- 1Johns Hopkins School of Medicine, The Brady Urological Institute, Baltimore, MD, United States
- 2Cellular and Molecular Medicine Program, Johns Hopkins School of Medicine, Baltimore, MD, United States
Dormancy is a key survival strategy in many organisms across the tree of life. Organisms that utilize some type of dormancy (hibernation, aestivation, brumation, diapause, and quiescence) are able to survive in habitats that would otherwise be uninhabitable. Induction into dormant states is typically caused by environmental stress. While organisms are dormant, their physical activity is minimal, and their metabolic rates are severely depressed (hypometabolism). These metabolic reductions allow for the conservation and distribution of energy while conditions in the environment are poor. When conditions are more favorable, the organisms are then able to come out of dormancy and reengage in their environment. Polyaneuploid cancer cells (PACCs), proposed mediators of cancer metastasis and resistance, access evolutionary programs and employ dormancy as a survival mechanism in response to stress. Quiescence, the type of dormancy observed in PACCs, allows these cells the ability to survive stressful conditions (e.g., hypoxia in the microenvironment, transiting the bloodstream during metastasis, and exposure to chemotherapy) by downregulating and altering metabolic function, but then increasing metabolic activities again once stress has passed. We can gain insights regarding the mechanisms underlying PACC dormancy by looking to the evolution of dormancy in different organisms.
Introduction: Dormancy, and Cancer
Metastatic cancer is resistant to almost all known systemic therapies and remains largely incurable (Pienta et al., 2020b). The inability to cure metastatic cancer leads to more than 10 million deaths globally per year (Bray et al., 2018). This resistance to therapy appears to be mediated, at least in part, by dormancy, a common survival strategy for many different organisms. Cancer cell dormancy occurs when cancer cells enter reversible cell cycle arrest known as quiescence (Yeh and Ramaswamy, 2015; Gao et al., 2017; Jahanban-Esfahlan et al., 2019; Recasens and Munoz, 2019). These dormant cancer cells lack proliferative and apoptotic markers but are still metabolically active and able to maintain essential cellular processes without continuous growth (Gao et al., 2017). Dormancy allows cancer cells the ability to survive new environments, initiate metastasis, become resistant to therapy, and evade immune detection (Recasens and Munoz, 2019). Cancer progression occurs when a dormant malignant cell gains the ability to re-enter cell cycle and restart uncontrolled proliferation. The ability to lay dormant during chemotherapy, stay metabolically active, and eventually reinitiate proliferation enables cancer cell survival and results in observed therapy resistant recurrence (Pienta et al., 2020c, 2021).
New evidence suggests that the dormant cancer cell capable of surviving stress by entering a quiescent state is the polyaneuploid cancer cell (PACC) (Lopez-Sánchez et al., 2014; Pienta et al., 2021). PACCs [also referred to as polyploid giant cancer cells (PGCCs), multinucleated cancer cells, blastomere-like cancer cells, and osteoclast-like cancer cells] are enlarged cancer cells that have undergone whole-genome multiplication of their aneuploid genome (Erenpreisa et al., 2000, 2008; Mosieniak and Sikora, 2010; Zhang et al., 2014, 2015; Fei et al., 2015; Chen et al., 2019; Pienta et al., 2020c). Polyaneuploid cancer cells have been observed in cell lines, in patients, and in mouse models across virtually all cancer types (Virchow, 1860; Illidge et al., 2000; Erenpreisa et al., 2008; Puig, 2008; Zhang et al., 2013, 2014; Ogden et al., 2015; Mittal et al., 2017; Niu et al., 2017; Mirzayans et al., 2018; Amend et al., 2019; Chen et al., 2019). Polyaneuploid cancer cells are capable of forming in response to many different environmental/applied stressors such as hypoxia, lack of nutrients, changes in pH, chemotherapy, or radiation (Illidge et al., 2000; Makarovskiy et al., 2002; Erenpreisa et al., 2008; Puig, 2008; Lopez-Sánchez et al., 2014; Mittal et al., 2017; Mirzayans et al., 2018; Amend et al., 2019; Chen et al., 2019; Lin et al., 2019). PACCs can form by endoreplication, failed cytokinesis, and fusion (Illidge et al., 2000; Mirzayans et al., 2018; Amend et al., 2019; Chen et al., 2019; Lin et al., 2019). Cancer cells with the ability to become a PACC have the capability to alter metabolic functions to enter quiescence to avoid DNA damage, potentially providing a mechanism of therapy resistance. When stress is lifted, PACCs exit quiescence and can repopulate an aneuploid population by neosis (cell budding). A better understanding of cancer cell and PACC dormancy is critical in the quest to cure cancer. This review focuses on what we can learn from dormancy observed in ecology to better understand how polyaneuploid cancer cells alter their metabolism and survive in a dormant state while under stress.
Dormancy: A Metabolic Strategy for Survival
Dormancy is a broadly used term to describe inactivity or lethargy (Navas and Carvalho, 2010). During dormancy, physical activity in organisms is minimal and metabolic rates can be altered or severely depressed (hypometabolism) (Navas and Carvalho, 2010; Mayer, 2016). Dormancy can be a response to various conditions such as circannual rhythm, temperature, or availability of resources (i.e., food and water) (Harlow, 1995; Lehmer et al., 2001; Navas and Carvalho, 2010). Dormancy can occur over short periods of time (less than a day), multiple days, an entire season, or, in extreme cases, even many years (Navas and Carvalho, 2010). While dormant organisms are physically inactive, they can still be aroused by disturbances without any major changes in their physiological state. There are many different forms of dormancy including hibernation (winter dormancy) (Geiser, 2013), aestivation (summer or dry season dormancy) (Storey and Storey, 2012), brumation (winter dormancy observed in ectotherms) (Wilkinson et al., 2017), diapause (period of suspended development in an insect, invertebrate, or mammal embryo in unfavorable conditions) (Denlinger, 2000; Tougeron, 2019), and quiescence (opportunistic inactivity observed in plants and cells) (Table 1) (Navas and Carvalho, 2010). During all of these dormant conditions organisms alter their metabolism to better survive unfavorable circumstances in their environment.
Hibernation
One of the most well-known and commonly recognized forms of dormancy is hibernation (Geiser, 2013). Hibernation can be split into two categories: obligate hibernation and facultative hibernation (Chayama et al., 2016). Obligate hibernation, the more common form of hibernation, occurs when a mammal hibernates at the same time every year (e.g., arctic ground squirrels and dwarf lemurs) (Chayama et al., 2016). The other form of hibernation, facultative hibernation, occurs only when an organism faces stress in their environment (e.g., black-tailed prairie dogs) (Lehmer et al., 2001; Chayama et al., 2016). Hibernation is characterized by a reduction in body temperature, energy expenditure, and other physiological functions in mammals (Geiser, 2013). These changes in metabolic functions allow for the conserved resources to be utilized throughout a multiday torpor (inactivity). Multiday torpor was originally hypothesized to only occur in winter due to the cold weather, however, it has since been revealed that it is most likely due to the lack of resources available during winter rather than the weather itself (Geiser, 2013). Hibernating species have evolved the ability to enter torpor to conserve energy by reducing their metabolic states in order to survive. Without evolving the ability to hibernate, many organisms would not have survived the environments they live in.
