- 1Groningen Institute for Evolutionary Life Sciences, University of Groningen, Groningen, Netherlands
- 2Department of Zoology, Stockholm University, Stockholm, Sweden
Adaptation to different environments can result in reproductive isolation between populations and the formation of new species. Food resources are among the most important environmental factors shaping local adaptation. The chemosensory system, the most ubiquitous sensory channel in the animal kingdom, not only detects food resources and their chemical composition, but also mediates sexual communication and reproductive isolation in many taxa. Chemosensory divergence may thus play a crucial role in resource-mediated adaptation and speciation. Understanding how the chemosensory system can facilitate resource-mediated ecological speciation requires integrating mechanistic studies of the chemosensory system with ecological studies, to link the genetics and physiology of chemosensory properties to divergent adaptation. In this review, we use examples of insect research to present seven key questions that can be used to understand how the chemosensory system can facilitate resource-mediated ecological speciation in consumer populations.
Introduction
Ecological speciation has been recognized as a major mechanism in generating biodiversity (Rundle and Nosil, 2005; Schluter, 2009; Nosil, 2012). Populations occurring in heterogeneous environments may experience divergent selection, resulting in reduced gene flow, which may eventually lead to the evolution of distinct species (Rundle and Nosil, 2005; Schluter, 2009; Nosil, 2012). Classical examples of ecological speciation in animals include the Darwin’s finches on the Galapagos islands, three-spined sticklebacks in limnetic or benthic habitats and host-plant races in herbivorous insects (reviewed in Schluter, 2009; Nosil, 2012). All animals rely on their sensory systems to sense and respond to their local environments. Among the different environmental factors, food resources are among the most important ones shaping local adaptation. The chemosensory system used to find food resources and avoid toxic substances are important targets of divergent selection (Smadja and Butlin, 2009; Hansson and Stensmyr, 2011; Stevens, 2013). Here, we present seven key questions that can be used to understand how the chemosensory system can facilitate resource-mediated ecological speciation in consumer populations (Figure 1). We specifically focus on insects, as they provide several model systems at the forefront of ecological speciation research and present striking evidence of speciation driven by host plant heterogeneity (Matsubayashi et al., 2010). In addition, the insect chemosensory system has been explored using state-of-the-art methods in the Drosophila genus for several decades, providing valuable examples on how insects adapt to their chemical environment.
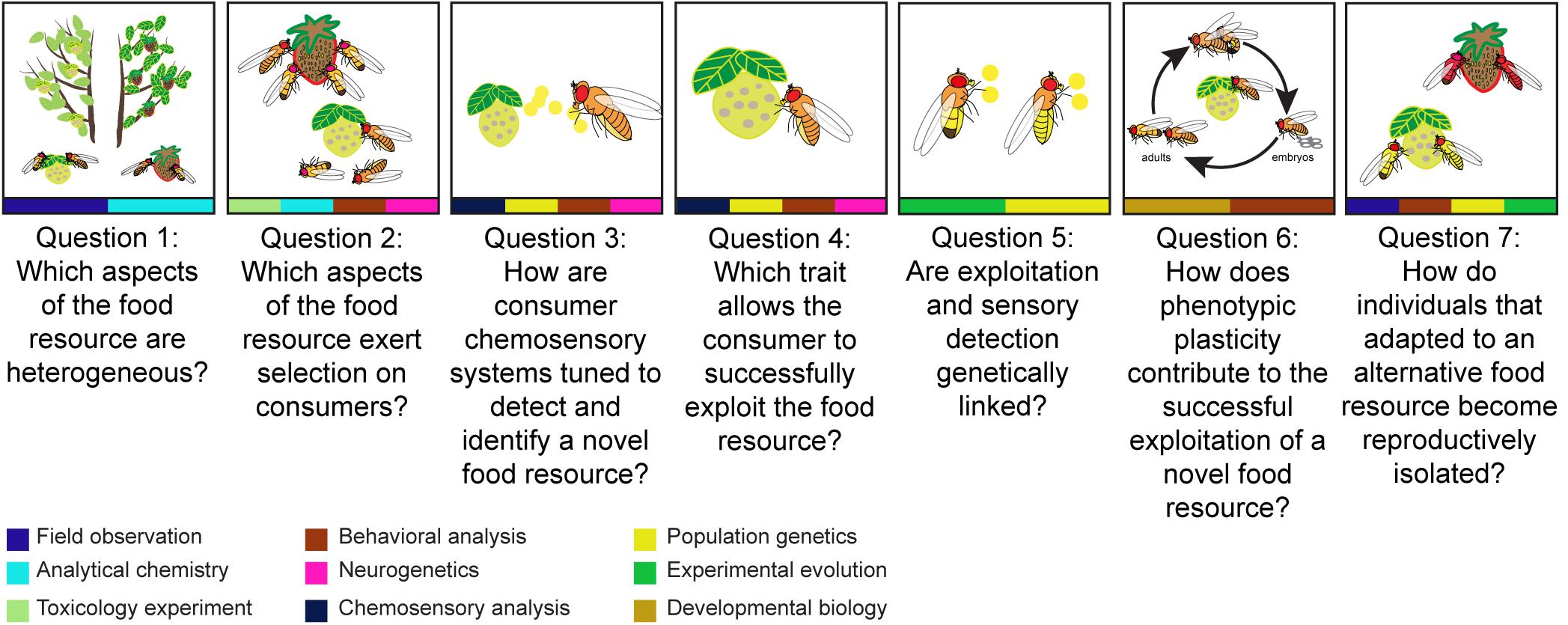
