- 1Department of Entomology, Virginia Polytechnic Institute and State University, Blacksburg, VA, United States
- 2Department of Genetics and Cell Biology, Tomsk State University, Tomsk, Russia
Haldane’s rule of speciation states that sterility or inviability affects the heterogametic sex of inter-species hybrids. Darwin’s corollary to Haldane’s rule implies that there are asymmetric phenotypes in inter-species hybrids from reciprocal crosses. Studying the phenotypes of F1 hybrids among closely related species of malaria mosquitoes can assist researchers in identifying the genetic factors and molecular mechanisms of speciation. To characterize phenotypes of sterile hybrid males in the Anopheles gambiae complex, we performed crosses between laboratory strains of An. merus and either An. gambiae or An. coluzzii. The reproductive tracts had normal external morphology in hybrid males from crosses between female An. merus and male An. gambiae or An. coluzzii. Despite being sterile, these males could copulate with females for a normal period of time and could transfer a mating plug to induce female oviposition and monogamy. In contrast, the entire reproductive tracts in hybrid males from crosses between female An. gambiae or An. coluzzii and male An. merus were severely underdeveloped. These males had atrophic testes and reduced somatic organs of the reproductive system including male accessary glands and ejaculatory duct. In addition, hybrid males with underdeveloped reproductive tracts displayed a shorter copulation time with females and failed to induce female oviposition and monogamy due to their inability to form and transfer a plug to females during mating. The asymmetry of the phenotypes associated with hybrid male sterility suggests that different genetic factors and molecular mechanisms are responsible for reproductive isolation in reciprocal crosses among species of the An. gambiae complex.
Introduction
Speciation is a fundamental evolutionary process that maintains the genetic and phenotypic differences among populations by means of reproductive isolation (Coyne and Orr, 2004). Reproductive isolation between species can be divided into two types: prezygotic and postzygotic (Mayr, 1942). Among them, intrinsic postzygotic isolation makes speciation irreversible by producing reduced sterility or viability of F1 hybrid offspring produced by closely related species (Muller, 1942). The accumulation of genetic mutations, which takes part in reproductive isolation, plays an important role during speciation. The accepted theoretical explanation about how hybrid incompatibility evolves is known as the Dobzhansky-Muller model, which was independently proposed by Dobzhansky (1937) and Muller (1942). The Dobzhansky-Muller model states that postzygotic isolation often results from incompatible mutations at two or more epistatically interacting loci in inter-species hybrids. In addition to the Dobzhansky-Muller explanatory model, several mutually related comparative rules have been identified in the evolution of intrinsic postzygotic isolation (Presgraves, 2010). First, with the divergence of two species, the strength of intrinsic postzygotic isolation in F1 hybrids accumulates gradually from zero to complete. Importantly, comparative data suggest and genetic data show that complete F1 hybrid sterility or inviability can often result from a few incompatible factors with a strong effect (Coyne et al., 1989; Presgraves, 2010). Second, according to Haldane’s rule (Haldane, 1922), F1 hybrid sterility or inviability commonly manifests in the heterogametic sex (XY- or ZW-type) during the early stage of speciation (Presgraves, 1998). Third, Darwin’s corollary to Haldane’s rule states that F1 hybrids from reciprocal inter-species crosses usually differ in the degree of sterility or lethality (Turelli and Moyle, 2007). The majority of research on how the intrinsic postzygotic isolation evolves have focused on Drosophila and information about phenotypes and mechanisms of hybrid sterility and inviability in other taxa is scarce.
African malaria mosquitoes of the Anopheles gambiae complex consist of at least nine sibling species (Coluzzi et al., 2002; Coetzee et al., 2013; Barron et al., 2019), which started diverging about 0.526 million years ago (Mya) (Thawornwattana et al., 2018) and, currently, have nearly indistinguishable morphologies but different ecological adaptations and epidemiological capacities. Early crossing experiments between members of the complex revealed various degrees of atrophy of the reproductive tract and sperm developmental defects in hybrid males (Davidson et al., 1967). It has been shown that these mosquito hybrids conform to Haldane’s rule and Darwin’s corollary (Presgraves, 1998). However, crosses between the more recently described incipient species An. gambiae and An. coluzzii [the former S and M forms, respectively (Coetzee et al., 2013)] produce fertile hybrids of both sexes (Diabaté et al., 2007; Aboagye-Antwi et al., 2015). G. Davidson and coauthors proposed the idea of releasing sterile hybrid males for the purpose of mosquito control. Females mated to sterile males would lay eggs that didn’t hatch leading to a decrease in population size. They established that these males competed successfully against pure-species males in mating with virgin females (Davidson et al., 1967). For such males to be effective in reducing mosquito populations, it is essential that females that mated with sterile males do not subsequently become inseminated by fertile males. To test these properties of hybrid males, consecutive mating experiments with mosquitoes of the An. gambiae complex were conducted (Bryan, 1972). In these experiments, the first cross was performed between a virgin female and a hybrid sterile male, and the second cross was done between the same female and a pure-species fertile male. These experiments showed that hybrid males from ♀ An. gambiae × ♂ An. merus or An. melas crosses failed to induce female monogamy; i.e., the females remained fertile and laid a normal number of eggs after consecutive mating with pure-species males. However, male hybrids from the reciprocal crosses succeeded in inducing female monogamy; i.e., the females became sterile for life (Bryan, 1972). The study suggested that the impaired secretory activity of the reduced male accessary glands (MAGs) in hybrid males of the ♀An. gambiae × ♂An. merus or An. melas cross was responsible for failing to induce female monogamy. Therefore, only hybrid males of the reciprocal cross could be used for control of mosquito populations. A deeper knowledge of the reproductive biology of mosquitoes would identify targets for the development of novel vector control strategies. Also, a better understanding of the developmental and behavioral phenotypes of inter-species mosquito hybrids will inform efforts focused on the control of malaria vectors through modern genetic approaches. A recent study highlighted the significant probability of CRISPR-based gene drives to spread into non-target species (Courtier-Orgogozo et al., 2020). Although introgression of gene drives into non-target species should be avoided, their spread to other malaria vectors could be beneficial. The possibility of introgression of an active synthetic sex ratio distortion system from An. gambiae to An. arabiensis has already been demonstrated (Bernardini et al., 2019). To predict and manage the potential introgression of transgenic constructs among target and non-target mosquito species, it is necessary to identify and exploit genetic factors responsible for maintaining species boundaries through hybrid sterility. Also, accurate characterization of phenotypes associated with the malfunctioning of inter-species hybrids is an important step toward understanding the evolution of reproductive barriers.
Studies of phenotypes associated with hybrid male sterility usually focus on testis shape, size, and weight, sperm morphology and behavior, as well as cellular aspects of spermatogenesis. Our recent work identifies and describes asymmetric cellular phenotypes in testes of hybrids from the reciprocal crosses between An. merus and An. coluzzii or An. gambiae. Male progeny of the cross when An. merus was the mother had abnormal chromosomal activities during meiosis, which resulted in non-motile diploid sperm. Male progeny of the reciprocal cross had arrested spermatogenesis at an early premeiotic stage (Liang and Sharakhov, 2019). However, male mosquito reproductive tracts include not only testes, but also MAGs, the ejaculatory duct, and the vasa deferentia. All these structures are important for male fertility and for inducing the postmating responses of females (Davidson et al., 1967; Baldini et al., 2012). To characterize possible malfunctions of the reproductive tracts associated with male sterility in the reciprocal inter-species hybrids, we performed crossing experiments using laboratory strains of An. merus MAF and An. gambiae ZANU or An. coluzzii MALI and MOPTI. We demonstrated that Darwin’s corollary to Haldane’s rule of speciation in the An. gambiae complex manifests itself in multiple phenomena in F1 hybrids including sex ratios, reproductive tract development, postcopulatory effects on females, mating plug transfer, and copulation time.
Materials and Methods
Mosquito Strains
Laboratory colonies were provided by the Malaria Research and Reference Reagent Resource (MR4) at the Biodefense and Emerging Infections Research Resources Repository (BEI). The strains used in this study included An. gambiae ZANU (MRA-594), An. coluzzii MOPTI (MRA-763), An. coluzzii MALI (MRA-860), and An. merus MAF (MRA-1156). Mosquitoes were reared at 27 ± 1°C, with a 12-h photoperiod and 70 ± 5% relative humidity. Larvae were fed with fish food. Although An. merus is a water-tolerant species, all larvae from pure species, hybrids, and backcrosses were maintained in fresh water with no apparent effect on survival rates, which is consistent with the previous research (White et al., 2013). Adult mosquitoes were fed with 10% sugar water. To stimulate egg development, females were fed on defibrinated sheep blood using artificial blood-feeders.
Crossing Experiments
To perform inter-species crosses, male and female pupae were differentiated and separated based on sex-specific differences in the morphology of their terminalia (Jones, 1957). Using the pupa instead of the adult stage helped us to avoid possible mating with virgin females. We checked the pupa sex 2–3 times after separation to ensure purity of the sex. Fifteen male and 30 female pupae were combined in one cage. After emergence, mass crossing experiments were performed between species. Crosses between An. gambiae and An. coluzzii were not conducted because they are known for not having postzygotic reproductive isolation (Diabaté et al., 2007; Hahn et al., 2012; Sawadogo et al., 2014). After 4–5 days of random mating, the females were fed on sheep blood. Two days later, an egg dish covered with moist filter paper was placed into the cage for female oviposition. Pictures of the eggs were taken and the number of eggs was counted using Egg Counter 1.0 software (Mollahosseini et al., 2012). To calculate the female to male sex ratio, the number of F1 pupae of each sex were counted each day. Backcrossing experiments were done using a similar method. Statistical analyses were performed using a binomial test following a Bonferroni approach to correct the P-values for correcting the Type I error.
Mating and Reinsemination Assays
Isolation of mating couples and reinsemination experiments were done as described previously (Thailayil et al., 2011) with slight modifications. Briefly, we separated male and female pupae and kept them in different cages. After emergence, 2–3-day-old virgin females were mixed with 4–6-day-old virgin hybrid males in one large cage. Copulating pairs, when they dropped to the cage floor, were gently covered with a 1 oz plastic portion cup and moved into another cage after they finished the copulation process. The copulation time was recorded. After mated females recovered for 2 days, they were transferred by a tube into a cage with five to tenfold excess of 4–6-day-old virgin An. coluzzii males. Two days later, females were fed on defibrinated sheep blood. After 1 day, twenty half-gravid females from each cross were put into small plastic tubes with moist filter paper inside to lay eggs individually. Egg numbers were counted manually and kept in water for 4 days to check the hatching rates. Videos of copulations were recorded using the camera of an iPhone 6s (Apple Technology company, Cupertino, United States).
Microscopic Analyses of Mosquito Reproductive Tracts and Mating Plugs
Male reproductive tracts were dissected in 1 × phosphate-buffered saline (PBS) solution using a Leica E24 stereomicroscope (Leica Camera, Wetzlar, Germany) and photographed using a Leica M165 FC fluorescent microscope with a LEICA DFC3000 G digital camera (Leica Camera, Wetzlar, Germany). The length and width of testes and MAGs from five to ten males were measured in centimeters using images with the ruler tool in Adobe Photoshop CS6 (Adobe Inc., San Jose, CA, United States). All our images had resolution of 300 pixels/inch. One centimeter on the images (1 cm) was equal to 118 pixels or to 0.625 mm of the actual object. The measurements were compared between hybrids and pure species and analyzed by JMP Pro 13 software (SAS, United States) using a ONE-WAY ANOVA approach. For the comparisons we are interested in, we further did post hoc test using Tukey’s HSD test with Sidak correction. To visualize mating plugs, atria of females were dissected in 1 × PBS solution within 2 h after mating with males using an Olympus SZ61 stereomicroscope (Olympus, Tokyo, Japan) and photographed by an Olympus BX41 phase-contrast microscope with a UC90 digital camera (Olympus, Tokyo, Japan). To observe autofluorescence of MAGs and mating plugs, a GFP filter from a Zeiss AXIO fluorescent microscope with an Axiocam 506 mono digital camera was used (Carl Zeiss AG, Oberkochen, Germany).
