- Department of Biology, Carleton University, Ottawa, ON, Canada
Insect defense sounds have been reported for centuries. Yet, aside from the well-studied anti-bat sounds of tiger moths, little is understood about the occurrence, function, and evolution of these sounds. We define a defense sound as an acoustic signal (air- or solid-borne vibration) produced in response to attack or threat of attack by a predator or parasitoid and that promotes survival. Defense sounds have been described in 12 insect orders, across different developmental stages, and between sexes. The mechanisms of defensive sound production include stridulation, percussion, tymbalation, tremulation, and forced air. Signal characteristics vary between species, and we discuss how morphology, the intended receiver, and specific functions of the sounds could explain this variation. Sounds can be directed at predators or non-predators, and proposed functions include startle, aposematism, jamming, and alarm, although experimental evidence for these hypotheses remains scant for many insects. The evolutionary origins of defense sounds in insects have not been rigorously investigated using phylogenetic methodology, but in most cases it is hypothesized that they evolved from incidental sounds associated with non-signaling behaviors such as flight or ventilatory movements. Compared to our understanding of visual defenses in insects, sonic defenses are poorly understood. We recommend that future investigations focus on testing hypotheses explaining the functions and evolution of these survival sounds using predator-prey experiments and comparative phylogenetics.
Introduction
When threatened or attacked by a predator, many insects produce sounds. Familiar examples are hisses of the Death’s-head hawkmoth, Acherontia atropos (Sphingidae) (Brehm et al., 2015), and the Madagascar hissing cockroach, Gromphadorhina portentosa (Blaberidae) (Nelson, 1979), as well as the tymbal clicks of tiger moths (Erebidae: Arctiinae) (e.g., Corcoran et al., 2010). However, insect defense sounds are widespread and diverse, including those produced by small stridulating bark beetles (Lewis and Cane, 1990) and large whistling caterpillars (Bura et al., 2011). Despite centuries of research reporting that insects use sounds for defense (e.g., Lesser, 1738; Sanborn, 1869; Darwin, 1889), we know very little about the roles these sounds play in insect survival (Conner, 2014). How do sounds stop an attack by a predator? Do different sound characteristics serve different functions? Why do only some insects produce defense sounds? To address these questions, we need a review of insect defensive sound production that highlights research to date and identifies what remains to be investigated. Previous reviews of insect defense sounds focused on potential functions (e.g., Conner, 2014), a single mechanism (e.g., stridulation, Masters, 1980), or a specific taxon (e.g., termite alarm signals, Hager et al., 2019; tiger moth anti-bat clicks, Waters, 2003; Conner and Corcoran, 2012). Our review takes a broader approach by exploring the diversity, function, and evolution of defense sounds across the Class Insecta. Specifically, we document which taxa, sexes, and developmental stages are reported to produce defense sounds, the mechanisms by which they produce them, and the diversity of their signal characteristics. We review hypotheses explaining the functions and evolutionary origins of defensive sound production, as well as the adaptive significance of signal variation. While we focus specifically on defense sounds, we recognize that some insects use other forms of acoustic anti-predator strategies such as adaptive silence and acoustic crypsis, and we refer readers to Conner (2014) for further discussion of these strategies.
What Is a Defense Sound?
What do we mean by “defense sound”? Variously called “distress signals” (Ossiannilsson, 1949), “protest sounds” (Alexander, 1956), “disturbance sounds” (Masters, 1979), and “defense sounds” (Bura et al., 2016), the general consensus is that these acoustic signals are produced in response to disturbance and used to repel predators or warn conspecifics (cf. Alexander, 1967). In social insects such as termites and bees, the sounds protect not only the individual, but the colony. We exclude from this review sounds associated with territorial and agonistic behaviors (e.g., those occurring in male-male interactions) as such sounds are generally aimed at competitors rather than predators. We also exclude cases of “incidental” sounds, meaning sounds associated with another defense like the chemical ejections of bombardier beetles (Eisner et al., 2001), unless the sounds are noted by the authors as being part of the defensive display. We recommend the following definition: “A defense sound is an acoustic signal produced in response to attack or threat of attack by a predator (or parasitoid) and that promotes survival.” Here we refer to an acoustic signal in the broadest sense, meaning a vibration that is airborne, waterborne (both commonly referred to as sounds), or solid-borne (commonly referred to as vibrations). For simplicity, hereafter we refer to all of these acoustic signals as “sounds” [see Hill (2014) and Yack (2016) for further discussion of acoustic signal nomenclature].
Which Insects Produce Defense Sounds?
Understanding which individuals possess a trait and how it varies is important for testing hypotheses on the function and evolution of that trait. Until now, a review of the distribution of insect defense sounds across taxa, sexes, and developmental stages had not been conducted. Using our definition of defense sounds, we reviewed the literature to identify which insect orders and families include species reported or proposed to produce sonic defenses. Among these examples, we also noted the sex and life stages of the species investigated. Our goal was not to identify every reported occurrence, but to obtain a general overview of the variation between taxa, sexes, and developmental stages to identify gaps in the literature and trends that will inform future studies.
Defensive sound production has been reported in at least 12 of the 28 currently recognized insect orders (Table 1 and Figure 1). However, the number of reports varies between orders, ranging from multiple species within 15 families of Lepidoptera to a single species in Odonata. Coleoptera and Hemiptera have the next most abundant number of reports at 12 and 10 families, respectively. Defense sounds are also commonly described in Blattodea (8 families) and Orthoptera (7 families). The remaining orders have reports from 6 or fewer families, though some of these families contain numerous well-known sound producers [e.g., buzzing in Apidae (Hymenoptera), Kirchner and Röschard, 1999]. Importantly, we cannot conclude based on a single sound-producing species that all members of a given family, or even a given genus, produce defense sounds. For example, not all Phasmatidae (Phasmatodea) stridulate when disturbed (Bedford, 1978), and in Sphingidae (Lepidoptera), both sound- and non-sound-producing larvae occur in the genus Manduca (Bura et al., 2016). While defensive sound production appears to be widespread in insects, it is certainly not an even distribution. We discuss why defense sounds might not occur in all species in the next subsection.
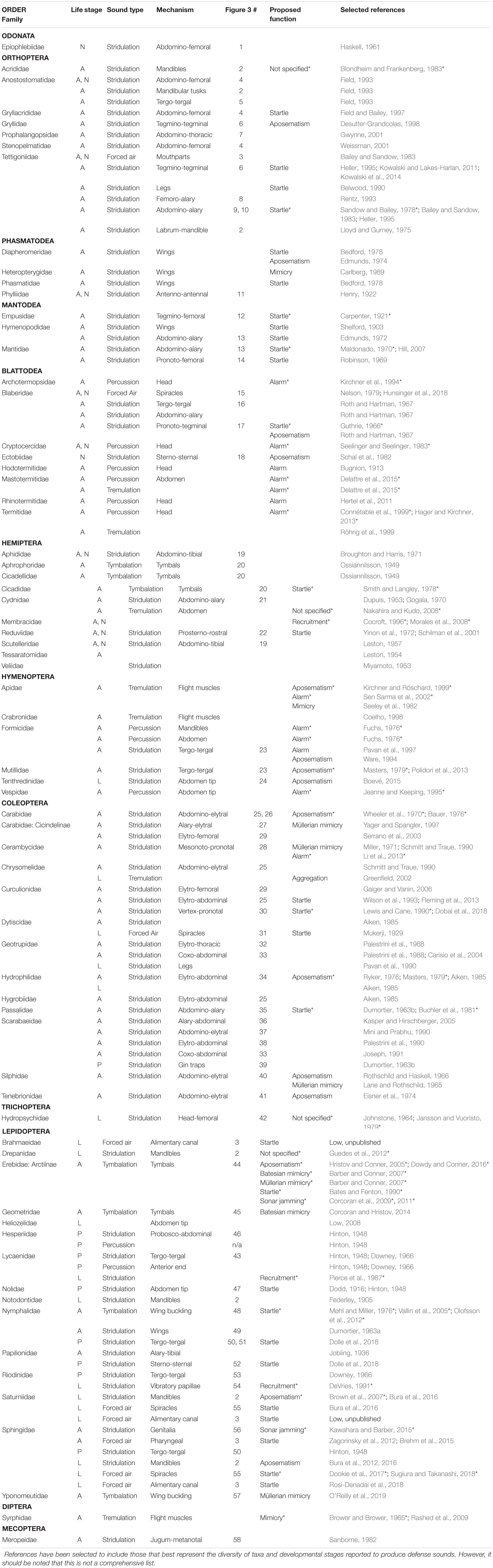
Table 1. Distribution of insect defense sounds across orders, families, and developmental stages including information on mechanisms and proposed functions. A, adult; N, nymph; L, larva; P, pupa. “Figure 3 #” refers to the corresponding label on Figure 3. Asterisks denote cases where the sound was tested with a predator/receiver in the selected reference.
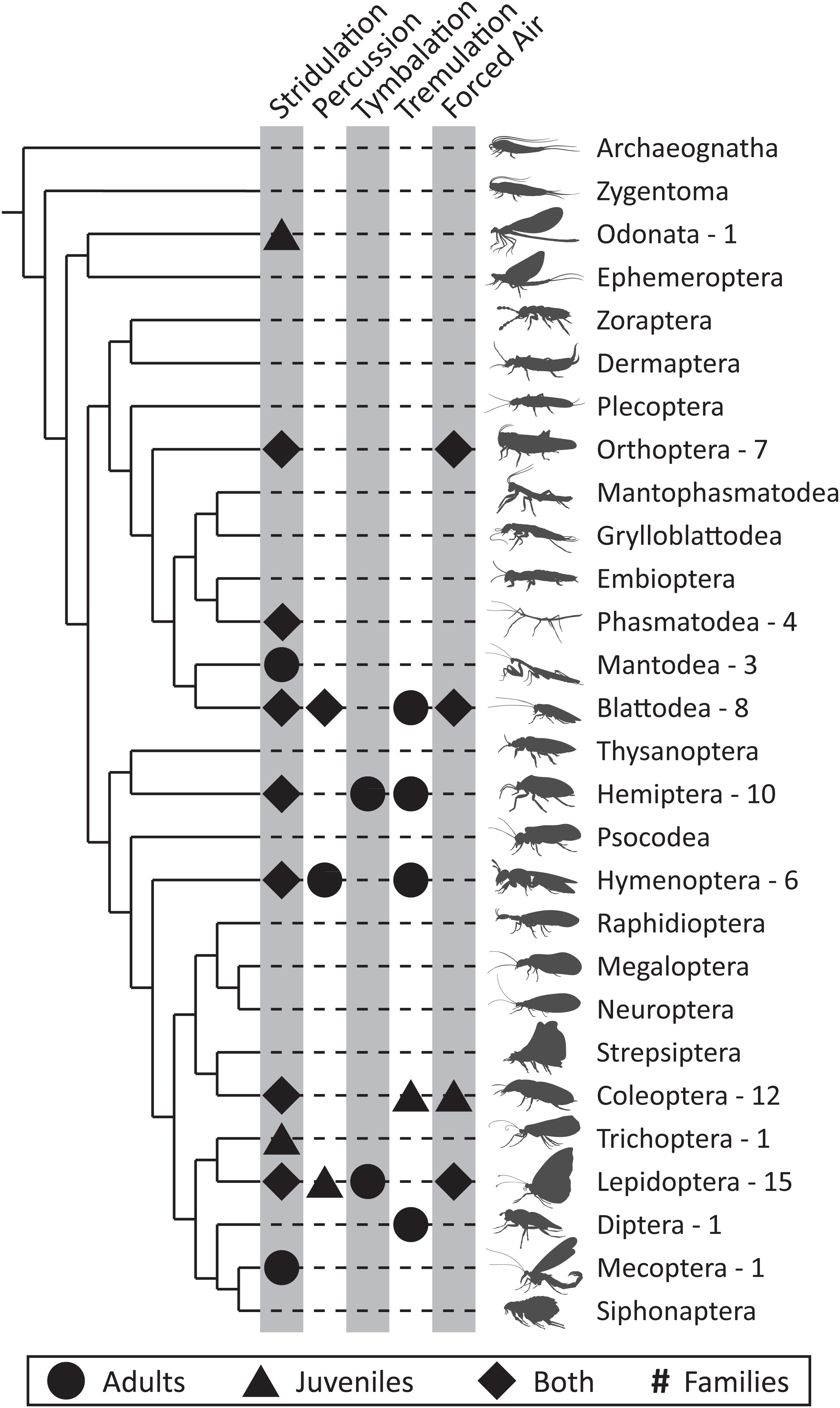
Figure 1. Distribution of defense sounds across orders and life stages of the Class Insecta. The five different mechanisms used to produce defense sounds (stridulation, percussion, tymbalation, tremulation, and forced air) are indicated within their respective columns. Shapes represent the life stage at which defensive sound production occurs, with pupae included under “Juveniles.” Numbers represent the number of families within that order where defensive sound production has been noted. Cladogram adapted from Misof et al. (2014).
Within species that produce defense sounds, the sex that produces these sounds can vary. It is important to note, however, that many studies do not indicate the sex of the specimens tested. Indeed, of the 404 species identified as sound producers in this review, sex was identified in fewer than half the species (194 of 404). In the 152 species for which both sexes were tested, both males and females produce sounds in the majority of cases (126 of 152). Examples include hissing cockroaches (e.g., Nelson and Fraser, 1980) and king crickets (e.g., Field, 1993). Sexual dimorphism of sound features was noted in 21 of these 126 species, and the differences are attributed to morphological traits such as body size (e.g., Coelho, 1998; Hill, 2007), or because the male and female use different mechanisms of sound production (e.g., Kowalski et al., 2014). In the remaining 26 (of 152) species where both sexes were tested, only one sex produces defense sounds. In 20 of these, it’s the male sex, and examples include cicadas (e.g., Smith and Langley, 1978) and katydids (e.g., Kowalski and Lakes-Harlan, 2011). In contrast, there are only 6 species where defense sounds are produced solely by the female, including Heteropteryx dilatata stick insects (Heteropterygidae) (Carlberg, 1989) and Ips pini beetles (Curculionidae) (Dobai et al., 2018). Based on the species investigated to date, when defensive sound production occurs in a species, it is typically produced by both sexes. When only one sex produces sounds, it is usually the male. This male bias could be attributable to males experiencing higher predation risk as they are more mobile when searching for a mate (e.g., Burk, 1982). We recommend that future studies aim to test both sexes wherever possible.
