- Department of Zoology, University of Oxford, Oxford, United Kingdom
Seabirds care for their offspring in remote breeding colonies where foraging sites are distant and may be unpredictable, and where chicks are left unaccompanied for extended periods during their parents’ foraging trips, leaving them vulnerable to predation or starvation. One way to mitigate this risk is for individuals to coordinate parenting duties with their partner. Many biparental and cooperatively breeding species are now known to coordinate their care, though the mechanisms underlying this are not well understood. In Manx shearwaters (Puffinus puffinus), both parents alternate shifts of incubation in a coordinated manner. To resolve which processes may underlie this routine, we imposed a wing-loading handicap on parents to reduce their foraging efficiency, forcing them to choose between an extended foraging trip or to return to the nest before their condition has recovered to optimal levels. We found that handicapped parents took significantly longer trips than normal, to which their partner responded by lengthening their incubation shift, suggesting shift durations are not pre-determined. However, the duration of foraging trips and the mass at which foraging birds returned to the nest appeared to be mediated by the condition of the partner. These results suggest that while foraging trip duration is largely driven by the need for the foraging bird to recoup its own condition losses, information-transfer between the parents may facilitate a more cooperative mechanism whereby the decisions made by foraging birds still account for the condition of their partner.
Introduction
In species exhibiting shared parental care, coordinating behaviour between all carers can increase parental investment efficiency, either by maximising benefits to offspring (e.g., through more adaptive food delivery: Raihani et al., 2010) or by minimising carer costs (e.g., by reducing predation: Foster and Treherne, 1981; Raihani et al., 2010). Behavioural coordination is apparent in a range of species exhibiting joint parental care, and manifests in, for example, synchronous nest visitation (e.g., prairie voles Microtus ochrogaster, Ahern et al., 2011), turn-taking behaviour (e.g., rufous hornero Furnarius rufus, Massoni et al., 2012), or correlation in levels of parental effort (e.g., convict cichlid fish Amatitlania nigrofasciata, Itzkowitz et al., 2002). However, the specific mechanisms which allow parents to divide the tasks associated with raising offspring have received little attention.
Coordination has been experimentally manipulated in a number of species, usually by altering levels of parental investment, for example by increasing brood size (e.g., Mariette and Griffith, 2015) or by imposing a handicap on one parent (e.g., Wright and Cuthill, 1990; Dearborn, 2001). Through such studies, coordination has been found to be variably flexible across species, and achieved through a spectrum of responsiveness of carers to one-another’s behaviour. At one extreme, coordination is active, and may be underlain by communication or negotiation between the parents. For example, in turn-taking ceremonies, components of the display may signal individual quality (e.g., great tits Parus major, Boucaud et al., 2016; common murres Urie aalge, Takahashi et al., 2017), and therefore provide information which allows the partner to mediate its own behaviour, or indicate readiness to take on parental duties (e.g., zebra finches Taeniopygia guttata, Boucaud et al., 2017). Active coordination may also arise when carers adjust their own behaviour to coincide or synchronise with that of other individuals: for example, cooperatively breeding pied babblers (Turdoides bicolor) wait for other carers to arrive before provisioning the nest together (Raihani et al., 2010). Elsewhere, apparent coordination is a passive consequence of, for example, limitations on inter-feed intervals due to commuting distance (e.g., chestnut-crowned babbler Pomatostomus ruficeps, Savage et al., 2017), individual responses to body condition (e.g., blue petrel Halobaena caerulea, Chaurand and Weimerskirch, 1994) or as a secondary consequence of other behaviours (e.g., Adélie penguins, Pygoscelis adeliae, select partners with similar preferred foraging trip durations to their own; Davis, 1988).
Seabirds are obligate biparental carers which provide for their offspring under extreme conditions. Long distance and often unpredictable foraging locations require parents to spend long periods away from the nest, leaving their offspring at risk of predation, exposure, and starvation. Consequently, seabirds are under especially strong selection to coordinate their parenting behaviour, though, notably, extreme environmental conditions may themselves preclude coordination (Bolton, 1995; Hamer et al., 1998). Indeed, as seabirds are long-lived and primarily monogamous, the two parents’ reproductive interests may be so intertwined that poor performance or “laziness” by one could theoretically lead to complete compensation by the other, since the alternative is total reproductive failure (Jones et al., 2002). Increasing empirical evidence shows that parental coordination is widespread in seabirds (great frigatebird Fregata minor, Dearborn, 2001; wedge-tailed shearwater Ardenna pacifica, Congdon et al., 2005; little auk Alle alle, Welcker et al., 2009; little penguin Eudyptula minor, Saraux et al., 2011; Manx shearwater Puffinus puffinus, Tyson et al., 2017). However, few studies have investigated how coordination is actually achieved.
The Manx shearwater is a Procellariiform seabird in which both males and females contribute to offspring care. During incubation, both parents take turns to alternate between incubating their single egg and foraging at sea for the total 51-day period (Brooke, 1978). To counter the commuting cost of reaching long distance foraging sites (approximately 215 km, Guilford et al., 2008; Dean et al., 2015), shearwaters must spend several days foraging at sea (approximately 7; Brooke, 1990). While the ability of shearwater eggs to withstand a break in incubation of up to 11 days (Brooke, 1990) means that temporary neglect of the egg can form part of a successful breeding attempt, this still entails a cost in terms of predation and damage risk, and slowed embryonic development (Matthews, 1954). As failed eggs are not replaced (Brooke, 1978), unsuccessful incubation represents breeding failure for the entire year. The two parents are consequently faced with an intrinsically linked dilemma: while the foraging bird must spend sufficiently long at sea to recover its body condition, it must return to incubate before its partner decides to neglect the egg. The low incidence of neglect in Manx shearwaters (Brooke, 1990; Shoji et al., 2011), as well as the observation that parents very rarely overlap at the nest during incubation, a decision that would reduce foraging efficiency for the parent that should have departed to forage, suggests that incubation is well coordinated in this species. However, the mechanisms underlying this have not been explored.
It is not known where the incubation scheduling of Manx shearwaters lies on the spectrum of coordination. To investigate this, we used a classic handicapping paradigm (e.g., Sanz et al., 2000; Paredes et al., 2005; Bijleveld and Mullers, 2009; Ratz et al., 2019) to disrupt the behaviour of parents. We forced parents beyond the extremes of their decision-making processes by introducing a physical constraint on locomotion using a wing-loading handicap, forcing them to choose between extending the foraging trip to compensate, or to return before they have fully recovered their body condition. We then assessed the partner’s response to a change in behaviour of the focal bird to determine the degree of responsiveness of shearwaters to their partners’ behaviour. For example, if parents actively negotiate their shifts, they should form expectations about the duration of each incubation shift and corresponding foraging trip. If shift duration was therefore pre-determined, we expected that either the incubating parent would choose to neglect the egg when its partner did not return when expected, or that the foraging parent would return to the nest well before its body condition had been sufficiently recouped. Alternatively, if shearwaters follow more individualistic rule following processes, we may expect that incubating birds simply wait indefinitely for their foraging partners to return, with apparent coordination emerging as a by-product. We aimed to determine how individuals make decisions relating to the duration of their foraging trips and incubation shifts, whether they factor their partner’s condition into this, and whether this was better explained by active cooperation or individualistic behaviour.
Materials and Methods
Data Collection
This study was carried out on Skomer Island, Wales (51° 44′ N, 5° 17′ W), during the incubation period between April and June of 2018 and 2019. Nests were introduced to the study as eggs were laid, and randomly assigned to the “control” or “handicapped” groups according to lay date, so that each nest in the handicapped group was matched to a control nest with a lay date within 1 day of its own. Adults were captured at the nest through the burrow entrance or purpose-built inspection hatches, and were identified with a permanent stainless steel ring (British Trust for Ornithology). Females were identified at the point of laying through cloacal inspection (Boersma and Davies, 1987), and males by inference. We monitored the attendance of incubating adults at the nest continuously until the egg hatched, allowing us to determine incubation shift duration of each adult, and the corresponding foraging trip duration of the absent parent. Parent shearwaters do not normally overlap at the nest during incubation. Where cold eggs were found in the burrow, we inspected the nest until one of the parents returned (at which point we deemed the egg “neglected”), the egg was depredated, or we reasoned that the burrow was abandoned. A total of 48 nests were used, comprising 23 handicapped (12 in 2018, 11 in 2019) and 25 control (12 in 2018, 13 in 2019) nests. Within experimental nests, we handicapped 10 females and 13 males.
To determine how body condition relates to the decisions made during incubation, we weighed incubating birds daily, from the day the egg was first found to the day it hatched, using a 500 g Pesola spring balance precise to 5 g. To account for the influence that differences in skeletal body size may have on measurements of mass, we calculated a “scaled mass index” as follows (Peig and Green, 2009):
Where Mi = individual body mass, L_i = individual tarsus length, bSMA = scaling component estimated by the regression of M on L, and L_0 = the mean tarsus length value for the entire sample. We measured tarsus length to the nearest 1 mm using digital callipers. Four individuals abandoned the nest before this measurement could be taken, and so condition was unknown for these birds.