Obligate Hibernation
The majority of ground squirrels are obligate hibernators, including the arctic ground squirrel. Obligate hibernation allows arctic ground squirrels to survive harsh winters in Alaska despite sub-zero temperatures, frozen soil, little to no food available, and near complete darkness (Loren and Barnes, 1999). Obligate hibernators, like the arctic ground squirrel, have endogenously timed annual dormancy in winter where they generate energy reserves during the warmer months and then that energy is conserved through a large reduction of basal metabolic rate, heart rate, blood flow, and temperature while they hibernate (Loren and Barnes, 1999; Singhal et al., 2020). Arctic ground squirrels can hibernate for up to 8 months out of every year and during this hibernation they lower their body temperature to adopt the lowest body temperature ever measured in a mammal (-2.9°C) (Loren and Barnes, 1999; Buck et al., 2008; Richter et al., 2015; Singhal et al., 2020; Rice et al., 2020). Arctic ground squirrels recycle broken down nutrients while in hibernation to enable survival (Rice et al., 2020). Muscle is broken down and the free nitrogen generated can be converted into necessary amino acids (Rice et al., 2020). Using those amino acids, the squirrels can synthesize new proteins necessary for continued survival during their torpor without needing to ingest new nutrients from the environment (Rice et al., 2020). Additionally, arctic ground squirrels often wake from their hibernation while conditions in Alaska are still harsh and still have enough energy reserve leftover to sustain life until conditions improve and they are able to successfully forage for food (Loren and Barnes, 1999). This means that they strictly only dispense energy while in hibernation when completely necessary so as not to waste energy. This annual hibernation to avoid the harsh winters allows arctic ground squirrels to survive and populate a habitat which they would otherwise be unable to survive in.
Facultative Hibernation
Black-tailed prairie dogs, facultative hibernators, are unlike obligate hibernators as they only enter a shallow torpor in times of stress rather than entering an annual multi-day torpor. This shallow torpor is most commonly induced when black-tailed prairie dogs are severely cold or deprived of food and water (Harlow, 1995; Lehmer et al., 2001; Harlow and Frank, 2001). Similar to obligate hibernators, black-tailed prairie dogs experience a drop in body temperature and metabolic rate during their short term torpor (Geiser, 2013). The physiological advantages for adapting to be a facultative hibernator are still not well known, but it is hypothesized that natural selection likely favored facultative hibernation as black-tailed prairie dogs have lower lipid peroxidation rates, allowing them to conserve fat for extended periods of time (Harlow and Frank, 2001). Black-tailed prairie dogs share a common ancestor with white-tailed prairie dogs yet white-tailed prairie dogs remained obligate hibernators while black-tailed prairie dogs evolved to be facultative hibernators (Harlow, 1995). Some hypotheses of why black-tailed prairie dogs evolved to be facultative hibernators include (1) a lengthened growing season and a higher abundance of food in the great plains reducing the need for long term hibernation, (2) a lack of refuge from their most common predator, the black-footed-ferret, forced them to evolve to hibernate less, and (3) the black-tailed prairie dog conserves fat rather than protein during fasting which could reduce its ability to successfully hibernate for long periods of time (Harlow, 1995). While the true reason for this evolution is still unknown, it is interesting to note that evolving to be facultative hibernators has allowed black-tailed prairie dogs to only hibernate when absolutely necessary for survival, not on an annual basis.
Aestivation
Aestivation, while similar to hibernation, occurs during the warmer months of the year instead of during the winter (Navas and Carvalho, 2010). Aestivation is also known as “light” hibernation as these organisms are able to wake from their dormant state very quickly after the stress is removed. Ectotherms, organisms that are dependent on external sources of body heat (i.e., “cold-blooded”), undergo an intrinsic metabolic depression in which their metabolic rate declines to about 10–20% of their resting metabolic rate at the same body temperature, while endotherms, organisms capable of the internal generation of heat (i.e., “warm-blooded”) undergo a fundamental physiological change in body temperature, reduction in metabolic rate, and water loss (Navas and Carvalho, 2010). Helix aspersa, commonly known as the garden snail, is an example of an ectotherm that performs aestivation (Pedler et al., 1996). When overheated or underfed these snails are able to create a seal on their shell to prevent any residual moisture from leaving their body (Pedler et al., 1996). The snails then enter aestivation for long or short periods of time to survive whatever harsh conditions they have encountered (Pedler et al., 1996). During this period the snail only allocates energy to the most necessary processes for survival. For example, energy is not allocated to reproductive efforts. While hibernation during winter is the most common form of torpor known, aestivation provides animals with a survival strategy during the summer months. The utilization of dormancy and an altered metabolism to survive harsh heat, drought, and lack of food allows these animals to live and populate in an environment that would otherwise be deadly to them.
Brumation
Brumation is winter dormancy performed by ectotherms (Wilkinson et al., 2017; Kundey et al., 2018). While (obligate or facultative) hibernating mammals may experience regular small arousals from torpidity during the winter months, ectotherms undergoing brumation are dependent upon the temperature of their environment and stay dormant until temperatures rise again (Wilkinson et al., 2017; Kundey et al., 2018). An example of an ectotherm that undergoes brumation is the tiger salamander (Kundey et al., 2018). If temperature is constant and within a normal range for the salamanders they will not undergo brumation at any point during the year. However, if the salamanders live in an area where the temperature drastically drops at any point in the year they automatically enter brumation to survive the temperature change (Kundey et al., 2018). Tiger salamanders are also able to enter into aestivation during summer on days that are extremely hot, but their aestivation periods are typically much shorter than their brumation periods (Kundey et al., 2018). Additionally, multiple studies have shown that entering and exiting brumation does not result in any change in memory retention, meaning that as soon as tiger salamanders come out of brumation they remember exactly where to find food and how to find a mate (Wilkinson et al., 2017; Kundey et al., 2018). Tiger salamanders’ unique adaptions to enter brumation allow them to survive harsh winters or summers that would otherwise eliminate them from the environment.