Figure 1. An overview of seven key questions that can be used to explore the processes leading up to resource-mediated ecological speciation, with methods at the bottom that can be used to study each question. To dissect resource-mediated ecological speciation, we first need to understand the different forms of food resource heterogeneity which could be variation in spatial location, temporal availability, and chemical composition of different food resources like the yellow and red fruits (Question 1). Then, it is important to identify which aspects of the food resources exert selection on consumers (Question 2). It could be because of the toxicity of the food resources. For example, flies which feed on yellow fruit but do not tolerate the associated toxins will die. To understand how consumers interact with food resources, it is necessary to explore how consumers detect (Question 3), exploit (Question 4), and adapt to heterogeneous resources (Question 5). Consumers can detect food resources by food odors (represented by yellow dots), exploit food resources by tolerating defensive compounds (represented by yellow proboscis) and adapt to food resources by the coupling of detection and digestion (represented by yellow odor dots and abdomen). Apart from genetic variation, phenotypic plasticity also modulates consumers’ exploitation of a resource (Question 6). Phenotypic plasticity determines whether successful exploitation can be learned and modified during an individual’s lifetime (represented by life cycles of flies). Finally, we come to the mechanisms preventing gene flow between individuals that specialized on alternative resources and those that did not (Question 7). When mating takes place at a specific food resource, while alternative resources are available, assortative mating can emerge as a by-product of divergence in foraging behavior. For example, the yellow flies are isolated from red flies. The approaches presented by the color at the bottom of each panel from left to right are: field observation (navy blue), analytical chemistry (light blue), toxicology experiment (light green), behavioral analysis (dark red), neurogenetics (pink), chemosensory analysis (dark blue), population genetics (yellow), experimental evolution (dark green), and developmental biology (ochre).
We start by identifying which aspects of food resources are heterogeneous in a given environment (Question 1), as this is the starting point of divergent selection. We then discuss which aspects of food exert selection on consumers (Question 2), and explore how consumers detect (Question 3), exploit (Question 4) and adapt to these resources (Question 5) to understand how local adaptation proceeds. Finally, we discuss whether successful exploitation of new food resources is genetically inherited or acquired during an individual’s lifetime (Question 6) and review the mechanisms that reduce gene flow between individuals that specialized on alternative resources (Question 7). Rather than providing a comprehensive review, we aim to show how answering these seven questions can explain chemosensory-mediated speciation, by integrating approaches traditionally employed by different scientific disciplines, such as chemical ecology, evolutionary biology, and neurobiology.
Question 1: Which Aspects of the Food Resource Are Heterogeneous?