Foraging Behaviour
To monitor foraging behaviour during the experiment, we fitted 48 individuals in 31 nests (19 individuals in 12 nests in 2018; 29 individuals in 19 nests in 2019) with geolocators (Migrate Technology Intigeo-C250 or Intigeo-C65), with 30 in the handicapped group and 18 the control group. Geolocators are miniaturised (<2.5 g) archival light loggers, which were programmed to record saltwater immersion data as the transition between wet and dry states and duration spent in either state. These were attached by two small cable ties to a plastic leg ring to ensure immersion when sitting on the sea (see Guilford et al., 2009). Deployment lasted from the first day individuals were captured at the nest, to the day the chick hatched, or, in the case of failure, when the bird was next found on the colony.
We used the immersion data to determine behavioural states. Data were binned into 10 minute epochs, in which immersion values represented the number of seconds the device had been immersed for, and could range between 0 (= no immersion) and 600 (= constant immersion). We applied a threshold model to these data using the criteria set out by Phalan et al. (2007) and Dean et al. (2013); values < 2% of the maximum possible total were labelled as “flight”; > 98% were labelled as “rest” and values in between as “forage.” This method has been validated for the Manx shearwater (Dean et al., 2013; Fayet et al., 2016). Foraging behaviour was summarised as the proportion of each 24 h period (00:00–23:59 UTC) spent in each of the three behavioural states.
Handicapping Protocol
The handicapping procedure followed a three-stage (A-B-C) experimental design (Figure 1). We handicapped adults following the completion of at least one foraging trip by both parents (phase “A”) since laying, and no later than 10 days prior to the expected hatch date. Drawing on handicapping methods outlined by Bijleveld and Mullers (2009), we bound together the two outermost primary feathers (P9 and P10) on both wings using 3 strips of 3 mm diameter marine cloth Tesa 4651 tape, in order to increase wing loading and therefore decrease flight efficiency (Pennycuick, 1989). The handicapped bird was allowed to complete a single foraging trip (phase “B”), and the tape was removed following its return to incubate (phase “C”).
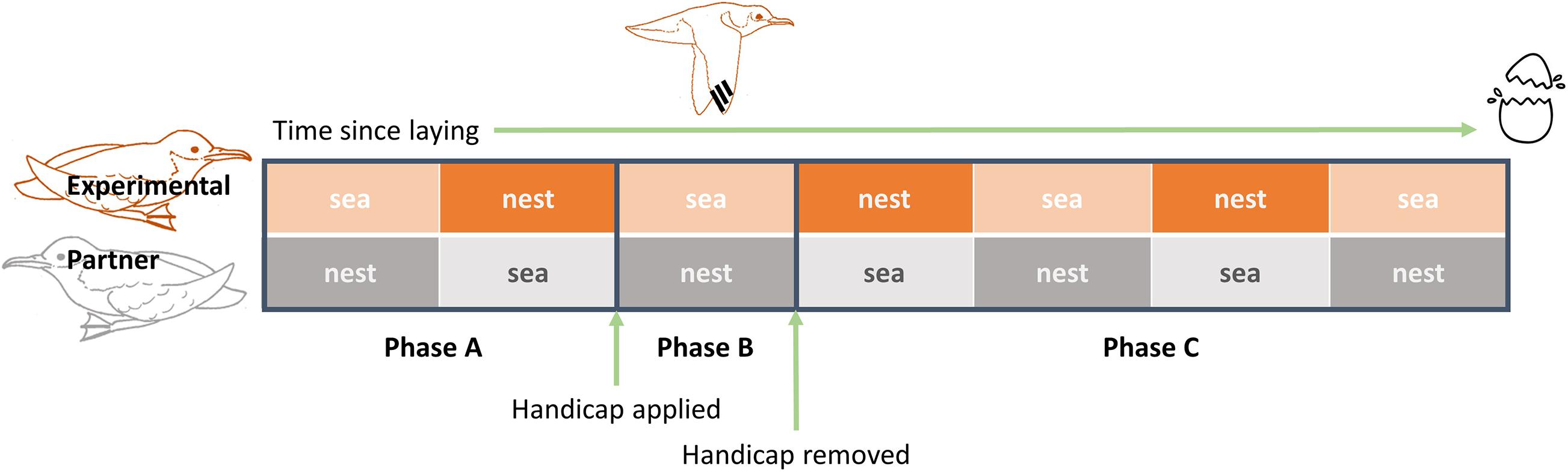
Figure 1. Cartoon schematic of the handicapping protocol. Both parents were observed until they each completed one complete incubation shift and foraging trip (Phase A). Following completion of this phase, the handicap was applied to the incubating experimental bird so it would be handicapped for the duration of its next foraging trip (Phase B). On its return from the trip, the handicap was removed, and the incubation behaviour of the two parents was observed until the egg hatched (Phase C).
Statistical Analysis
All analyses and data processing were carried out in R version 3.5.1 (R Core Team, 2018). To construct linear mixed effects models (LMMs) and generalised mixed effects models (GLMMs), we used the R package lme4 (Bates et al., 2015), and obtained p-values via Sattherthwaite’s degrees of freedom method using the package lmerTest (Kuznetsova et al., 2017). Generalised beta mixed models were constructed using the package glmmTMB (Magnusson et al., 2020). Data are presented as means with 95% confidence intervals unless otherwise specified.
To determine the consequences of the handicapping treatment for the entire incubation period, we examined incidence of neglect, incubation duration, and productivity as a function of nest group (“control” vs. “handicapped”). Permutation (randomisation) tests were used to assess differences in total days of neglect and incubation duration, respectively, between handicapped and control nests. In each case, the observed values for each variable (number of days of neglect or incubation duration) were randomly assigned to the two groups, and the mean difference between the two randomised groups was calculated. This was repeated over 10,000 iterations, and two-tailed p-values were calculated as the proportion of permutations in which the calculated mean difference was greater than the observed difference. As egg failure often results in curtailing of the incubation period, we compared the duration of the entire incubation period between only successful control and handicapped nests. All other analyses included all nests regardless of breeding success. Productivity was measured as the number of chicks hatched per egg laid, and was compared between the two groups using a Pearson’s Chi-squared test.
We hypothesised that handicapping may lead to a change in the proportional contribution of each parent to incubation. To explore this possibility, we first examined whether males and females normally differed in their shares of incubation using a t-test. We then used a linear model to test whether the ratio of female to male care differed between “handicapped” and “control” nests.
To assess the effects of the handicapping treatment on incubation shift duration, shift number, body condition changes, and foraging behaviour, we fitted a set of GLMMs and LMMs according to the structures outlined in Table 1. For the purpose of analysis, we divided individuals into 4 “treatment” groups, according to the stage in the handicapping period. The “none” group comprised all control birds throughout the incubation period, as well as both birds in handicapped nests prior to the handicapping phase (phase “A”). The “handicapped/partner-handicapped” group comprised experimental and partner birds, respectively, in the handicapped nests during the handicapping period itself (phase “B”). The “post-handicap” group comprised experimental birds in the incubation shift or foraging trip immediately following the handicap period (phase “C”). Finally, the “post-partner handicap” group comprises partner birds in the incubation shift or foraging trip immediately following the handicap period (phase “C”).
To control for repeat measures, we included a random intercept of either “ring” or “ring” nested within “nest” in all models as appropriate. To control for potential temporal variation in our behavioural measures, we additionally included the fixed effects of number of days since laying and year. To control for any sex-related variation in behaviour, we included the fixed effect of sex. Finally, as body condition might reasonably be expected to explain variation in incubation shift duration and/or foraging trip duration, we included condition at the start of each shift or trip, respectively, as a fixed effect in models 1, 3, 6, 7, and 8.
If decisions about foraging trip duration are made following a negotiation process, we might expect that the observed trip duration and/or the returning body condition of the foraging bird may relate to the condition of its partner when the foraging bird left the nest. To examine this, we included the fixed effect of partner body condition (g) in models 3 and 4b.
Models 1, 2, and 3, which explored variation in incubation shift duration, incubation shift number, and foraging trip duration, were fitted as GLMMs with a Poisson error structure to account for their count-based response variables. As our measures of foraging behaviour were given as proportions of each 24 h period, we fitted models 6, 7, and 8 as mixed-effects beta regression models to account for the proportion-based error structure.
Ethical Note
All methods were approved by the British Trust for Ornithology Unconventional Methods Technical Panel (permit number C\5311), Natural Resources Wales, Islands Conservation Advisory Committee, and University of Oxford’s Local Ethical Review Process (reference number APA/1/5/ZOO/NASPA). The decision was taken to use a wing-taping protocol for the handicapping procedure due to its temporary and reversible nature. Alternative methods, including wing clipping and weighting, would last longer than the experiment or would handicap the animal indefinitely, or at least until the next moult, should it fail to return to the colony. As per a recommendation by the University’s Animal Welfare and Ethical Review Board (AWERB), we conducted a pilot of the experiment on a set of 4 early laying nests in April and May 2018. Following assessment of the impacts on these nests, which were acceptable within the experimental context, we expanded the experiment. Handling time, both during daily weighing and the deployment and retrieval of geolocators, was normally < 5 min.