Diapause
Diapause is a form of developmental arrest in insects that is equivalent to hibernation in mammals (Denlinger, 2000). Diapause is characterized by low metabolic activity, low behavioral activity, morphogenesis and reproductive functional arrest, and the slowing of growth (Denlinger, 2000; Tougeron, 2019). Diapause is obligatory in some species of insects and facultative in others (Denlinger, 2000). Diapause is induced by abiotic cues that indicate the onset of adverse conditions in the environment (Denlinger, 2000; Antonova-Koch et al., 2013). While diapause most commonly occurs during the winter, it can also occur during summer in months of extreme heat when there is a lack of resources (Denlinger, 2000). The arrest induced by diapause occurs in species-specific life stages (Antonova-Koch et al., 2013; Tougeron, 2019). For example, in the silk moth Bombyx mori, diapause occurs early in embryonic development, while in the gypsy moth Lymantria dispar, diapause occurs at the completion of embryonic development (Denlinger, 2000). Diapause allows insects at different life stages to halt growth and activity in adverse environments to enhance their survival. Without evolving to perform diapause, insects may have only been able to populate very specific regions of the world making them susceptible to predation and overcrowding.
Quiescence
Cellular quiescence, also known as the G0 stage in cell cycle, is defined as reversible proliferation arrest. Unicellular organisms as well as individual cells of various prokaryotic and eukaryotic microorganisms can survive in a quiescent state for long periods of time (days, months, or years) without added nutrients (Gray et al., 2004). Unicellular organisms and cells in complex organisms utilize and spend a part of their life in quiescence (Sagot and Laporte, 2019). Entry into quiescence is associated with dramatic decreases and changes in metabolic activities (Sagot and Laporte, 2019). While metabolism may slow and change while these cells are in a non-proliferative state, these cells are not completely inactive as they still perform basic maintenance for survival. Unicellular organisms are more frequently quiescent and can remain in a quiescent state waiting for signals such as temperature, oxygen, or nutrients to exit quiescence and begin proliferating again (Sagot and Laporte, 2019). An example of an organism that regularly utilizes quiescence is Saccharomyces cerevisiae, a species of yeast (Wloch-Salamon et al., 2017; Sagot and Laporte, 2019). When stressed or nutrient deprived it has been shown that around 75% of a stationary-phase culture of S. cerevisiae will enter into a quiescent state (Wloch-Salamon et al., 2017). S. cerevisiae quiescent cells differ from non-quiescent cells in the same culture as they develop a widened cell wall (Aragon et al., 2008), increase storage of carbohydrates (Aragon et al., 2008), sequester proteins (Suresh et al., 2015), cluster telomeres (Tjaden, 2015), and undergo transcriptional shut down (McKnight et al., 2015). Additionally, it has been shown that cell volume influences quiescence exit efficiency (Sagot and Laporte, 2019). S. cerevisiae cells exiting quiescence must reach a critical size before they are able to enter the S phase of cell cycle and thus larger quiescent yeast generally emit buds faster than smaller quiescent yeast (Laporte et al., 2018). Quiescence is a unique form of cellular dormancy that allows unicellular and multicellular organisms the capability to survive stress in many various forms by essentially shutting down reproductive efforts and lowering metabolic functions until the stress is removed. Saccharomyces cerevisiae provide a unique model that can be used to better understand cancer cell metabolism during dormancy.
Discussion: Understanding Different Types of Dormancy Provides Insight Into Dormant PACC Metabolism
All types of hibernation give organisms a mechanism of survival in unfavorable environments. Common themes for all types of dormancy are lowered levels of activity, little to no reproductive efforts, lowered intake of nutrients, and reduced metabolic rates. PACCs require a survival mechanism and altering their metabolism while in a dormant state is a possible mechanism to survive stresses such as chemotherapy. Understanding and comparing the different types of dormancy in nature provides insight into how altered PACC metabolism during dormancy is helping these cells survive and provides a possible opportunity for therapeutic intervention.
Throughout evolution, organisms have adapted many different metabolic strategies for survival. A hallmark of cancer is reprogramming energy metabolism (Hanahan and Weinberg, 2011). One of the most well characterized metabolic changes in cancer cells is the Warburg effect: that cancer cells preferentially utilize glycolysis to generate the majority of their ATP, even while in aerobic conditions (Warburg, 1930; Hanahan and Weinberg, 2011). When cancer cells are stressed their metabolic signatures can be further altered. Cancer cells undergoing stress such as starvation, radiation, or chemotherapy are known to have an altered metabolism compared to their non-stress counterparts, with evidence of accumulation of lipids and an increase in autophagy to survive (Hanahan and Weinberg, 2011).
To study the metabolism of dormant cancer cells under stress, the dormant population must first be identified. Upon treatment of a cancer cell population with chemotherapy, an emergence of physically enlarged cells with high genomic content are observed in vitro (Erenpreisa et al., 2008; Zhang et al., 2014; Amend et al., 2019; Pienta et al., 2020a,c). These cells, defined as PACCs, exhibit temporary polyploidization of their aneuploid genome while simultaneously altering their metabolism to survive environmental stress (Pienta et al., 2020a,c). Following polyploidization, PACCs halt cell proliferation, entering a dormant state (Pienta et al., 2021). Eventually, when stress is removed, PACCs exit the dormant state and generate progeny to establish a recurrence (Puig, 2008; Zhang et al., 2013; Amend et al., 2019). PACCs are a transient state of cancer cells that are hypothesized to utilize dormancy and an altered metabolism for survival (Pienta et al., 2020a,c). While under stress, PACCs appear to utilize different metabolic functions to survive, but the exact PACC metabolic signature has yet to be defined (Sirois et al., 2019). Cellular quiescence is associated with low metabolic rates, altered glucose metabolism, lipid accumulation, and an activation of autophagy to provide nutrients for survival (Valcourt et al., 2012). The quiescent state in PACCs can be compared to dormancy in organisms that are able to remain in an inactive state for long periods of time under stress for enhanced survival. Comparing dormant PACC metabolism to the metabolism of other dormant organisms across the tree of life may provide valuable insight into PACC biology and survival mechanisms.
Different types of dormancy have evolved for survival of organisms across multiple kingdoms of life. The key component in all different types of dormancy is altered (though not necessarily diminished) metabolic activity for prolonged survival without further intake of necessary nutrients. Similarities can be observed between all types of dormancy and PACC dormancy (Table 1). For example, while in obligate hibernation, the arctic ground squirrel recycles cellular components for energy so that intake of nutrients during hibernation is unnecessary. Facultative hibernation highlights that dormancy is not exclusively an annual or periodic event but can occur near-immediately as needed for survival. Quiescence allows yeast the ability to exit the active cell cycle during stress to conserve resources until the stress is removed and they can begin proliferating again. All of these strategies are key survival mechanisms that cancer cells seem to access.