In nature, several aspects of a food resource can be heterogeneous at the same time and induce divergent selection. For example, spatial and temporal variation in food availability may influence the chances of finding mates (Singer and Parmesan, 2010; Fei et al., 2014; Kambach et al., 2016), chemical composition may affect resource perception and feeding (Caillaud and Via, 2000; Webster and Cardé, 2017), and variation in nutritional quality may lead to changes in growth, survival, and reproduction (Wetzel et al., 2016; Moreau et al., 2017; Verschut and Hambäck, 2018). An example in which several forms of resource heterogeneity simultaneously act on consumers can be found in the desert fly, Drosophila mojavensis (Diptera: Drosophilidae) (Figure 2), which exploits spatially heterogeneous host cacti. These cacti occur in the Sonoran Desert of North America and are distributed along isolated sites including the Baja California Peninsula, the mainland Sonoran Desert, the Mojave Desert, and the Santa Catalina Island (Heed, 1978). They are susceptible to microbe-induced necroses, which serve as food sources for D. mojavensis (Fogleman and Danielson, 2001). Different necroses from these cacti occur at different times of the year and last for periods of variable duration (Breitmeyer and Markow, 1998), constituting a temporal heterogeneous food resource. An additional, but temporally overlooked form of resource heterogeneity, is microbe-induced heterogeneity. The microbes associated with cacti fermentate the carbohydrates in the cactus and produce specific volatile profiles which are important cues for host detection and exploitation (Becher et al., 2012; Date et al., 2013). These microbes also metabolize the cactus tissues and affect the cactus secondary metabolites which are mainly toxins including triterpene glycosides, medium chain fatty acids, alkaloids, and sterols diols, increasing or decreasing the toxicity of the cactus and thus changing the availability of the nutrients (Fogleman and Danielson, 2001). Together, these different sources of heterogeneity including spatial, temporal, and microbe-induced heterogeneity impose divergent selection on D. mojavensis, targeting the flies’ abilities to recognize the odors of its native host, locate the resource and tolerate its secondary metabolites.
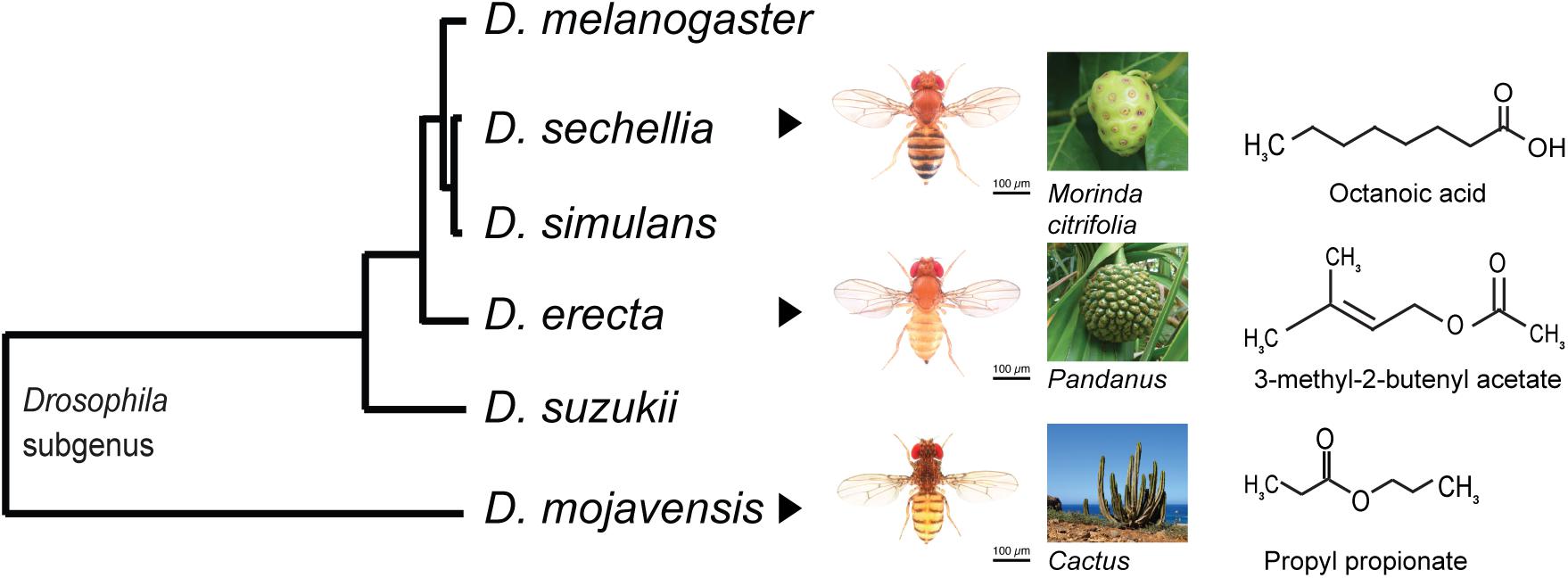
Figure 2. Phylogenetic tree of several Drosophila species with their hosts and the key host volatiles. Drosophila sechellia can tolerate the octanoic acid emitted by its host Morinda citrifolia. The seasonal specialist Drosophila erecta is attracted to its host Pandanus by the volatile 3-methyl-2-butenyl acetate. Drosophila mojavensis is attracted by the propyl propionate emitted from its host cactus. Drosophila images are from Nicolas Gompel; host images were taken from https://pixabay.com/.