The majority of reports of defensive sound production come from adult insects, but many juvenile life stages, including larvae, nymphs, and pupae, also produce these sounds (Table 1 and Figure 1). We did not, however, find any accounts of insect eggs producing sounds in response to a disturbance. Of the 69 families identified in our study in which defense sounds occur (Table 1), 30 families include reports from juveniles. These examples primarily occur in Lepidoptera (12 families), but also Coleoptera (5), Hemiptera (4), and Blattodea (3). Juvenile sound producers include nymphs in Ectobiidae (Blattodea) (Schal et al., 1982), larvae in Bombycoidea (Lepidoptera) (Bura et al., 2016), and pupae in Lycaenidae and Riodinidae (Lepidoptera) (Hinton, 1948; Downey, 1966). There is no obvious relationship between juvenile and adult defensive sound production within a species. In hemipterans such as Reduviidae (e.g., Yinon et al., 1972) and Membracidae (e.g., Cocroft, 1996), both juveniles and adults can produce defense sounds. However, in Lepidoptera and other holometabolous orders, where a juvenile produces defense sounds, the adult may not. For instance, of the 33 Bombycoidea caterpillar species known to produce defense sounds (Low, unpublished), only two species are reported to produce sounds as adults (e.g., Brehm et al., 2015) and the mechanisms used by the caterpillars and adults differ. Yack (2016) proposed that juvenile insects are under-represented in the literature on acoustic communication, and we argue that this pertains to defensive sound production as well. Juvenile insects are attacked by many predators and parasitoids, and as such there should be selective pressures to produce defense sounds. Comparative analyses among juvenile sonic defenses would be ideal for testing hypotheses on the functions and evolution of these signals, as in juveniles, defensive sound production is not confounded by sounds used in sexual selection. Future research should focus on identifying the distribution and diversity of defensive sound production in juvenile insects including eggs.
Why Don’t All Insects Produce Defense Sounds?
Why is there such diversity with respect to who does and does not produce defense sounds? There are several, non-mutually exclusive explanations. First, morphological or phenotypic traits may enhance or constrain a species’ ability to evolve sound production. For example, smaller insects may not produce airborne defense sounds because such sounds could be too quiet for a predator to hear (Bennet-Clark, 1998), and indeed, we did not find any accounts of defensive sound production in orders with tiny insects (e.g., Psocodea, Thysanoptera, and Siphonaptera, Figure 1). Sclerotization, or hardness, of an insect’s cuticle may restrict sound production to insects with hard exoskeletons (though this would vary by mechanism), and we did find the most defense sound producers in Coleoptera adults and Lepidoptera pupae (Table 1). Additionally, some insects may have co-opted a pre-existing mechanism used in a different signaling context, such as cricket and katydid defensive sound production that is thought to have evolved from sexual signaling (Alexander, 1960) (see section “Evolutionary Origins” below). Second, the assemblage of predators may influence who does or does not produce defense sounds. For instance, tymbal click production varies seasonally in tiger moths of south-eastern Canada whereby species that emerge during peak bat foraging season are more likely to click than species that emerge earlier in the year (Ratcliffe and Nydam, 2008). Third, there are costs to producing defense sounds that may preclude some insects from using them due to trade-offs with other defenses. Corcoran and Woods (2015) found that the metabolic rate of the tiger moth Bertholdia trigona (Arctiinae) is 66% higher when producing sonar jamming sounds than when at rest, but is negligible when compared to the 277% higher rate of flight. If defense sounds are costly to produce, perhaps insects that have already invested in other costly defenses (e.g., chemical defenses, Zvereva and Kozlov, 2015; Knapp et al., 2020) will not produce them. Fourth, the insect’s habitat may not be conducive to transmitting sounds effectively due to interference with sound propagation from absorption, scattering, or masking by background noise (Römer, 2020). For example, insects may be living in wood or soil where the distance that sounds can travel is heavily influenced by both the source amplitude and the properties of the substrate that can absorb or interfere with the sound wave. Hager and Kirchner (2013) found termite vibrational signals produced by an individual have a range of only 0.4 m due to attenuation properties of the soil that dampen the signal amplitude. Despite this limited range, the signals can be propagated through the rest of the nest by receiving termites that relay the signal onward. Finally, sampling biases may explain differences in reports of defensive sound production between taxa, sexes, or developmental stages. For instance, Lepidoptera, Coleoptera, and Hemiptera are not only speciose orders, but also generally well-studied, thereby increasing the likelihood of researchers encountering species that produce defense sounds. Also, some signals may go undetected without the use of specialized recording equipment, such as laser vibrometers that are required to record solid-borne defense sounds. Similarly, in many instances, defense sounds are intended for close range communication with a predator and do not travel long distances (e.g., Bura et al., 2016). In these cases, unless a researcher is explicitly attacking an insect and recording sounds, the signals may not be noticed. For example, Walters et al. (2001) investigated the antipredator defenses of Manduca sexta caterpillars (Sphingidae), but overlooked their defensive clicking [subsequently reported by Bura et al. (2012)] by not recording sounds during their trials. Hypotheses to explain the evolution and variation of traits such as communication signals are being increasingly tested using phylogenetic comparative methods (e.g., acoustic communication in Orthoptera, Song et al., 2020). We believe that using phylogenetic comparative analyses that incorporate morphological traits, predator types, habitats, and other defenses will be particularly insightful for testing and developing hypotheses explaining why some species produce defense sounds while others do not.
Diversity of Mechanisms
Insects are highly acoustic animals and have evolved numerous mechanisms of sound production that occur on all different body parts. The hardened exoskeleton of insects has enabled the evolution of this range of mechanisms, although even soft-bodied insects such as caterpillars can produce sounds using forced air or limited sclerotized body parts (e.g., Bura et al., 2016). Sound-producing mechanisms in insects have been reviewed by several authors, including Haskell (1961), Dumortier (1963b), Ewing (1989), and Hill (2008). These authors use a variety of terms to describe different mechanisms, including stridulation, percussion, vibration, click mechanisms, air expulsion, frictional mechanisms, and vibrating membranes. Even though sound production in insects has been reviewed, sounds produced specifically in a defensive context have not. Here, we describe the various mechanisms used for producing defense sounds as well as the locations on the body where these mechanisms occur.
We recognize five categories of sound-producing mechanisms derived from terms used by Ewing (1989) and Hill (2014): stridulation, percussion, tymbalation, tremulation, and forced air (Figure 2). Stridulation involves the rubbing of two body parts together or one body part on the substrate to produce sounds. The body parts may be unspecialized, such as mandibles rubbing against one another (e.g., Brown et al., 2007), or specialized, such as a file and scraper (e.g., Dobai et al., 2018, Figure 2A). Coleoptera exhibit the greatest diversity of stridulatory mechanisms (Figure 3). Percussion, which involves “tapping” or “drumming” the substrate, is common among ants (e.g., Fuchs, 1976) and termites (e.g., Hager and Kirchner, 2013, Figure 2B) that use these sounds as alarm signals. Tymbalation occurs via the buckling action of specialized body parts, often called tymbals, and is found in Lepidoptera and Hemiptera. This mechanism is well-known in moths that produce anti-bat clicks (e.g., Conner and Corcoran, 2012; Corcoran and Hristov, 2014, Figure 2C), and in cicada anti-predator squawks (e.g., Smith and Langley, 1978). Tremulation involves bodily motions that are transmitted as vibrations through the substrate and is usually produced by bobbing movements (Virant-Doberlet and Čokl, 2004). We broaden this definition of tremulation to include the vibration of flight muscles of both Diptera (e.g., Rashed et al., 2009) and Hymenoptera (e.g., Sen Sarma et al., 2002, Figure 2D). Forced air mechanisms are produced via airflow from an orifice such as spiracles (e.g., Bura et al., 2011) or the alimentary canal (e.g., Brehm et al., 2015; Rosi-Denadai et al., 2018, Figure 2E). All five of these broad categories are used by different insects for defense sounds, but the locations where each is found varies.
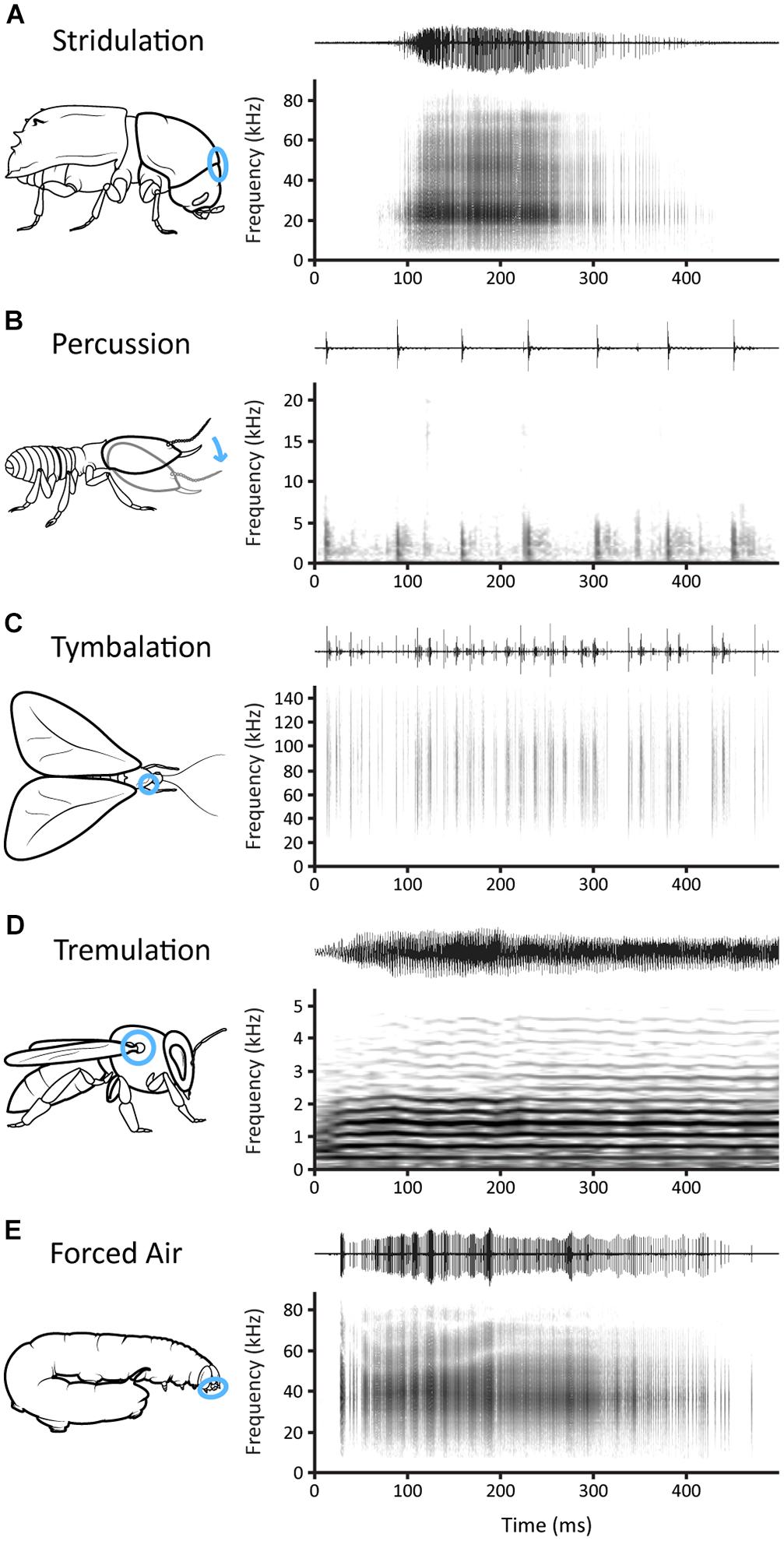
Figure 2. The five types of mechanisms that insects use to produce defense sounds, illustrated by examples from five different insects. Blue circles correspond to the location of the mechanism in the chosen example. Waveforms and corresponding spectrograms show 500 ms of sound from each species. (A) Stridulation: a single chirp produced by an Ips pini bark beetle. Chirps are produced using a file and scraper mechanism located between the head and pronotum. (B) Percussion: a series of drumming signals by a Macrotermes natalensis termite. The signals are produced by tapping the substrate with its head. (C) Tymbalation: a series of clicks produced by a Eubaphe unicolor moth. Clicks are produced via a pair of tymbals located one on each side of the prothorax. (D) Tremulation: a group piping signal produced by Apis florea bees. Piping sounds are produced through vibrations of the flight muscles. (E) Forced Air: a single vocalization produced by an Amphion floridensis caterpillar. Vocalizations are made by airflow from the alimentary canal. Sound files were contributed by the Yack Lab (I. pini and A. floridensis), F. Hager (M. natalensis), A. Corcoran (E. unicolor), and S. Fuchs (A. florea). See text and Table 1 for further details on these examples.