RNA Extraction and Reverse Transcribed Polymerase Chain Reaction (RT-PCR)
Male reproductive organs, including testes and MAGs, were dissected from thirty 0–12-h-old virgin adults of An. coluzzii MOPTI, An. merus MAF, and inter-species hybrids of the ♀An. coluzzii MOPTI × ♂An. merus and ♀An. merus × ♂An. coluzzii MOPTI crosses. Total RNA was extracted with a Direct-ZolTM RNA MiniPrep Kit (Zymo Research, Irvine, California, United States) and diluted in 50 μl diethyl pyrocarbonate (DEPC)-treated water. Gene expression was tested using two-step RT-PCR. cDNA for selected genes was generated using a SuperScriptTM III First-Strand Synthesis System (Invitrogen, Carlsbad, CA, United States). A 10 μl mixture (2 μl of total RNA, 1 μl of 50 μM oligo(dT)20 primer, 1 μl of 10 mM dNTP mix solution, and 6 μl of DEPC-treated water) was incubated at 45°C for 5 min. After being chilled on ice for at least 1 min, 10 μl of cDNA synthesis mix containing 2 μl of 10 × RT buffer, 1 μl of 25 mM MgCl2, 2 μl of 0.1 M dithiothreitol (DTT), 1 μl of RNaseOUTTM (40 U/μl), and 1 μl of SuperScriptTM III RT (200 U/μl) was added into each RNA/primer mixture. After incubation at 50°C for 50 min, reactions were terminated at 85°C for 5 min. Later, 1 μl of RNase H was added into each tube for a 20 min incubation at 37°C. Next, the synthesized cDNA was used for PCR. Each 20 μl PCR mix consisted of 1 μl cDNA, 10 μl 2X PlatinumTM II Hot-start PCR Master Mix (Invitrogen, Carlsbad, CA, United States), 0.5 μl of 10-μM forward and reverse primers, and water. PCR was performed in the C1000 TouchTM thermal cycler platform (Bio-Rad Laboratories, Hercules, CA, United States) starting with a 2 min incubation at 94°C followed by 32 cycles of 94°C for 15 s, 60°C for 30 s, 68°C for 10 s, 68°C for 1 min, and a final hold at 4°C. Amplification products were visualized in a 2% agarose gel and photographed under the same parameters for each gene.
Mosquito Mating Interruption Assay
Male and female pupae were separated and kept in different cages. After emergence, 2–3-day-old virgin females were mixed with 4–6-day-old virgin hybrid males into one large cage. To interrupt the mosquito copulation process, mating pairs, when they dropped to the cage floor, were gently and quickly covered with a 1 oz plastic portion cup. The cup with the cover was immediately taken out after 2–4, 8–10, or 14–15 s from the start of the mating process and shaken vigorously by hand to forcedly separate the copulating pairs. Separated males and females from nine mating pairs were dissected to check the formation and transfer of mating plugs.
Results
Sex Ratio Bias in the F1 Progeny Is Asymmetric Between the Reciprocal Inter-Species Crosses
Sex ratio bias can be associated with sex differences in organismal fitness. To test if sex ratio distortion exists in inter-species hybrids, we counted the numbers of male and female pupae in the F1 progeny from intra-species crosses within An. coluzzii MOPTI, An. coluzzii MALI, and An. gambiae ZANU as well as from reciprocal inter-species crosses between An. merus and An. coluzzii or An. gambiae. In total, we counted 1,890 female pupae and 2,073 male pupae in the F1 progeny from the intra-species crosses and 3,038 female pupae and 3,009 male pupae in the F1 progeny from the all inter-species crosses (Supplementary Table 1). Using a binomial test and Bonferoni approach to correct the Type I error, no significant sex ratio bias was observed in the progeny from the intra-species crosses except for one blood-feeding experiment with An. coluzzii MOPTI (P = 0.0007) and one with An. coluzzii MALI (P = 0.0222). By comparison, a significant sex ratio bias existed in the F1 progeny from 13 of 30 blood-feeding experiments involving all inter-species crosses (P ≤ corrected P-value, Supplementary Table 1). In addition, we observed an asymmetric pattern in the sex ratio distortion when the reciprocal inter-species crosses were compared (Figure 1A). All blood feeding experiments for the six inter-species crosses of female An. coluzzii or An. gambiae with male An. merus produced more females than males (♀/♂ > 1). In contrast, 15 of 17 blood feeding experiments for seven reciprocal inter-species crosses produced more males than females (♀/♂ < 1). A higher proportion of males was also observed in the progeny of pure species in 11 out of 15 blood feeding experiments for six crosses (Supplementary Table 1). To obtain insights about the temporal dynamics associated with the asymmetrical pattern of the sex ratios in the F1 hybrids from the reciprocal inter-species crosses, we further investigated the daily percentages of male pupae in F1 progeny from the crosses between An. coluzzii MOPTI and An. merus for 7 consecutive days when ♀ + ♂ pupae appearing each day = 100%. Interestingly, similar to An. coluzzii, male pupae in the F1 progeny from the ♀An. merus × ♂An. coluzzii cross tended to appear earlier than female pupae. We observed more males during days 1–3 (♂ > 50%), but typically more females in days 4–7 for these crosses. In contrast, the F1 hybrids from ♀An. coluzzii × ♂An. merus showed the opposite pattern with typically ♀ < 50% during days 1–3 (Figure 1B and Supplementary Table 2). To understand the statistical differences of daily emergence, we performed ONE-WAY ANOVA followed by Tukey HSD test with Sidak correction. There were statistically significant differences in the percentage of male pupae that appeared in day 1 between the An. coluzzii progeny (65.7%) and the progeny of ♀An. coluzzii × ♂An. merus (22.4%, ∗P = 0.0006 < 0.0170, the P-value by Sidak correction), as well as between the progeny of ♀An. merus × ♂An. coluzzii and ♀An. coluzzii × ♂An. merus (90%, △P = 0.0061 < 0.0170).
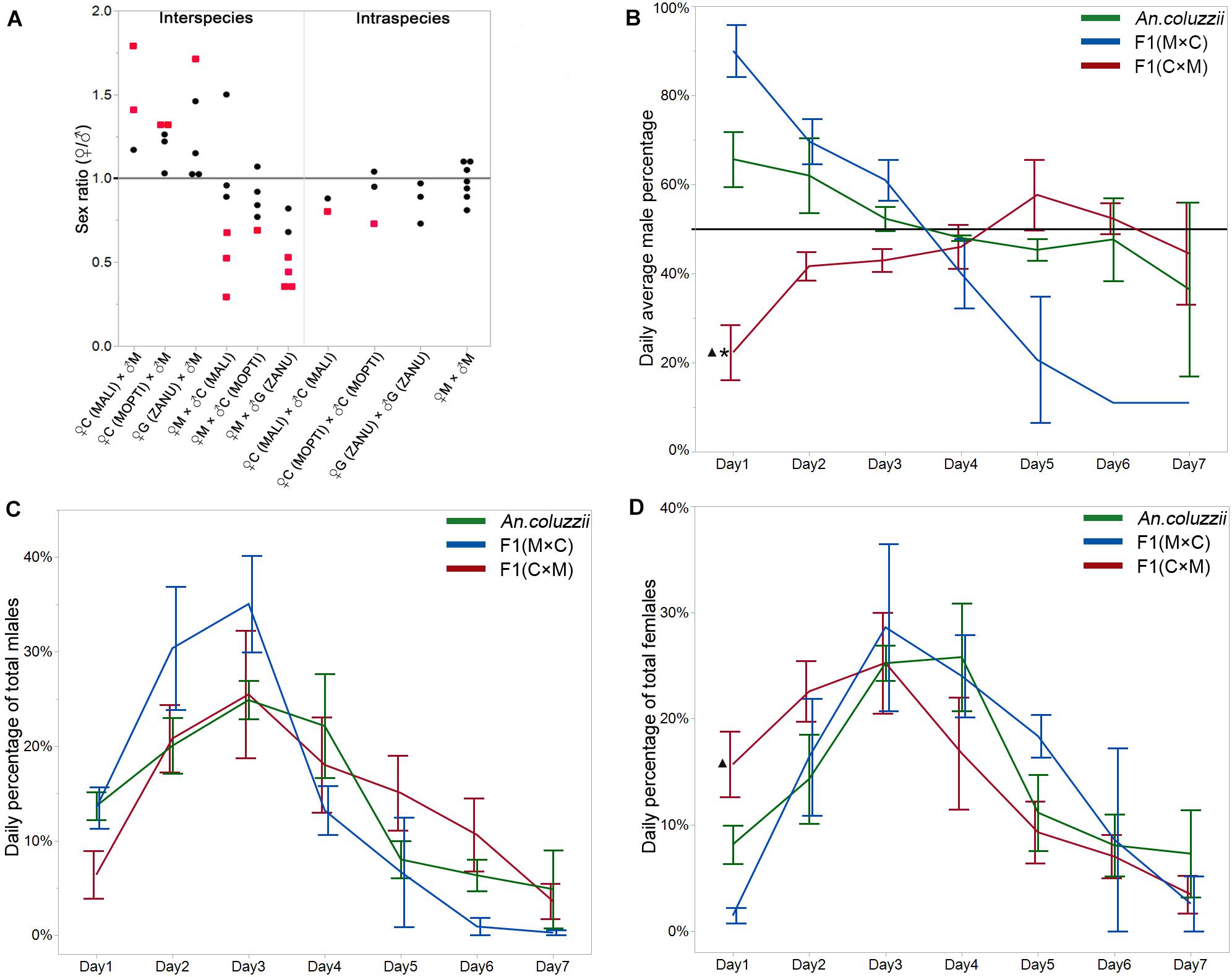
Figure 1. Sex ratios and daily percentages of each sex in the intra-and inter-species crosses. (A) Female-to-male sex ratios in the F1 generations from the inter- and intra- species crosses. Each dot represents the sex ratio value from a single cross. Red dots indicate statistically significant deviations from a sex ratio of 1. (B) Daily average percentage of male pupae in the F1 generations from inter- and intra-species crosses when newly appeared ♀ + ♂ each day = 100%. A horizontal line indicates a male percentage of 50%. (C) Daily average percentage of total males when the percentage of males for all 7 days combined = 100%. (D) Daily average percentage of total females when the percentage of females for all 7 days combined = 100%. *indicates statistical significance of data from the inter-species cross compared to pure species. ▲indicates statistical significance of data from the inter-species cross compared to the reciprocal inter-species cross. M, An. merus; C, An. coluzzii; G, An. gambiae. Names of the An. coluzzii and An. gambiae strains are shown in parentheses.
We observed that the F1 hybrids of the ♀An. coluzzii × ♂An. merus cross significantly differed from the F1 hybrids of the ♀An. merus × ♂An. coluzzii cross in their sex ratio bias (♀/♂ > 1 vs. ♀/♂ < 1) and in the temporal dynamics of the sex ratio during the first 3 days of pupation (♀ < 50% vs. ♂ < 50%). Is the observed pattern in the F1 progeny of the ♀An. coluzzii × ♂An. merus cross caused by slowly developing males or faster developing females? To understand the underlying cause for the difference in the temporal dynamics and in the asymmetrical pattern of the sex ratios between the reciprocal inter-species crosses, we further investigated the daily percentages of the total male and female pupae in F1, which is the percentage of each sex each day when the percentage of each sex for all 7 days combined = 100%. Surprisingly, using ONE-WAY ANOVA followed by Tukey HSD test with Sidak correction, we found that the patterns of male and female development were similar between An. coluzzii and both reciprocal crosses (Figure 1C and Supplementary Table 2). However, the average percentage of total F1 females appearing in day 1 in the ♀An. coluzzii × ♂An. merus cross was significantly higher (15.7%) than the average percentage of total F1 females appearing in day 1 in the reciprocal cross (1.4%) (△P = 0.0072 < 0.0170, the p-value by Sidak correction) (Figure 1D and Supplementary Table 2). This result indicates that the rate of female development is much faster in F1 hybrids of the ♀ An. coluzzii × ♂ An. merus cross than the opposite cross. It is possible that heterosis of the hybrid females rather than reduced fitness of the hybrid males explains the observed bias toward females in this cross. Thus, the somatic development of F1 hybrids can be affected in crosses involving species of the An. gambiae complex.