Results
All experimental birds except one returned to incubate following the handicapped foraging trip. This remaining bird abandoned its breeding attempt during the handicapping period, but was recovered at the nest later, permitting recovery of the tape and geolocator. Three of the 48 geolocators deployed were not retrieved as the individuals carrying them abandoned the breeding attempt and were not seen again that year. Of the remaining loggers, 2 failed to collect data.
The Entire Incubation Period
More days of neglect were observed in handicapped than control nests (control = 1 day; handicapped = 10 days), and 6/10 days of neglect in the handicapped group were observed at the end of an incubation shift during which the partner was handicapped. However, this difference was not significant (two-tailed p = 0.26; permutations = 10,000). The duration of the entire incubation period did not differ between the two groups [control = 49.71 (48.83, 50.60) days, handicapped = 50.53 (49.67, 51.37); two-tailed p = 0.86; permutations = 10,000]. Productivity (chicks hatched per eggs laid) did not significantly differ between control and handicapped groups (control = 0.58, handicapped = 0.82; df = 1, χ2 = 2.25, p = 0.13; Table 2).
Incubation Behaviour
Males and females did not differ in their incubation shift duration [female: 5.86 (5.22, 6.58) days, male: 6.01 (5.36, 6.73) days; model 1: z = 0.38, p = 0.70], or the number of shifts they took on [females: 4.44 (3.88, 5.08) shifts, males: 4.97 (4.37, 5.65) shifts; model 2: z = 1.19, p = 0.24]. Despite this, the slight differences between males and females appeared to accumulate over the incubation period, with males being found to take on a greater share of incubation overall [males = 0.54; females = 0.46; 95% CI for difference (0.11, 0.039), t = 4.027, p = 0.00012]. The ratio of female to male care did not differ between control and handicapped nests [control = 0.90(0.78, 1.02); handicapped = 0.91 (0.78, 1.03); F(1,45) = 0.0015, p = 0.97].
Incubation shift duration was significantly predicted by the starting condition of incubating birds; the heavier the incubating bird at the start of the incubation shift, the longer the shift, with duration (in days) increasing by 0.0042 (0.0028, 0.0056)% for each gram increase in starting mass (model 1: z = 6.0, p < 0.0001).
Treatment had a significant influence on the duration of the incubation shift (model 1, Figure 2). Partners of handicapped birds sustained incubation shifts which were significantly longer than control birds or prior to the handicapping phase [none: 4.42 (4.14, 4.75) days; partner handicapped: 9.95 (8.51, 11.63) days; z = 9.8, p < 0.0001]. Following their handicapped foraging trips, experimental birds also sustained longer than normal incubation shifts [post-handicap: 6.64 (5.52, 7.98) days; z = 4.2, p < 0.0001]. By their second incubation shift following the handicapping phase, partner birds incubated for as long as birds in the “none” category [post-partner handicap: 4.23 (3.34, 5.36) days; z = −0.4, p = 0.70], suggesting a return to normal shift lengths.
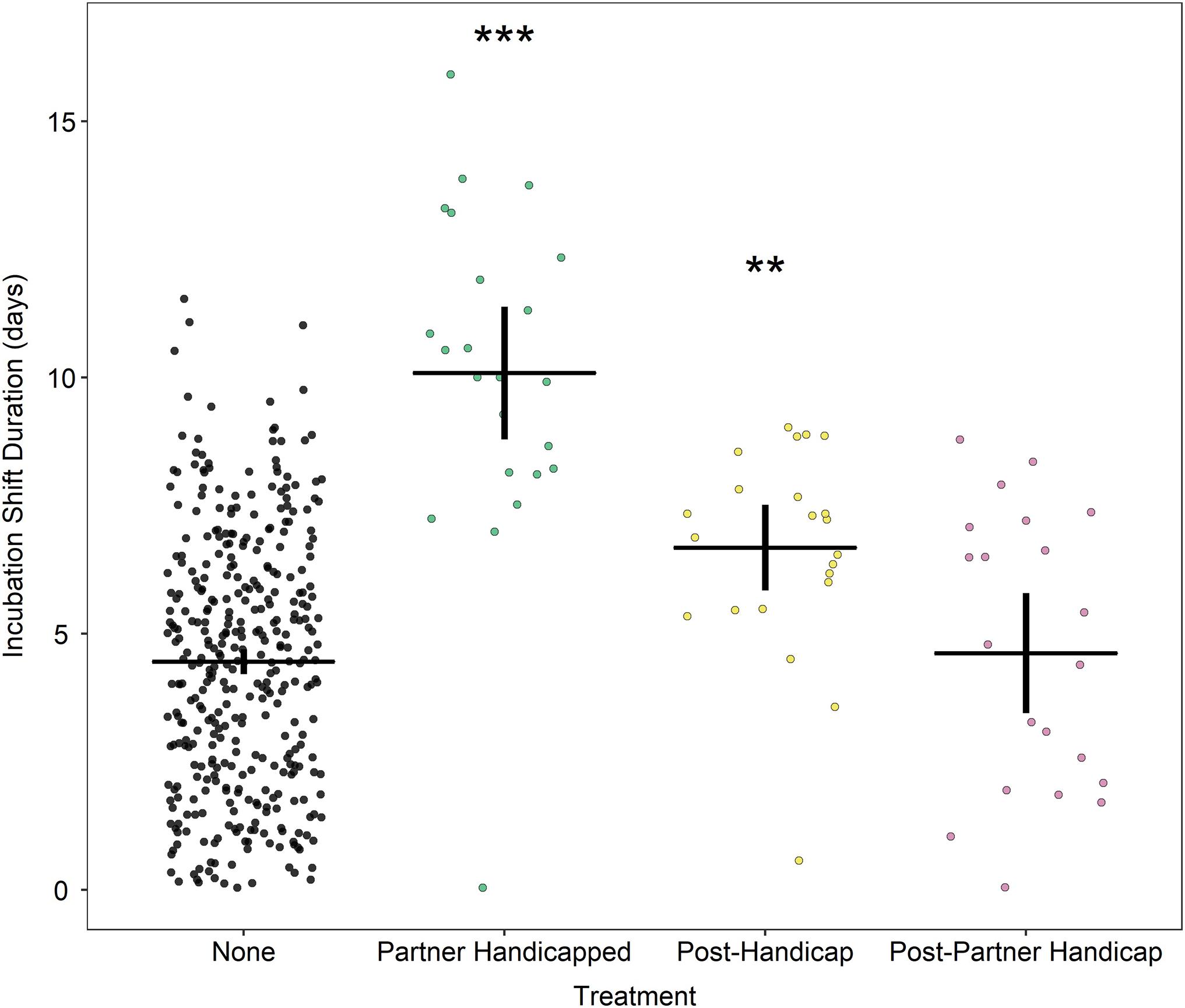
Figure 2. Duration of incubation shift in days according to treatment group. Horizontal line indicates mean; vertical lines indicate 95% confidence intervals Stars indicate significant differences relative controls where ***p < 0.0001; **p < 0.01. Data points are “jittered” in X-axis for readability.
Foraging Behaviour
In line with the observed effects on incubation shift duration, treatment group had a significant effect on duration of the foraging trip (model 3, Figure 3). Handicapped birds took significantly longer foraging trips than birds in the “none” category [none: 5.33 (4.99, 5.69) days, handicapped: 10.60 (9.15, 12.27) days; z = 8.6, p < 0.0001], corresponding to the increased incubation shift of their partners (section “Incubation Behaviour”). Following their extended incubation shift, partner birds went on foraging trips that were not different to normal [post-partner handicap: 6.49 (5.35, 7.87) days; z = 1.9, p = 0.055]. The condition of the partner on departure of the foraging bird from the nest influenced its subsequent foraging trip duration positively. For each gram increase in the partner’s body mass, the trip duration of the foraging bird increased by 0.0020 (−0.0019, 0.0041) days (z = 2.8, p = 0.0046).