Obligate hibernators hibernate annually every winter. During obligate hibernation arctic ground squirrels can recycle and reuse select cellular components to make up for their lack of nutrient intake. Arctic ground squirrel skeletal muscle is broken down and the free nitrogen generated is converted into necessary amino acids which are synthesized into proteins that are essential for continued survival during hibernation (Rice et al., 2020). This metabolic survival mechanism can be compared to elevated autophagy in cancer cells (Table 1). When cancer cells are stressed, autophagy is induced. Autophagy is the process in which cells engulf and break down portions of their cytoplasm to be recycled for future use. This process can generate a high level of fatty acids and energy for the cells. PACCs that enter quiescence due to chemotherapy treatment are under high levels of stress and are hypothesized to have increased autophagy levels (Dudkowska et al., 2021). High levels of autophagy could give PACCs a metabolic advantage during quiescence by slowly breaking down cellular components for energy instead of importing nutrients from the toxic environment. This metabolic “recycling” allows arctic ground squirrels and PACCs the ability to better survive dormancy without exerting excess energy to obtain more resources and limiting risk from the harsh environment.
Facultative hibernation allows mammals the advantage of entering torpor only when it is necessary. This allows mammals to continue foraging, hunting, and mating until the moment they become too stressed by changes in their environment and they must decrease metabolic activities and enter torpor to survive. Black-tailed prairie dogs have been shown to have lower lipid peroxidation rates while undergoing torpor (Harlow, 1995). This implies a storage of lipids and fats, but not a high usage of them, indicating the hibernating black-tailed prairie dogs are either using other sources of energy to survive, slowly breaking down these lipids for energy to ensure they have enough energy for long term survival, or they are sequestering toxins in these lipids to protect other cells in the body from damage. Cancer cells mimic facultative hibernation when conditions are bad (e.g., chemotherapy is introduced to the environment) by forming PACCs which are in a metabolically depressed quiescent state (Table 1). While in a quiescent state, PACCs appear to have high number of lipid droplets that are not quickly degraded (Figure 1) (Sirois et al., 2019). These lipids could be sequestering toxins or they could be saved as energy reserves for when PACCs exit quiescence and proliferate again. PACCs and black-tailed prairie dogs only enter dormancy when absolutely necessary to survive stressors that would otherwise destroy the rest of the population. The ability to only lay dormant when absolutely necessary allows PACCs and black-tailed prairie dogs to live and inhabit environments that may have been deadly to them otherwise.
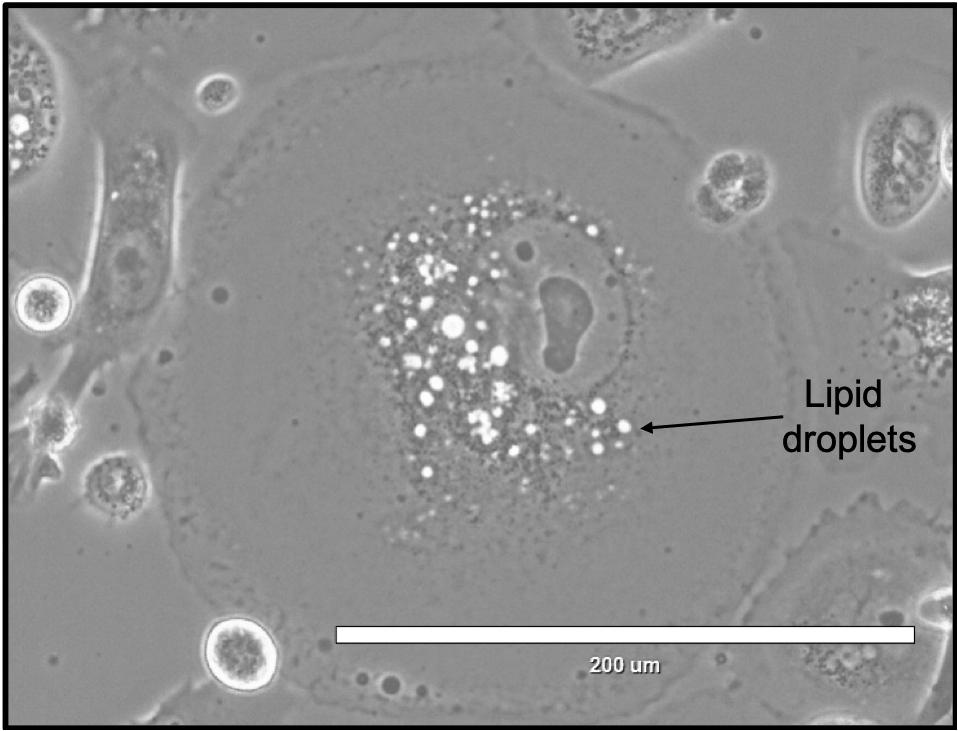
Figure 1. PACCs accumulate lipid droplets. This image shows a single PACC with a high level of lipids (black arrow) surrounding the nucleus.
Quiescence is a key survival strategy for many unicellular organisms. Quiescence allows yeast to survive nutrient deprived and other harsh environments. Quiescent cells are found all throughout the human body and are vital for normal tissue homeostasis (Coller, 2011; Yao, 2014). Infrequent cell cycle (entering and exiting quiescence) has been described as a fundamental mechanism that can contribute to the evolution of therapy resistance in cancer (Coward and Harding, 2014; Donovan et al., 2014). Cellular quiescence can provide metabolic adaptions as well as protection against stress and toxicities which is important for long-lived cells and, unfortunately, for cancer cells (Yao, 2014). It is hypothesized that PACCs enter into quiescence and alter their metabolism as a survival strategy when they encounter stress (e.g., hypoxia, chemotherapy or radiation) and then re-enter the cell cycle when the stress is lifted (Erenpreisa et al., 2008; Lopez-Sánchez et al., 2014; Pienta et al., 2020b). Some parallels of yeast and PACC quiescence seem to be that larger quiescent yeast cells are more successful at survival and exiting quiescence while PACCs utilize larger size to house additional cellular machinery to metabolize toxins as well as access additional nutrients though autophagy (Table 1). Additionally, quiescent yeast sequester proteins and undergo transcriptional shut down to survive. Similarly, PACCs appear to have high levels of lipids (Sirois et al., 2019) which means they are most likely harboring fat to use during their dormant state as well as not proliferating to save energy.