F1 Hybrids of the Inter-Species Crosses Largely Obey Haldane’s Rule
Next, we investigated the effect of inter-species hybridization on male fertility in our laboratory colonies. According to Haldane’s rule, the sterility of inter-species F1 hybrids usually affects heterogametic sex (Haldane, 1922). Therefore, we expected that hybrid Anopheles males carrying X and Y chromosomes should be sterile, but not hybrid females. To test Haldane’s rule, we performed crossing and backcrossing experiments between An. merus and An. gambiae ZANU or An. coluzzii MOPTI and MALI. Backcrossing experiments between F1 hybrids and parental species showed that most of the crosses between An. gambiae or An. coluzzii and An. merus produce sterile F1 males and fertile F1 females (Supplementary Table 3). The sterility of F1 hybrid males was manifested by the lack of hatching eggs laid by An. coluzzii or An. merus females that had mated with them. In addition, we tested whether motile sperm was present in the spermatheca of the mated females. Indeed, we observed sperm swirling around the perimeter of the spermatheca in females mated with pure-species males (Supplementary Video 1). Also, a multitude of mature sperm with actively moving tails were seen after crushing the spermatheca of such females (Supplementary Video 2). In contrast, we observed no mature sperm inside the spermatheca of females mated with hybrid males (Supplementary Video 3). However, immature sperm with larger heads and shorter tails were seen in the crushed spermatheca of one of 20 analyzed An. merus females mated with hybrid males from the ♀An. merus × ♂An. coluzzii MALI cross (Supplementary Figure 1). In addition, one backcrossing experiment between female An. merus and F1 hybrid males from the ♀An. merus × ♂An. coluzzii MOPTI cross produced one son (from 207 laid eggs) that survived to adulthood. This result indicates that escapees from hybrid male sterility are still possible in crosses involving species of the An. gambiae complex. Surprisingly, F1 hybrid females from the ♀An. gambiae ZANU × ♂An. merus cross failed to produce any eggs after three repeated backcrosses with either male An. merus or male An. gambiae ZANU (Table 1). The lack of oviposition by F1 hybrid females could be specific to inter-species crosses involving the female genotype of the ZANU laboratory strain, which may have more prominent behavioral constrains of hybrid females to mating or oviposition. F1 hybrid females from the ♀An. merus × ♂An. gambiae ZANU cross lay a normal number of fertile eggs (Supplementary Table 3). Overall, the performed inter-species hybridizations had a much greater negative impact on male fertility than on female fertility of F1 hybrids, thus, confirming Haldane’s rule for our laboratory strains.
F1 Male Hybrids of the Reciprocal Inter-Species Crosses Differ by the Degree of Reproductive Tract Abnormality
To investigate phenotypes associated with hybrid male sterility, we dissected male reproductive tracts including testes, MAGs, and ejaculatory ducts from young adults of An. merus, An. gambiae, and An. coluzzii as well as F1 hybrids from inter-species crosses. In the pure species, reproductive organs have similar shapes and sizes. MAGs have an oval shape and testes have a spindle-like shape. Each testis is connected to a MAG by a vas deferens that is further connected to the ejaculatory duct (Supplementary Figure 2 and Figure 2).
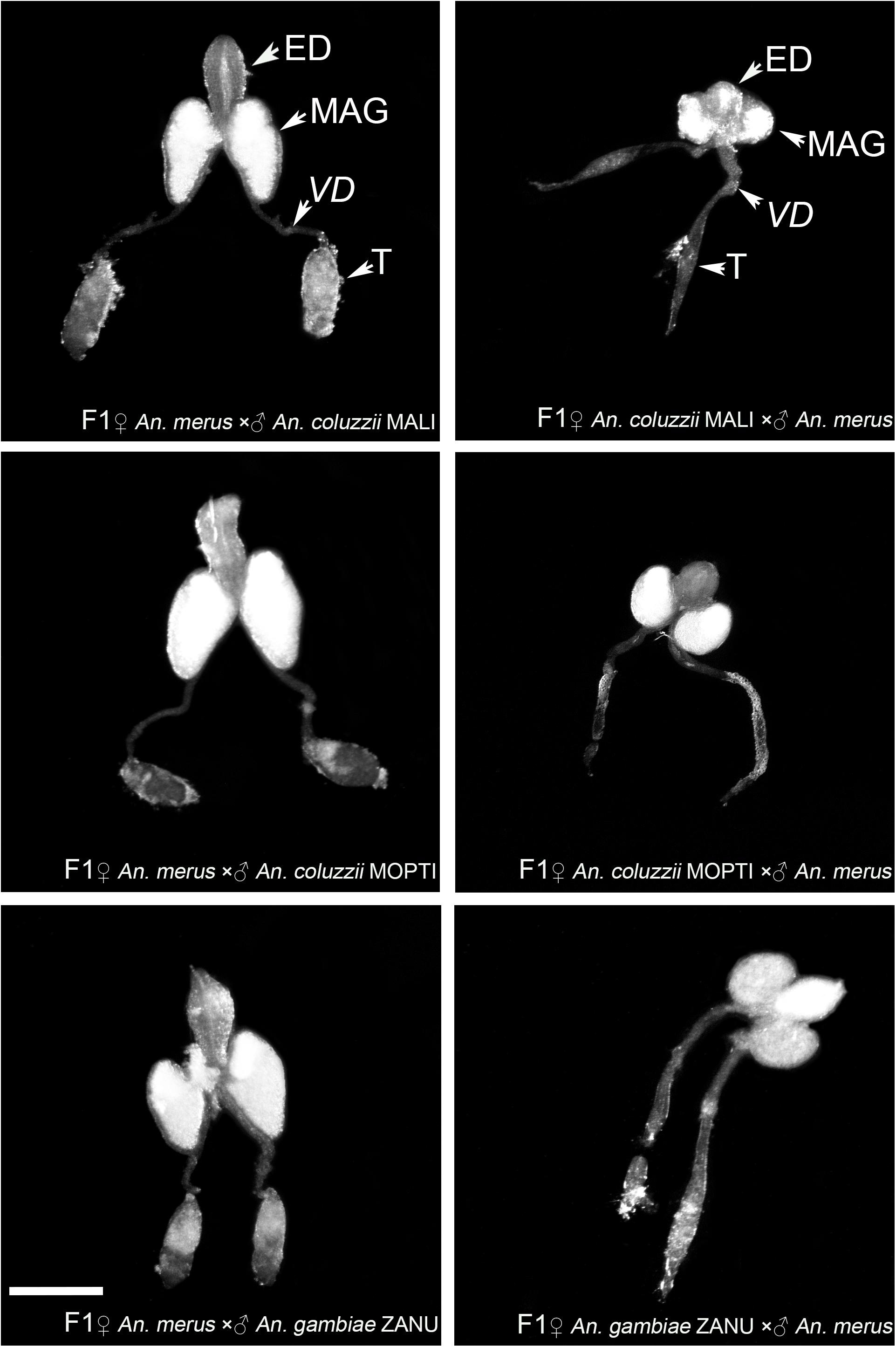
Figure 2. Morphology of the male reproductive tracts in inter-species hybrids. MAG, male accessary gland; ED, ejaculatory duct; T, testis; VD, vas deferens. Scale bar = 0.5 mm.
We found an obvious asymmetric pattern in the morphology and size of the MAGs, ejaculatory ducts, and testes when reciprocal crosses were compared. Hybrid males from crosses between female An. merus and male An. gambiae or An. coluzzii displayed normal looking reproductive organs. They were similar in shape and size with reproductive organs of pure species. In contrast, hybrid males from crosses between female An. gambiae or An. coluzzii and male An. merus showed severely underdeveloped reproductive organs. Visibly reduced round-shaped MAGs and ejaculatory ducts as well as thin testes were characteristics of the abnormal morphology of the reproductive tracts in these hybrids (Figure 2).
To quantify the observed differences, we recorded and compared the length and width of MAGs and testes from the pure species and the hybrids. Using ONE-WAY ANOVA, we found statistically significant difference (P-value < 0.0001) in both length and width of MAGs and testes. To test the difference between the pure species and the hybrids, we further performed post hoc test using Tukey’s HSD test with Sidak correction. Both the length and width of MAGs in F1 hybrids from the ♀An. coluzzii/gambiae × ♂ An. merus cross were significantly smaller than in pure species (P-value < 0.0051, based on Sidak correction, Supplementary Table 4), except for the width of MAG in hybrids from ♀An. coluzzii MOPTI × ♂ An. merus and length of testis from ♀An. gambiae ZANU × ♂ An. merus. In contrast, the length and width of MAGs in hybrids from the ♀An. merus × ♂ An. coluzzii/gambiae cross were slightly, but significantly, larger or similar to that of pure species (Figure 3A and Supplementary Table 4). Thus, F1 males from the ♀An. merus × ♂ An. coluzzii/gambiae cross had normal-like MAGs and F1 males from the ♀An. coluzzii/gambiae × ♂ An. merus cross had reduced MAGs. Our measurements and statistical analyses showed that the length and width of the pure-species testes were significantly larger than that of hybrid males from all interspecific crosses, especially from the ♀An. coluzzii/gambiae × ♂ An. merus cross (Figure 3B and Supplementary Table 4). The analysis of spermatogenesis in our previous work (Liang and Sharakhov, 2019) demonstrated that testes of hybrids from the ♀An. coluzzii/gambiae × ♂ An. merus cross lack spermatids or meiotic divisions and were classified as degenerate or atrophic. In contrast, testes of hybrids from the reciprocal cross had incomplete meiotic divisions producing abnormal spermatids, which were classified as normal-like or dysgenic.
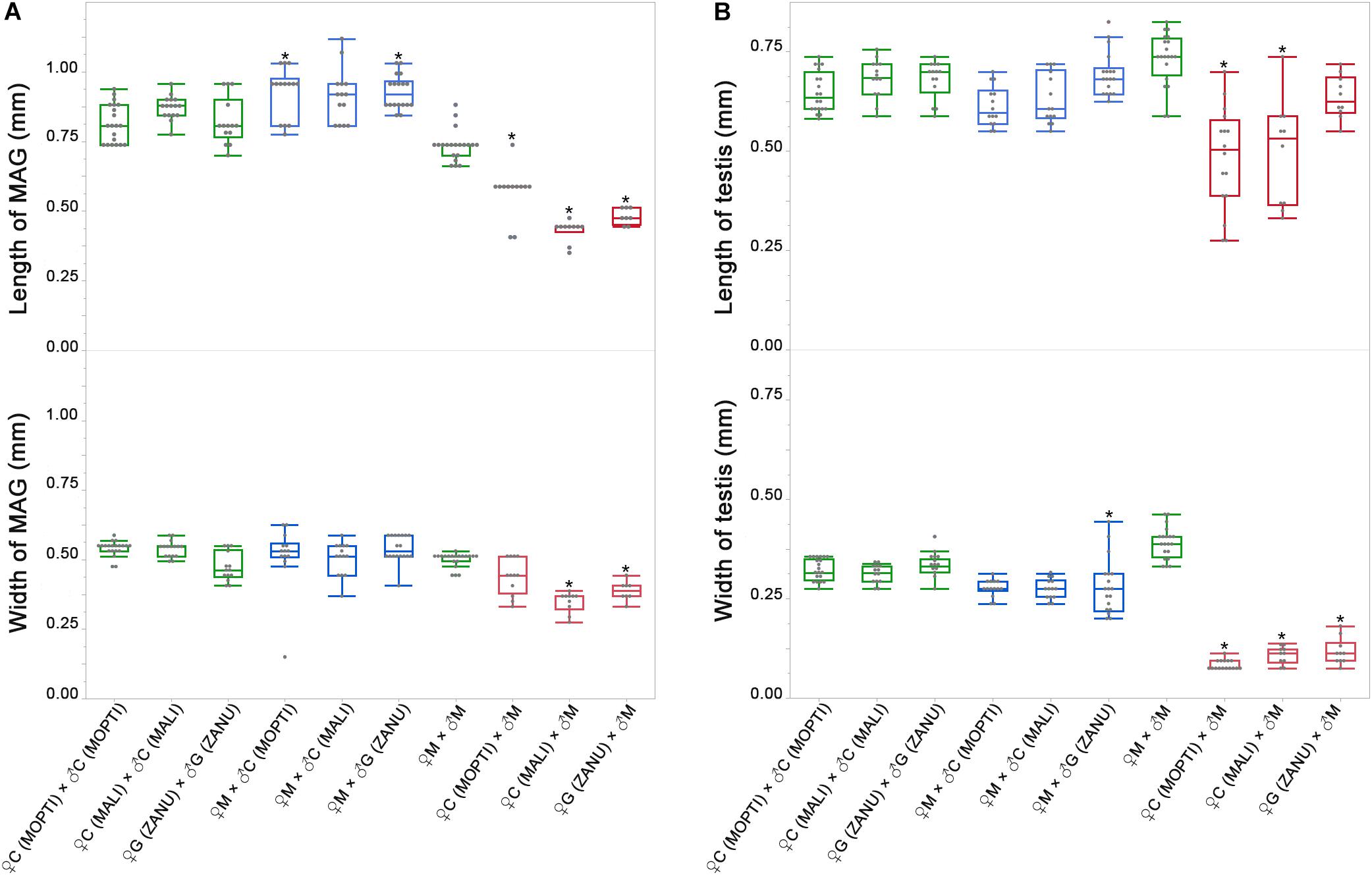
Figure 3. Widths and lengths of the male accessary glands (MAGs) and testes in pure species and in inter-species crosses. (A) Widths and lengths of MAGs in pure species and in inter-species crosses. (B) Widths and lengths of testes in pure species and in inter-species crosses. Green box plots represent measurements in pure species and blue and red box plots represent measurements in F1 hybrids. The lengths and widths of MAGs and testes are shown on the Y axes in mm. M, An. merus; C, An. coluzzii; G, An. gambiae. Names of the An. coluzzii and An. gambiae strains are in parentheses with capital letters. The Tukey’s HSD test was used to calculate P-values for comparison of the measurements between hybrids and pure species. *P < 0.0051, the P-value by Sidak correction.