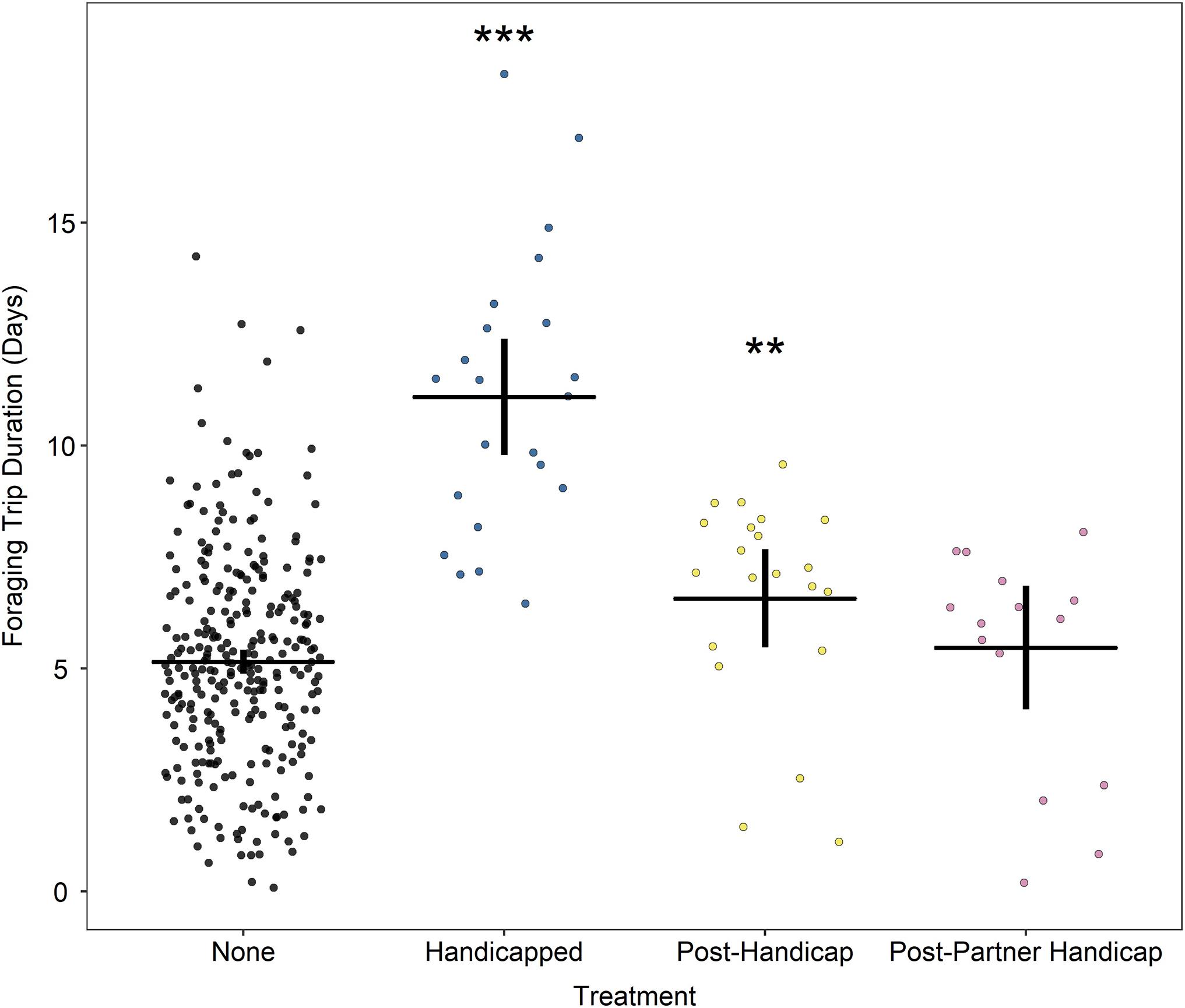
Figure 3. Duration of foraging trip in days according to treatment group. Horizontal line indicates mean; vertical lines indicate 95% confidence intervals. Stars indicate significant differences relative to controls where ***p < 0.0001; **p < 0.01. Data are “jittered” in X-axis for readability.
The proportion of each day of the foraging trip dedicated to foraging behaviour did not vary with treatment [model 6: none: 0.43 (0.41, 0.45), handicapped: 0.39 (0.35, 0.43); z = −1.9, p 0.054; post-partner handicap: 0.43 (0.38, 0.48); z = 0.09, p = 0.93; post-handicap: 0.41 (0.36, 0.46), z = −0.9, p = 0.36]. During the handicapped phase, experimental birds spent more time resting compared to those in the “none” category [model 7: none: 0.38 (0.36, 0.41), handicapped: 0.52 (0.46, 0.57); z = 4.7, p < 0.0001], though returned to normal levels of on their next, non-handicapped trip [post-handicap: 0.33 (0.27, 0.39); z = −1.8, p = 0.069]. On their trips following the extended incubation shift, partners did not differ in the proportion of time they spent resting [post-partner handicap: 0.41 (0.35, 0.48); z = 0.8, p = 0.40]. Experimental birds spent a lesser proportion of their time in flight during the handicapping phase [model 8: none: 0.18 (0.16, 0.20), handicapped: 0.093 (0.071, 0.12); z = −5.1, p < 0.0001], but spent more time flying in their trip following handicapping [post-handicap: 0.25 (0.20, 0.30), z = 3.0, p = 0.0030]. On their foraging trip following the return of the handicapped experimental bird, partners did not differ in the proportion of time they spent in flight [post-partner handicap: 0.15 (0.11, 0.19); z = −1.5, p = 0.14]. Figure 4 illustrates the proportion of time spent in each behavioural state for each treatment group.
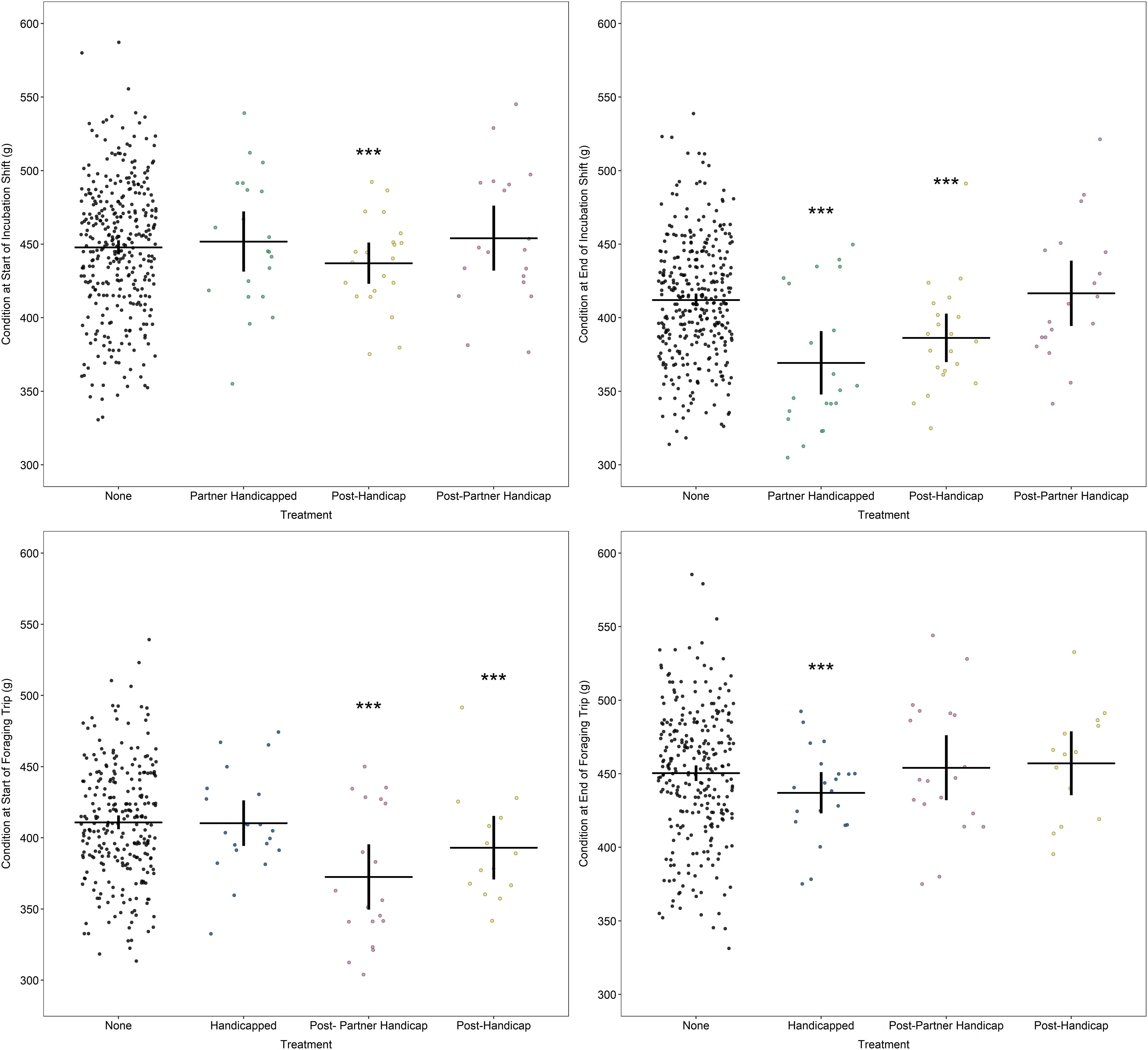
Figure 4. Proportion of each day of foraging trip spent in each of rest, flight, and forage behaviours for each level of the handicapping treatment, where dark red = “None,” dark blue = “Handicapped,” cream = “Post-Partner Handicapped” and light blue = “Post-Handicapped.” Horizontal line indicates mean, vertical lines indicate 95% confidence intervals. Stars indicate significant differences relative to controls where ***p < 0.0001. Data are “jittered” in X-axis for readability.