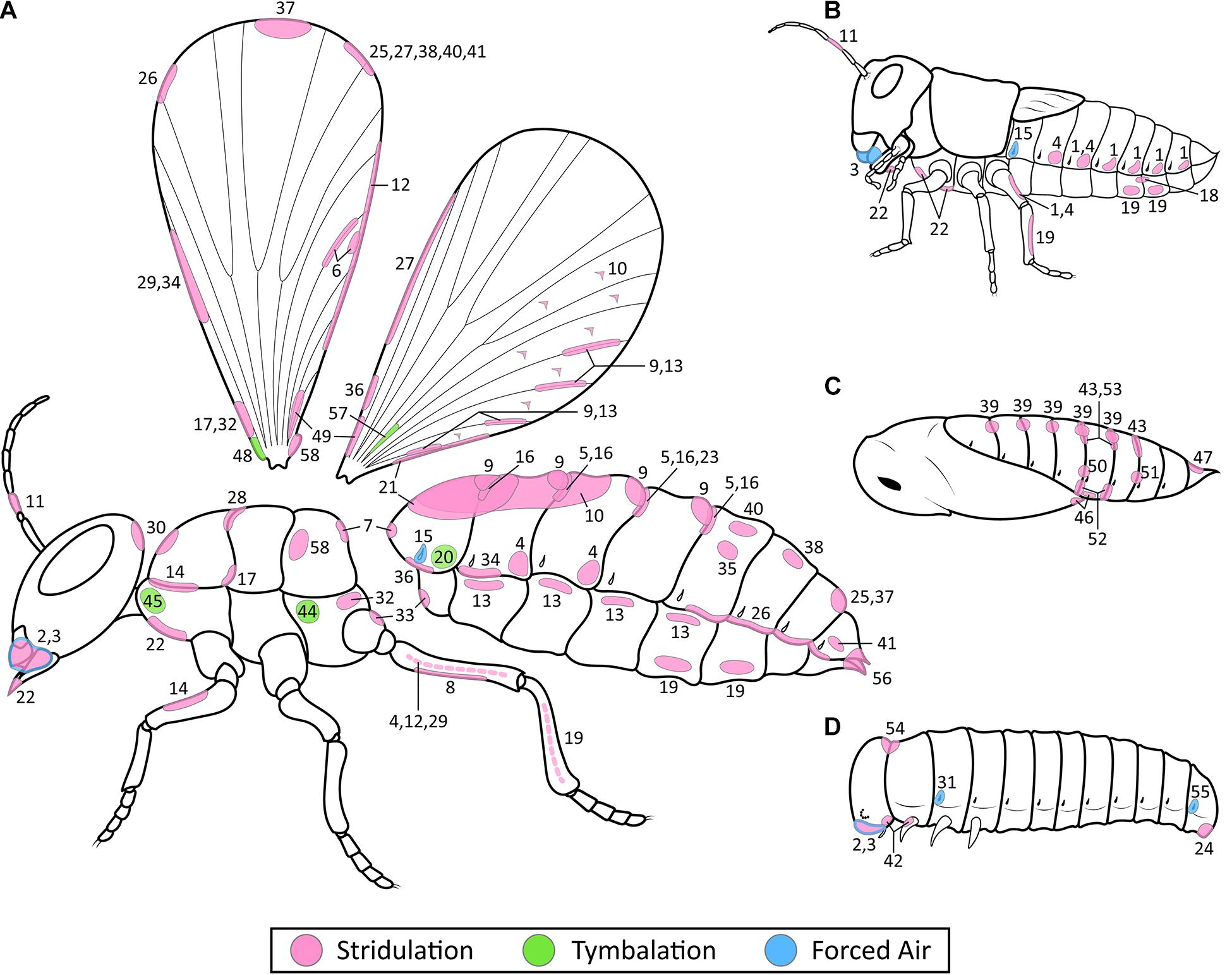
Figure 3. Location of stridulation, tymbalation, and forced air mechanisms used for producing defense sounds in adult and juvenile insects. Percussion and tremulation were excluded as they generally employ undifferentiated body parts. Each number represents a position where a sound-producing mechanism has been recorded in the literature. (A) Generic adult insect. (B) Generic nymph. (C) Generic pupa. (D) Generic larva. The numbers correspond to their order of appearance in Table 1, which includes information on the orders, families, and literature citations. Mechanisms by insect order are as follows: 1: Odonata. 2–3: Orthoptera and Lepidoptera. 4–10: Orthoptera. 11: Phasmatodea. 12–14: Mantodea. 15–18: Blattodea. 19–22: Hemiptera. 23–24: Hymenoptera. 25–41: Coleoptera. 42: Trichoptera. 43–57: Lepidoptera. 58: Mecoptera. Tymbal mechanisms (numbers 20, 44, 45, 48, and 57) are in green. Forced air mechanisms (numbers 3, 15, 31, and 55) are in blue. Stridulatory mechanisms (all other numbers) are in pink. In stridulatory mechanisms that involve two rubbing parts, both parts are given the same number. A dotted line represents a mechanism located on the inner surface of that appendage. Note that numbers 8 and 35 do not have a corresponding location marked on the wings as the entire wing is used in these mechanisms.
Mechanisms used to produce defense sounds have evolved on almost every part of the body, as illustrated in Figure 3. We excluded percussion and tremulation from the figure as these usually involve large undifferentiated regions of the body, such as the head of termites and the flight muscles of flies (Table 1). Also excluded from the figure are mechanisms such as “rustling of the wings” where no specific location is mentioned. Our results show that mechanisms of defensive sound production occur on numerous different body regions in both adults (Figure 3A) and juveniles (Figures 3B–D). Of the 58 mechanisms illustrated, 49 are stridulatory. Stridulatory mechanisms predominantly occur either between neighboring segments (e.g., #7, Figure 3A; #52, Figure 3C), or on a section of the body that can be rubbed by appendages such as the hind legs (e.g., #19, Figures 3A,B) or, in adults only, the wings (e.g., #26, Figure 3A). Tymbals used to produce defense sounds are only noted in adults. These are located on the prothorax (#45, Figure 3A) or metathorax (#44, Figure 3A) in Lepidoptera, and on the first abdominal segment in Hemiptera (#20, Figure 3A). However, some Nymphalidae butterflies use a portion of their forewing membrane as a tymbal-like structure that buckles to produce clicks (#48, Figure 3A) (Møhl and Miller, 1976), and some Yponomeutidae moths use a part of their hind wing in a similar manner (#57, Figure 3A) (O’Reilly et al., 2019). Forced air mechanisms are found in both adults and juveniles. Spiracles are used for air expulsion in larvae of Coleoptera (#31, Figure 3D) and Lepidoptera (#55, Figure 3D), as well as both adults and nymphs in Blattodea (#15, Figures 3A,B). Airflow via the alimentary canal is found in both adults and juveniles of Orthoptera (#3, Figures 3A,B) and Lepidoptera (#3, Figures 3A,D).
Why might a mechanism evolve where it does on a given insect? For stridulation, the mechanisms are found where body parts can be easily rubbed together (Dumortier, 1963b) and the neuromuscular control is likely already available. In hard-bodied insect adults, the site possibilities are vast as demonstrated by the diversity within Coleoptera. In soft-bodied insect larvae, stridulatory mechanisms are restricted to sclerotized areas such as the head and legs (Figure 3D). Tymbalation requires a flexible region of the exoskeleton as well as a method of buckling this region. Tymbals located on the main body occur on or near the thorax where locomotory muscles can be used to pull the tymbal in and out (Wessel et al., 2014). On the other hand, tymbal-like mechanisms on wings will require either an opposing structure to press against (e.g., Møhl and Miller, 1976), or rely on movement of the wings themselves to buckle the flexible wing membrane (e.g., O’Reilly et al., 2019). For forced air, which pair of spiracles evolved for sound production likely depends on the underlying control of airflow within each insect’s tracheal system. Directional airflow is controlled by the coordinated action of the spiracles and ventilatory movements (Heinrich et al., 2013). A spiracle used for sound production likely evolved from one originally used in air output, as demonstrated in hissing cockroaches (Nelson, 1979). The alimentary canal mechanism of forced air may originate from a sucking-pump as proposed for Acherontia atropos moths (Brehm et al., 2015), or from regurgitation as proposed in Amphion floridensis caterpillars (Sphingidae) (Rosi-Denadai et al., 2018). Percussion mechanisms use body parts already equipped with the flexibility to drum on a surface, while for tremulation the only described mechanisms used for defense sounds are flight muscles that can be decoupled from the wings to prevent flight. The morphological variation among and between these mechanisms creates a diversity of signal characteristics, and we explore this relationship in the next section.
How and Why Defense Sounds Vary
Insect defense sounds demonstrate wide variation in their physical characteristics. Sound unit durations (unit being an individual sound as perceived by the human ear, Broughton, 1976) range from 250 μs (tiger beetle clicking, Yager and Spangler, 1997) to periods of over a minute (bee hissing and their mimics, Sen Sarma et al., 2002). Dominant frequencies range from 152 Hz (wasp buzzing, Coelho, 1998) to 90 kHz (hawkmoth stridulation, Kawahara and Barber, 2015). The loudest sound level reported is 110 dB SPL at 8–10 cm (butterfly clicks, Møhl and Miller, 1976) while the quietest reported is 49.8 dB SPL at 2 cm (caterpillar whistling, Sugiura and Takanashi, 2018). This wide variation in signal characteristics disagrees with past proposals that defense sounds tend to share similar characteristics (e.g., Masters, 1980; Schmitt and Traue, 1990; Field, 2001). So why do defense sounds vary? While there are many factors that could explain this variation, we consider the following: morphological influences, the sensory system of the receiver, and the information content of the signal.
An insect’s morphological features can impact their signal characteristics. First, the physical structure of the mechanism itself directly influences the traits of the sound produced (Dumortier, 1963c). For example, the number of ridges on a tiger moth’s tymbal correlates to the maximum click rate of their sounds (Dowdy and Conner, 2019). Second, sound production and propagation are highly affected by body size. Smaller insects not only have less muscle power available to produce sounds, but also have smaller resonating structures that are more efficient at transmitting high frequency than low frequency airborne sounds (Bennet-Clark, 1998). Third, the form of the resonating structures influences the frequencies that are transmitted. For instance, the unspecialized gaster of ants provides a resonating structure that transmits broadband alarm signals (Masters et al., 1983). Crickets on the other hand use a specialized portion of their forewing called the “harp,” and the frequency and bandwidth of the sounds produced depend on how well the harp is coupled to the sound-producing mechanism (Montealegre-Z et al., 2011). However, the latter example has only been studied in a mating context. Fourth, properties of the insect’s exoskeleton will influence the sounds produced. For example, a certain degree of sclerotization is necessary for both percussion and stridulation. Perhaps this is why soft-bodied larvae use fewer of these mechanisms, and why the mechanisms are isolated to sclerotized body parts (see Figure 3D). Researchers should take into account morphological factors when testing hypotheses to explain why defense sounds differ in their physical characteristics.
Just as the hearing of prey insects is shaped by the sounds of their predators (see Yack et al., 2020), the defense sounds of prey should be shaped by their receivers. A receiver may be a predator (vertebrate or invertebrate), parasitoid, or non-predator (conspecific or heterospecific). If the receiver is very specific, then we might expect these signals to be specialized to that receiver. Tiger moth tymbal clicks are an example of targeted defense sounds. The dominant frequency of these clicks is usually ultrasonic, ranging from 28 to 82 kHz (Corcoran et al., 2010), and matches the ultrasonic hearing of insectivorous bats (Sales and Pye, 1974). Similarly, vibratory signals in termites that function to warn colony members of a threat contain most energy between 1 and 5 kHz, matching the frequencies that initiate conspecific behavioral responses (Hager and Kirchner, 2013). In contrast, a defense sound may target a wide range of predators each with different acoustic sensitivities. In such cases, we might expect the sounds to be of broader bandwidth and simpler temporal structure to reflect a range in sensory processing and cognitive skills (Masters, 1980). For instance, stridulation of Dasymutilla sp. wasps (Mutillidae) is broadband, simple, and shown to inhibit attacks by both mice and spiders (Masters, 1979, 1980). Additionally, Antheraea polyphemus caterpillars (Saturniidae) are attacked by a range of invertebrate and vertebrate predators, and their stridulatory defense sounds are broadband and simple in temporal structure (Brown et al., 2007).
Comparing characteristics of sounds produced within a species but in different contexts can lend insights into the selective pressures on defense sounds enacted by receivers. Figure 4 illustrates a few examples of insects that produce sound in both defensive and reproductive contexts. Dendroctonus valens and I. pini bark beetles (Curculionidae) as well as Cyphoderris monstrosa orthopterans (Prophalangopsidae) produce defense sounds that are simpler in temporal structure (e.g., lower pulse rates and shorter durations) than their songs aimed at potential mates (Mason, 1991; Lindeman and Yack, 2015; Dobai et al., 2018; Mason, pers. comm.). One possible explanation for defense sounds being simpler in these species is that they target a broad predator audience, whereas songs directed at potential mates advertise individual characteristics (e.g., skill, health, size, and energy reserves) that are communicated by signal traits. For example, D. valens courtship sounds consist of interrupted chirps proposed to advertise male body size and condition to females, and females prefer more complex interrupted chirps than simpler ones (Figure 4) (Lindeman and Yack, 2015). In Manulea japonica tiger moths (Arctiinae), the opposite is true, as courtship songs produced by males are simpler in temporal structure than defense sounds (Nakano et al., 2013). Females must distinguish between courtship songs and bat sounds as the latter induces a defensive response in females including sound production and/or escape. Perhaps courtship songs evolved to be simpler in temporal structure to prevent the female from responding with an evasive response. At present, there are few studies in insects that compare signal traits of sounds produced in different behavioral contexts (e.g., defense, reproduction, and aggression). In addition to the above-mentioned examples, see also Kowalski and Lakes-Harlan (2010) on ground crickets, and Stölting et al. (2004) on cicadas. We encourage more such studies to better understand receiver selective pressures on defense sound characteristics.
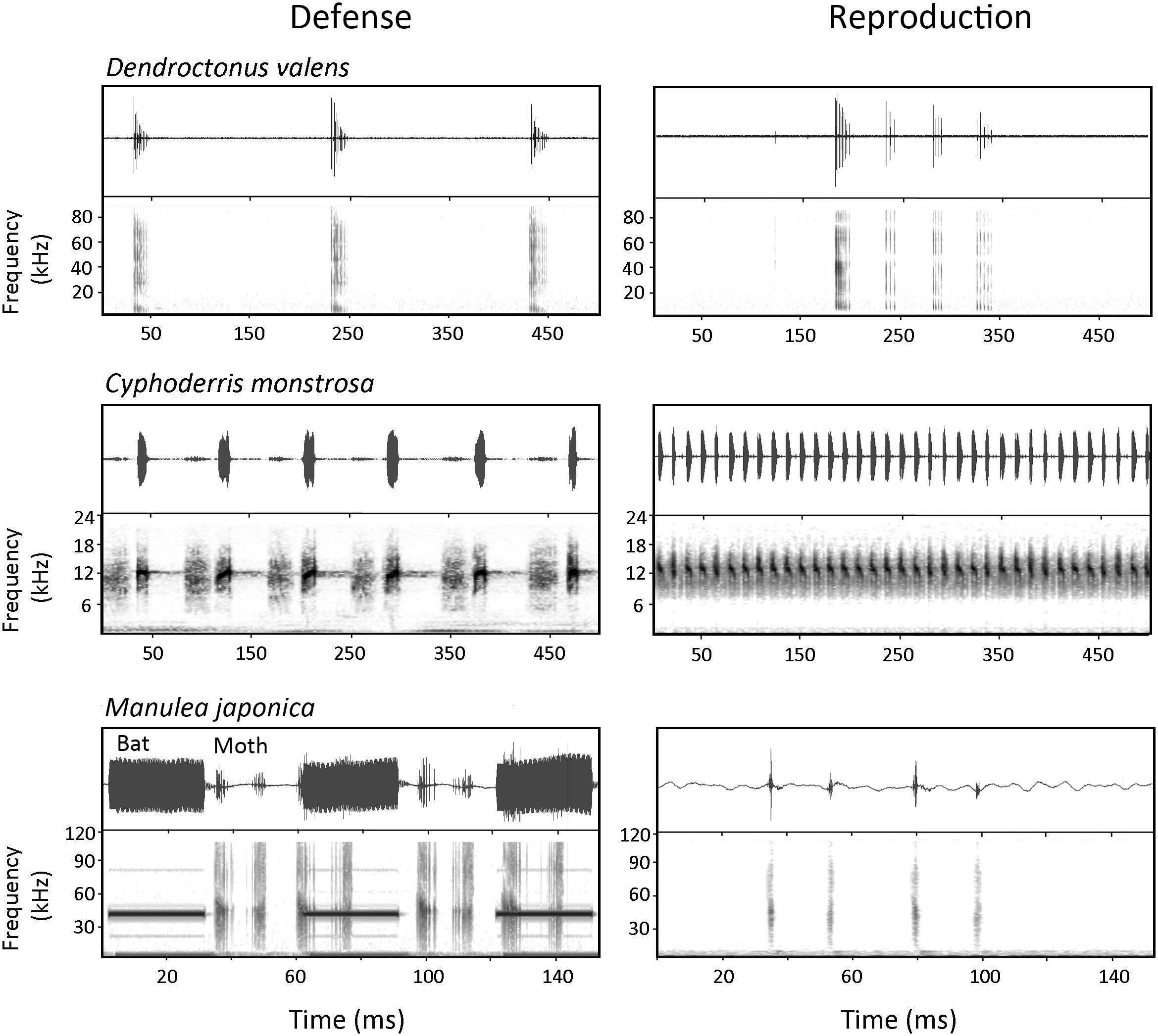
Figure 4. Sounds produced by insects in both defense and reproduction to illustrate contextual differences in signal characteristics. In a defensive context, the male bark beetle Dendroctonus valens produces ‘simple’ chirps that are temporally simple compared to the more complex ‘interrupted’ chirps that are predominant during courtship displays. The male orthopteran Cyphoderris monstrosa produces a series of disyllabic chirps as defense sounds while the calling song is a continuous series of rapid trills. The male Manulea japonica tiger moth produces a burst of tymbal clicks in response to simulated bat calls (simulated bat calls in the spectrogram are shown as longer duration calls at ∼40 kHz that precede the moth clicks), while the courtship tymbal clicks are simpler in structure. Sound files were contributed by the Yack Lab (D. valens), A. Mason (C. monstrosa), and R. Nakano (M. japonica). See text and Table 1 for further details on these examples.