Hybrid Sterile Males of the Reciprocal Inter-Species Crosses Differ in Their Ability to Induce Female Oviposition and Monogamy
It is known that the majority of once mated An. gambiae females become permanently refractory to further mating (Tripet et al., 2003). Mating also increases the number of eggs developed after a blood meal and induces a female’s ability to lay eggs (Klowden and Russell, 2004; Baldini et al., 2013). Receptivity to mating and oviposition are partially mediated by the steroid hormone 20-hydroxyecdysone (20E), which is synthesized in MAGs and transferred with the mating plug to the female’s atrium during copulation (Thailayil et al., 2011; Baldini et al., 2013; Gabrieli et al., 2014; Mitchell and Catteruccia, 2017). Since MAGs play important roles in inducing female post-mating responses, including oviposition and monogamy, we conducted backcrossing experiments and remating assays to test the function of normal-like and reduced reproductive organs of F1 hybrids from the reciprocal crosses. Our mass mating backcrossing experiment involving An. merus and An. coluzzii demonstrated that F1 hybrid males with normal-like reproductive organs induced females to lay a similar number of eggs as pure species males do. In contrast, mating with F1 hybrid males from the reciprocal crosses (which have reduced MAGs and ejaculatory ducts) resulted in significantly fewer eggs laid by females (Supplementary Figure 3). However, a similar tendency was not observed in crosses involving An. merus and An. gambiae ZANU. Anopheles gambiae ZANU females mated with F1 males from the ♀An. gambiae ZANU × ♂An. merus cross produced a similar number of eggs as An. gambiae ZANU females mated with An. gambiae ZANU males (t = -0.1035, df = 4, P = 0.9225). In fact, ZANU females typically laid fewer eggs than MOPTI or MALI females.
To investigate if there were differences between F1 males with two types of MAGs in inducing female monogamy, we conducted a remating assay as described previously (Thailayil et al., 2011). For this experiment, we generated F1 males by performing reciprocal crosses between An. coluzzii and An. merus. Two days later, An. coluzzii females were mated with F1 hybrid males from the reciprocal crosses. Then, the same females were remated with An. coluzzii males. To assess the effect of male reproductive tract development on induction of female monogamy, we counted the numbers of laid and hatched eggs. When females were first mated with F1 hybrid males with normal-like reproductive organs (the ♀An. merus × ♂An. coluzzii cross) and then remated with An. coluzzii males, 17 of 20 individual An. coluzzii females laid eggs but none of them hatched. By comparison, when females were first mated with F1 hybrid males with reduced reproductive organs (the ♀An. coluzzii × ♂An. merus cross) and then remated with An. coluzzii males, 15 of 20 An. coluzzii individual females laid eggs and the vast majority of these eggs hatched (Figure 4 and Supplementary Table 5). The results from both experiments demonstrate that F1 males with normal-like reproductive tracts are able to induce female oviposition and monogamy similarly to pure-species males. Hybrid males with reduced reproductive tracts fail to induce female oviposition and monogamy.
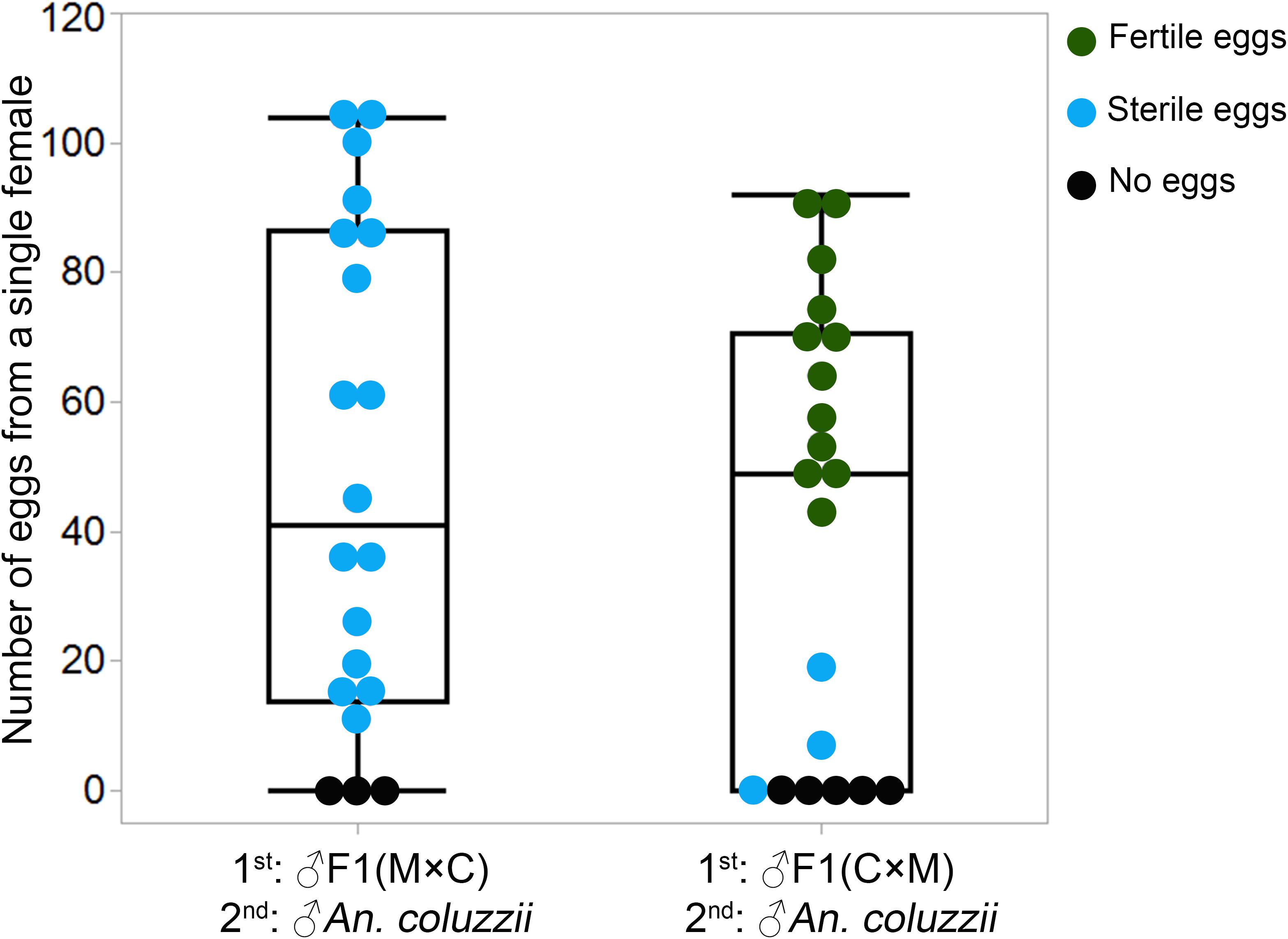
Figure 4. Number of eggs laid by each An. coluzzii female consecutively mated with individual males of inter-species hybrids and An. coluzzii. C × M, ♀An. coluzzii × ♂An. merus; M × C, ♀An. merus × ♂An. coluzzii.
The aforementioned post-mating responses of females are regulated in part by the male-synthetized 20E hormone that is packaged together with other seminal secretions into a gelatinous mating plug and transferred to the female atrium during mating (Rogers et al., 2009; Mancini et al., 2011; Gabrieli et al., 2014). Failure of the F1 males from the ♀An. coluzzii × ♂An. merus cross to induce female oviposition and monogamy points to problems with either synthesis or transfer of the 20E hormone during copulation. We proposed to test the following hypotheses to explain the observed phenomena. First, genes important for 20E hormone synthesis and mating plug formation could be strongly downregulated in collapsed MAGs. In fact, genes important for spermatogenesis were strongly downregulated in the atrophic testes of the such males (Liang and Sharakhov, 2019). A strong downregulation of MAG-specific genes could make it problematic for 20E hormone synthesis and mating plug formation. Second, the MAG-specific genes could function normally but the mating plug could not be formed and transferred to females due to the developmental abnormalities in somatic parts of the male reproductive organs. Below, we describe the experiments conducted to test these hypotheses.
Hybrid Sterile Males From the Reciprocal Inter-Species Crosses Differ in Their Ability to Form and Transfer Mating Plugs Into Atria of Females During Copulation
To understand the reason for the failure of hybrid males with collapsed MAGs to induce female post-mating responses, we asked if their MAGs expressed genes important for the 20E hormone pathway (AGAP004665-CYP306A1, AGAP005992-CYP302A1, AGAP000284-CYP315A1, and AGAP002429-CYP314A1), for mating plug formation (AGAP009368-plugin, AGAP009099-Tgase, AGAP008276-protease, AGAP013150-protease, AGAP005791-protease, AGAP007041-fibrinogen, and AGAP003083-lipase), and for the synthesis of the accessory gland proteins (AGAP006587-acp, AGAP009373-acp, and AGAP009362-acp). Primer sequences for RT-PCR are listed in Supplementary Table 6. Our RT-PCR results showed no apparent differences between pure-species and hybrid males from the reciprocal crosses in the pattern of expression of these genes (Figure 5), even though some of these genes, such as AGAP013150, AGAP006587, and AGAP009362, showed species-specific differences in the level of expression or size of the transcripts. We concluded that the failure of F1 males from the ♀An. coluzzii × ♂An. merus cross to induce female oviposition and monogamy is unlikely to be due to misexpression of the MAG-enriched genes.
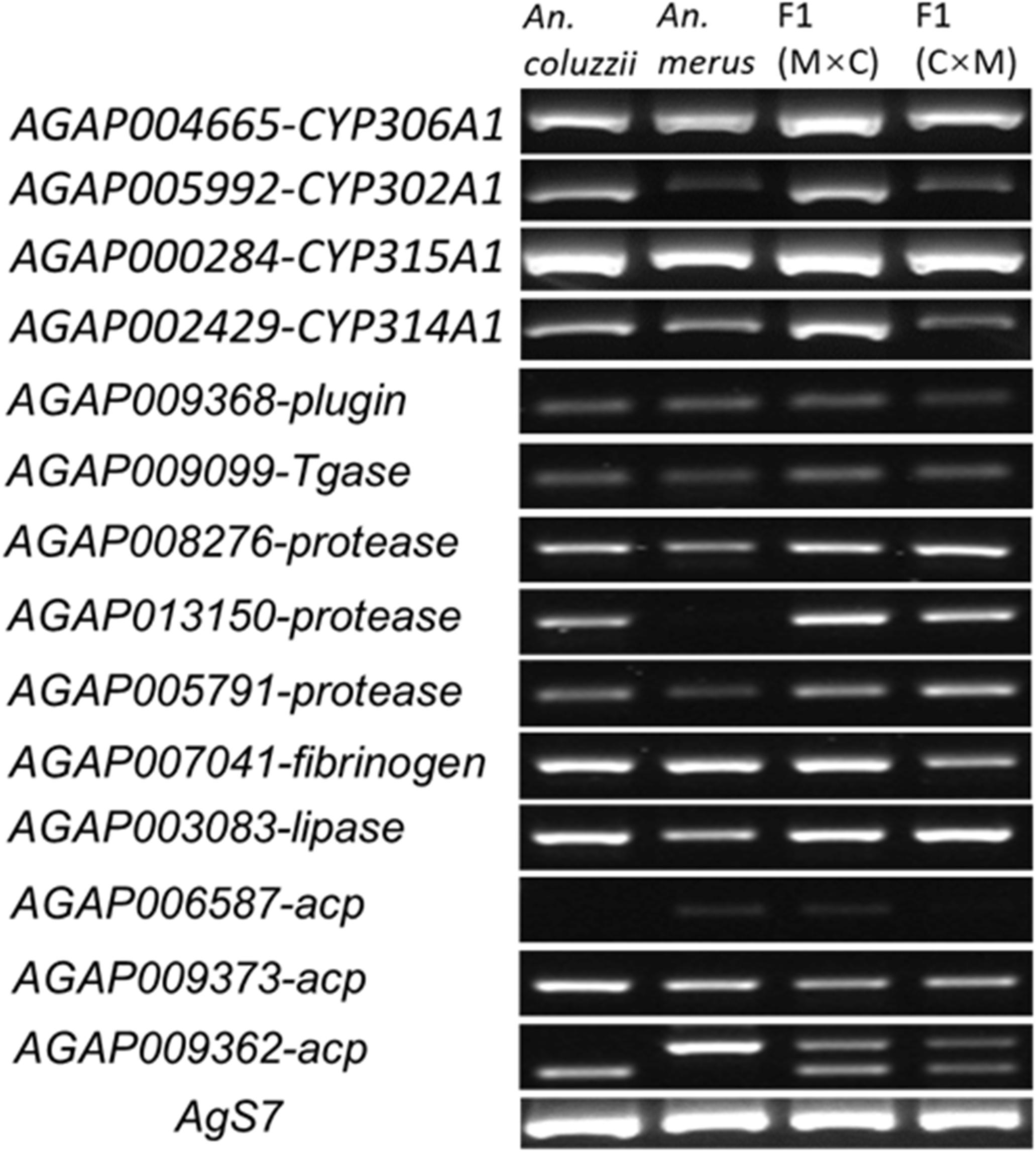
Figure 5. The RT-PCR analysis of gene expression in the male reproductive tract. M × C, ♀An. merus × ♂An. coluzzii MOPTI; C × M, ♀An. coluzzii MOPTI × ♂An. merus.