Apart from natural variation, human-mediated resource manipulation has become a major driver of consumer adaptation by dramatically changing resource abundance and distribution. A classic example is the introduction and domestication of apple varieties in North America in the mid-1800s. As a result, the local apple maggot fly, Rhagoletis pomonella (Diptera: Tephritidae), which originally fed on hawthorn, started to feed on apples and rapidly spread through North America (Bush, 1969; Feder et al., 1994; Feder and Filchak, 1999). The subsequent introduction of sweeter and earlier ripening apples contributed to a larger diet breadth and longer developmental time for the flies, leading to the formation of a specialized apple race (Feder and Filchak, 1999; Filchak et al., 2000; Hood et al., 2020). An even more recent example is the spread of the spotted wing Drosophila, Drosophila suzukii (Diptera: Drosophilidae) on commercially grown soft fruits like cherries, blueberries, raspberries, and grapes. Because this species is attracted to ripening fruit odors and is equipped with an enlarged ovipositor for piercing ripening fruits (Keesey et al., 2015; Revadi et al., 2015; Karageorgi et al., 2017), it utilizes resources at a much earlier stage than other Drosophilids and rapidly invaded North America and Europe from Japan (Lee et al., 2011; Cloonan et al., 2018). Food and beverages for human consumption resulting from yeast fermentation like beer, wine and bread also offer novel food resources for many Drosophila species that naturally feed on yeast and enable them to expand their niches to breweries, wineries, and bakeries (Günther and Goddard, 2019). Consequently, feeding on human-mediated resources may cause consumer populations to become morphologically and genetically distinct from their ancestors, potentially leading to ecological speciation (Capy et al., 2000; Haerty et al., 2003).
Question 2: Which Aspects of the Food Resource Exert Selection on Consumers?
To understand how resource heterogeneity underlies divergent selection and speciation in consumer organisms, it is necessary to identify which aspect of the resource is actually exerting selection. This is not trivial, as several aspects of a resource may be heterogeneous at the same time, while adaptation may be primarily driven by one critical factor. For example, the phenology of introduced apple varieties is the trait that initially generated selection on the apple maggot fly to shift their developmental and diapause times (Bush, 1969; Feder and Filchak, 1999; Filchak et al., 2000). Subsequently, the apple race became allochronically isolated from the hawthorn race (Filchak et al., 2000). Among the aspects of the food resource exerting selection on consumers, the chemical composition is most obvious because different nutritional compounds or toxins require different adaptive responses in consumers. For example, because of differences in the chemical composition of pea aphid’s Acyrthosiphon pisum (Homoptera: Aphididae) host plants, clover and alfalfa, different host plant races of pea aphid reject their alternative host plant and mainly feed and mate on their native plants (Caillaud and Via, 2000; Campo et al., 2003). Similarly, differences in volatile profile and secondary metabolites between cactus species are responsible for host specialization and population differentiation among D. mojavensis populations (Fogleman and Danielson, 2001).