Body Condition
Experimental birds began their handicapped foraging trips at a similar level of body condition to those birds in the “none” category [model 4a, foraging start condition: none: 411 (402, 419)g, handicapped: 406 (392, 419)g; df = 0.03, t = −0.8, p = 0.41]. However, despite taking considerably longer trips than normal, these birds returned from their handicapped foraging trips in poorer condition [model 4b, foraging end condition: none: 453 (444, 462) g, handicapped: 433 (419, 446) g; df = 0.02, t = −3.7, p = 0.00024]. This was further mediated by the condition of the partner: those birds whose partners were in better condition themselves returned to the nest in better condition, with ending condition increasing by 0.14 g for each gram increase in the partner’s condition (model 4b, foraging end condition: df = 0.02, t = 2.4, p = 0.019).
Experimental birds, which returned from their handicapped trips in poor condition, subsequently began their ensuing incubation shift at a low starting condition [model 5, incubation start condition: none: 450 (441, 458) g, post-handicap: 429 (416, 442) g; df = 0.03, −3.8, p = 0.00017]. As these birds continued to lose weight during the incubation shift, by the start of their next foraging trip following the handicap, experimental birds were in lower condition than normal [model 4a, foraging start condition: post-handicap: 385 (369, 400) g; df = 0.03, t = −3.6, p = 0.00042]. However, their condition by the end of this trip did not differ to control birds, suggesting increased foraging gains [model 4b, foraging end condition: post-handicap: 452 (436, 467) g; df = 0.02, t = −0.2, p = 0.83].
The partners of experimental birds began their incubation shifts during the handicapping phase in similar condition to birds in the “none” category [model 5, incubation start condition: partner handicapped: 450 (437, 464) g; df = 0.03, t = 0.1, p = 0.92]. These birds subsequently took on considerably longer incubation shifts (see section “Incubation Behaviour”) and so lost increased mass during this time. Consequently, partner birds began their first foraging trip following the handicapping phase in worse condition than normal [model 4a, foraging start condition: post-partner handicap: 366 (352, 380) g; df = 0.02, t = −7.5, p < 0.0001]. Partner birds appeared to compensate for this increased mass loss on the ensuing foraging trip, however, as they returned from this trip in normal condition, suggesting increased foraging gains [model 4b, ending condition: post-partner handicap: 452 (438, 467) g; df = 0.02, t = −0.3, p = 0.78]. Figure 5 illustrates the condition values for each phase of the experiment for each of the treatment groups.
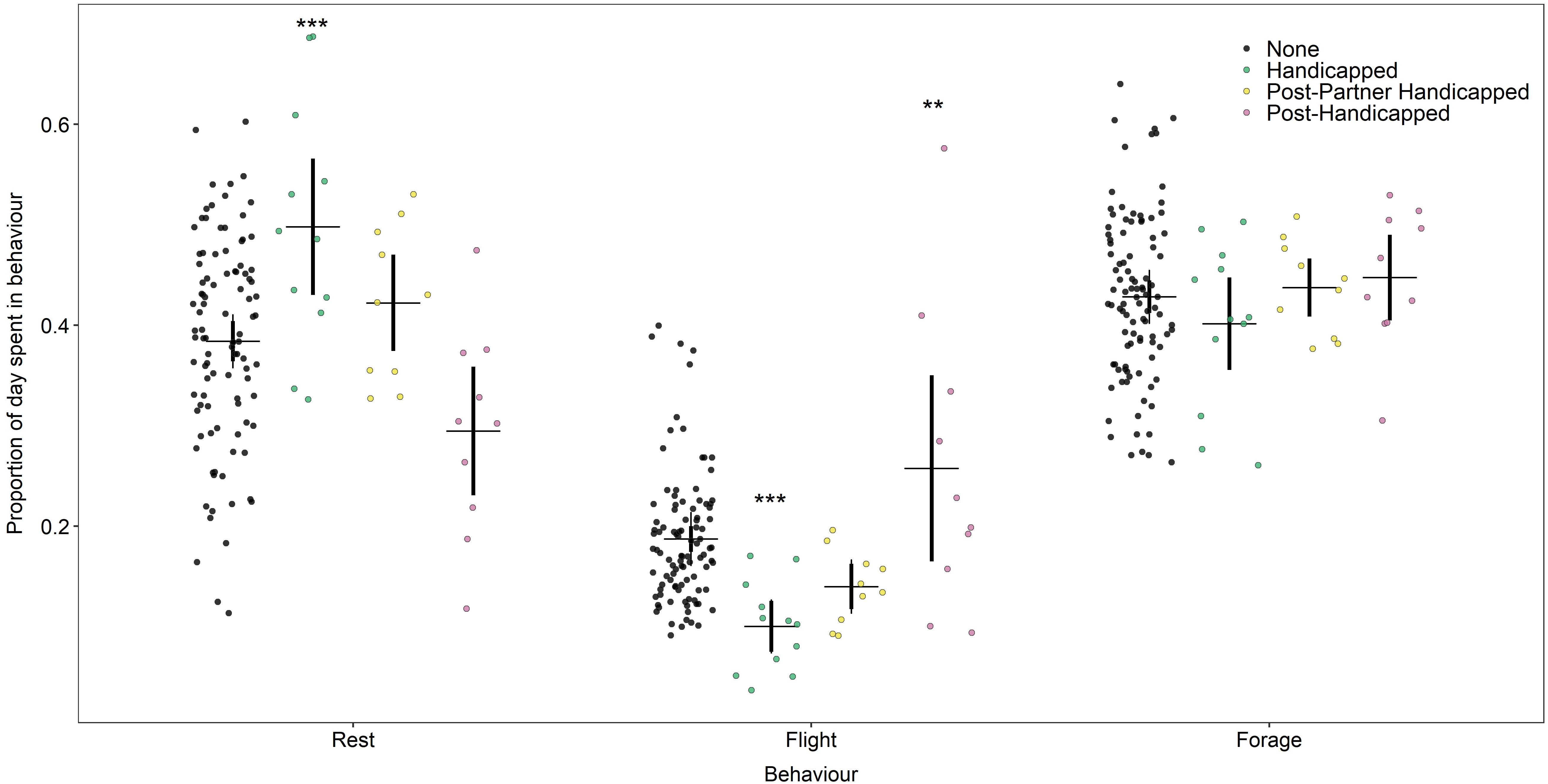
Figure 5. Body condition at the start (left plots) and end (right plots) of incubation (top panels) and foraging (bottom panels) according to treatment. Horizontal line indicates mean; vertical lines indicate 95% confidence intervals. Stars indicate significant differences relative to controls where ***p < 0.0001; **p < 0.01. Data points are “jittered” in X-axis for readability.
Discussion
Manx shearwaters that were handicapped for the duration of one foraging trip spent twice as long at sea as prior to the manipulation. Their partners compensated for this reduced nest attendance by incubating continuously during this time. However, handicapped birds returned to from their trips in poorer condition than normal, suggesting that they were returning to the nest earlier than might be optimal from the perspective of regaining body condition. The extent to which these birds returned early was dependent on the condition of their partners, with those whose partners were in better condition spending correspondingly longer at sea and returning at a higher mass.
As incubating partners lost mass continuously during the protracted incubation shift, these birds ended the shift in worse condition than normal. Consequently, once the handicapped bird returned to the nest, a conflict emerged in the preferred strategies of the two parents: while the partner would benefit from a long foraging trip to recoup its lost condition, the experimental bird may not be able to sustain the corresponding lengthened incubation shift due to its own compromised condition. Foraging partners appeared to win this conflict, with post-handicapping incubation shifts averaging 1.6 days longer than normal, suggesting that incubating birds simply wait for the foraging bird to return to the nest. These results together suggest that while incubating shearwaters simply adopt a “sit-and-wait” strategy for controlling their shift durations, foraging birds make use of condition-dependent information to adopt a trip duration that is optimal for the pair as a whole. Such a mechanism may permit flexibility in the behavioural responses of the two parents, while maximising fitness returns from the breeding attempt.
The observation that the incubating bird continues to wait at the nest, even when their partners are away for considerably abnormal durations of time, suggests that these decisions are unlikely to be pre-determined through a negotiation process. Instead, regulation of incubation shifts in Manx shearwaters appears to be mediated primarily by the decisions made by the foraging bird, as has been observed in short-tailed shearwaters (Ardenna tenuirostris, Carey, 2011) and great frigatebirds (Dearborn, 2001). That the condition of the foraging bird takes priority in incubation scheduling may be due to the inability or unwillingness of Manx shearwaters to adjust their foraging behaviour dynamically. While the foraging gains of experimental birds were clearly reduced, there was no associated change in effort. This contrasts with previous studies, where handicapped birds were found either to increase (Navarro and González-Solís, 2007) or decrease (Tajima and Nakamura, 2003; Jacobs et al., 2013; Serota and Williams, 2019) foraging effort. As experimental birds were observed to reduce flight time and increase time spent resting, this is unlikely to reflect a failure to identify behavioural changes. Observed foraging effort was comparable to previous studies (Dean et al., 2013), and may indicate that breeding shearwaters are already foraging at their maximum capacity and cannot upregulate this to compensate for a decrease in efficiency. Consequently, only by extending the trip duration can shearwaters increase their foraging gains. Indeed, the increased trip durations observed for partner birds post-handicapping were sufficient to recoup their condition to normal levels, despite maintaining normal foraging effort. If Manx shearwaters are constrained to increase foraging gains by increasing trip duration, this provides a rationale for the foraging bird to maintain control of trip length, further supporting the notion that incubation scheduling is mediated by the need for the foraging bird to regain lost mass, as is expected in long-lived species, which are selected to prioritise their own condition (Stearns, 1992). The finding that handicapped nests exhibited similar levels of equity in the proportional contributions of each parent to care suggests that this is an effective mechanism to maintain coordination and equality in investment.