Next, we tested whether the mating plug could actually be formed and transferred to the female atrium during copulation. Lipids inside MAGs produce a strong autofluorescence that can be used to observe mating plug formation and transfer (Gabrieli et al., 2014). Using a fluorescent microscope with a GFP filter, we observed strong autofluorescence in MAGs of An. coluzzii and hybrid males obtained from the reciprocal inter-species crosses (Figure 6). This result agreed with our RT-PCR data (Figure 5) suggesting that the collapsed MAGs of the hybrid males were still able to perform their secretory function.
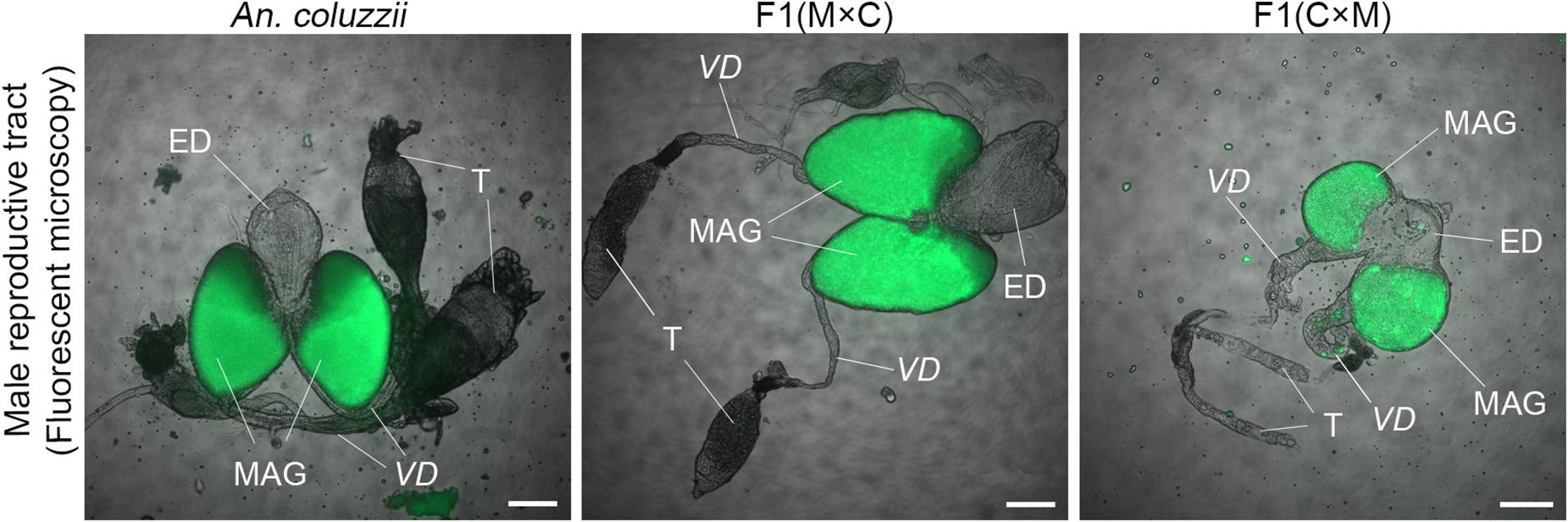
Figure 6. Autofluorescent MAG-derived lipids in the male reproductive tract. MAG-derived lipids autofluoresce in MAGs of pure species and all hybrids as well as in vasa deferentia of hybrid males from the ♀An. coluzzii × ♂An. merus cross. ED, ejaculatory duct; T, testis; VD, vas deferens; M × C, ♀An. merus × ♂An. coluzzii MOPTI; C × M, ♀An. coluzzii MOPTI × ♂An. merus. Scale bar = 100 μm.
Mating plugs normally have strong autofluorescence and can be observed in atria of females within 2 h post-mating (Smidler et al., 2018). Here, we tested if there were mating plugs in atria of An. coluzzii females after their mating with inter-species hybrid males and An. coluzzii males. Our results showed that An. coluzzii males as well as hybrid males with normal-like reproductive organs (the ♀An. merus × ♂An. coluzzii cross) produced and transferred mating plugs into female atria (Figure 7). In contrast, hybrid males with reduced reproductive organs (the ♀An. coluzzii × ♂An. merus cross) failed to produce and transfer plugs into the atria of mated females. The same data were obtained by observing the atria of mated females under a phase-contrast microscope (Figure 7). In fact, empty atria of females mated with hybrid males with collapsed MAGs looked similar to empty atria of virgin females (Supplementary Figure 4). We concluded that the failure of F1 males from the ♀An. coluzzii × ♂An. merus cross to induce female oviposition and monogamy is due to the inability of the males to form and transfer the mating plug to the atria of females during copulation.
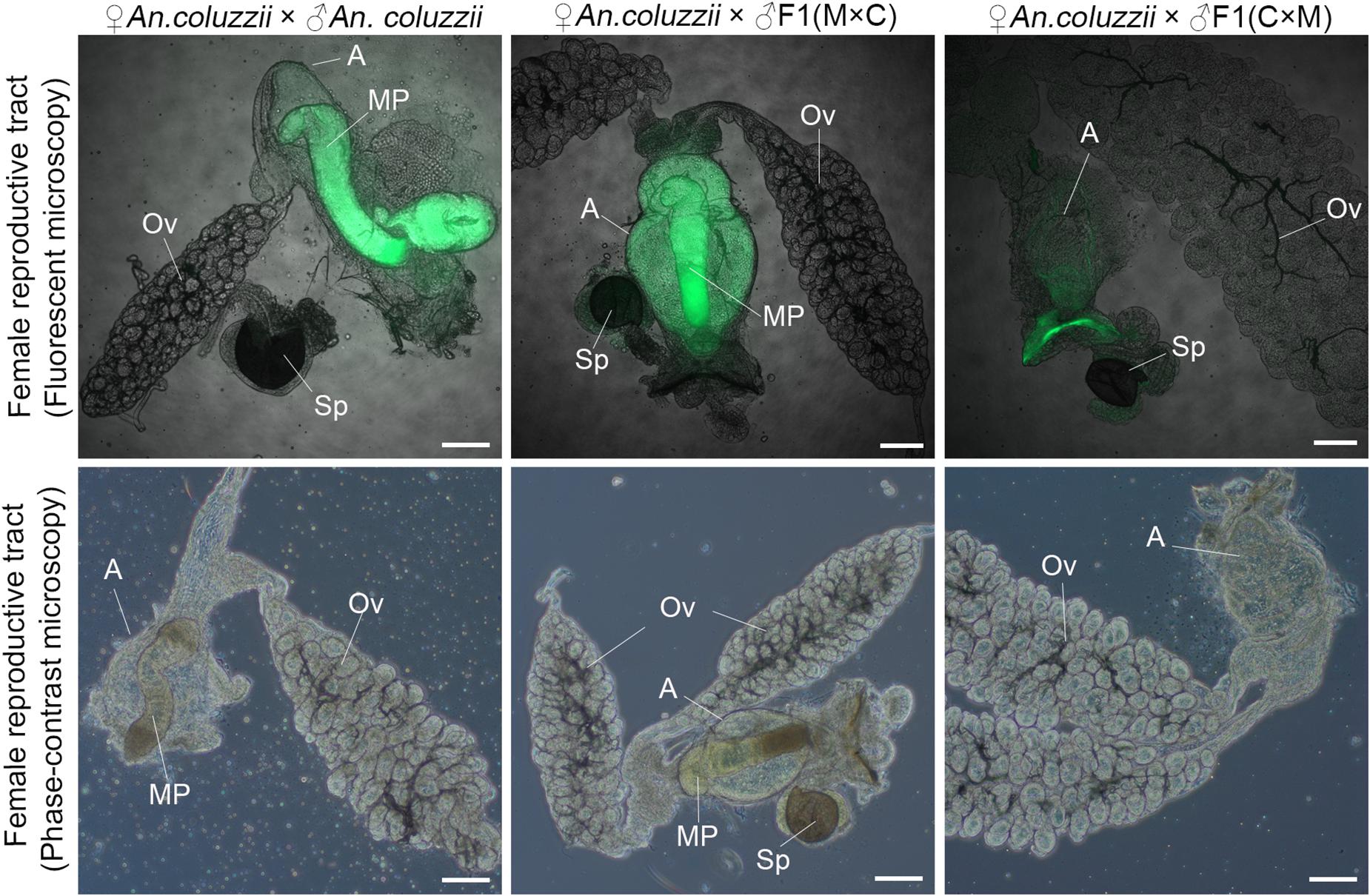
Figure 7. Analysis of the mating plug transfer to the atrium of An. coluzzii females. The presence or absence of the mating plug in the atria are shown for females mated with an An. coluzzii male and with hybrid males from the reciprocal inter-species crosses. MAG-derived lipids autofluoresce in green color. ED, ejaculatory duct; T, testis; Ov, ovary; A, atrium; MP, mating plug; Sp, spermatheca; M × C, ♀An. merus × ♂An. coluzzii MOPTI; C × M, ♀An. coluzzii MOPTI × ♂An. merus. Scale bar = 100 μm.
Since hybrid males from the ♀An. coluzzii × ♂An. merus cross cannot transfer the mating plug into females, we decided to investigate their reproductive tracts in greater detail. Using phase-contrast and brightfield microscopy, we examined MAGs, ejaculatory ducts, and vasa deferentia of mated males (Supplementary Figure 5). We observed that unlike An. coluzzii males or hybrid males from the ♀An. merus × ♂An. coluzzii cross, hybrid males from the ♀An. coluzzii × ♂An. merus cross typically had a strong accumulation of MAG-derived lipids in their vasa deferentia. Also, vasa deferentia in these males were usually thicker than in hybrid males from the reciprocal cross or in An. coluzzii males (Figure 6 and Supplementary Figure 5). We also noticed autofluorescence in the vas deferens of hybrid males from the ♀An. coluzzii × ♂An. merus cross (Figure 6). Moreover, testes of some hybrid males from this cross can have autofluorescence suggesting that MAG-derived lipids can flow in the wrong direction in the male reproductive tract (Supplementary Figure 6). These observations suggest that hybrid males from the ♀An. coluzzii × ♂An. merus cross have structural abnormalities of the ejaculatory duct or in its connection with MAGs. The specific characteristic of ejaculatory ducts in anopheline species is that they are heavily musculated (Hodapp and Jones, 1961). Because the ejaculatory duct is surrounded by large, well-tracheated, circular muscles, it is also called the ejaculatory pump (Clements, 1992), implying that it plays an active role in transferring the seminal fluid. It is possible that the severe underdevelopment of the ejaculatory duct in the ♀An. coluzzii × ♂An. merus hybrids prevents a proper transfer of the seminal fluid from MAGs to the ejaculatory duct and to female atria during mating. Instead, seminal fluid can flow into vasa deferentia and even into the atrophic testes. Thus, in addition to the germline tissue, the somatic tissue of reproductive organs can be negatively affected in these hybrid males.
Hybrid Sterile Males From the Reciprocal Inter-Species Crosses Differ in the Duration of Mating With Females
We asked if the failure of hybrid males from the ♀An. coluzzii × ♂An. merus cross to form and transfer mating plugs into females’ atria during copulation had an impact on mating behavior. We specifically tested whether the duration of mating between these hybrid males and An. coluzzii females (Supplementary Video 4) differed from the duration of mating between hybrid males from the reciprocal cross and An. coluzzii females (Supplementary Video 5). Our study showed that F1 males from the ♀An. merus × ♂An. coluzzii cross had copulation time similar (P = 0.3082) to that of pure species. These two groups of males copulated for about 20 s on average. However, hybrid males with reduced reproductive organs mated with An. coluzzii females for only about 10.5 s on average (Figure 8 and Supplementary Table 7), which is significantly shorter (P < 0.0001) than that for pure species and hybrid males from the reciprocal cross.