Defense sound characteristics are also proposed to vary according to the message being conveyed (Bura et al., 2016). However, only two authors have tested this hypothesis in insects. Corcoran et al. (2010) found that the signal characteristics of tiger moth anti-bat clicks cluster into two groups: sound units with low duty cycle (proportion of time occupied by sound) are proposed to function as warning sounds, and high duty cycle units as sonar jamming sounds. In Bombycoidea caterpillars, short duration sounds are associated more with chemical defense than longer sounds, which are instead proposed to function in deimatic displays (Bura et al., 2016). The functional implications of different signal characteristics in conveying different messages to receivers are discussed further in the next section.
Proposed Functions of Defense Sounds
A defense sound can be directed at either the attacker or a conspecific. Proposed functions of sounds directed at the attacker include deimatism, aposematism, mimicry, or interference, while those aimed at conspecifics (and sometimes heterospecifics) are usually alarm signals. We define each of these proposed functions based on the broader defense literature (Ruxton et al., 2018) and provide examples if available. We also summarize the predicted receiver responses and defense sound characteristics for each functional category in Figure 5.
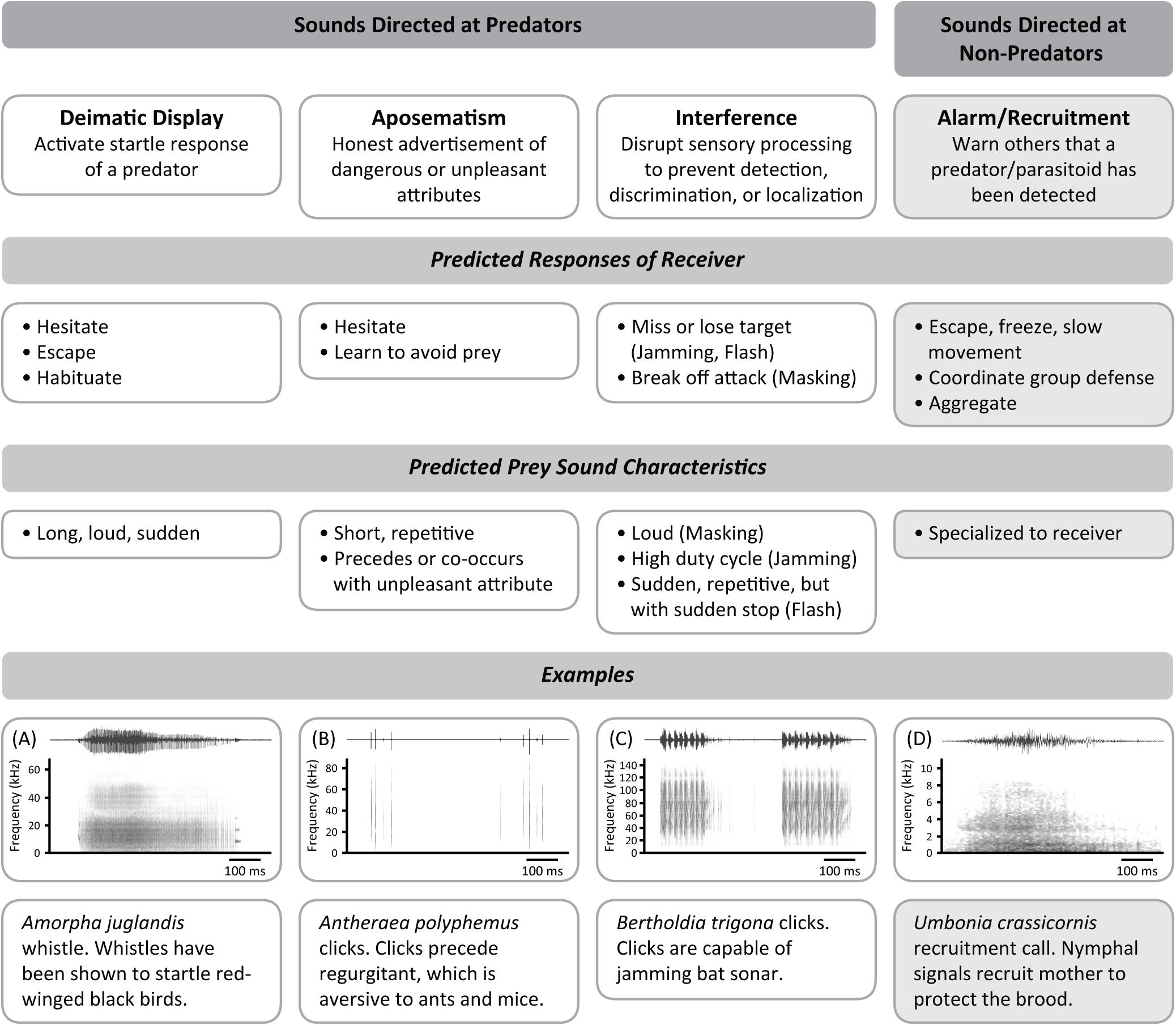
Figure 5. Hypotheses explaining the function of insect defense sounds with corresponding predictions of both prey characteristics and receiver responses. Sound files (A–D), shown as examples, were contributed by the Yack Lab (A. juglandis and A. polyphemus), A. Corcoran (B. trigona), and R. Cocroft (Umbonia crassicornis). See text for further discussion of these functions and corresponding examples.
Signals Directed at Predators
Deimatic Displays
Deimatic displays have been defined as signals that act on the innate startle response of a predator (Skelhorn et al., 2016; Umbers et al., 2017). Although there has been debate on what comprises a deimatic display and how a predator will react (see Umbers et al., 2015; Skelhorn et al., 2016; Umbers and Mappes, 2016), predicted predator responses typically include the predator fleeing from the prey or hesitating long enough for the prey to escape, as well as habituation to the signal with repeated exposure, provided the prey is not otherwise defended (Figure 5) (Skelhorn et al., 2016; Umbers et al., 2017). In the visual realm for example, eyespots in butterflies and caterpillars are often recognized as a form of deimatic display that can cause predators to retreat (e.g., Vallin et al., 2005; Hossie and Sherratt, 2013) and habituate with repeated exposure (e.g., Blest, 1957). In the acoustic realm, there are few experimentally tested examples of deimatic sounds involving predator responses (Table 1). Stridulation in Henschoutedenia epilamproides cockroaches (Blaberidae) startles rats and voles, causing them to withdraw (Guthrie, 1966). Clicking in Aglais io butterflies (Nymphalidae) causes bats to scream, jump, and retreat (Møhl and Miller, 1976), and causes mice to run away without attacking (Olofsson et al., 2012). Whistling in Amorpha juglandis caterpillars (Sphingidae) startles birds, causing them to dive and fly away (Bura et al., 2011; Dookie et al., 2017, Figure 5A), though birds habituate with repeated exposure to the sounds (Dookie et al., 2017). Deimatic sounds in insects have been characterized as being long, loud, and sudden – physical characteristics that are proposed to activate the predator’s startle response (e.g., Hill, 2007; Kowalski et al., 2014). For instance, A. juglandis whistles are 440 ± 272 ms long, 69 to 82 dB SPL at 5 cm, and elicited upon contact (Bura et al., 2011). While insect defense sounds are sometimes proposed to be deimatic based on their signal characteristics alone (e.g., Kowalski et al., 2014; Bura et al., 2016), it is key when assessing any defensive function that the predator’s responses be recorded (Skelhorn et al., 2016). Also, some insects combine deimatic sounds with visual displays, and the components of such multimodal defenses may operate synergistically, or individually to target different predators (Rowe and Halpin, 2013). For example, while clicks alone in A. io butterflies startle bats and mice, it is the hidden eyespots alone (which are revealed simultaneously with the sounds) that have a startling effect on birds (Vallin et al., 2005). This example highlights the necessity of studying responses to defense sounds by different predators. Future research should involve experiments with predators (and not focus only on signal characteristics of the prey) as well as investigate potential synergistic effects of the insect’s entire defensive display.
Aposematism
Aposematism is the honest advertisement of dangerous or unpleasant attributes (Edmunds, 1974; Ruxton et al., 2018; Caro and Ruxton, 2019). These attributes include not only distastefulness or toxicity, but also stinging spines, ability to fight back, and a vicious bite. Naïve predators are predicted to hesitate prior to sampling novel aposematic prey, while experienced predators will have learned to associate the signal with the unpleasant attribute and reject such prey based on the signal alone (Figure 5) (Speed, 2000). For example, red is a common aposematic color that can cause naïve predators to hesitate (Exnerová et al., 2007) and also facilitates learning more so than other colors (Rönkä et al., 2018). A. polyphemus caterpillars (Saturniidae) provide strong evidence for their sounds being aposematic. After simulated attacks or attacks from chicks, caterpillars produce clicking sounds followed by regurgitation. The regurgitant is aversive to ants and mice, and chicks stop their attacks following the caterpillar’s regurgitation (Brown et al., 2007, Figure 5B). In another caterpillar, Saturnia pyri (Saturniidae), short chirping sounds are induced by simulated predator attacks and these sounds precede or accompany the secretion of defensive phenolic chemicals from scoli (bristle-bearing outgrowths) (Bura et al., 2009). These chemicals were shown in previous studies to be aversive to birds and ants (Deml and Dettner, 1993, 1995). Learned avoidance to an aposematic sound has been demonstrated in bats. Bats learn to associate tiger moth tymbal clicks with distastefulness in as few as two exposures to clicking moths, and will thereafter avoid the moths in a lab setting (Hristov and Conner, 2005). Similar to acoustic deimatism, some defended insects may combine sounds with warning coloration or odors, and such multimodal displays may increase the efficacy of an aposematic display or improve predator’s learning of defended prey (Rowe and Halpin, 2013).
While it has been hypothesized that some sound characteristics may be more effective as aposematic signals than others (e.g., Bura et al., 2016), this has not yet been experimentally validated. However, we can draw on visual aposematic characteristics to make predictions. To improve prey discrimination and enhance learning, visual aposematic signals are predicted to be simple, symmetrically patterned, and conspicuous (Forsman and Merilaita, 1999; Ruxton et al., 2018). Simple visual signals such as bold patterns and few colors may be more reliably recognized than complex patterns (Cott, 1940), while symmetric patterns are easier to detect, learn, and remember than asymmetric patterns (Forsman and Merilaita, 1999). We predict the equivalent acoustic traits to be short (simple) and repetitive (symmetrical) sound units that are distinct from the background noise (conspicuous). For example, A. polyphemus aposematic clicks are short (24.7 ± 17.2 ms in duration), repetitive (52.7 ± 82.2 clicks in a train of sound), and distinct (ranging from 58.1 to 78.8 dB SPL at 10 cm) (Brown et al., 2007). Additionally, Masters (1980) noted a short and repetitive trend among disturbance stridulations of beetles, true bugs, and velvet ants, and Bura et al. (2016) found that short sounds correspond to the presence of chemical defenses (regurgitation or secretions from scoli) in caterpillars. These traits may aid in learning and memory retention as reported for clicker training with vertebrates (Smith and Davis, 2008). It has been reported that some moths produce ultrasonic aposematic sounds continually during flight (O’Reilly et al., 2019). Such proposed warning sounds that are always turned ‘on,’ analogous to warning coloration, would be interesting to explore further with live predators. We recommend that future studies focus on identifying effective aposematic sound characteristics and their impacts on a predator’s psychology.
Mimicry
Defensive mimicry occurs when one individual (the mimic) copies the appearance or behavior of another (the model) to gain a selective advantage (Jamie, 2017). There are several ways that mimicry may be used for protective measures, but how a predator is predicted to respond depends primarily on its experience with the model. We address three forms of acoustic mimicry. First, in Müllerian mimicry both the mimic and model are defended, and the predator is predicted to respond as it would to an aposematic signal (Figure 5). Müllerian mimicry may also facilitate a predator’s learning and memory retention of aposematic signals (Bradbury and Vehrencamp, 2011; Ruxton et al., 2018). Acoustic Müllerian mimicry is proposed to occur among defended clicking tiger moth species (Barber and Conner, 2007) and between defended clicking tiger moths and tiger beetles (Yager and Spangler, 1997). Second, in Batesian mimicry an undefended mimic copies an aposematic model. The predicted response of a predator that has had previous experience with the model is to avoid the mimic, whereas if the mimic is encountered prior to the model, then we would not expect a naïve predator to learn to avoid the signal. For example, Barber and Conner (2007) reported that after having sampled Cycnia tenera, a noxious clicking tiger moth (Arctiinae), red and big brown bats refuse palatable, clicking Euchaetes egle tiger moths. Another proposed acoustic Batesian mimic is Eubaphe unicolor (Geometridae), a palatable moth that produces tymbal clicks very similar to its sympatric, unpalatable tiger moth species (Corcoran and Hristov, 2014). Third, it has been proposed that insects can mimic the alarm calls of their predators. In vertebrates, such calls signal danger to conspecifics who react by increasing their vigilance or seeking cover (Fallow et al., 2013). An example of acoustic mimicry in insects is whistling in A. juglandis (Sphingidae) that is proposed to mimic the alarm calls of the caterpillar’s avian predator (Dookie et al., 2017). Further studies on acoustic mimicry in insects should include comparisons of acoustic characteristics between models and mimics to determine their degree of similarity, playback studies to monitor predator responses, and palatability trials using different predators.