We can envisage two scenarios under which this model of decision-making may operate. In the first, foraging birds feed until their mass reaches some set threshold, at which point they return to the nest, regardless of the condition of the partner. Under this strategy, we expect that both successful (gain mass quickly) and unsuccessful (gain mass slowly) foragers would return to the nest at the same mass, but the former group would spend fewer days at sea (Figure 6A). The infrequency of neglect in this study, combined with the extremely long incubation shifts exhibited by partners, suggests incubating shearwaters do not normally reach a physiological limit beyond which they have insufficient energy reserves to maintain incubation. Maintaining a “buffer” of condition may ensure incubating birds can sustain an unexpectedly long shift if adverse environmental conditions retain the partner at sea for longer than usual. Consequently, a “sit and wait” strategy could be effective for incubating birds.
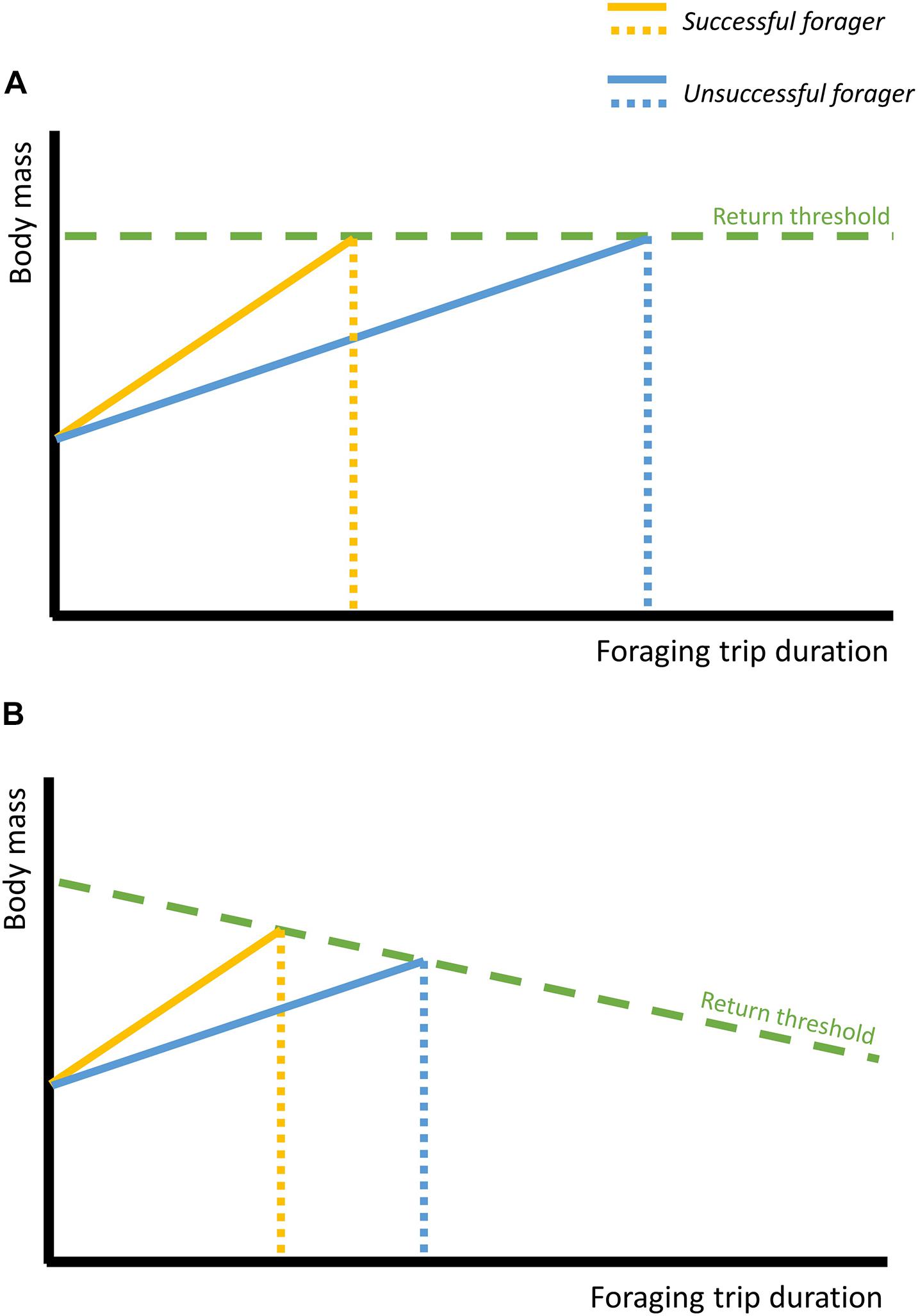
Figure 6. Illustrative model to demonstrate the outcome of a return mass threshold-based decision paradigm for foraging behaviour. Successful foragers, which gain mass quickly, are coloured in yellow; unsuccessful foragers, which gain mass slowly, are coloured in blue. (A) Fixed return threshold. Successful foragers reach their return threshold mass rapidly and so return to the nest after a short trip. Unsuccessful foragers reach their return threshold mass more slowly, and so spend more time away from the nest. Both birds return at the same mass. (B) Declining return threshold. Successful foragers reach their return threshold mass rapidly and so return to the nest after a short trip and at a relatively high mass. Unsuccessful foragers reach their return threshold mass more slowly, and so take a longer trip and return at a relatively lower mass.
However, clearly parents will be unable to sustain incubation indefinitely, and an extremely protracted foraging trip by the partner will force the incubating bird to abandon the nest, either due to an extreme decline in its body condition, or because of the perceived low likelihood of its partner returning to the nest. Though no relationship could be found between incubation shift duration and the probability of neglect in this study, it is possible that this was due to its low frequency. In other seabirds, neglect occurs when the body condition of the incubating bird falls below some threshold (e.g., herring gull Larus argentatus, Sibly and McCleery, 1985; blue petrel, Chaurand and Weimerskirch, 1994; Ancel et al., 1998). A similar relationship may exist in Manx shearwaters. While Manx eggs are resistant to chilling, just 3 days of exposure reduces survival to 28% (Harris, 1966; Brooke, 1990). As the breeding success of the parents is intertwined, both parents should thus attempt to minimise the likelihood of neglect. We can therefore envisage a second, more cooperative decision-making strategy for foraging birds, in which the returning mass threshold may decrease as the duration of the foraging trip increases (Figure 6B). Such a strategy would allow birds to prioritise and act on their own condition when deciding on trip duration, while implicitly taking into account their partner’s needs. That experimental birds returned from their long-duration trips in worse condition lends support to this second model, and is a common finding in seabird handicapping studies, where despite reducing parental care, handicapped individuals still suffer some reduction in condition (Navarro and González-Solís, 2007; Bijleveld and Mullers, 2009; Harding et al., 2009; Jacobs et al., 2013), suggesting this may be widespread.
The slope of this declining return mass threshold could be set by evolutionary or behavioural processes. In the former case, the rate of decline may evolve to match the average change in probability of neglect for incubating shearwaters across the population. There is clearly considerable flexibility and resilience in the time the incubating bird can sustain on the nest, and so this may be an effective means to minimise the probability of neglect from the perspective of the foraging bird. Alternatively, the slope of the decline may be set by information transfer between the parents. On reunion at the nest, Manx shearwater pairs engage in conspicuous vocalisations (Brooke, 1990), which may serve as an opportunity to exchange information about body condition (Jones et al., 2002). Such information could allow the foraging bird to set the gradient of its return threshold slope according to the condition of the incubating partner, ensuring that that foraging trip duration is optimised to the needs of the pair as whole. Our results lend greater support to this more flexible model, with the finding that both foraging trip duration and return mass were mediated by the condition of the partner: birds whose partners were in better condition spent longer at sea, and returned to the nest at a higher mass.