Toxins are a major driver of food specialization for many herbivorous and frugivorous insects as species that tolerate these toxins can escape competition (Karageorgi et al., 2019; Auer et al., 2020). For example, the noni fruit, Morinda citrifolia (L.) (Rubiaceae), produces hexanoic and octanoic acids that are toxic to most insects (Legal et al., 1994; Amlou et al., 1998). However, Drosophila sechellia (Diptera: Drosophilidae), a species endemic to the Seychelles islands, tolerates and even prefers it for feeding and oviposition (R’Kha et al., 1991; Legal et al., 1992; Matsuo et al., 2007; Figure 2). The precursor 3,4-dihydroxyphenylalanine (l-DOPA) in the noni fruit increases female fertility and egg volume in D. sechellia, facilitating the egg survival in the toxic environment, enhancing the fitness of adults and offspring to develop on noni fruit and helping D. sechellia escape competition with its co-occurring sibling species Drosophila simulans (Diptera: Drosophilidae) (Lavista-Llanos et al., 2014). Similarly, the monarch butterfly, Danaus plexippus (Lepidoptera: Nymphalidae) feeds on various glycoside-containing milkweeds of the Apocynaceae family that are toxic and repel other insect species. The larvae and adults of the monarch butterfly are physiologically insensitive to the glycoside that otherwise affect an insect’s cardiac physiology and functioning (Holzinger et al., 1992; Karageorgi et al., 2019). To study how different aspects of the food resource exert selection on consumer organisms, integration between toxicology, analytical chemistry, and neurogenetics is needed to identify the toxic effects (Legal et al., 1992), to find chemical cues influencing behavior (Legal et al., 1994), and to test the organisms’ behavioral, neurological, and physiological responses, for example by using sensory mutants (Auer et al., 2020).
Question 3: How Are Consumer Chemosensory Systems Tuned to Detect and Identify a Novel Food Resource?
The chemosensory system and its associated neurons generally form the first line of contact between consumers and their food resources (Dicke, 2000; Bruce et al., 2005; Missbach et al., 2014). The ability to detect novel stimuli is pivotal to accessing new resources and changing foraging habits, making the mechanism of resource localization a critical step in resource-mediated ecological speciation. In insects, the chemosensory receptors involved in these processes are distributed in three principal families that have distinct functions in resource detection: the gustatory receptors (GRs) for taste and contact cues (Montell, 2013; Scott, 2018), the odorant receptors (ORs) for olfaction (Vosshall et al., 1999; Liang and Luo, 2010; Joseph and Carlson, 2015), and the ionotropic receptors (IRs) for olfaction, taste, thermosensation, and hygrosensation (Benton, 2008; Silbering and Benton, 2010; Enjin et al., 2016; Rimal and Lee, 2018). Genes encoding classical olfactory receptors and their associated sensory neurons are subject to divergent selection in the Drosophila genus (Anholt, 2020). For example, the central African Drosophila erecta (Diptera: Drosophilidae) locates the fruits of Pandanus candelabrum (P. Beauv.) (Pandanaceae) via an increased number of olfactory sensory neurons that respond to 3-methyl-2-butenyl acetate (Rio et al., 1983; Linz et al., 2013; Figure 2). Wild African Drosophila melanogaster detects and uses its host marula fruit Sclerocarya birrea (A. Rich.) (Anacardiaceae) through the strong response of its Or22a-expressing neurons to the marula volatile ethyl isovalerate (Mansourian et al., 2018). Likewise, the preference of D. suzukii for ripening fruits correlates with the duplication of an OR gene and positive selection for ORs tuned to fresh fruit aromas (Revadi et al., 2015; Ramasamy et al., 2016). The leaf-mining fruit fly, Scaptomyza flava (Diptera: Drosophilidae), has receptors tuned to volatiles from Brassicaceae leaves rather than yeasts (Goldman-Huertas et al., 2015; Matsunaga et al., 2019). In the mosquito Aedes aegypti (Diptera: Culicidae), the transition from forest mammal to human hosts in urban centers coincided with an increased expression of its OR AegOr4, which detects the human body odorant sulcatone (McBride et al., 2014).
Of all Drosophila species, the mechanisms by which chemosensory genes contribute to resource adaptation have been especially well characterized for D. sechellia. The olfactory response of D. sechellia to noni fruits maps to one distinct glomerulus in the antennal lobes (AL) (Figure 3), the first olfactory processing center in the brain, receiving input from Or22a, specific to methyl esters (a noni fruit volatile) (Dekker et al., 2006; Auer et al., 2020). Sequence comparison and functional tests showed that the higher attraction of D. sechellia to methyl esters compared to that of D. simulans and D. melanogaster is determined by three amino acids in the N-terminus of Or22a, exemplifying that a molecular difference in Or22a orthologs can contribute to species–specific olfactory behaviors. Olfactory receptor genes are, however, not the only chemosensory genes contributing to adaptation of D. sechellia to noni fruits. A single amino acid change in the ionotropical receptor gene Ir75b is sufficient to provoke the attraction of D. sechellia to hexanoic acid (Prieto-Godino et al., 2017). Another change in the chemoreceptor repertoire of D. sechellia (compared to its sibling species) involves the odorant-binding proteins (OBPs) Obp57d and Obp57e (Matsuo et al., 2007). OBPs are located in the lymph of sensilla (Larter et al., 2016) and are hypothesized to bind and transfer odorants to the OSNs (Vogt et al., 1991; Leal, 2013; Pelosi et al., 2014; Figure 3). A mutation in the promoter region of D. sechellia’s Obp57e gene prevents its expression, removing taste avoidance toward hexanoic and octanoic acid and reducing repulsion toward noni fruit (Matsuo et al., 2007; Tomioka et al., 2012). When Obp57e and Obp57d were deleted in D. melanogaster, or when hybrids between the sibling species were produced, the behavioral repulsion to the acids also changed (Matsuo et al., 2007), showing that molecular differences in OBP orthologs can contribute to the species-specific olfactory behaviors underlying ecological differences.