Interference Signals
Another way that insects may thwart attack is to interfere with a predator’s sensory processing capabilities by impeding detection, discrimination, or localization of prey. For example, flash coloration (a form of flash behavior) can cause a predator to lose track of its original target when a flash of color is revealed while fleeing, then hidden when stopped (Loeffler-Henry et al., 2018; Caro et al., 2020). Predicted predator responses to interference signals vary based on how the signals affect their cognitive processing. To date, three types of acoustic interference have been described in insects: sonar jamming, flash noise, and masking (Figure 5). Sonar jamming prevents prey localization by disrupting the returning echolocation signals of bats and causing the bats to miss their target (Corcoran et al., 2011). Jamming sounds are predicted to have a high duty cycle (proportion of time occupied by sound) to effectively disrupt echoes (Corcoran et al., 2009), and indeed, B. trigona tiger moths (Arctiinae) produce tymbal sounds with a 44% duty cycle (Corcoran et al., 2011, Figure 5C). However, after reporting that hawkmoths produce jamming sounds via genital stridulation with duty cycles as low as 18%, Kawahara and Barber (2015) proposed that a duty cycle threshold of about 20% or higher is necessary to jam bat sonar. The second type of acoustic interference is flash noise. Similar to flash coloration, flash noise occurs when the prey produces a sound that a predator can track acoustically (Edmunds, 1974). The predator is predicted to lose track of the prey when the signaler falls silent. For instance, a disturbed grasshopper (species unspecified) may be tracked via its wing-beat sounds, but when it lands and is again silent, the predator is unable to pinpoint its location (Edmunds, 1974). We believe that this would be especially true if the grasshopper stops flapping before it hits the ground, leaving a gap between where the sound was last heard and where the insect lands. However, the function of flash noises in hindering predators remains speculative. Masking, the third type of acoustic interference, occurs when one sound makes other sounds difficult to hear (Carterette and Friedman, 1978). Smith and Langley (1978) proposed that cicada disturbance squawks decrease the auditory acuity of a mouse by being loud, possibly preventing the mouse from hearing its own predators while handling the cicada. This could thereby make the cicada unprofitable to pursue, and the mouse would be predicted to break off the attack.
Signals Directed at Non-predators
Alarm Signals
Defense sounds directed at non-predators are referred to as alarm signals and are used to warn conspecifics, and sometimes heterospecifics, that a predator or parasitoid has been detected (Bradbury and Vehrencamp, 2011). Thus, alarm signals are usually found in group-living animals. Acoustic alarm signals in insects are predominantly transmitted and perceived through the substrate as vibrations, though some may have an airborne component (Hunt and Richard, 2013). Generally, receivers of an alarm signal respond by escaping, slowing their movement, coordinating a group defense, or approaching the signaler to provide aid (Figure 5). For example, drumming in Camponotus spp. carpenter ants (Formicidae) induces a context-dependent response where workers that were mostly still prior to the signal will freeze altogether, but those that were already in motion begin moving faster (Fuchs, 1976). Piping by returning foragers of Apis florea honeybees (Apidae) alerts the colony to a nearby threat and initiates coordinated hissing among the whole colony (Sen Sarma et al., 2002). The hissing in turn is thought to be an aposematic signal to approaching predators. Coordinated group responses to alarm signals also include tapping within nests by Parachartergus colobopterus (Vespidae) that calls the wasps outside to spray venom at intruders (Jeanne and Keeping, 1995). Alarm signals can also coordinate group formation, as observed in disturbed Stolas sp. beetle larvae (Chrysomelidae) where vibrational signals recruit other larvae to form a circle (Greenfield, 2002). This coordinated response may offer a survival advantage through safety in numbers (Dury et al., 2014). A rather unique type of alarm signal is the “stop” signal of honeybees (Apidae). When Apis cerana bees encounter danger at a foraging site, they prevent further recruitment to the site by using vibratory signals to stop any waggle dance that would otherwise recruit bees to that site (Dong et al., 2019). Insects that use alarm signals to recruit help to the signaler include Umbonia sp. treehopper nymphs (Membracidae) that produce vibrations when disturbed by a predator (Cocroft, 1996; Ramaswamy and Cocroft, 2009, Figure 5D). As the vibrational signals are transmitted across the brood, the mother responds by approaching the disturbance and often succeeds in repelling the attacker. Recruitment calls can also be directed toward heterospecifics, as seen in Lycaenidae and Riodinidae caterpillars that use solid-borne vibrations to induce ants to attend to them (Pierce et al., 2002). Tending by ants offers protection from predators and parasitoids, with untended caterpillars facing more predation by wasps than tended ones (Pierce et al., 1987; DeVries, 1991). Despite the examples that demonstrate the benefits of acoustic alarm signals to a colony, these signals have mostly been studied in the eusocial Hymenoptera and Blattodea. However, as many other substrate-bound insects (e.g., caterpillars and sawfly larvae) form groups (see Costa, 2006), the role of vibratory communication in mediating group defensive responses should be further explored in these insects.
Evolutionary Origins
How did defense sounds evolve in the first place? There are two possibilities discussed in the literature: (1) they evolved from non-signaling behaviors; or (2) they were co-opted from acoustic signals that evolved for another function. Ideally, testing hypotheses on the evolutionary origins of a trait are conducted using a phylogenetic comparative approach which requires examples of homologous traits at various stages of evolution as well as a robust phylogeny (Petak, 2019). However, to the best of our knowledge this approach has not been used to test hypotheses explaining the origins of insect defense sounds. Nonetheless, we can speculate on the evolutionary origins of sonic defenses given the limited evidence available.
A communication signal can evolve from a non-signaling behavior through the process of ritualization (Scott et al., 2010; Petak, 2019). Non-signaling behaviors such as walking, flying, or using a spray apparatus can produce incidental sounds which act as cues, meaning traits that have not evolved to alter a recipient’s behavior (Yack et al., 2020). Cues can evolve into signals through ritualization whereby they become more conspicuous and stereotyped (Petak, 2019). Ritualization seems the most likely explanation for how sonic defenses evolved, especially when a defense sound is the only form of acoustic communication in a species. Several such hypotheses have been proposed for insects. Alexander (1956) suggested that defense sounds evolved from incidental sounds produced during a struggle with a predator. Nelson and Fraser (1980) proposed that hissing in cockroaches evolved from a spray apparatus since some insects produce defensive froths from their spiracles or other body openings, and can even produce incidental sounds while doing so (e.g., Carpenter, 1938). Tiger moth tymbals and the clicks they produce are proposed to have evolved through modification of the thoracic pleura which buckle when the moth is walking or flying, and so may have been associated with escape behaviors (Fullard, 1992).
A communication signal can also evolve secondarily from a pre-existing signal. For example, ultrasonic courtship signals were co-opted from anti-bat clicks in several tiger moth species (Simmons and Conner, 1996; Weller et al., 1999). Though evolving from a pre-existing signal has been less commonly proposed for defense sounds, there are a few examples. Defense sounds in crickets and katydids are proposed to have evolved from sexual signals due to the ubiquity of acoustic sexual signals in this group and the relative lack of defensive sound production (Alexander, 1960). Cases where only one sex produces both sexual and defense sounds may also indicate that the sonic defenses evolved from a pre-existing sexual signal. For instance, many species of bark beetles show sexual dimorphism in sound production whereby only one sex produces sounds in both sexual and defensive contexts (Bedoya et al., 2019). In cicadas, Smith and Langley (1978) proposed that disturbance squawks evolved secondarily to courtship sounds as both sound types, when produced via tymbals, are found only in males. If signals evolved initially for defense, it is difficult to explain the lack of tymbal sound production in the opposite sex (Smith and Langley, 1978). Territorial signals may provide another origin for some defense sounds, possibly in caddisfly larvae (Hydropsychidae) that produce sounds both when poked with forceps and when other larvae attempt to enter their shelter (Johnstone, 1964). To the best of our knowledge, co-opting a pre-existing acoustic communication signal for use in defensive sound production has yet to be formally investigated.
Future research on the evolutionary origins of defense sounds must involve phylogenetic comparative studies. Such studies require documentation of a series of variable, but homologous, morphological and associated behavioral traits within extant species, as well as a robust phylogeny to assess which traits are ancestral or derived. Behavioral traits are more challenging to document than morphological traits as they must be studied in living specimens. Consequently, there are currently few phylogenetic comparative analyses on the evolution of insect defensive displays (but see Vidal-García et al., 2020). This review has identified several insect groups that are promising for studying evolutionary origins of defense sounds in that the groups display variable sonic defenses and recent phylogenies are available. These insect groups include praying mantids (e.g., Vidal-García et al., 2020), tiger moths (e.g., Zaspel et al., 2014; Dowdy and Conner, 2019), Lepidoptera pupae (e.g., Dolle et al., 2018; Espeland et al., 2018), and Bombycoidea caterpillars (e.g., Bura et al., 2016). These model systems will allow us not only to test hypotheses on evolutionary origins of defense sounds, but also test hypotheses on why some insects evolve sonic defenses and others do not.
Conclusion
Defense sounds occur widely in insects. Yet, compared to our understanding of visual defenses, there are major gaps in our understanding of which insects produce defense sounds, how these sounds promote survival, and how they evolved. Here, we recommend some key, non-mutually exclusive lines of investigation and questions to focus on in future research. First, further testing is necessary to better document the extent of defensive sound production throughout the Class Insecta. Because defense sounds often occur only during close encounters with predators and thus may be “quiet,” they may go unnoticed unless “attacked” by the experimenter. Also, defense sounds that are ultrasonic, or transmitted as solid-borne vibrations, can easily be overlooked by scientists not using specialized equipment. We recommend conducting experiments on those orders that are not well-represented in the literature, being careful to document any differences in the sex of the species being assessed. We also recommend further investigations on juvenile stages such as larvae, nymphs, pupae, and eggs. Second, what are the key selective pressures that led to the evolution of defense sounds? Variables including the types of predators, the presence of other defenses, body size, and life history traits should be documented and analyzed using phylogenetic comparative methods, meta-analyses, and developmental studies to address this question. Third, what explains the wide variation in signal characteristics? How much of this variation is related to factors such as the type of mechanism, the type of message being conveyed, and the hearing of the receiver(s)? We encourage experimental investigations to test the hypothesis that different acoustic characteristics are more efficient at conveying different messages, such as aposematism or deimatism. Fourth, how did defensive sound production evolve originally? Did it evolve from escape or fighting behaviors, or from pre-existing signals used in another context? To understand the evolutionary origins of defense sounds, phylogenetic comparative analyses are necessary. Fifth, and perhaps most important, testing hypotheses on the survival benefits of these sounds requires experiments with natural predators. Most studies have been conducted by humans pinching insects and reporting the sounds. While these studies are important, experiments with a diversity of natural predators are necessary to record the effectiveness of sonic defenses. Such studies should monitor the initial predator responses as well as the effects over time, such as habituation and learning rates. Additionally, the behavior of the prey should be closely monitored to determine how long the display lasts, if the prey tries to escape, and if there are other behaviors associated with sound production. While predator-prey experiments can be challenging to conduct, they are essential to furthering our understanding of the efficacy of survival sounds in insects. Defense sounds clearly play an important role in the survival strategies of many insects, and further research on acoustic communication is critical for establishing a comprehensive understanding of insect predator-prey interactions.
Author Contributions
ML led the project and wrote the first draft. All authors contributed to data collection, editing the manuscript, and figure and table preparation. All authors approved the final version of the manuscript.
Funding
This work was supported by Discovery Grants from the Natural Sciences and Engineering Research Council of Canada (RGPIN 2014-05947 and RGPIN-2020-07056) to JY, and a Postgraduate Scholarship (Doctoral program) from the Natural Sciences and Engineering Research Council of Canada (PGSD3-518236-2018) to ML.
Conflict of Interest
The authors declare that the research was conducted in the absence of any commercial or financial relationships that could be construed as a potential conflict of interest.
Acknowledgments
We are grateful to all those who contributed sounds and images for use in this work: F. Hager, A. Corcoran, R. Cocroft, S. Fuchs, A. Mason, and R. Nakano, and their team members. We also thank the Yack lab members for their feedback at various stages of this manuscript’s development. We thank K. Brzezinski for line drawings in Figures 1–3.
References
Aiken, R. B. (1985). Sound production by aquatic insects. Biol. Rev. 60, 163–211. doi: 10.1111/j.1469-185X.1985.tb00714.x
Alexander, R. D. (1956). A Comparative Study of Sound Production in Insects, With Special Reference to the Singing Orthoptera and Cicadidae of the Eastern United States. Ph.D. dissertation, The Ohio State University, Columbus, OH.
Alexander, R. D. (1960). “Sound communication in Orthoptera and Cicadidae,” in Animal Sounds and Communication, eds W. E. Lanyon and W. N. Tavolga (Washington, DC: American Institute of Biological Sciences), 38–92.
Alexander, R. D. (1967). Acoustical communication in arthropods. Annu. Rev. Entomol. 12, 495–526. doi: 10.1146/annurev.en.12.010167.002431
Bailey, W. J., and Sandow, J. D. (1983). Mechanisms of defensive stridulation in the bushcricket Mygalopsis marki Bailey (Copiphorini, Tettigoniidae). Acta Zool. 64, 117–122. doi: 10.1111/j.1463-6395.1983.tb00789.x
Barber, J. R., and Conner, W. E. (2007). Acoustic mimicry in a predator prey interaction. Proc. Natl. Acad. Sci. 104, 9331–9334. doi: 10.1073/pnas.0703627104
Bates, D. L., and Fenton, M. B. (1990). Aposematism or startle? Predators learn their responses to the defenses of prey. Can. J. Zool. 68, 49–52. doi: 10.1139/z90-009
Bauer, T. (1976). Experimente zur frage der biologischen bedeutung des stridulationsverhaltens von kafern. Z. Tierpsychol. 42, 57–65. doi: 10.1111/j.1439-0310.1976.tb00956.x
Bedford, G. O. (1978). Biology and ecology of the Phasmatodea. Annu. Rev. Entomol. 23, 125–149. doi: 10.1146/annurev.en.23.010178.001013
Bedoya, C. L., Hofstetter, R. W., Nelson, X. J., Hayes, M., Miller, D. R., and Brockerhoff, E. G. (2019). Sound production in bark and ambrosia beetles. Bioacoustics 30, 1–16. doi: 10.1080/09524622.2019.1686424
Belwood, J. J. (1990). “Anti-predator defences and ecology of neotropical forest katydids, especially the Pseudophyllinae,” in The Tettigoniidae: Biology, Systematics and Evolution, eds W. J. Bailey and D. C. F. Rentz (New York, NY: Springer-Verlag), 8–26.
Bennet-Clark, H. C. (1998). Size and scale effects as constraints in insect sound communication. Philos. Trans. R. Soc. B 353, 407–419. doi: 10.1098/rstb.1998.0219
Blest, A. D. (1957). The function of eyespot patterns in the Lepidoptera. Behaviour 11, 209–258. doi: 10.1163/156853956X00048
Blondheim, S. A., and Frankenberg, E. (1983). ‘Protest’ sounds of a grasshopper: predator-deterrent signal? Psyche 90, 387–394. doi: 10.1155/1983/98675
Boevé, J.-L. (2015). Multimodal defensive strategies in larvae of two Hemichroa sawfly species. J. Hymenopt. Res. 46, 25–33. doi: 10.3897/JHR.46.7064
Bradbury, J. W., and Vehrencamp, S. (2011). Principles of Animal Communication, 2nd Edn. Sunderland: Sinauer Associates.