Our interpretation of the foraging gain reduction observed here is that it reflects a combination of the compromised foraging efficiency of handicapped individuals and their decision to return to the nest early to minimise the probability of desertion by the partner. However, an alternative explanation is that this reduction in body mass is adaptive: as reduced body mass reduces wing loading, this could in theory compensate for the increased energetic expenditure associated with the handicap (Blem, 1976). Adaptive mass loss has been observed in other species, and may be associated with unfavourable foraging conditions (burrowing parrot Cyanoliseus patagonus, Masello and Quillfeldt, 2003), or, more commonly, the switch in breeding stage from incubation to the more energetically intensive chick provisioning period (thick-billed murres Uria lomvia, Croll et al., 1991; collared flycatcher Ficedula albicollis, Cichoń, 2001, Wilson’s storm petrel Oceanites oceanicus Quillfeldt et al., 2006). However, our mass changes were observed over a much more restricted time period and exclusively during incubation. Existing evidence suggests that higher masses during incubation are adaptive by providing fasting endurance (Chastel et al., 1995; Cuthill and Houston, 1997). Furthermore, when examining mass changes during incubation, adults tend to gain mass when they are released from energetic constraints, for example when environmental conditions are favourable, further suggesting that high masses are adaptive (Holt et al., 2002; Quillfeldt et al., 2006). Finally, the handicapped birds in our study returned to the nest at a much lower condition than the observed range of control birds, suggesting that their reduction was beyond the natural adaptive range of masses. Given this evidence, we believe that our observed mass changes are more likely to reflect the energetic constraints imposed by the handicap, than an adaptive strategy by the handicapped bird.
The duration of incubation shifts, and their corresponding foraging trips, in the Manx shearwater appear to be primarily decided by the foraging bird. The partners of shearwaters that were handicapped for the duration of one foraging trip sustained incubation shifts that were more than double the duration of normal shifts, suggesting they simply wait for the partner to return the nest. However, the decisions made by foraging birds appear to be mediated by the state of their partner, with handicapped birds returning to the nest earlier and at a lower mass when their partners were in worse condition. This provides evidence that incubation scheduling in this species is maintained by a cooperative strategy whereby shearwaters exchange information about their metabolic needs and condition in order to optimise their foraging trip durations in a way that benefits the pair as a whole. These results suggest that simple decision-making mechanisms with some condition-dependent information are sufficient to maintain cooperation between parents without the need for pre-determined negotiation of behaviour.
Data Availability Statement
The raw data supporting the conclusions of this article will made available by the authors, upon request without undue reservation.
Ethics Statement
The animal study was reviewed and approved by the University of Oxford Animal Welfare and Ethical Review Board.
Author Contributions
NG and TG conceived and designed the experiment. NG, MS, JW, and CV carried out the fieldwork. NG conducted the analyses with support from JW. NG wrote the final manuscript with contributions and feedback from all other authors. All authors contributed to the article and approved the submitted version.
Funding
This work was supported by the Biotechnology and Biological Sciences Research Council (BBSRC) grant (BB/M011224/1) to NG.
Conflict of Interest
The authors declare that the research was conducted in the absence of any commercial or financial relationships that could be construed as a potential conflict of interest.
Acknowledgments
We thank the Wildlife Trust of South and West Wales and Island Conservation Advisory Committee for permitting this work to take place on Skomer Island, and the wardens for logistical support while on the island. We also thank Daryl McLeod, Bryony Baker, and Lucinda Zawadzki for fieldwork support on Skomer, Chris Tyson for helpful feedback on an earlier version of the manuscript, Ollie Padget for statistical advice, and OxNav members for useful discussion.
References
Ahern, T. H., Hammock, E. A. D., and Young, L. J. (2011). Parental division of labor, coordination, and the effects of family structure on parenting in monogamous prairie voles (Microtus ochrogaster). Dev. Psychobiol. 53, 118–131. doi: 10.1002/dev.20498
Ancel, A., Petter, L., and Groscolas, R. (1998). Changes in egg and body temperature indicate triggering of egg desertion at a body mass threshold in fasting incubating blue petrels (Halobaena caerulea). J. Comp. Physiol. B Biochem. Syst. Environ. Physiol. 168, 533–539. doi: 10.1007/s003600050174
Bates, D., Mächler, M., Bolker, B. M., and Walker, S. C. (2015). Fitting linear mixed-effects models using lme4. J. Stat. Softw. 1406, 1–48.
Bijleveld, A. I., and Mullers, R. H. E. (2009). Reproductive effort in biparental care: an experimental study in long-lived Cape gannets. Behav. Ecol. 20, 736–744. doi: 10.1093/beheco/arp054
Blem, C. R. (1976). Patterns of lipid storage and utilization in birds. Am. Zool. 16, 671–684. doi: 10.1093/icb/16.4.671
Boersma, P., and Davies, E. (1987). Sexing monomorphic birds by vent measurements. Auk 104, 779–783. doi: 10.1093/auk/104.4.779
Bolton, M. (1995). Food delivery to nestling storm petrels: limitation or regulation? Funct. Ecol. 9, 161–170. doi: 10.2307/2390560
Boucaud, I. C. A., Aguirre Smith, M. L. N., Valère, P. A., and Vignal, C. (2016). Incubating females signal their needs during intrapair vocal communication at the nest: a feeding experiment in great tits. Anim. Behav. 122, 77–86. doi: 10.1016/j.anbehav.2016.09.021
Boucaud, I. C. A., Perez, E. C., Ramos, L. S., Griffith, S. C., and Vignal, C. C. (2017). Acoustic communication in zebra finches signals when mates will take turns with parental duties. Behav. Ecol. 28, 645–656. doi: 10.1093/beheco/arw189
Brooke, M. D. L. (1978). Some factors affecting the laying date, incubation and breeding success of the Manx shearwater, Puffinus puffinus. J. Anim. Ecol. 47, 477–495. doi: 10.2307/3795
Carey, M. J. (2011). Incubation routine, duration of foraging trips and regulation of body mass in Short-tailed Shearwaters (Ardenna tenuirostris). Emu 111, 166–171. doi: 10.1071/mu10043
Chastel, O., Weimerskirch, H., and Jouventin, P. (1995). Body condition and seabird reproductive performance: a study of three petrel species. Ecology 76, 2240–2246. doi: 10.2307/1941698
Chaurand, T., and Weimerskirch, H. (1994). Incubation routine, body mass regulation and egg neglect in the Blue Petrel Halobaena caerulea. Ibis 136, 285–290. doi: 10.1111/j.1474-919x.1994.tb01097.x
Cichoń, M. (2001). Body-mass changes in female collared flycatchers: state-dependent strategy. Auk 118, 550–552. doi: 10.1093/auk/118.2.550
Congdon, B., Krockenberger, A., and Smithers, B. (2005). Dual-foraging and co-ordinated provisioning in a tropical Procellariiform, the wedge-tailed shearwater. Mar. Ecol. Prog. Ser. 301, 293–301. doi: 10.3354/meps301293
Croll, D. A., Gaston, A. J., and Noble, D. G. (1991). Adaptive loss of mass in thick-billed murres. Condor 93, 496–502. doi: 10.2307/1368181
Cuthill, I. C., and Houston, A. I. (1997). “Managing time and energy,” in Behavioural Ecology: An Evolutionary Approach, eds. J. Krebs and N. B. Davies (Cambridge: Blackwell Scientific), 97–120.