Analyzing parallel relationships between dormant organisms can give us insight into how PACCs survive dormancy. Figure 2 demonstrates the parallel relationships between two different types of organisms that utilize dormancy (black-tailed prairie dogs and yeast) and PACCs. Black-tailed prairie dogs feed on grass around their burrows to put on weight for winter months when food is scarce and potential hibernation may occur. Only when a stressor is introduced (snow/no food) will the black-tailed prairie dogs actually begin facultative hibernation for survival. When the environment is favorable again the black-tailed prairie dogs are able to exit their temporary torpor and resume normal daily activities and reproductive efforts (Figure 2A). Similarly, when S. cerevisiae are starved, the majority of the population becomes quiescent and some individuals become enlarged. As stated earlier, recovering, larger S. cerevisiae are more successful at exiting quiescence. The budded progeny of S. cerevisiae return to the same size as the original S. cerevisiae population (Figure 2B). Lastly, when in a stable environment, cancer cells are continuously proliferating. However, when stressed with chemotherapy, typical cancer cells become PACCs through failed cytokinesis, fusion, or endoreplication (Erenpreisa et al., 2008; Amend et al., 2019; Lin et al., 2019; Chen et al., 2019). Polyaneuploid cancer cell are identified as large cells with a high of genomic content. Under stress PACCs are the majority of the population and enter into a quiescent state to survive. In this quiescent state PACCs contain high levels of lipids (Sirois et al., 2019), they do not reproduce (Pienta et al., 2021), and they are physically enlarged, similar to the enlarged S. cerevisiae. When stress is released, PACCs are able to undergo division (i.e., restart reproductive efforts) in the form of neosis (cell budding) to produce new, smaller progeny that are the same size as the original cell population (Figure 2C) (Sundaram et al., 2004; Zhang et al., 2013; Amend et al., 2019).
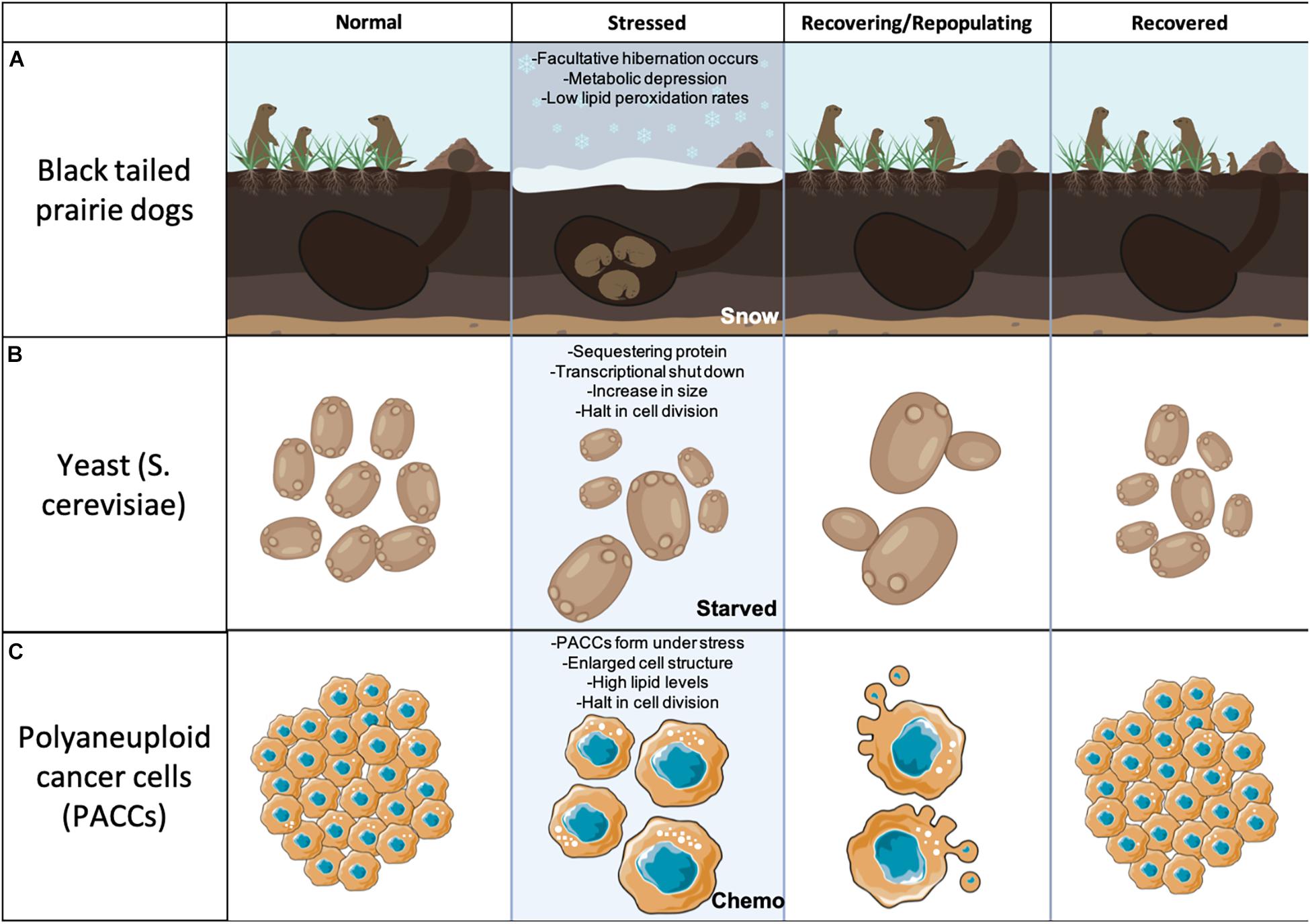
Figure 2. Parallel relationships between black-tailed prairie dogs, S. cerevisiae, and PACCs. (A) Black-tailed prairie dogs in a normal, non-stressed environment are active in the environment. When stress (snow) is applied the black-tailed prairie dogs enter facultative hibernation. When the snow goes away the black-tailed prairie dogs are able to exit their dormant state, become active again, and reproduce. (B) S. cerevisiae are first shown in a normal environment. When stressed (starved) they enter into a quiescent state and some become enlarged. When stress is released they are able to exit quiescence and repopulate utilizing budding. (C) Cancer cells in a normal environment are constantly proliferating. When stress (chemotherapy) is applied many of these cells undergo failed cytokinesis or endoreplication and become PACCs. PACCs are larger than the original cancer cells and enter into a quiescent non-proliferative state, and contain high levels of lipid droplets. When stress is released PACCs are able to undergo various forms of division (i.e., restart reproductive efforts) such as neosis or asymmetrical division to produce new, smaller progeny that are the same size as the original cell population.
All three of these examples demonstrate dormancy aiding in the survival of these groups. During dormancy all of these groups alter their metabolism to better survive in a dormant state. Whether it be black-tailed prairie dogs that only enter torpor when absolutely necessary for survival, or S. cerevisiae growing in size to aid in their eventual repopulation, there are parallels to PACC metabolism.