Brehm, G., Fischer, M., Gorb, S., Kleinteich, T., Kühn, B., Neubert, D., et al. (2015). The unique sound production of the Death’s-head hawkmoth (Acherontia atropos (Linnaeus, 1758)) revisited. Sci. Nat. 102:43. doi: 10.1007/s00114-015-1292-5
Broughton, W. B. (1976). Proposal for a new term ‘echeme’ to replace ‘chirp’ in animal acoustics. Physiol. Entomol. 1, 103–106. doi: 10.1111/j.1365-3032.1976.tb00896.x
Broughton, W. B., and Harris, K. M. (1971). First recording of the sound produced by the black citrus aphid, Toxoptera aurantii (Boy.). Bull. Entomol. Res. 60, 559–563. doi: 10.1017/S0007485300042322
Brower, J. V. Z., and Brower, L. P. (1965). Experimental studies of mimicry. 8. Further investigations of honeybees (Apis mellifera) and their dronefly mimics (Eristalis spp.). Am. Nat. 99, 173–187. doi: 10.1086/282365
Brown, S. G., Boettner, G. H., and Yack, J. E. (2007). Clicking caterpillars: acoustic aposematism in Antheraea polyphemus and other Bombycoidea. J. Exp. Biol. 210, 993–1005. doi: 10.1242/jeb.001990
Buchler, E. R., Wright, T. B., and Brown, E. D. (1981). On the functions of stridulation by the passalid beetle Odontotaenius disjunctus (Coleoptera: Passalidae). Anim. Behav. 29, 483–486. doi: 10.1016/S0003-3472(81)80108-X
Bura, V. L., Fleming, A. J., and Yack, J. E. (2009). What’s the buzz? Ultrasonic and sonic warning signals in caterpillars of the great peacock moth (Saturnia pyri). Naturwissenschaften 96, 713–718. doi: 10.1007/s00114-009-0527-8
Bura, V. L., Hnain, A. K., Hick, J. N., and Yack, J. E. (2012). Defensive sound production in the tobacco hornworm, Manduca sexta (Bombycoidea: Sphingidae). J. Insect Behav. 25, 114–126. doi: 10.1007/s10905-011-9282-8
Bura, V. L., Kawahara, A. Y., and Yack, J. E. (2016). A comparative analysis of sonic defences in Bombycoidea caterpillars. Sci. Rep. 6:31469. doi: 10.1038/srep31469
Bura, V. L., Rohwer, V. G., Martin, P. R., and Yack, J. E. (2011). Whistling in caterpillars (Amorpha juglandis, Bombycoidea): sound-producing mechanism and function. J. Exp. Biol. 214, 30–37. doi: 10.1242/jeb.046805
Burk, T. (1982). Evolutionary significance of predation on sexually signalling males. Florida Entomol. 65:90. doi: 10.2307/3494148
Carisio, L., Palestrini, C., and Rolando, A. (2004). Stridulation variability and morphology: an examination in dung beetles of the genus Trypocopris (Coleoptera, Geotrupidae). Popul. Ecol. 46, 27–37. doi: 10.1007/s10144-004-0170-3
Carlberg, U. (1989). Defensive stridulation in Heteropteryx dilatata Parkinson (Insecta: Phasmida). Zool. Anz. 223, 165–173.
Caro, T., Raees, H., and Stankowich, T. (2020). Flash behavior in mammals? Behav. Ecol. Sociobiol. 74:44. doi: 10.1007/s00265-020-2819-0
Caro, T., and Ruxton, G. D. (2019). Aposematism: unpacking the defences. Trends Ecol. Evol. 34, 595–604. doi: 10.1016/j.tree.2019.02.015
Carpenter, G. D. H. (1921). Experiments on the relative edibility of insects, with special reference to their coloration. Trans. R. Entomol. Soc. Lond. 69, 1–105. doi: 10.1111/j.1365-2311.1921.tb02803.x
Carpenter, G. D. H. (1938). Audible emission of defensive froth by insects. Proc. Zool. Soc. Lond. Ser. A 108, 243–252. doi: 10.1111/j.1469-7998.1938.tb07897.x
Carterette, E., and Friedman, M. (1978). Handbook of Perception, Volume IV: Hearing. London: Academic Press, doi: 10.1016/B978-0-12-161904-6.X5001-5
Cocroft, R. B. (1996). Insect vibrational defence signals. Nature 382, 679–680. doi: 10.1007/s00376-0122057-0
Coelho, J. R. (1998). An acoustical and physiological analysis of buzzing in cicada killer wasps (Sphecius speciosus). J. Comp. Physiol. A 183, 745–751. doi: 10.1007/s003590050297
Conner, W. E. (2014). “Adaptive sounds and silences: acoustic anti-predator strategies in insects,” in Insect Hearing and Acoustic Communication, Animal Signals and Communication, ed. B. Hedwig (Berlin: Springer-Verlag), 65–79. doi: 10.1007/978-3-642-40462-7
Conner, W. E., and Corcoran, A. J. (2012). Sound strategies: the 65-million-year-old battle between bats and insects. Annu. Rev. Entomol. 57, 21–39. doi: 10.1146/annurev-ento-121510-133537
Connétable, S., Robert, A., Bouffault, F., and Bordereau, C. (1999). Vibratory alarm signals in two sympatric higher termite species: Pseudacanthotermes spiniger and P. militaris (Termitidae, Macrotermitinae). J. Insect Behav. 12, 329–342. doi: 10.1023/A:1020887421551
Corcoran, A. J., Barber, J. R., and Conner, W. E. (2009). Tiger moth jams bat sonar. Science 325, 325–327. doi: 10.1126/science.1174096
Corcoran, A. J., Barber, J. R., Hristov, N. I., and Conner, W. E. (2011). How do tiger moths jam bat sonar? J. Exp. Biol. 214, 2416–2425. doi: 10.1242/jeb.054783
Corcoran, A. J., Conner, W. E., and Barber, J. R. (2010). Anti-bat tiger moth sounds: form and function. Curr. Zool. 56, 358–369. doi: 10.1093/czoolo/56.3.358
Corcoran, A. J., and Hristov, N. I. (2014). Convergent evolution of anti-bat sounds. J. Comp. Physiol. A 200, 811–821. doi: 10.1007/s00359-014-0924-0
Corcoran, A. J., and Woods, H. A. (2015). Negligible energetic cost of sonar jamming in a bat–moth interaction. Can. J. Zool. 93, 331–335. doi: 10.1139/cjz-2014-0231
Darwin, C. (1889). The Descent of Man and Selection in Relation to Sex, 2nd Edn. New York, NY: D. Appleton and Co.
Delattre, O., Sillam-Dussès, D., Jandák, V., Brothánek, M., Rücker, K., Bourguignon, T., et al. (2015). Complex alarm strategy in the most basal termite species. Behav. Ecol. Sociobiol. 69, 1945–1955. doi: 10.1007/s00265-015-2007-9
Deml, R., and Dettner, K. (1993). Biogenic amines and phenolics characterize the defensive secretion of saturniid caterpillars (Lepidoptera: Saturniidae): a comparative study. J. Comp. Physiol. B 163, 123–132. doi: 10.1007/BF00263597
Deml, R., and Dettner, K. (1995). Effects of emperor moth larval secretions, hemolymph, and components on microorganisms and predators. Entomol. Exp. Appl. 76, 287–293. doi: 10.1111/j.1570-7458.1995.tb01972.x
Desutter-Grandcolas, L. (1998). First analysis of a disturbance stridulation in crickets, Brachytrupes tropicus (Orthoptera: Grylloidea: Gryllidae). J. Insect Behav. 11, 149–158. doi: 10.1023/A:1020878802954
DeVries, P. (1991). Mutualism between Thisbe irenea butterflies and ants, and the role of ant ecology in the evolution of larval-ant associations. Biol. J. Linn. Soc. 43, 179–195. doi: 10.1111/j.1095-8312.1991.tb00592.x
Dobai, A., Sivalinghem, S., Guedes, R. N. C., and Yack, J. E. (2018). Acoustic communication in the pine engraver bark beetle: do signals vary between behavioural contexts? Physiol. Entomol. 43, 30–41. doi: 10.1111/phen.12222
Dodd, F. (1916). “Noise-producing Lepidoptera,” in Contributions à L’étude des Grands Lépidoptères d’Australie, Études de Lépidoptérologie Comparée, eds C. Oberthür, C. Houlbert, and F. Dodd (Rennes: Imprimerie Oberthür), 13–14.
Dolle, P., Klein, P., Fischer, O. W., Schnitzler, H. U., Gilbert, L. E., and Boppré, M. (2018). Twittering pupae of papilionid and nymphalid butterflies (Lepidoptera): novel structures and sounds. Ann. Entomol. Soc. Am. 111, 341–354. doi: 10.1093/aesa/say029
Dong, S., Tan, K., Zhang, Q., and Nieh, J. C. (2019). Playbacks of Asian honey bee stop signals demonstrate referential inhibitory communication. Anim. Behav. 148, 29–37. doi: 10.1016/j.anbehav.2018.12.003
Dookie, A. L., Young, C. A., Lamothe, G., Schoenle, L. A., and Yack, J. E. (2017). Why do caterpillars whistle at birds? Insect defence sounds startle avian predators. Behav. Processes 138, 58–66. doi: 10.1016/j.beproc.2017.02.002
Dowdy, N. J., and Conner, W. E. (2016). Acoustic aposematism and evasive action in select chemically defended arctiine (Lepidoptera: Erebidae) species: nonchalant or not? PLoS One 11:e0152981. doi: 10.1371/journal.pone.0152981
Dowdy, N. J., and Conner, W. E. (2019). Characteristics of tiger moth (Erebidae: Arctiinae) anti-bat sounds can be predicted from tymbal morphology. Front. Zool. 16:45. doi: 10.1186/s12983-019-0345-6
Dumortier, B. (1963a). “Ethological and physiological study of sound emissions in Arthropoda,” in Acoustic Behaviour of Animals, ed. R.-G. Busnel (London: Elsevier Publishing Company), 583–649.
Dumortier, B. (1963b). “Morphology of sound emission apparatus in Arthropoda,” in Acoustic Behaviour of Animals, ed. R.-G. Busnel (London: Elsevier Publishing Company), 277–345.
Dumortier, B. (1963c). “The physical characteristics of sound production in Arthropoda,” in Acoustic Behaviour of Animals, ed. R.-G. Busnel (London: Elsevier Publishing Company), 346–373.
Dupuis, C. (1953). Notes, remarques et observations diverses sur les Hémiptères. VI – appareil stridulatoire et stridulation des cydnidae et tessaratomidae. Cah. des Nat. 8, 25–27.
Dury, G. J., Bede, J. C., and Windsor, D. M. (2014). Preemptive circular defence of immature insects: definition and occurrences of cycloalexy revisited. Psyche 2014:642908. doi: 10.1155/2014/642908
Edmunds, M. (1972). Defensive behaviour in Ghanian praying mantids. Zool. J. Linn. Soc. 51, 1–32. doi: 10.1111/j.1096-3642.1972.tb00771.x
Eisner, T., Aneshansley, D. J., Eisner, M., Rutowski, R., Chong, B., and Meinwald, J. (1974). Chemical defense and sound production in Australian tenebrionid beetles (Adelium spp.). Psyche 81, 189–208. doi: 10.1155/1974/63815
Eisner, T., Yack, J. E., and Aneshansley, D. J. (2001). Acoustic concomitants of the defensive discharges of a primitive bombardier beetle (Metrius contractus). Chemoecology 11, 221–223. doi: 10.1007/PL00001854
Espeland, M., Breinholt, J., Willmott, K. R., Warren, A. D., Vila, R., Toussaint, E. F. A., et al. (2018). A comprehensive and dated phylogenomic analysis of butterflies. Curr. Biol. 28, 770–778. doi: 10.1016/j.cub.2018.01.061
Ewing, A. W. (1989). Arthropod Bioacoustics: Neurobiology and Behaviour. New York, NY: Cornell University Press.
Exnerová, A., Štys, P., Fučíková, E., Veselá, S., Svádová, K., Prokopová, M., et al. (2007). Avoidance of aposematic prey in European tits (Paridae): learned or innate? Behav. Ecol. 18, 148–156. doi: 10.1093/beheco/arl061
Fallow, P. M., Pitcher, B. J., and Magrath, R. D. (2013). Alarming features: birds use specific acoustic properties to identify heterospecific alarm calls. Proc. R. Soc. B 280:20122539. doi: 10.1098/rspb.2012.2539
Field, L. H. (1993). Structure and evolution of stridulatory mechanisms in New Zealand wetas (Orthoptera: Stenopelmatidae). Int. J. Insect Morphol. Embryol. 22, 163–183. doi: 10.1016/0020-7322(93)90008-O
Field, L. H. (2001). The Biology of Wetas, King Crickets and their Allies. New York, NY: CABI Publishing.
Field, L. H., and Bailey, W. J. (1997). Sound production in primitive Orthoptera from Western Australia: sounds used in defence and social communication in Ametrus sp. and Hadrogryllacris sp. (Gryllacrididae: Orthoptera). J. Nat. Hist. 31, 1127–1141. doi: 10.1080/00222939700770591
Fleming, A. J., Lindeman, A. A., Carroll, A. L., and Yack, J. E. (2013). Acoustics of the mountain pine beetle (Dendroctonus ponderosae) (Curculionidae, Scolytinae): sonic, ultrasonic, and vibration characteristics. Can. J. Zool. 91, 235–244. doi: 10.1139/cjz-2012-0239
Forsman, A., and Merilaita, S. (1999). Fearful symmetry: pattern size and asymmetry affects aposematic signal efficacy. Evol. Ecol. 13, 131–140. doi: 10.1023/A:1006630911975
Fuchs, S. (1976). An informational analysis of the alarm communication by drumming behavior in nests of carpenter ants (Camponotus, Formicidae, Hymenoptera). Behav. Ecol. Sociobiol. 1, 315–336. doi: 10.1007/BF00300070
Fullard, J. H. (1992). The neuroethology of sound production in tiger moths (Lepidoptera, Arctiidae) I. Rhythmicity and central control. J. Comp. Physiol. A 170, 575–588. doi: 10.1007/BF00199334
Gaiger, F., and Vanin, S. A. (2006). The elytro-femoral stridulatory apparatus in Curculionidae (Coleoptera), with notes on the acoustic behaviour of Arniticus hylobioides (Boheman 1843) and Erodiscus proximus (Viana 1959), and thanatosis display in the latter species. Ann. Soc. Entomol. Fr. 42, 165–170. doi: 10.1080/00379271.2006.10700619
Gogala, M. (1970). Artspezifitat der Lautausserungen bei Erdwanzen (Heteroptera, Cydnidae). Z. Vgl. Physiol. 70, 20–28. doi: 10.1007/BF00299533
Greenfield, M. D. (2002). Signalers and Receivers: Mechanisms and Evolution of Arthropod Communication. Oxford: Oxford University Press.