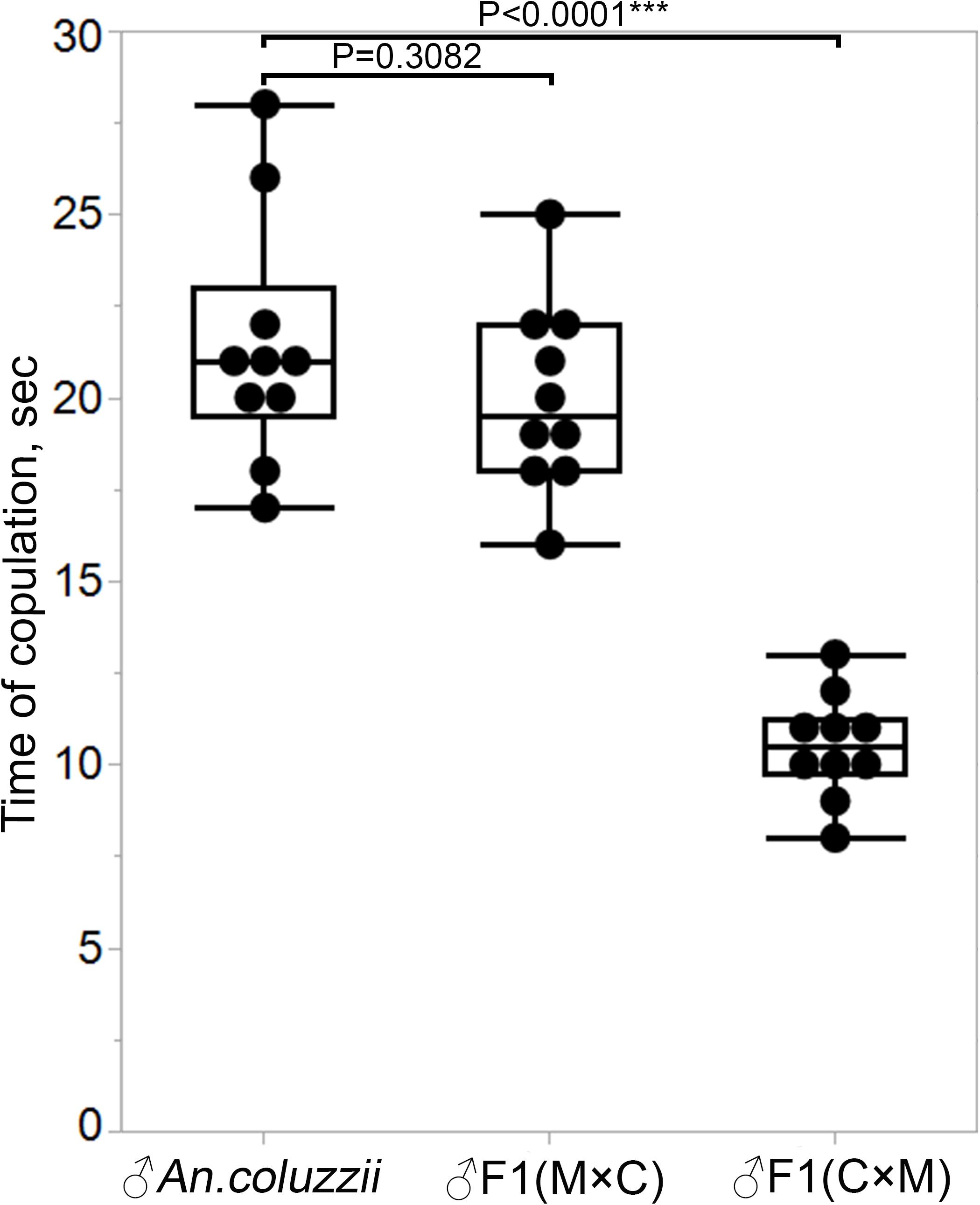
Figure 8. Copulation time between An. coluzzii females and males of An. coluzzii or F1 hybrids. M × C, ♀An. merus × ♂An. coluzzii; C × M, ♀An. coluzzii × ♂An. merus. The two-sample pooled t-test was used to calculate the P-value.
We next asked at what time the mating plug is formed and transferred to females during copulation in pure species. To answer this question, we conducted a mating interruption assay, in which we forcefully separated male and female An. coluzzii at 2–4, 8–10, and 14–15 s after they started copulation. The dissection of copulating pairs disrupted after 2–4 s found no mating plugs in either males or females. It was relatively easy to separate mating couples by shaking the cage at this stage. It was most difficult to interrupt mating at 8–10 s and we detected the already formed mating plug attached to the male aedeagus using phase-contrast microscopy (Figures 9A,B). Using a fluorescent microscope with a GFP filter, we confirmed the presence of the autofluorescent mating plug associated with the male (Figure 9C). The separated females did not have any mating plug material in their atria at 8–10 s after the start of copulation (Figure 9D). Thus, we found that the mating plug is being formed and transferred from the male into the female atrium at the midpoint after the start of copulation. However, the transfer was not completed by this time as the plug was not yet located in the female atrium but was still associated with the male aedeagus (Figure 9E). We also analyzed mating pairs separated at 14–15 s after the start of copulation. These females had mating plugs in their atria, indicating that the transfer was complete by this time. Thus, the process of plug formation and transfer probably determines the duration of mating in An. coluzzii. Hybrids from the ♀An. coluzzii × ♂An. merus cross did not produce mating plugs and, therefore, their mating with An. coluzzii females ended at ∼10.5 s after the start of copulation, when normally the mating plug is being formed and transferred into a female’s atrium.
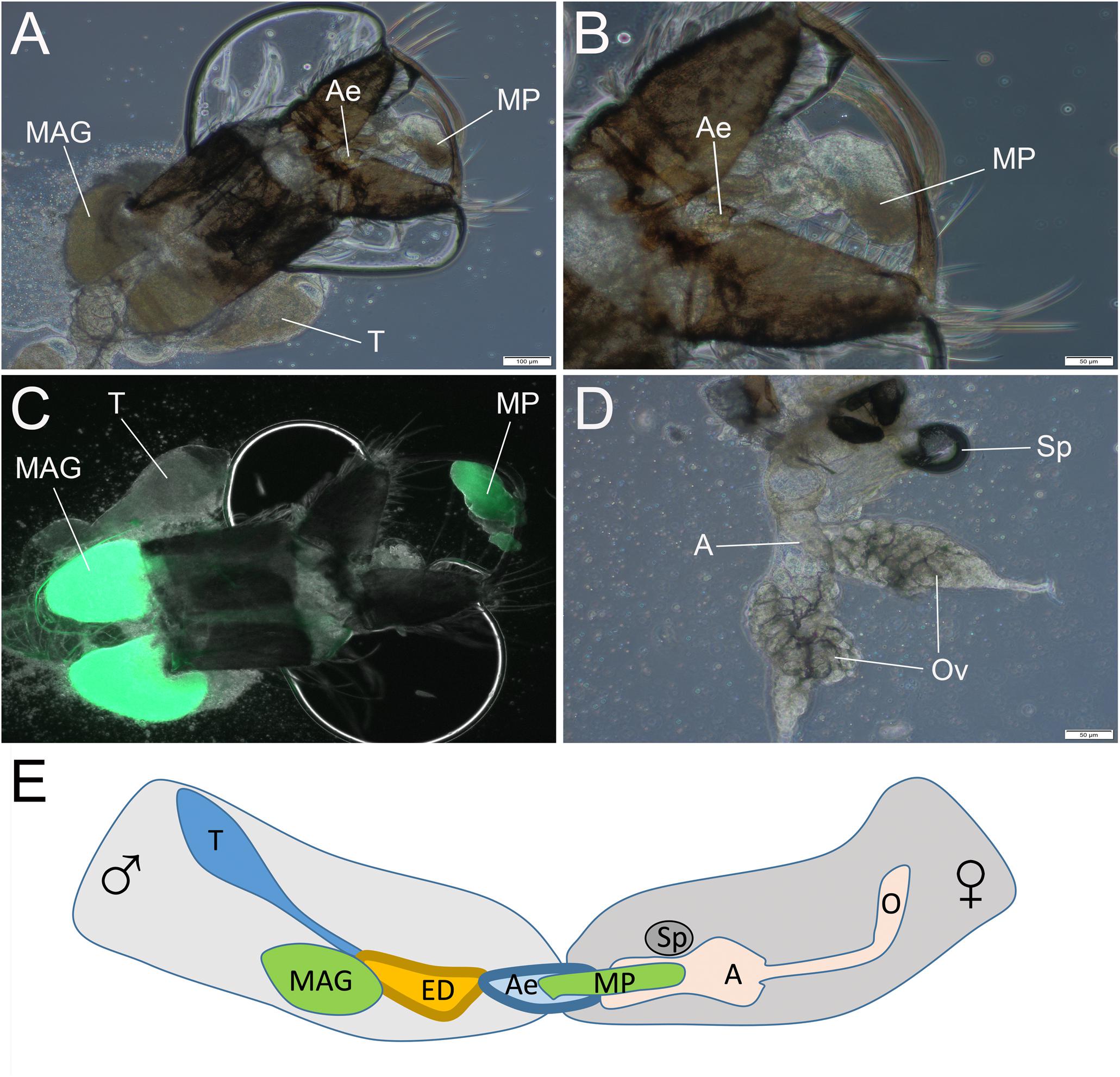
Figure 9. The transfer of the mating plug from male into female during copulation. The observation is done by disruption of mating at 8–10 s after the start of copulation. (A,B) The mating plug attached to the aedeagus. (C) The autofluorescent mating plug. (D) Absence of the mating plug in the atrium of the mated female. (E) Scheme showing the mating plug transfer from male into female atrium at 8–10 s after the start of the mating process. T, testis; MAG, male accessary gland; ED, ejaculatory duct; Ae, aedeagus; MP, mating plug; A, atrium; and Ov, ovary. Scale bars are 100 μm in (A,C) and 50 μm in (B,D).
Discussion
Charles Darwin, in the chapter on ‘Hybridism’ in the Origin of Species, articulated the generality and evolutionary significance of asymmetry in the degree of hybrid sterility: “The degree of sterility does not strictly follow systematic affinity, but is governed by several curious and complex laws. It is generally different, and sometimes wildly different, in reciprocal crosses between the same two species” (Darwin, 2001). This phenomenon is named “Darwin’s Corollary of Haldane’s Rule” (Turelli and Moyle, 2007). Multiple examples of hybrid asymmetry have been found across a wide range of taxa, including mammals (Good et al., 2007), fish (Bolnick and Near, 2005), toads (Brandvain et al., 2014), insects (Dobzhansky, 1934; Coyne and Orr, 1989; Presgraves, 1998; Presgraves, 2002), nematodes (Woodruff et al., 2010), plants (Scopece et al., 2007), and fungi (Dettman et al., 2003). It is relatively easy to observe and describe asymmetry of reciprocal F1s if one cross produces fertile hybrids and the other cross produces sterile hybrids. However, if both crosses produce sterile F1 males, a detailed comparative study must be performed to understand the causes of sterility in reciprocal crosses. For example, differences in the sizes and the internal structure of testes as well as in the process of meiosis have been described for sterile hybrid males obtained from reciprocal crosses between D. pseudoobscura and D. persimilis (Dobzhansky, 1934). Here, we identified and characterized multiple phenotypes of hybrids from reciprocal inter-species crosses between An. gambiae/coluzzii and An. merus. We specifically studied sex ratios, morphology, size of the male reproductive tract, postcopulatory effects on females, mating plug transfer, and mating behavior.
The identified asymmetric phenotypes of hybrids from the reciprocal crosses between species of the An. gambiae complex are summarized in Table 2. Briefly, the F1 hybrids of the ♀An. merus × ♂An. coluzzii cross resembled pure species, even though the F1 males were sterile. In contrast, all of the compared phenotypes were abnormal in the reciprocal cross. The opposite pattern of sex ratios in the F1 progeny from the reciprocal crosses observed in our experiments (Figure 1A) confirmed a similar trend of an early study using wild-caught An. merus and An. gambiae (Davison, 1964). Sex ratio distortion was also documented in F1 hybrids between laboratory strains of An. coluzzii and An. arabiensis (Slotman et al., 2004). Our further analysis suggested that the sex differences in organismal fitness may contribute to the asymmetric sex ratios between the reciprocal crosses. Specifically, our data point to the faster egg-to-pupae development in F1 females from the ♀An. coluzzii × ♂An. merus cross (Figures 1B–D). This phenotype is indicative of female heterosis that may skew the sex ratio toward females in this cross.