Conclusion
Despite increasing knowledge regarding molecular mechanisms that drive cancer cell dormancy and their potential targets, the best therapeutic approach to targeting dormancy still remains unclear. Evolution provides examples of how various organisms’ metabolic activities are altered during dormancy to enhance survival. Learning from models in nature like arctic ground squirrels (breaking down and reusing nutrients), black-tailed prairie dogs (low lipid peroxidation rates), and yeast (larger size assists with exiting quiescence) can help us elucidate PACC metabolism during dormancy. Learning more about PACC metabolism in dormancy may be the key to learning how to target these cells while in a dormant state. For example, the next steps point to targeting lipid metabolism or autophagy initiation which may be key factors to PACC survival in a quiescent state. Disrupting PACC metabolism in a quiescent state will ultimately lead to apoptosis of these cells. Successfully targeting these cells will provide a critical therapeutic opportunity to reduce metastatic chemotherapy-resistant tumor burden.
Author Contributions
LK: writing – original draft preparation and conceptualization. KP: funding acquisition and writing – reviewing and editing. SA: conceptualization, funding acquisition, and writing – reviewing and editing. All authors contributed to the article and approved the submitted version.
Funding
This work was supported by the US Department of Defense CDMRP/PCRP (W81XWH-20-10353), the Patrick C. Walsh Prostate Cancer Research Fund and the Prostate Cancer Foundation to SA; and NCI grants U54CA143803, CA163124, CA093900, and CA143055, and the Prostate Cancer Foundation to KP. This work was also supported by the William and Carolyn Stutt Research Fund, Ronald Rose, MC Dean, Inc., William and Marjorie Springer, Mary and Dave Stevens, Louis Dorfman, and the Jones Family Foundation.
Conflict of Interest
KP is a consultant to Cue Biopharma, Inc., a founder and an equity holder in Keystone Biopharma, Inc., and an equity holder in PEEL therapeutics. SA is also an equity holder in Keystone Biopharma Inc.
The remaining author declares that the research was conducted in the absence of any commercial or financial relationships that could be construed as a potential conflict of interest.
References
Amend, S. R., Torga, G., Lin, K. C., Kostecka, L. G., de Marzo, A., Austin, R. H., et al. (2019). Polyploid giant cancer cells: unrecognized actuators of tumorigenesis, metastasis, and resistance. Prostate 79, 1489–1497. doi: 10.1002/pros.23877
Antonova-Koch, Y., Riehle, M. A., Arik, A. J., and Brown, M. R. (2013). “Chapter 38 - Insulin-Like Peptides,” in Handbook of Biologically Active Peptides 2nd Edition, ed. A. Kastin (Amsterdam: Elsevier),Google Scholar
Aragon, A. D., Rodriguez, A. L., Meirelles, O., Roy, S., Davidson, G. S., Tapia, P. H., et al. (2008). Characterization of Differentiated Quiescent and Nonquiescent Cells in Yeast Stationary-Phase Cultures. Mol. Biol. Cell 19, 1271–1280. doi: 10.1091/mbc.e07-07-0666
Bray, F., Ferlay, J., Soerjomataram, I., Siegel, R. L., Torre, L. A., and Jemal, A. (2018). Global cancer statistics 2018: GLOBOCAN estimates of incidence and mortality worldwide for 36 cancers in 185 countries. CA Cancer J. Clin. 68, 394–424. doi: 10.3322/caac.21492
Buck, C. L., Breton, A., Kohl, F., and Tøien, O. (2008). “Overwinter body temperature patterns in free-living arctic squirrels (Spermophilus parryii),” in Hypometabolism in animals: torpor hibernation and cryobiology, eds B. G. Lovegrove and A. E. McKechnie (Pietermaritzburg: University of KwaZulu-Natal), 317–326.
Chayama, Y., Ando, L., Tamura, Y., Miura, M., and Yamaguchi, Y. (2016). Decreases in body temperature and body mass constitute pre-hibernation remodelling in the Syrian golden hamster, a facultative mammalian hibernator. R. Soc. Open Sci. 3:160002. doi: 10.1098/rsos.160002
Chen, J., Niu, N., Zhang, J., Qi, L., Shen, W., Donkena, K. V., et al. (2019). Polyploid Giant Cancer Cells (PGCCs): the Evil Roots of Cancer. Curr. Cancer Drug Targets 19, 360–367. doi: 10.2174/1568009618666180703154233
Coller, H. A. (2011). The Essence of Quiescence. Science 334, 1074–1075. doi: 10.1126/science.1216242
Coward, J., and Harding, A. (2014). Size Does Matter: why Polyploid Tumor Cells are Critical Drug Targets in the War on Cancer. Front. Oncol. 4:123. doi: 10.3389/fonc.2014.00123
Denlinger, D. L. (2000). “Chapter 18 - Molecular Regulation of Insect Diapause,” in Cell and Molecular Response to Stress, eds K. B. Storey and J. M. Storey (Amsterdam: Elsevier), 259–275. doi: 10.1016/S1568-1254(00)80020-0
Donovan, P., Cato, K., Legaie, R., Jayalath, R., Olsson, G., Hall, B., et al. (2014). Hyperdiploid tumor cells increase phenotypic heterogeneity within Glioblastoma tumors. Mol. BioSyst. 10, 741–758. doi: 10.1039/C3MB70484J
Dudkowska, M., Staniak, K., Bojko, A., and Sikora, E. (2021). “Chapter Five - The role of autophagy in escaping therapy-induced polyploidy/senescence,” in Advances in Cancer Research, eds D. A. Gewirtz and P. B. Fisher (Cambridge: Academic Press), 209–247. doi: 10.1016/bs.acr.2021.01.004
Erenpreisa, J., Ivanov, A., Wheatley, S. P., Kosmacek, E. A., Ianzini, F., Anisimov, A. P., et al. (2008). Endopolyploidy in irradiated p53-deficient tumour cell lines: persistence of cell division activity in giant cells expressing Aurora B- kinase. Cell Biol. Int. 32, 1044–1056. doi: 10.1016/j.cellbi.2008.06.003
Erenpreisa, J. A., Cragg, M. S., Fringes, B., Sharakhov, I., and Illidge, T. M. (2000). Release of Mitotic Descendants by Giant Cells from Irradiated Burkitt’s Lymphoma Cell Lines. Cell Biol. Int. 24, 635–648. doi: 10.1006/cbir.2000.0558
Fei, F., Zhang, D., Yang, Z., Wang, S., Wang, X., Wu, Z., et al. (2015). The number of polyploid giant cancer cells and epithelial-mesenchymal transition-related proteins are associated with invasion and metastasis in human breast cancer. J. Exp. Clin. Cancer Res. 34:158. doi: 10.1186/s13046-015-0277-8
Gao, X., Zhang, M., Tang, Y., and Liang, X. (2017). Cancer cell dormancy: mechanisms and implications of cancer recurrence and metastasis. Onco Targets Ther. 10, 5219–5228. doi: 10.2147/OTT.S140854
Gray, J. V., Petsko, G. A., Johnston, G. C., Ringe, D., Singer, R. A., and Werner-Washburne, M. (2004). Sleeping Beauty’: quiescence in Saccharomyces cerevisiae. Microbiol. Mol. Biol. Rev. 68:20.