Davis, L. S. (1988). Coordination of incubation routines and mate choice in Adelie Penguins (Pygoscelis adeliae). Auk 105, 428–432. doi: 10.1093/auk/105.3.428
Dean, B., Freeman, R., Kirk, H., Leonard, K., Phillips, R. A., Perrins, C. M., et al. (2013). Behavioural mapping of a pelagic seabird: combining multiple sensors and a hidden Markov model reveals the distribution of at-sea behaviour. J. R. Soc. 10:20120570. doi: 10.1098/rsif.2012.0570
Dean, B., Kirk, H., Fayet, A., Shoji, A., Freeman, R., Leonard, K., et al. (2015). Simultaneous multi-colony tracking of a pelagic seabird reveals cross-colony utilization of a shared foraging area. Mar. Ecol. Prog. Ser. 538, 239–248. doi: 10.3354/meps11443
Dearborn, D. C. (2001). Body condition and retaliation in the parental effort decisions of incubating great frigatebirds (Fregata minor). Behav. Ecol. 12, 200–206. doi: 10.1093/beheco/12.2.200
Fayet, A. L., Freeman, R., Shoji, A., Kirk, H. L., Padget, O., Perrins, C. M., and Guilford, T. (2016). Carry-over effects on the annual cycle of a migratory seabird: an experimental study. J. Animal Ecol. 85, 1516–1527. doi: 10.1111/1365-2656.12580
Foster, W. A., and Treherne, J. E. (1981). Evidence for the dilution effect in the selfish herd from fish predation on a marine insect. Nature 293, 466–467. doi: 10.1038/293466a0
Guilford, T., Meade, J., Willis, J., Phillips, R. A., Boyle, D., Roberts, S., et al. (2009). Migration and stopover in a small pelagic seabird, the Manx shearwater Puffinus puffinus: insights from machine learning. Proc. Biol. Sci. 276, 1215–1223. doi: 10.1098/rspb.2008.1577
Guilford, T. C., Meade, J., Freeman, R., Biro, D., Evans, T., Bonadonna, F., et al. (2008). GPS tracking of the foraging movements of Manx Shearwaters Puffinus puffinus breeding on Skomer Island, Wales. Ibis 150, 462–473. doi: 10.1111/j.1474-919x.2008.00805.x
Hamer, K. C., Lynnes, A. S., and Hill, J. K. (1998). Regulation of chick provisioning rate in Manx Shearwaters: experimental evidence and implications for nestling obesity. Funct. Ecol. 12, 625–630. doi: 10.1046/j.1365-2435.1998.00223.x
Harding, A. M. A., Kitaysky, A. S., Hamer, K. C., Hall, M. E., Welcker, J., Talbot, S. L., et al. (2009). Impacts of experimentally increased foraging effort on the family: offspring sex matters. Anim. Behav. 78, 321–328. doi: 10.1016/j.anbehav.2009.05.009
Harris, M. P. (1966). Breeding biology of the manx shearwater Puffinus puffinus. Ibis 108, 17–33. doi: 10.1111/j.1474-919x.1966.tb07249.x
Holt, S., Whitfield, D. P., Duncan, K., Rae, S., and Smith, R. D. (2002). Mass loss in incubating Eurasian dotterel: adaptation or constraint? J. Avian Biol. 33, 219–224. doi: 10.1034/j.1600-048X.2002.330303.x
Itzkowitz, M., Santangelo, N., and Richter, M. (2002). How similar is the coordination of parental roles among different pairs? An examination of a monogamous fish. Ethology 108, 727–738. doi: 10.1046/j.1439-0310.2002.00805.x
Jacobs, S. R., Elliott, K. H., and Gaston, A. J. (2013). Parents are a drag: long-lived birds share the cost of increased foraging effort with their offspring, but males pass on more of the costs than females. PLoS One 8:e54594. doi: 10.1371/journal.pone.0054594
Jones, K. M., Ruxton, G., and Monaghan, P. (2002). Model parents: is full compensation for reduced partner nest attendance compatible with stable biparental care? Behav. Ecol. 13, 838–843. doi: 10.1093/beheco/13.6.838
Kuznetsova, A., Brockhoff, P. B., and Christensen, R. H. B. (2017). lmerTest package: tests in linear mixed effects models. J. Stat. Softw. 82:26. doi: 10.18637/jss.v082.i13
Magnusson, A., Skaug, H. J., Nielsen, A., Berg, C. W., Kristensen, K., Maechler, M., et al. (2020). Package “glmmTMB”. Generalized Linear Mixed Models Using Template Model Builder. Cran R Proj.
Mariette, M. M., and Griffith, S. C. (2015). The adaptive significance of provisioning and foraging coordination between breeding partners. Am. Nat. 185, 270–280. doi: 10.1086/679441
Masello, J. F., and Quillfeldt, P. (2003). Body size, body condition and ornamental feathers of Burrowing Parrots: variation between years and sexes, assortative mating and influences on breeding success. Austral Ornithol. 103:2, 149–161. doi: 10.1071/MU02036
Massoni, V., Reboreda, J. C., Lopez, G. C., Aldatz, M. F., López, G. C., Aldatz, M. F., et al. (2012). High coordination and equitable parental effort in the rufous hornero. Condor 114, 564–570. doi: 10.1525/cond.2012.110135
Matthews, G. V. T. T. (1954). Some aspects of incubation in the Manx shearwater Procellaria Puffinus, with particular reference to chilling resistance in the embryo. Ibis 96, 432–440. doi: 10.1111/j.1474-919x.1954.tb02334.x
Navarro, J., and González-Solís, J. (2007). Experimental increase of flying costs in a pelagic seabird: effects on foraging strategies, nutritional state and chick condition. Oecologia 151, 150–160. doi: 10.1007/s00442-006-0559-0
Paredes, R., Jones, I. L., and Boness, D. J. (2005). Reduced parental care, compensatory behaviour and reproductive costs of thick-billed murres equipped with data loggers. Anim. Behav. 69, 197–208. doi: 10.1016/j.anbehav.2003.12.029
Peig, J., and Green, A. J. (2009). New perspectives for estimating body condition from mass/length data: the scaled mass index as an alternative method. Oikos 118, 1883–1891. doi: 10.1111/j.1600-0706.2009.17643.x
Pennycuick, C. J., (1989). Bird Flight Performance?: A Practical Calculation Manual. Oxford: Oxford University Press.
Phalan, B., Phillips, R. A., Silk, J. R. D., Afanasyev, V., Fukuda, A., Fox, J., et al. (2007). Foraging behaviour of four albatross species by night and day. Mar. Ecol. Prog. Ser. 340, 271–286. doi: 10.3354/meps340271
Quillfeldt, P., Masello, J. F., and Lubjuhn, T. (2006). Variation in the adult body mass of Wilson’s storm petrels Oceanites oceanicus during breeding. Polar Biol. 29, 372–378. doi: 10.1007/s00300-005-0066-5
Raihani, N. J., Nelson-Flower, M. J., Moyes, K., Browning, L. E., and Ridley, A. R. (2010). Synchronous provisioning increases brood survival in cooperatively breeding pied babblers. J. Anim. Ecol. 79, 44–52. doi: 10.1111/j.1365-2656.2009.01606.x
Ratz, T., Nichol, T. W., and Smiseth, P. T. (2019). Parental responses to increasing levels of handicapping in a burying beetle. Behav. Ecol. 31, 73–80.
Sanz, J. J., Kranenbarg, S., and Tinbergen, J. M. (2000). Differential response by males and females to manipulation of partner contribution in the great tit (Parus major). J. Anim. Ecol. 69, 74–84. doi: 10.1046/j.1365-2656.2000.00373.x
Saraux, C., Le Bohec, C., Durant, J. M., Viblanc, V. A., Gauthier-Clerc, M., Beaune, D., et al. (2011). Reliability of flipper-banded penguins as indicators of climate change. Nature 469, 203–208. doi: 10.1038/nature09630
Savage, J. L., Browning, L. E., Manica, A., Russell, A. F., and Johnstone, R. A. (2017). Turn-taking in cooperative offspring care: by-product of individual provisioning behavior or active response rule? Behav. Ecol. Sociobiol. 71:162.
Serota, M. W., and Williams, T. D. (2019). Adjustment of total activity as a response to handicapping European starlings during parental care. Anim. Behav. 148, 19–27. doi: 10.1016/j.anbehav.2018.11.009
Shoji, A., Elliott, K. H., Aris-Brosou, S., Crump, D., and Gaston, A. J. (2011). Incubation patterns in a central-place forager affect lifetime reproductive success: scaling of patterns from a foraging bout to a lifetime. PLoS One 6:e17760. doi: 10.1371/journal.pone.0017760
Sibly, R., and McCleery, R. (1985). Optimal decision rules for herring gulls. Anim. Behav. 33, 449–465. doi: 10.1016/s0003-3472(85)80069-5
Tajima, K., and Nakamura, M. (2003). Response to manipulation of partner contribution: a handicapping experiment in the Barn Swallow. Ornithol. Sci. 2, 65–72. doi: 10.2326/osj.2.65
Takahashi, L. S., Storey, A. E., Wilhelm, S. I., and Walsh, C. J. (2017). Turn-taking ceremonies in a colonial seabird: does behavioral variation signal individual condition? Auk 134, 530–541. doi: 10.1642/auk-17-26.1
Tyson, C., Kirk, H., Fayet, A., Van Loon, E. E., Shoji, A., Dean, B., et al. (2017). Coordinated provisioning in a dual-foraging pelagic seabird. Anim. Behav. 132, 73–79. doi: 10.1016/j.anbehav.2017.07.022
Welcker, J., Harding, A. M. A., Karnovsky, N. J., Steen, H., Strøm, H., and Gabrielsen, G. W. (2009). Flexibility in the bimodal foraging strategy of a high Arctic alcid, the little auk Alle alle. J. Avian Biol. 40, 388–399. doi: 10.1111/j.1600-048x.2008.04620.x
Keywords: behavioural coordination, parental care, parental investment, sexual conflict, Manx shearwater
Citation: Gillies N, Syposz M, Wynn J, Vansteenberghe C and Guilford T (2021) Responses of Manx Shearwaters to Handicapping and Its Implications for the Coordination of Care. Front. Ecol. Evol. 9:655923. doi: 10.3389/fevo.2021.655923
Received: 19 January 2021; Accepted: 13 April 2021;
Published: 07 May 2021.
Edited by:
Ann Valerie Hedrick, University of California, Davis, United StatesReviewed by:
Yutaka Watanuki, Hokkaido University, JapanMasaki Shirai, Central Research Institute of Electric Power Industry (CRIEPI), Japan
Copyright © 2021 Gillies, Syposz, Wynn, Vansteenberghe and Guilford. This is an open-access article distributed under the terms of the Creative Commons Attribution License (CC BY). The use, distribution or reproduction in other forums is permitted, provided the original author(s) and the copyright owner(s) are credited and that the original publication in this journal is cited, in accordance with accepted academic practice. No use, distribution or reproduction is permitted which does not comply with these terms.
*Correspondence: Natasha Gillies, Z2lsbGllc25lQGdtYWlsLmNvbQ==