In our study, we observed asymmetry in the morphology of male reproductive organs of hybrids from the reciprocal inter-species crosses (Figure 2). Similar phenotypes have been reported in experimentally obtained hybrids using wild-caught species of the An. gambiae complex (Davidson et al., 1967). In addition, we demonstrated that the size of testes and MAGs of hybrids from the reciprocal crosses are significantly different from the size of testes and MAGs of pure species (Figure 3). Thus, our laboratory strains of An. merus, An. coluzzii, and An. gambiae produced F1 hybrids with the same abnormalities in their reproductive organs as those observed in natural populations. Another similarity between wild-caught and laboratory mosquito hybrids was observed in the experiments that tested their effect on female monogamy. Forced mating of wild-caught mosquitoes demonstrated that male hybrids from the ♀An. gambiae × ♂An. merus or An. melas crosses failed to induce female monogamy. Similarly, we have shown that F1 males from the ♀An. coluzzii × ♂An. merus cross involving laboratory colonies failed to induce female monogamy (Figure 4). We demonstrated that An. coluzzii and An. merus females that mated with hybrid males from the ♀An. coluzzii × ♂An. merus cross laid significantly fewer eggs than females mated with pure-species males or hybrid males from the reciprocal cross (Supplementary Figure 3). Because we did mass mating in this experiment and did not dissect individual females to count the number of developed eggs, we could not discriminate between the effect of the mating on increased egg production and inducing a female’s ability to lay eggs. Previous research demonstrated that male-transferred the 20E hormone causes an increased investment in egg production via a MISO-dependent pathway (Baldini et al., 2013). Also, transferring of 20E from a male to a female during mating induces oviposition (Thailayil et al., 2011). In any case, mating with hybrid males with small MAGs has a negative impact on the mosquito reproductive output. A previous study suggested that the inability of these males to induce female refractoriness to further copulations were due to impaired secretory activity of the MAGs (Bryan, 1972). However, our analysis of gene expression does not support this hypothesis. The RT-PCR results showed no apparent differences between pure-species and hybrid males from the reciprocal crosses in the pattern of expression of the MAG-enriched genes (Figure 5). Therefore, failure of the F1 males with reduced reproductive organs to induce female oviposition and monogamy is unlikely due to impaired secretory activity of the MAGs. Instead, we detected an abnormal flow of seminal fluid into vasa deferentia and even into the atrophic testes of some of these males (Figure 6 and Supplementary Figures 5, 6). Our study suggests that structural abnormalities of the ejaculatory duct or its connection with MAGs in the hybrid males from the ♀An. coluzzii × ♂An. merus cross prevented transfer of the mating plug to female atria during mating (Figure 7).
Failure of hybrid males from the ♀An. coluzzii × ♂An. merus cross to form and transfer mating plugs into females’ atria has a negative impact on mosquito mating behavior by significantly shortening the copulation time (Figure 8). Our study of the mating dynamics and plug transfer in An. coluzzii (Figure 9) agrees with an early study of forced mating using decapitated males of An. gambiae (Giglioli and Mason, 1966). Penetration of the female by the An. gambiae male’s aedeagus was followed by a quiescent phase of 5–6 s and then by a phase of the aedeagus moving backwards and forward in a pumping motion for about 15 s. When mating was interrupted just as the male’s reproductive organs began their pumping action, no mating plug was present in the atrium although the female was already inseminated. However, when mating was interrupted when male reached a state of excitation while continuing pumping, a mating plug was extruded from the aedeagus. Only when male and female separation occurred voluntarily was a mating plug found in the atrium of the female (Giglioli and Mason, 1966). Thus, the study with decapitated males of An. gambiae and our mating interruption assay with An. coluzzii both showed that the mating plug is still being formed and transferred into a female’s atrium around midpoint (∼8 to 10 s) during copulation (Figure 9). Our data suggest that the failure of mating plug formation caused the significant reduction in copulation time (∼10.5 s) between F1 males from the ♀An. coluzzii × ♂An. merus cross and the An. coluzzii females.
It is now clear that the reciprocal crosses between the same two species of the An. gambiae complex produced wildly different hybrid males. Compared with parental species, F1 males from the ♀An. coluzzii/An. gambiae × ♂An. merus cross suffered from serious defects of the reproductive tract including testes, MAGs, and the ejaculatory duct. The atrophic testes lacked any spermatogenic activity as cell division was arrested at the early pre-meiotic stage (Liang and Sharakhov, 2019). In contrast, somatic parts of the reproductive tract (MAGs, ejaculatory duct, and vasa deferentia) of F1 males from the ♀An. merus × ♂An. coluzzii/An. gambiae cross appear and function normally. Moreover, dysgenic testes of these hybrids can produce sperm although they are abnormal due to incomplete meiotic divisions (Liang and Sharakhov, 2019). Since only germ-line cells are affected in F1 males from the ♀An. merus × ♂An. coluzzii/An. gambiae cross, we believe that this male sterility is caused by incompatibility among testis-specific genes. In contrast, both somatic and germ-line cells are affected in F1 males from the reciprocal cross; therefore, incompatibility among a greater number of more broadly expressed genes likely cause the observed phenotypes. Since sterility of F1 males from the ♀An. coluzzii/An. gambiae × ♂An. merus cross reflects a more advanced stage of evolution, it would be more difficult to identify genes that initially caused hybrid sterility. Studying F1 males from the ♀An. merus × ♂An. coluzzii/An. gambiae cross, on the other hand, may lead to discovery of testis-specific genes that cause hybrid sterility at the early stage of speciation.
How can the Dobzhansky-Muller model contribute to our understanding of the observed asymmetric reproductive isolation in malaria mosquitoes? Since the model states that two or more epistatically interacting loci cause postzygotic isolation, at least some interacting loci should represent uniparentally inherited factors that are incompatible with other factors in inter-species hybrids. Such factors can be sex chromosomes, mitochondria, or epigenetically imprinted chromosomes (Turelli and Moyle, 2007). Among them, sex chromosomes received special attention from researchers (Payseur et al., 2018). The X chromosome disproportionately (in comparison to autosomes) contributes to sterility or lethality of heterogametic hybrids in multiple organisms (Coyne and Orr, 2004). The X chromosome is largely resistant to introgression between species, consistent with studies indicating that the X chromosome may harbor factors responsible for reproductive isolation (Coyne and Orr, 2004; White et al., 2009; Martin et al., 2013). The observed extensive autosomal but not X-chromosomal introgression between members of An. gambiae (Fontaine et al., 2015; Thawornwattana et al., 2018) predicts the relative paucity of hybrid sterility factors linked to autosomes. The “large X effect” has been confirmed by studies of hybrid male sterility in Anopheles mosquitoes. One of the first examinations of hybrid sterility in backcrosses of An. gambiae and An. arabiensis using the X-linked white-eye marker demonstrated the roles of the X chromosome and X-autosomal interaction in male hybrid sterility (Curtis, 1982). The large X chromosome effect on hybrid sterility has also been supported with a quantitative trait loci (QTL) mapping approach using crosses between An. coluzzii and An. arabiensis (Slotman et al., 2004), An. coluzzii and An. merus (Kay, 2017), and An. coluzzii and An. quadriannulatus (Deitz et al., 2020). The cumulative effect of the X chromosome accounted for greater than 60% of the phenotypic variation in An. coluzzii × An. quadriannulatus male hybrids. Interestingly, hybrid individuals harboring the An. coluzzii X chromosome had, on average, higher sterility scores than male hybrids with the An. quadriannulatus X chromosome (Deitz et al., 2020). This observation suggests that the origin of the X chromosome plays a major role in the phenotypic asymmetry of the inter-species hybrids. Because F1 males from the ♀An. coluzzii/An. gambiae × ♂An. merus crosses have more serious defects of the reproductive tracts than F1 males from the reciprocal crosses, it is reasonable to expect that the An. coluzzii or An. gambiae X chromosomes play a greater role in hybrid male sterility than the An. merus X chromosome, which agrees with the QTL mapping study (Kay, 2017). Due to the Xag and Xbcd inversions fixed between An. coluzzii and An. arabiensis as well as Xag inversion fixed between An. coluzzii and An. quadriannulatus (Coluzzi et al., 2002), the mapping of QTL within the X chromosome is impossible. This limitation does not apply to the An. coluzzii/An. gambiae × An. merus crosses as these species have collinear X chromosomes making possible mapping X-linked hybrid sterility genes.
Anopheles merus is the most basal lineage in the An. gambiae complex, which split from the other lineages about 0.526 Mya (Thawornwattana et al., 2018). Therefore, its chromosomes are expected to harbor the most ancestral alleles. The lineage leading to An. coluzzii and An. gambiae diverged from the other species in the complex about 0.509 Mya (Thawornwattana et al., 2018). Unlike An. merus, both An. coluzzii and An. gambiae have wide geographic distributions that overlap with the distributions of many other species within the complex. The X chromosome has likely played a major role in the development of reproductive isolation between sympatric species of the complex, which is seen from resistance of the X chromosome to inter-species gene flow (Fontaine et al., 2015; Thawornwattana et al., 2018). The X chromosomes of An. coluzzii and An. gambiae could have accumulated incompatibility factors after the split of the An. coluzzii/An. gambiae lineage from An. merus. As a result, we observed the asymmetry of phenotypes in F1 hybrids from the reciprocal crosses between An. coluzzii/An. gambiae × An. merus. Future studies of genetic factors contributing to hybrid male sterility in these reciprocal crosses will improve our understanding of the evolutionary processes and molecular mechanisms leading to speciation in the An. gambiae complex.
Data Availability Statement
The original contributions presented in the study are included in the article/Supplementary Material, further inquiries can be directed to the corresponding author/s.
Author Contributions
IVS conceived and coordinated the project, acquired funding for this study, and participated in the analyses. JL maintained mosquito colonies, performed crossing experiments, and conducted microscopic and behavioral analyses. JMH maintained mosquito colonies and did measurements of reproductive organs. The manuscript was prepared and approved by all the authors.
Funding
The study was supported by the Fralin Life Sciences Institute and the USDA National Institute of Food and Agriculture Hatch Project 223822 to IVS.
Conflict of Interest
The authors declare that the research was conducted in the absence of any commercial or financial relationships that could be construed as a potential conflict of interest.
Acknowledgments
The following mosquito strains were obtained through BEI Resources, NIAID, NIH: An. coluzzii, Strain Mali-NIH, Eggs, MRA-860 contributed by Nora J. Besansky; An. coluzzii, Strain MOPTI, Eggs, MRA-763 contributed by Gregory C. Lanzaro; An. gambiae, Strain ZANU, MRA-594 contributed by Hilary Ranson and Frank H. Collins; An. merus, Strain MAF, MRA-1156 contributed by Maureen Coetzee. We thank Janet Webster for proofreading the manuscript.
Supplementary Material
The Supplementary Material for this article can be found online at: https://www.frontiersin.org/articles/10.3389/fevo.2021.660207/full#supplementary-material
References
Aboagye-Antwi, F., Alhafez, N., Weedall, G. D., Brothwood, J., Kandola, S., Paton, D., et al. (2015). Experimental swap of Anopheles gambiae’s assortative mating preferences demonstrates key role of X-chromosome divergence island in incipient sympatric speciation. PLoS Genet. 11:e1005141. doi: 10.1371/journal.pgen.1005141
Baldini, F., Gabrieli, P., Rogers, D. W., and Catteruccia, F. (2012). Function and composition of male accessory gland secretions inAnopheles gambiae: a comparison with other insect vectors of infectious diseases. Pathog. Glob. Health 106, 82–93. doi: 10.1179/2047773212y.0000000016
Baldini, F., Gabrieli, P., South, A., Valim, C., Mancini, F., and Catteruccia, F. (2013). The Interaction between a Sexually Transferred Steroid Hormone and a Female Protein Regulates Oogenesis in the Malaria Mosquito Anopheles gambiae. PLoS Biol. 11:e1001695. doi: 10.1371/journal.pbio.1001695
Barron, M. G., Paupy, C., Rahola, N., Akone-Ella, O., Ngangue, M. F., Wilson-Bahun, T. A., et al. (2019). A new species in the major malaria vector complex sheds light on reticulated species evolution. Sci. Rep. 9:14753.
Bernardini, F., Kriezis, A., Galizi, R., Nolan, T., and Crisanti, A. (2019). Introgression of a synthetic sex ratio distortion system from Anopheles gambiae into Anopheles arabiensis. Sci. Rep. 9:5158.
