Keywords: Africa, bird of prey, flight behavior, raptor, wind turbine, wind power
Citation: McClure CJW, Dunn L, McCabe JD, Rolek BW, Botha A, Virani MZ, Buij R and Katzner TE (2021) Flight Altitudes of Raptors in Southern Africa Highlight Vulnerability of Threatened Species to Wind Turbines. Front. Ecol. Evol. 9:667384. doi: 10.3389/fevo.2021.667384
Received: 12 February 2021; Accepted: 13 September 2021;
Published: 08 October 2021.
Edited by:
Virginia Morandini, Oregon State University, United StatesReviewed by:
Darryl Jones, Griffith University, AustraliaWouter Vansteelant, Estación Biológica de Doñana (EBD), Spain
At least a portion of this work is authored by Todd E. Katzner on behalf of the U.S. Government and, as regards Dr. Katzner and the U.S. Government, is not subject to copyright protection in the United States. Foreign and other copyrights may apply. This is an open-access article distributed under the terms of the Creative Commons Attribution License (CC BY). The use, distribution or reproduction in other forums is permitted, provided the original author(s) and the copyright owner(s) are credited and that the original publication in this journal is cited, in accordance with accepted academic practice. No use, distribution or reproduction is permitted which does not comply with these terms.
*Correspondence: Christopher J. W. McClure, Y21jY2x1cmVAcGVyZWdyaW5lZnVuZC5vcmc=