Hanahan, D., and Weinberg, R. A. (2011). Hallmarks of Cancer: the Next Generation. Cell 144, 646–674. doi: 10.1016/j.cell.2011.02.013
Harlow, H. J. (1995). Fasting Biochemistry of Representative Spontaneous and Facultative Hibernators: the White-Tailed Prairie Dog and the Black-Tailed Prairie Dog. Physiol. Zool. 68, 915–934. doi: 10.1086/physzool.68.5.30163938
Harlow, H. J., and Frank, C. L. (2001). The role of dietary fatty acids in the evolution of spontaneous and facultative hibernation patterns in prairie dogs. J. Comp. Physiol. B 171, 77–84. doi: 10.1007/s003600000148
Illidge, T. M., Cragg, M. S., Fringes, B., Olive, P., and Erenpreisa, J. A. (2000). Polyploid giant cells provide a survival mechanism for p53 mutant cells after DNA damage. Cell Biol. Int. 24, 621–633. doi: 10.1006/cbir.2000.0557
Jahanban-Esfahlan, R., Seidi, K., Manjili, M. H., Jahanban-Esfahlan, A., Javaheri, T., and Zare, P. (2019). Tumor Cell Dormancy: threat or Opportunity in the Fight against Cancer. Cancers 11:1207. doi: 10.3390/cancers11081207
Kundey, S. M. A., Lessard, A., Fitz, A., and Panwar, M. (2018). Tiger salamanders’ (Ambystoma tigrinum) response retention and usage of visual cues following brumation. Behav. Processes 157, 502–508. doi: 10.1016/j.beproc.2018.06.008
Laporte, D., Jimenez, L., Gouleme, L., and Sagot, I. (2018). Yeast quiescence exit swiftness is influenced by cell volume and chronological age. Microb. Cell 5, 104–111. doi: 10.15698/mic2018.02.615
Lehmer, E. M., Van Horne, B., Kulbartz, B., and Florant, G. L. (2001). Facultative Torpor in Free-Ranging Black-Tailed Prairie Dogs (Cynomys Ludovicianus). J. Mammal. 82, 551–557. doi: 10.1644/1545-15422001082<0551:FTIFRB<2.0.CO;2
Lin, K.-C., Torga, G., Sun, Y., Axelrod, R., Pienta, K. J., Sturm, J. C., et al. (2019). The role of heterogeneous environment and docetaxel gradient in the emergence of polyploid, mesenchymal and resistant prostate cancer cells. Clin. Exp. Metastasis 36, 97–108. doi: 10.1007/s10585-019-09958-1
Lopez-Sánchez, L. M., Jimenez, C., Valverde, A., Hernandez, V., Peñarando, J., Martinez, A., et al. (2014). CoCl2, a Mimic of Hypoxia, Induces Formation of Polyploid Giant Cells with Stem Characteristics in Colon Cancer. PLoS One 9:e99143. doi: 10.1371/journal.pone.0099143
Loren, C., and Barnes, B. M. (1999). Annual cycle of body composition and hibernation in free-living arctic ground squirrels. J. Mammal. 80:13.
Makarovskiy, A. N., Siryaporn, E., Hixson, D. C., and Akerley, W. (2002). Survival of docetaxel-resistant prostate cancer cells in vitro depends on phenotype alterations and continuity of drug exposure. Cell. Mol. Life Sci. 59, 1198–1211. doi: 10.1007/s00018-002-8498-3
Mayer, W. V. (2016). “Dormancy,” in Encyclopedia Britannica Available online at: https://www.britannica.com/science/dormancy (accessed December 1, 2020).
McKnight, J. N., Boerma, J. W., Breeden, L. L., and Tsukiyama, T. (2015). Global Promoter Targeting of a Conserved Lysine Deacetylase for Transcriptional Shutoff During Quiescence Entry. Mol. Cell 59, 732–743. doi: 10.1016/j.molcel.2015.07.014
Mirzayans, R., Andrais, B., and Murray, D. (2018). Roles of Polyploid/Multinucleated Giant Cancer Cells in Metastasis and Disease Relapse Following Anticancer Treatment. Cancers 10:118. doi: 10.3390/cancers10040118
Mittal, K., Donthamsetty, S., Kaur, R., Yang, C., Gupta, M. V., Reid, M. D., et al. (2017). Multinucleated polyploidy drives resistance to Docetaxel chemotherapy in prostate cancer. Br. J. Cancer 116, 1186–1194. doi: 10.1038/bjc.2017.78
Mosieniak, G., and Sikora, E. (2010). Polyploidy: the link between senescence and cancer. Curr. Pharm. Des. 16, 734–740. doi: 10.2174/138161210790883714
Niu, N., Mercado-Uribe, I., and Liu, J. (2017). Dedifferentiation into blastomere-like cancer stem cells via formation of polyploid giant cancer cells. Oncogene 36, 4887–4900. doi: 10.1038/onc.2017.72
Ogden, A., Rida, P. C. G., Knudsen, B. S., Kucuk, O., and Aneja, R. (2015). Docetaxel-induced polyploidization may underlie chemoresistance and disease relapse. Cancer Lett. 367, 89–92. doi: 10.1016/j.canlet.2015.06.025
Pedler, S., Fuery, C. J., Withers, P. C., Flanigan, J., and Guppy, M. (1996). Effectors of metabolic depression in an estivating pulmonate snail (Helix aspersa): whole animal and in vitro tissue studies. J. Comp. Physiol. B 166, 375–381. doi: 10.1007/BF02336920
Pienta, K. J., Hammarlund, E. U., Austin, R. H., Axelrod, R., Brown, J. S., and Amend, S. R. (2020a). Cancer cells employ an evolutionarily conserved polyploidization program to resist therapy. Semin. Cancer Biol. doi: 10.1016/j.semcancer.2020.11.016 [Online ahead of print]
Pienta, K. J., Hammarlund, E. U., Axelrod, R., Amend, S. R., and Brown, J. S. (2020b). Convergent Evolution, Evolving Evolvability, and the Origins of Lethal Cancer. Mol. Cancer Res. 18, 801–810. doi: 10.1158/1541-7786.MCR-19-1158
Pienta, K. J., Hammarlund, E. U., Axelrod, R., Brown, J. S., and Amend, S. R. (2020c). Poly-aneuploid cancer cells promote evolvability, generating lethal cancer. Evol. Appl. 13, 1626–1634. doi: 10.1111/eva.12929
Pienta, K. J., Hammarlund, E. U., Brown, J. S., Amend, S. R., and Axelrod, R. M. (2021). Cancer recurrence and lethality are enabled by enhanced survival and reversible cell cycle arrest of polyaneuploid cells. Proc. Natl. Acad. Sci. U. S. A. 118:e2020838118. doi: 10.1073/pnas.2020838118
Puig, P.-E. (2008). Tumor cells can escape DNA-damaging cisplatin through DNA endoreduplication and reversible polyploidy. Cell Biol. Int. 32, 1031–1043.