Guedes, R. N. C., Matheson, S. M., Frei, B., Smith, M. L., and Yack, J. E. (2012). Vibration detection and discrimination in the masked birch caterpillar (Drepana arcuata). J. Comp. Physiol. A 198, 325–335. doi: 10.1007/s00359-012-0711-8
Gwynne, D. T. (2001). Katydid and Bush-Crickets: Reproductive Behaviour and Evolution of the Tettigoniidae. Ithaca, NY: Cornell University Press.
Hager, F. A., and Kirchner, W. H. (2013). Vibrational long-distance communication in the termites Macrotermes natalensis and Odontotermes sp. J. Exp. Biol. 216, 3249–3256. doi: 10.1242/jeb.086991
Hager, F. A., Krausa, K., and Kirchner, W. H. (2019). “Vibrational behavior in termites (Isoptera),” in Biotremology: Studying Vibrational Behavior, eds P. S. Hill, R. Lakes-Harlan, V. Mazzoni, P. Narins, M. Virant-Doberlet, and A. Wessel (New York, NY: Springer International Publishing), 309–327. doi: 10.1007/978-3-030-22293-2
Heinrich, E. C., McHenry, M. J., and Bradley, T. J. (2013). Coordinated ventilation and spiracle activity produce unidirectional airflow in the hissing cockroach, Gromphadorhina portentosa. J. Exp. Biol. 216, 4473–4482. doi: 10.1242/jeb.088450
Heller, K.-G. (1995). Acoustic signalling in palaeotropical bushcrickets (Orthoptera: Tettigonioidea: Pseudophyllidae): does predation pressure by eavesdropping enemies differ in the Palaeo- and Neotropics? J. Zool. 237, 469–485. doi: 10.1111/j.1469-7998.1995.tb02775.x
Hertel, H., Hanspach, A., and Plarre, R. (2011). Differences in alarm responses in drywood and subterranean termites (Isoptera: Kalotermitidae and Rhinotermitidae) to physical stimuli. J. Insect Behav. 24, 106–115. doi: 10.1007/s10905-010-9240-x
Hill, P. S. (2014). “Stretching the paradigm or building a new? Development of a cohesive language for vibrational communication,” in Studying Vibrational Communication, eds R. B. Cocroft, M. Gogala, P. S. Hill, and A. Wessel (New York, NY: Springer Berlin Heidelberg), 13–30. doi: 10.1007/978-3-662-43607-3_2
Hill, S. A. (2007). Sound generation in Mantis religiosa (Mantodea: Mantidae): stridulatory structures and acoustic signal. J. Orthoptera Res. 16, 35–49.
Hossie, T. J., and Sherratt, T. N. (2013). Defensive posture and eyespots deter avian predators from attacking caterpillar models. Anim. Behav. 86, 383–389. doi: 10.1016/j.anbehav.2013.05.029
Hristov, N. I., and Conner, W. E. (2005). Sound strategy: acoustic aposematism in the bat-tiger moth arms race. Naturwissenschaften 92, 164–169. doi: 10.1007/s00114-005-0611-7
Hunsinger, E., Root-Gutteridge, H., Cusano, D. A., and Parks, S. E. (2018). A description of defensive hiss types in the flat horned hissing cockroach (Aeluropoda insignis). Bioacoustics 27, 261–271. doi: 10.1080/09524622.2017.1327371
Hunt, J. H., and Richard, F. J. (2013). Intracolony vibroacoustic communication in social insects. Insectes Soc. 60, 403–417. doi: 10.1007/s00040-013-0311-9
Jamie, G. A. (2017). Signals, cues and the nature of mimicry. Proc. R. Soc. B Biol. Sci. 284:20162080. doi: 10.1098/rspb.2016.2080
Jansson, A., and Vuoristo, T. (1979). Significance of stridulation in larval Hydropsychidae (Trichoptera). Behaviour 71, 167–186.
Jeanne, R. L., and Keeping, M. G. (1995). Venom spraying in Parachartergus colobopterus: a novel defensive behavior in a social wasp (Hymenoptera: Vespidae). J. Insect Behav. 8, 433–442. doi: 10.1007/BF01995317
Jobling, B. (1936). On the stridulation of the females of Parnassius mnemosyne L. Proc. R. Entomol. Soc. Lond. A. 11, 66–68. doi: 10.1111/j.1365-3032.1936.tb00871.x
Johnstone, G. W. (1964). Stridulation by larval Hydropsychidae (Trichoptera). Proc. R. Entomol. Soc. Lond. A. 39, 146–150. doi: 10.1111/j.1365-3032.1964.tb00997.x
Joseph, K. J. (1991). SEM study of the stridulatory organs in the giant dung beetle Heliocopris dominis (Scarabaeidae) with observations on the significance of the sound production. Entomon 16, 319–322.
Kasper, J., and Hirschberger, P. (2005). Stridulation in Aphodius dung beetles: songs and morphology of stridulatory organs in North American Aphodius species (Scarabaeidae). J. Nat. Hist. 39, 91–99. doi: 10.1080/00222930310001018877
Kawahara, A. Y., and Barber, J. R. (2015). Tempo and mode of antibat ultrasound production and sonar jamming in the diverse hawkmoth radiation. Proc. Natl. Acad. Sci.U.S.A. 112, 6407–6412. doi: 10.1073/pnas.1416679112
Kirchner, W. H., Broecker, I., and Tautz, J. (1994). Vibrational alarm communication in the damp-wood termite Zootermopsis nevadensis. Physiol. Entomol. 19, 187–190. doi: 10.1111/j.1365-3032.1994.tb01041.x
Kirchner, W. H., and Röschard, J. (1999). Hissing in bumblebees: an interspecific defence signal. Insectes Soc. 46, 239–243. doi: 10.1007/s000400050140
Knapp, M., Řeřicha, M., and Židlická, D. (2020). Physiological costs of chemical defence: repeated reflex bleeding weakens the immune system and postpones reproduction in a ladybird beetle. Sci. Rep. 10:9266. doi: 10.1038/s41598-020-66157-9
Kowalski, K. N., and Lakes-Harlan, R. (2010). Sounds, behaviour, and auditory receptors of the armoured ground cricket, Acanthoplus longipes. J. Insect Sci. 10:59. doi: 10.1673/031.010.5901
Kowalski, K. N., and Lakes-Harlan, R. (2011). Temporal patterns of intra- and interspecific acoustic signals differ in two closely related species of Acanthoplus (Orthoptera: Tettigoniidae: Hetrodinae). Zoology 114, 29–35. doi: 10.1016/j.zool.2010.09.002
Kowalski, K. N., Lakes-Harlan, R., Lehmann, G. U. C., and Strauß, J. (2014). Acoustic defence in an insect: characteristics of defensive stridulation and differences between the sexes in the tettigoniid Poecilimon ornatus (Schmidt 1850). Zoology 117, 329–336. doi: 10.1016/j.zool.2014.04.007
Lane, C., and Rothschild, M. (1965). A case of Müllerian mimicry of sound. Proc. R. Entomol. Soc. Lond. A 40, 156–158. doi: 10.1111/j.1365-3032.1965.tb00305.x
Leston, D. (1954). Strigils and stridulation in Pentatomoidea (Hem.): some new data and a review. Entomol. Mon. Mag. 90, 49–56.
Leston, D. (1957). The stridulatory mechanisms in terrestrial species of Hemiptera Heteroptera. Proc. Zool. Soc. Lond. 128, 369–386. doi: 10.1111/j.1096-3642.1957.tb00331.x
Lewis, E. E., and Cane, J. H. (1990). Stridulation as a primary anti-predator defence of a beetle. Anim. Behav. 40, 1003–1004. doi: 10.1016/S0003-3472(05)81011-5
Li, L., Sun, F., Hu, J., Yin, Y., and Chi, D. (2013). Ultrastructure of stridulating organ of Xylotrechus rusticus L. (Coleoptera, Cerambycidae) and behavioral responses to alarm sounds. J. For. Res. 24, 547–552. doi: 10.1007/s11676-013-0386-1
Lindeman, A. A., and Yack, J. E. (2015). What is the password? Female bark beetles (Scolytinae) grant males access to their galleries based on courtship song. Behav. Processes 115, 123–131. doi: 10.1016/j.beproc.2015.03.009
Lloyd, J. E., and Gurney, A. B. (1975). Labral stridulation in a katydid (a coconut-infesting “treehopper”) (Orthoptera: Tettigoniidae: Mecopodinae). Entomol. News 86, 47–50.
Loeffler-Henry, K., Kang, C., Yip, Y., Caro, T., and Sherratt, T. N. (2018). Flash behavior increases prey survival. Behav. Ecol. 29, 528–533. doi: 10.1093/beheco/ary030
Low, C. (2008). Seismic behaviors of a leafminer, Antispila nysaefoliella (Lepidoptera: Heliozelidae). Florida Entomol. 91, 604–609. doi: 10.1653/0015-4040-91.4.604
Maldonado, H. (1970). The deimatic reaction in the praying mantis Stagmatoptera biocellata. Z. Vgl. Physiol. 68, 60–71. doi: 10.1007/BF00297812
Mason, A. C. (1991). Hearing in a primitive ensiferan: the auditory system of Cyphoderris monstrosa (Orthoptera: Haglidae). J. Comp. Physiol. A 168, 351–363. doi: 10.1007/BF00198354
Masters, W. M. (1979). Insect disturbance stridulation: its defensive role. Behav. Ecol. Sociobiol. 5, 187–200. doi: 10.1007/BF00293305
Masters, W. M. (1980). Insect disturbance stridulation: characterization of airborne and vibrational components of the sound. J. Comp. Physiol. A 135, 259–268. doi: 10.1007/BF00657254
Masters, W. M., Tautz, J., Fletcher, N. H., and Markl, H. (1983). Body vibration and sound production in an insect (Atta sexdens) without specialized radiating structures. J. Comp. Physiol. A 150, 239–249. doi: 10.1007/BF00606374
Miller, P. L. (1971). A note on stridulation in some cerambycid beetles and its possible relation to ventilation. J. Entomol. A 46, 63–68. doi: 10.1111/j.1365-3032.1971.tb00108.x
Mini, A., and Prabhu, V. K. K. (1990). Stridulation in the coconut rhinoceros beetle Oryctes rhinoceros (Coleoptera: Scarabaeidae). Proc. Anim. Sci. 99, 447–455. doi: 10.1007/BF03186407
Misof, B., Liu, S., Meusemann, K., Peters, R. S., Donath, A., Mayer, C., et al. (2014). Phylogenomics resolves the timing and pattern of insect evolution. Science 346, 763–767. doi: 10.1017/CBO9781107415324.004
Miyamoto, S. (1953). Biology of Microvelia diluta Distant, with descriptions of its brachypterous form and larval stages. Sieboldia 1, 113–133.
Møhl, B., and Miller, L. A. (1976). Ultrasonic clicks produced by the peacock butterfly: a possible bat-repellant mechanism. J. Exp. Biol. 64, 639–644.
Montealegre-Z, F., Jonsson, T., and Robert, D. (2011). Sound radiation and wing mechanics in stridulating field crickets (Orthoptera: Gryllidae). J. Exp. Biol. 214, 2105–2117. doi: 10.1242/jeb.056283
Morales, M. A., Barone, J. L., and Henry, C. S. (2008). Acoustic alarm signalling facilitates predator protection of treehoppers by mutualist ant bodyguards. Proc. R. Soc. B Biol. Sci. 275, 1935–1941. doi: 10.1098/rspb.2008.0410
Mukerji, D. (1929). Sound production by a larva of Cybister (Dytiscidae). J. Bombay Nat. Hist. Soc. 33, 653–655.
Nakahira, T., and Kudo, S. I. (2008). Maternal care in the burrower bug Adomerus triguttulus: defensive behavior. J. Insect Behav. 21, 306–316. doi: 10.1007/s10905-008-9129-0
Nakano, R., Takanashi, T., Surlykke, A., Skals, N., and Ishikawa, Y. (2013). Evolution of deceptive and true courtship songs in moths. Sci. Rep. 3:2003. doi: 10.1038/srep02003
Nelson, M. C. (1979). Sound production in the cockroach, Gromphadorhina portentosa: the sound-producing apparatus. J. Comp. Physiol. A 132, 27–38. doi: 10.1007/BF00617729
Nelson, M. C., and Fraser, J. (1980). Sound production in the cockroach, Gromphadorhina portentosa: evidence for communication hy hissing. Behav. Ecol. Sociobiol. 6, 305–314. doi: 10.1007/BF00292773
Olofsson, M., Jakobsson, S., and Wiklund, C. (2012). Auditory defence in the peacock butterfly (Inachis io) against mice (Apodemus flavicollis and A. sylvaticus). Behav. Ecol. Sociobiol. 66, 209–215. doi: 10.1007/s00265-011-1268-1
O’Reilly, L. J., Agassiz, D. J. L., Neil, T. R., and Holderied, M. W. (2019). Deaf moths employ acoustic Müllerian mimicry against bats using wingbeat-powered tymbals. Sci. Rep. 9:1444. doi: 10.1038/s41598-018-37812-z
Ossiannilsson, F. (1949). Insect drummers: a study on the morphology and function of the sound-producing organ of Swedish Homoptera Auchenorrhyncha with notes on their sound production. Opusc. Entomol. Suppl. 10, 1–146.
Palestrini, C., Pavan, G., and Zunino, M. (1990). Acoustic signals and stridulatory apparatus in Copris incertus Say (Coleoptera Scarabaeidae: Coprinae). Acta Zool. Mex. 39, 1–18.
Palestrini, C., Piazza, R., and Zunino, M. (1988). Segnali sonori in tre specie di Geotrupini (Coleoptera Scarabaeoidea Geotrupidae). Boll. Della Soc. Entomol. Ital. 119, 139–151.
Pavan, G., Palestrini, C., and Trevisan, E. (1990). Contribution to the knowledge of Thorectes intermedius (Costa) larval stridulation (Coleoptera: Scarabaeoidea: Geotrupidae). Elytron 4, 153–159.