Bolnick, D. I., and Near, T. J. (2005). Tempo Of Hybrid Inviability In Centrarchid Fishes (Teleostei: Centrarchidae). Evolution 59, 1754–1767. doi: 10.1554/04-563.1
Brandvain, Y., Pauly, G. B., May, M. R., and Turelli, M. (2014). Explaining Darwin’s Corollary to Haldane’s Rule: the Role of Mitonuclear Interactions in Asymmetric Postzygotic Isolation Among Toads. Genetics 197, 743–747. doi: 10.1534/genetics.113.161133
Bryan, J. H. (1972). Biological sciences: further Studies on Consecutive Matings in the Anopheles gambiae Complex. Nature 239, 519–520. doi: 10.1038/239519a0
Clements, A. N. (1992). The Biology of Mosquitoes: Development, Nutrition and Reproduction. London: Chapman &Hall.
Coetzee, M., Hunt, R. H., Wilkerson, R., Della Torre, A., Coulibaly, M. B., and Besansky, N. J. (2013). Anopheles coluzzii and Anopheles amharicus, new members of the Anopheles gambiae complex. Zootaxa 3619, 246–274. doi: 10.11646/zootaxa.3619.3.2
Coluzzi, M., Sabatini, A., della Torre, A., Di Deco, M. A., and Petrarca, V. (2002). A polytene chromosome analysis of the Anopheles gambiae species complex. Science 298, 1415–1418. doi: 10.1126/science.1077769
Courtier-Orgogozo, V., Danchin, A., Gouyon, P. H., and Boete, C. (2020). Evaluating the probability of CRISPR-based gene drive contaminating another species. Evol. Appl. 13, 1888–1905. doi: 10.1111/eva.12939
Coyne, J., Orr, H., Otte, D., and Endler, J. (1989). “Two rules of speciation,” in Speciation and its Consequences, eds D. Otte and J. A. Endler (Sunderland: Sinauer Associates), 180–207.
Coyne, J. A., and Orr, H. A. (1989). Patterns Of Speciation In Drosophila. Evolution 43, 362–381. doi: 10.2307/2409213
Curtis, C. F. (1982). “The mechanism of hybrid male sterility from crosses in the Anopheles gambiae and Glossina morsitans complexes,” in Recent Developments in the Genetics of Disease Vectors, eds W. M. Steiner, W. J. Tabachnick, K. S. Rai, and S. Narang (Champaign, IL: Stipes Publishing Company), 290–312.
Darwin, C. (2001). On the origin of species by means of natural selection or, The Preservation of favoured races in the struggle for life. Cambridge: Harvard University.
Davidson, G., Paterson, H. E., Coluzzi, M., Mason, G. F., and Micks, D. W. (1967). “The Anopheles gambiae Complex,” in Genetics of Insect Vectors of Diesease, eds J. W. Wright and R. Pal (Amsterdam: : Elsevier Publishing Company), 211–249.
Davison, G. (1964). Anopheles gambiae, a compplex of species. Bull. World Health Organ. 31, 625–634.
Deitz, K. C., Takken, W., and Slotman, M. A. (2020). The Genetic Architecture of Post-Zygotic Reproductive Isolation Between Anopheles coluzzii and An. quadriannulatus. Front. Genet. 11:925. doi: 10.3389/fgene.2020.00925
Dettman, J. R., Jacobson, D. J., Turner, E., Pringle, A., and Taylor, J. W. (2003). Reproductive isolation and phylogenetic divergence in Neurospora: comparing methods of species recognition in a model eukaryote. Evolution 57, 2721–2741. doi: 10.1554/03-074
Diabaté, A., Dabire, R. K., Millogo, N., and Lehmann, T. (2007). Evaluating the effect of postmating isolation between molecular forms of Anopheles gambiae (Diptera: Culicidae). J. Med. Entomol. 44, 60–64. doi: 10.1093/jmedent/41.5.60
Dobzhansky, T. (1934). Studies on hybrid sterility. I. Spermatogenesis in pure and hybrid Drosophila pseudoobscura. Zeitschr Zelljorsch U Mikrosk Anat 21, 169–223. doi: 10.1007/bf00374056
Fontaine, M. C., Pease, J. B., Steele, A., Waterhouse, R. M., Neafsey, D. E. I, Sharakhov, V., et al. (2015). Extensive introgression in a malaria vector species complex revealed by phylogenomics. Science 347:42.
Gabrieli, P., Kakani, E. G., Mitchell, S. N., Mameli, E., Want, E. J., Mariezcurrena Anton, A., et al. (2014). Sexual transfer of the steroid hormone 20E induces the postmating switch in Anopheles gambiae. Proc. Natl. Acad. Sci. U. S. A. 111, 16353–16358. doi: 10.1073/pnas.1410488111
Giglioli, M. E. C., and Mason, G. F. (1966). The mating plug in anopheline mosquitoes. Proc. R. Entomol. Soc. London A. 41, 123–129. doi: 10.1111/j.1365-3032.1966.tb00355.x
Hahn, M. W., White, B. J., Muir, C. D., and Besansky, N. J. (2012). No evidence for biased co-transmission of speciation islands in Anopheles gambiae. Philos. Trans. R. Soc. B Biol. Sci. 367, 374–384. doi: 10.1098/rstb.2011.0188
Haldane, J. B. S. (1922). Sex ratio and unisexual sterility in hybrid animals. J. Genet. 12, 101–109. doi: 10.1007/bf02983075
Hodapp, C. J., and Jones, J. C. (1961). The Anatomy of the Adult Male Reproduction System of Aedes aegypti (Linnaeus) (Diptera, Culicidae). Ann. Entomol. Soc. Am. 54, 832–844. doi: 10.1093/aesa/54.6.832
Good, J. M., Handel, M. A., and Nachman, M. W. (2007). Asymmetry and Polymorphism of Hybrid Male Sterility during the Early Stages of Speciation in House Mice. Evolution 62, 50–65.
Jones, J. C. (1957). A simple method for sexing living Anopheles larvae (Diptera. Culicidae). Ann. Entomol. Soc. Am. 50, 104–106. doi: 10.1093/aesa/50.1.104
Kay, R. (2017). Finding Genomic Hybrid Incompatibilities Between Members of the Anopheles gambiae Complex. Ph.D. thesis. University of California, Riverside.
Klowden, M. J., and Russell, R. C. (2004). Mating affects egg maturation in Anopheles gambiae Giles (Diptera: Culicidae). J. Vector Ecol. 29, 135–139.
Liang, J. T., and Sharakhov, I. V. (2019). Premeiotic and meiotic failures lead to hybrid male sterility in the Anopheles gambiae complex. Proc. R. Soc. B Biol. Sci. 286:20191080. doi: 10.1098/rspb.2019.1080
Mancini, E., Baldini, F., Tammaro, F., Calzetta, M., Serrao, A., George, P., et al. (2011). Molecular characterization and evolution of a gene family encoding male-specific reproductive proteins in the African malaria vector Anopheles gambiae. BMC Evol. Biol. 11:292. doi: 10.1186/1471-2148-11-292
Martin, S. H., Dasmahapatra, K. K., Nadeau, N. J., Salazar, C., Walters, J. R., Simpson, F., et al. (2013). Genome-wide evidence for speciation with gene flow in Heliconius butterflies. Genome Res. 23, 1817–1828.
Mayr, E. (1942). Systematics and the origin of species, from the viewpoint of a zoologist. Cambridge: Harvard University Press.
Mitchell, S. N., and Catteruccia, F. (2017). Anopheline Reproductive Biology: impacts on Vectorial Capacity and Potential Avenues for Malaria Control. Cold Spring Harb. Perspect. Med. 7:a025593. doi: 10.1101/cshperspect.a025593
Mollahosseini, A., Rossignol, M., Pennetier, C., Cohuet, A., Anjos, A. D., Chandre, F., et al. (2012). A user-friendly software to easily count Anopheles egg batches. Parasit. Vectors 5:122. doi: 10.1186/1756-3305-5-122
Payseur, B. A., Presgraves, D. C., and Filatov, D. A. (2018). Introduction: sex chromosomes and speciation. Mol. Ecol. 27, 3745–3748. doi: 10.1111/mec.14828
Presgraves, D. C. (1998). Haldane’s Rule in Taxa Lacking a Hemizygous X. Science 282, 952–954. doi: 10.1126/science.282.5390.952
Presgraves, D. C. (2002). A Fine-Scale Genetic Analysis of Hybrid Incompatibilities in Drosophila. Genetics 163, 955–972. doi: 10.1093/genetics/163.3.955
Presgraves, D. C. (2010). Darwin and the Origin of Interspecific Genetic Incompatibilities. Am. Nat. 176, S45–S60.
Rogers, D. W., Baldini, F., Battaglia, F., Panico, M., Dell, A., Morris, H. R., et al. (2009). Transglutaminase-mediated semen coagulation controls sperm storage in the malaria mosquito. PLoS Biol. 7:e1000272. doi: 10.1371/journal.pbio.1000272
Sawadogo, P., Namountougou, M., Toé, K., Rouamba, J., Maïga, H., Ouédraogo, K., et al. (2014). Swarming behaviour in natural populations of Anopheles gambiae and An. coluzzii: review of 4 years survey in rural areas of sympatry, Burkina Faso (West Africa). Acta Trop. 132, S42–52.
Scopece, G., Musacchio, A., Widmer, A., and Cozzolino, S. (2007). Patterns of reproductive isolation in Mediterranean deceptive orchids. Evolution 61, 2623–2642. doi: 10.1111/j.1558-5646.2007.00231.x
Slotman, M., Della Torre, A., and Powell, J. R. (2004). The genetics of inviability and male sterility in hybrids between Anopheles gambiae and An. arabiensis. Genetics 167, 275–287. doi: 10.1534/genetics.167.1.275
Smidler, A. L., Scott, S. N., Mameli, E., Shaw, W. R., and Catteruccia, F. (2018). A transgenic tool to assess Anopheles mating competitiveness in the field. Parasit. Vectors 11, 651.
Thailayil, J., Magnusson, K., Godfray, H. C., Crisanti, A., and Catteruccia, F. (2011). Spermless males elicit large-scale female responses to mating in the malaria mosquito Anopheles gambiae. Proc. Natl. Acad. Sci. U. S. A. 108, 13677–13681. doi: 10.1073/pnas.1104738108
Thawornwattana, Y., Dalquen, D., and Yang, Z. (2018). Coalescent Analysis of Phylogenomic Data Confidently Resolves the Species Relationships in the Anopheles gambiae Species Complex. Mol. Biol. Evol. 35, 2512–2527. doi: 10.1093/molbev/msy158
Tripet, F., Toure, Y. T., Dolo, G., and Lanzaro, G. C. (2003). Frequency of multiple inseminations in field-collected Anopheles gambiae females revealed by DNA analysis of transferred sperm. Am. J. Trop. Med. Hyg. 68, 1–5. doi: 10.4269/ajtmh.2003.68.1.0680001
Turelli, M., and Moyle, L. C. (2007). Asymmetric Postmating Isolation: darwin’s Corollary to Haldane’s Rule. Genetics 176, 1059–1088. doi: 10.1534/genetics.106.065979
White, B. J., Kundert, P. N., Turissini, D. A., Van Ekeris, L., Linser, P. J., and Besansky, N. J. (2013). Dose and developmental responses of Anopheles merus larvae to salinity. J. Exp. Biol. 216, 3433–3441. doi: 10.1242/jeb.087189
White, M. A., Ane, C., Dewey, C. N., Larget, B. R., and Payseur, B. A. (2009). Fine-scale phylogenetic discordance across the house mouse genome. PLoS Genet. 5:e1000729. doi: 10.1371/journal.pgen.1000729
Keywords: mosquito, Anopheles gambiae complex, hybrid male sterility, Haldane’s rule, male accessary glands
Citation: Liang J, Hodge JM and Sharakhov IV (2021) Asymmetric Phenotypes of Sterile Hybrid Males From Reciprocal Crosses Between Species of the Anopheles gambiae Complex. Front. Ecol. Evol. 9:660207. doi: 10.3389/fevo.2021.660207
Received: 12 February 2021; Accepted: 17 May 2021;
Published: 10 June 2021.
Edited by:
Alessandro Minelli, University of Padua, ItalyReviewed by:
Paolo Gabrieli, University of Milan, ItalyDiego Ayala, Institut de Recherche Pour le Développement (IRD), France
Copyright © 2021 Liang, Hodge and Sharakhov. This is an open-access article distributed under the terms of the Creative Commons Attribution License (CC BY). The use, distribution or reproduction in other forums is permitted, provided the original author(s) and the copyright owner(s) are credited and that the original publication in this journal is cited, in accordance with accepted academic practice. No use, distribution or reproduction is permitted which does not comply with these terms.
*Correspondence: Igor V. Sharakhov, aWdvckB2dC5lZHU=