Recasens, A., and Munoz, L. (2019). Targeting Cancer Cell Dormancy. Trends Pharmacol. Sci. 40, 128–141. doi: 10.1016/j.tips.2018.12.004
Rice, S. A., Ten Have, G. A. M., Reisz, J. A., Gehrke, S., Stefanoni, D., Frare, C., et al. (2020). Nitrogen recycling buffers against ammonia toxicity from skeletal muscle breakdown in hibernating arctic ground squirrels. Nat. Metab. 2:12. doi: 10.1038/s42255-020-00312-4
Richter, M. M., Williams, C. T., Lee, T. N., Tøien, Ø, Florant, G. L., Barnes, B. M., et al. (2015). Thermogenic capacity at subzero temperatures: how low can a hibernator go? Physiol. Biochem. Zool. 88, 81–89. doi: 10.1086/679591
Sagot, I., and Laporte, D. (2019). The cell biology of quiescent yeast – a diversity of individual scenarios. J. Cell Sci. 132:jcs213025. doi: 10.1242/jcs.213025
Singhal, N. S., Bai, M., Lee, E. M., Luo, S., Cook, K. R., and Ma, D. K. (2020). Cytoprotection by a naturally occurring variant of ATP5G1 in Arctic ground squirrel neural progenitor cells. Elife 9:e55578. doi: 10.7554/eLife.55578
Sirois, I., Aguilar-Mahecha, A., Lafleur, J., Fowler, E., Vu, V., Scriver, M., et al. (2019). A Unique Morphological Phenotype in Chemoresistant Triple-Negative Breast Cancer Reveals Metabolic Reprogramming and PLIN4 Expression as a Molecular Vulnerability. Mol. Cancer Res. 17, 2492–2507. doi: 10.1158/1541-7786.MCR-19-0264
Storey, K. B., and Storey, J. M. (2012). Aestivation: signaling and hypometabolism. J. Exp. Biol. 215, 1425–1433. doi: 10.1242/jeb.054403
Sundaram, M., Guernsey, D. L., Rajaraman, M. M., and Rajaraman, R. (2004). Neosis: a Novel Type of Cell Division in Cancer. Cancer Biol. Ther. 3, 207–218. doi: 10.4161/cbt.3.2.663
Suresh, H. G., da Silveira Dos Santos, A. X., Kukulski, W., Tyedmers, J., Riezman, H., Bukau, B., et al. (2015). Prolonged starvation drives reversible sequestration of lipid biosynthetic enzymes and organelle reorganization in Saccharomyces cerevisiae. Mol. Biol. Cell 26, 1601–1615. doi: 10.1091/mbc.E14-11-1559
Tjaden, B. (2015). De novo assembly of bacterial transcriptomes from RNA-seq data. Genome Biol. 16:1. doi: 10.1186/s13059-014-0572-2
Tougeron, K. (2019). Diapause research in insects: historical review and recent work perspectives. Entomol. Exp. Appl. 167, 27–36. doi: 10.1111/eea.12753
Valcourt, J. R., Lemons, J. M. S., Haley, E. M., Kojima, M., Demuren, O. O., and Coller, H. A. (2012). Staying alive. Cell Cycle 11, 1680–1696. doi: 10.4161/cc.19879
Virchow, R. (1860). “Cellular Pathology as based upon physiological and pathological histology. Twenty lectures delivered in. 1858,”in Translated from the second edition of the original by F. Chance. With notes and numerous emendations principally from MS. notes of the author, and illustrated by. engravings on wood. (London: John Churchill, MDCCCLX).
Warburg, O. H. (1930). The Metabolism of Tumours: Investigations from the Kaiser Wilhelm Institute for Biology, Berlin-Dahlem. London: Constable & Co. Ltd.
Wilkinson, A., Hloch, A., Mueller-Paul, J., and Huber, L. (2017). The effect of brumation on memory retention. Sci. Rep. 7:40079. doi: 10.1038/srep40079
Wloch-Salamon, D. M., Fisher, R. M., and Regenberg, B. (2017). Division of labour in the yeast: Saccharomyces cerevisiae. Yeast 34, 399–406. doi: 10.1002/yea.3241
Yao, G. (2014). Modelling mammalian cellular quiescence. Interface Focus 4:20130074. doi: 10.1098/rsfs.2013.0074
Yeh, A. C., and Ramaswamy, S. (2015). Mechanisms of Cancer Cell Dormancy—Another Hallmark of Cancer? Cancer Res. 75, 5014–5022. doi: 10.1158/0008-5472.CAN-15-1370
Zhang, L., Wu, C., and Hoffman, R. M. (2015). Prostate Cancer Heterogeneous High-Metastatic Multi-Organ-Colonizing Chemo-Resistant Variants Selected by Serial Metastatic Passage in Nude Mice Are Highly Enriched for Multinucleate Giant Cells. PLoS One 10:e0140721. doi: 10.1371/journal.pone.0140721
Zhang, S., Mercado-Uribe, I., Hanash, S., and Liu, J. (2013). iTRAQ-Based Proteomic Analysis of Polyploid Giant Cancer Cells and Budding Progeny Cells Reveals Several Distinct Pathways for Ovarian Cancer Development. PLoS One 8:e80120. doi: 10.1371/journal.pone.0080120
Keywords: hibernation, dormancy, polyaneuploid cancer cells, cancer, evolution
Citation: Kostecka LG, Pienta KJ and Amend SR (2021) Polyaneuploid Cancer Cell Dormancy: Lessons From Evolutionary Phyla. Front. Ecol. Evol. 9:660755. doi: 10.3389/fevo.2021.660755
Received: 29 January 2021; Accepted: 15 June 2021;
Published: 07 July 2021.
Edited by:
Etienne Baratchart, Lund University, SwedenReviewed by:
Andriy Marusyk, Moffitt Cancer Center, United StatesAnna K. Miller, Moffitt Cancer Center, United States
Arturo Araujo, University of Roehampton London, United Kingdom
Copyright © 2021 Kostecka, Pienta and Amend. This is an open-access article distributed under the terms of the Creative Commons Attribution License (CC BY). The use, distribution or reproduction in other forums is permitted, provided the original author(s) and the copyright owner(s) are credited and that the original publication in this journal is cited, in accordance with accepted academic practice. No use, distribution or reproduction is permitted which does not comply with these terms.
*Correspondence: Laurie G. Kostecka, bGtvc3RlYzFAamhtaS5lZHU=; orcid.org/0000-0003-2124-3485