Pavan, G., Priano, M., De Carli, P., Fanfani, A., and Giovannotti, M. (1997). Stridulatory organ and ultrasonic emission in certain species of ponerine ants (genus: Ectatomma and Pachycondyla, Hymenoptera, Formicidae). Bioacoustics 8, 209–221. doi: 10.1080/09524622.1997.9753363
Petak, I. (2019). “Ritualization,” in Encyclopedia of Animal Cognition and Behavior, eds J. Vonk and T. Shackelford (Cham: Springer International Publishing), 1–4. doi: 10.1007/978-3-319-47829-6_1888-1
Pierce, N. E., Braby, M. F., Heath, A., Lohman, D. J., Mathew, J., Rand, D. B., et al. (2002). The ecology and evolution of ant association in the Lycaenidae (Lepidoptera). Annu. Rev. Entomol. 47, 733–771. doi: 10.1146/annurev.ento.47.091201.145257
Pierce, N. E., Kitching, R. L., Buckley, R. C., Taylor, M. F. J., and Benbow, K. F. (1987). The costs and benefits of cooperation between the Australian lycaenid butterfly, Jalmenus evagoras, and its attendant ants. Behav. Ecol. Sociobiol. 21, 237–248. doi: 10.1007/BF00292505
Polidori, C., Ruffato, G., Borruso, L., Settanni, C., and Pavan, G. (2013). Stridulatory organ and distress call in males and females of a small velvet ant (Hymenoptera: Mutillidae). Bioacoustics 22, 121–135. doi: 10.1080/09524622.2012.736241
Ramaswamy, K., and Cocroft, R. B. (2009). Collective signals in treehopper broods provide predator localization cues to the defending mother. Anim. Behav. 78, 697–704. doi: 10.1016/j.anbehav.2009.06.017
Rashed, A., Khan, M. I., Dawson, J. W., Yack, J. E., and Sherratt, T. N. (2009). Do hoverflies (Diptera: Syrphidae) sound like the Hymenoptera they morphologically resemble? Behav. Ecol. 20, 396–402. doi: 10.1093/beheco/arn148
Ratcliffe, J. M., and Nydam, M. L. (2008). Multimodal warning signals for a multiple predator world. Nature 455, 96–99. doi: 10.1038/nature07087
Rentz, D. C. F. (1993). Tettigoniidae of Australia Vol. 2: The Austrosaginae, Zaprochilinae and Phasmodinae. Melbourne: CSIRO Publishing.
Robinson, M. H. (1969). The defensive behaviour of some orthopteroid insects from Panama. Trans. R. Entomol. Soc. Lond. 121, 281–303. doi: 10.1111/j.1365-2311.1969.tb00521.x
Röhrig, A., Kirchner, W. H., and Leuthold, R. H. (1999). Vibrational alarm communication in the African fungus-growing termite genus Macrotermes (Isoptera, Termitidae). Insectes Soc. 46, 71–77. doi: 10.1007/s000400050115
Römer, H. (2020). Insect acoustic communication: the role of transmission channel and the sensory system and brain of receivers. Funct. Ecol. 34, 310–321. doi: 10.1111/1365-2435.13321
Rönkä, K., De Pasqual, C., Mappes, J., Gordon, S., and Rojas, B. (2018). Colour alone matters: no predator generalization among morphs of an aposematic moth. Anim. Behav. 135, 153–163. doi: 10.1016/j.anbehav.2017.11.015
Rosi-Denadai, C. A., Scallion, M. L., Merrett, C. G., and Yack, J. E. (2018). Vocalization in caterpillars: a novel sound-producing mechanism for insects. J. Exp. Biol. 221:jeb169466. doi: 10.1242/jeb.169466
Roth, L. M., and Hartman, H. B. (1967). Sound production and its evolutionary significance in the Blattaria. Ann. Entomol. Soc. Am. 60, 740–752. doi: 10.1093/aesa/60.4.740
Rothschild, M., and Haskell, P. T. (1966). Stridulation of the garden tiger moth, Arctia caja L., audible to the human ear. Proc. R. Entomol. Soc. Lond. A 41, 167–170. doi: 10.1111/j.1365-3032.1966.tb00337.x
Rowe, C., and Halpin, C. G. (2013). Why are warning displays multimodal? Behav. Ecol. Sociobiol. 67, 1425–1439. doi: 10.1007/s00265-013-1515-8
Ruxton, G. D., Allen, W. L., Sherratt, T. N., and Speed, M. P. (2018). Avoiding Attack: The Evolutionary Ecology of Crypsis, Aposematism, and Mimicry, 2nd Edn. Oxford: Oxford University Press.
Ryker, L. C. (1976). Acoustic behavior of Tropisternus ellipticus, T. columbianus, and T. lateralis limbalis in Western Oregon (Coleoptera: Hydrophilidae). Coleopt. Bull. 30, 147–156.
Sales, G. D., and Pye, J. D. (1974). Ultrasonic Communication by Animals. New York, NY: John Wiley & Sons, Inc.
Sanborne, P. M. (1982). Stridulation in Merope tuber (Mecoptera: Meropeidae). Can. Entomol. 114, 177–180. doi: 10.4039/Ent114177-3
Sandow, J. D., and Bailey, W. J. (1978). An experimental study of defensive stridulation in Mygalopsis ferruginea Redtenbacher (Orthoptera: Tettigoniidae). Anim. Behav. 26, 1004–1011. doi: 10.1016/0003-3472(78)90089-1
Schal, C., Fraser, J., and Bell, W. J. (1982). Disturbance stridulation and chemical defence in nymphs of the tropical cockroach Megaloblatta blaberoides. J. Insect Physiol. 28, 541–552. doi: 10.1016/0022-1910(82)90035-X
Schilman, P. E., Lazzari, C. R., and Manrique, G. (2001). Comparison of disturbance stridulations in five species of Triatominae bugs. Acta Trop. 79, 171–178. doi: 10.1016/S0001-706X(01)00095-X
Schmitt, M., and Traue, D. (1990). Morphological and bioacoustic aspects of stridulation in Criocerinae (Coleoptera, Chrysomelidae). Zool. Anz. 225, 225–240.
Scott, J. L., Kawahara, A. Y., Skevington, J. H., Yen, S.-H., Sami, A., Smith, M. L., et al. (2010). The evolutionary origins of ritualized acoustic signals in caterpillars. Nat. Commun. 1:4. doi: 10.1038/ncomms1002
Seeley, T. D., Seeley, R. H., and Akratanakul, P. (1982). Colony defense strategies of the honeybees in Thailand. Ecol. Monogr. 52, 43–63. doi: 10.2307/2937344
Seelinger, G., and Seelinger, U. (1983). On the social organisation, alarm and fighting in the primitive cockroach Cryptocercus punctulatus Scudder. Z. Tierpsychol. 61, 315–333. doi: 10.1111/j.1439-0310.1983.tb01347.x
Sen Sarma, M., Fuchs, S., Werber, C., and Tautz, J. (2002). Worker piping triggers hissing for coordinated colony defence in the dwarf honeybee Apis florea. Zoology 105, 215–223. doi: 10.1078/0944-2006-00064
Serrano, A. R., Diogo, A. C., Viçoso, E., and Fonseca, P. J. (2003). New stridulatory structures in a tiger beetle (Coleoptera: Carabidae: Cicindelinae): morphology and sound characterization. Coleopt. Bull. 57, 161–166. doi: 10.1649/538
Simmons, R. B., and Conner, W. E. (1996). Ultrasonic signals in the defense and courtship of Euchaetes egle Drury and E. bolteri Stretch (Lepidoptera: Arctiidae). J. Insect Behav. 9, 909–919. doi: 10.1007/BF02208978
Skelhorn, J., Holmes, G. G., and Rowe, C. (2016). Deimatic or aposematic? Anim. Behav. 113, e1–e3. doi: 10.1016/j.anbehav.2015.07.021
Smith, R. L., and Langley, W. M. (1978). Cicada stress sound: an assay of its effectiveness as a predator defense mechanism. Southwest. Nat. 23, 187–195. doi: 10.2307/3669767
Smith, S. M., and Davis, E. S. (2008). Clicker increases resistance to extinction but does not decrease training time of a simple operant task in domestic dogs (Canis familiaris). Appl. Anim. Behav. Sci. 110, 318–329. doi: 10.1016/j.applanim.2007.04.012
Song, H., Béthoux, O., Shin, S., Donath, A., Letsch, H., Liu, S., et al. (2020). Phylogenomic analysis sheds light on the evolutionary pathways towards acoustic communication in Orthoptera. Nat. Commun. 11:4939. doi: 10.1038/s41467-020-18739-4
Speed, M. P. (2000). Warning signals, receiver psychology and predator memory. Anim. Behav. 60, 269–278. doi: 10.1006/anbe.2000.1430
Stölting, H., Moore, T. E., and Lakes-Harlan, R. (2004). Acoustic communication in Okanagana rimosa (Say) (Homoptera: Cicadidae). Zoology 107, 243–257. doi: 10.1016/j.zool.2004.07.003
Sugiura, S., and Takanashi, T. (2018). Hornworm counterattacks: defensive strikes and sound production in response to invertebrate attackers. Biol. J. Linn. Soc. 123, 496–505. doi: 10.1093/biolinnean/blx156
Umbers, K. D. L., De Bona, S., White, T. E., Lehtonen, J., Mappes, J., and Endler, J. A. (2017). Deimatism: a neglected component of antipredator defence. Biol. Lett. 13:20160936. doi: 10.1098/rsbl.2016.0936
Umbers, K. D. L., Lehtonen, J., and Mappes, J. (2015). Deimatic displays. Curr. Biol. 25, R58–R59. doi: 10.1016/j.cub.2014.11.011
Umbers, K. D. L., and Mappes, J. (2016). Towards a tractable working hypothesis for deimatic displays. Anim. Behav. 113, e5–e7. doi: 10.1016/j.anbehav.2016.01.002
Vallin, A., Jakobsson, S., Lind, J., and Wiklund, C. (2005). Prey survival by predator intimidation: an experimental study of peacock butterfly defence against blue tits. Proc. R. Soc. B 272, 1203–1207. doi: 10.1098/rspb.2004.3034
Vidal-García, M., O’Hanlon, J. C., Svenson, G. J., and Umbers, K. D. L. (2020). The evolution of startle displays: a case study in praying mantises. Proc. Biol. Sci. 287:20201016. doi: 10.1098/rspb.2020.1016
Virant-Doberlet, M., and Čokl, A. (2004). Vibrational communication in insects. Neotrop. Entomol. 33, 121–134. doi: 10.1590/S1519-566X2004000200001
Walters, E. T., Illich, P. A., Weeks, J. C., and Lewin, M. R. (2001). Defensive responses of larval Manduca sexta and their sensitization by noxious stimuli in the laboratory and field. J. Exp. Biol. 204, 457–469.
Ware, A. B. (1994). Factors eliciting stridulation by the ponerine ant Streblognathus aethiopicus Smith (Hymenoptera: Formicidae). Afr. Entomol. 2, 31–36.
Waters, D. A. (2003). Bats and moths: what is there left to learn? Physiol. Entomol. 28, 237–250. doi: 10.1111/j.1365-3032.2003.00355.x
Weissman, D. B. (2001). “North and central america jerusalem crickets (Orthoptera: Stenopelmatidae): taxonomy, distribution, life cycle, ecology and related biology of the American species,” in The Biology of Wetas, King Crickets and Their Allies, ed. L. H. Field (New York, NY: CABI Publishing), 57–110.
Weller, S. J., Jacobson, N. L., and Conner, W. E. (1999). The evolution of chemical defences and mating systems in tiger moths (Lepidoptera: Arctiidae). Biol. J. Linn. Soc. 68, 557–578. doi: 10.1111/j.1095-8312.1999.tb01188.x
Wessel, A., Mühlethaler, R., and Hartung, V. (2014). “The tymbal: evolution of a complex vibration-producing organ in the Tymbalia (Hemiptera excl. Sternorrhyncha),” in Studying Vibrational Communication, eds R. B. Cocroft, M. Gogala, P. S. Hill, and A. Wessel (New York, NY: Springer Berlin Heidelberg), 395–444. doi: 10.1007/978-3-662-43607-3
Wheeler, J. W., Chung, R. H., Oh, S. K., Benfield, E. F., and Neff, S. E. (1970). Defensive secretions of cychrine beetles (Coleoptera: Carabidae). Ann. Entomol. Soc. Am. 63, 469–471. doi: 10.1093/aesa/63.2.469
Wilson, L., Henry, C. S., Johnson, J., and McCaffrey, J. (1993). Sound production in Phrydiuchus tau (Coleoptera, Curculionidae). Ann. Entomol. Soc. Am. 86, 621–630. doi: 10.1093/aesa/86.5.621
Yack, J. E. (2016). “Vibrational signaling,” in Insect Hearing, eds G. S. Pollack, A. C. Mason, R. R. Fay, and A. N. Popper (Switzerland: Springer International Publishing), 99–124. doi: 10.1007/978-3-319-28890-1
Yack, J. E., Raven, B. H., Leveillee, M. B., and Naranjo, M. (2020). What does an insect hear? Reassessing the role of hearing in predator avoidance with insights from vertebrate prey. Integr. Comp. Biol. 60, 1036–1057. doi: 10.1093/icb/icaa097
Yager, D. D., and Spangler, H. G. (1997). Behavioral response to ultrasound by the tiger beetle Cicindela marutha Dow combines aerodynamic changes and sound production. J. Exp. Biol. 200, 649–659.
Yinon, U., Amitai, P., and Shulov, A. (1972). The stridulatory mechanism and the analysis of sound produced by the bug Holotrichius innesi (Horvath) (Heteroptera: Reduviidae). Comp. Biochem. Physiol. A 41, 373–381. doi: 10.1016/0300-9629(72)90068-0
Zagorinsky, A. A., Zhantiev, R. D., and Korsunovskaya, O. S. (2012). The sound signals of hawkmoths (Lepidoptera, Sphingidae). Entomol. Rev. 92, 601–604. doi: 10.1134/S0013873812060012
Zaspel, J. M., Weller, S. J., Wardwell, C. T., Zahiri, R., and Wahlberg, N. (2014). Phylogeny and evolution of pharmacophagy in tiger moths (Lepidoptera: Erebidae: Arctiinae). PLoS One 9:e101975. doi: 10.1371/journal.pone.0101975
Keywords: defense, acoustic, mechanism, signal variation, communication, predator, prey, disturbance
Citation: Low ML, Naranjo M and Yack JE (2021) Survival Sounds in Insects: Diversity, Function, and Evolution. Front. Ecol. Evol. 9:641740. doi: 10.3389/fevo.2021.641740
Received: 14 December 2020; Accepted: 04 February 2021;
Published: 08 March 2021.
Edited by:
Fernando Montealegre-Z, University of Lincoln, United KingdomReviewed by:
Reinhard Lakes-Harlan, University of Giessen, GermanyAaron Corcoran, University of Colorado Colorado Springs, United States
Copyright © 2021 Low, Naranjo and Yack. This is an open-access article distributed under the terms of the Creative Commons Attribution License (CC BY). The use, distribution or reproduction in other forums is permitted, provided the original author(s) and the copyright owner(s) are credited and that the original publication in this journal is cited, in accordance with accepted academic practice. No use, distribution or reproduction is permitted which does not comply with these terms.
*Correspondence: Jayne E. Yack, amF5bmV5YWNrQGN1bmV0LmNhcmxldG9uLmNh