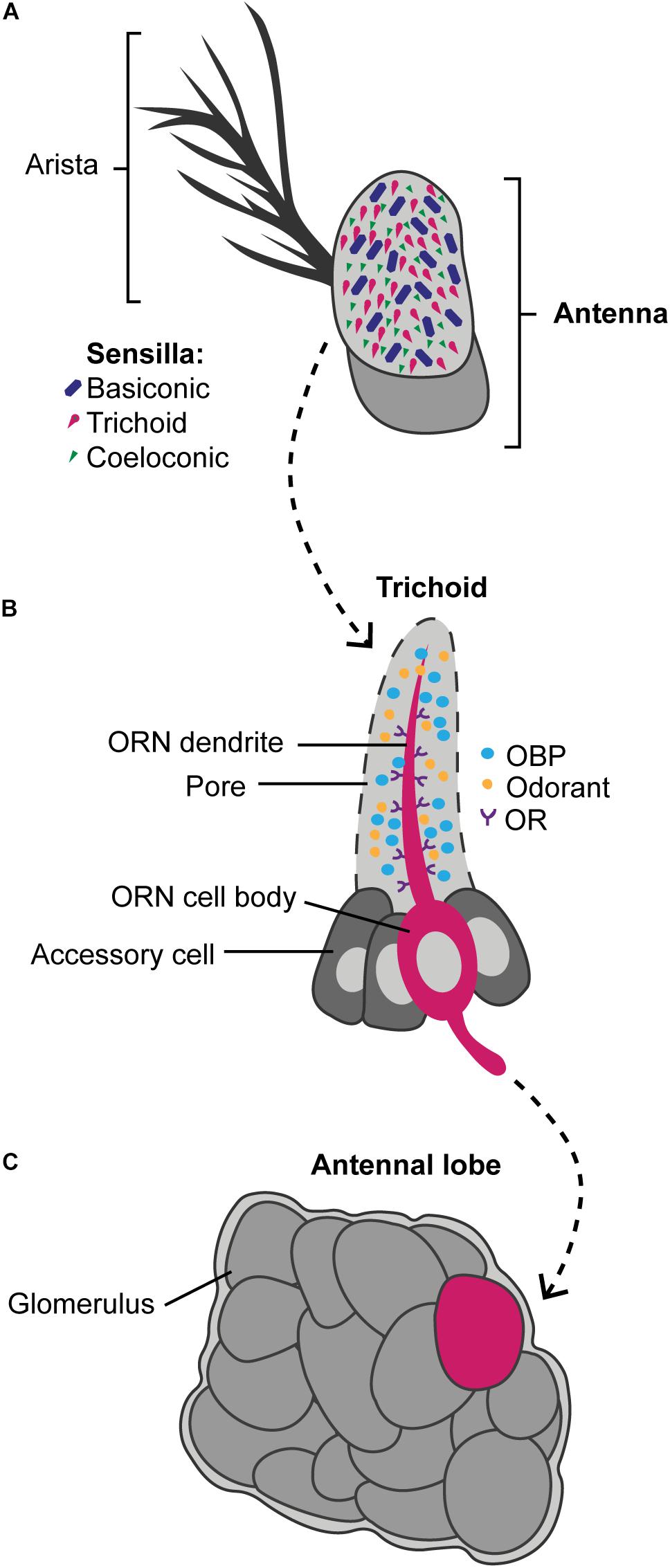
Figure 3. A simplified overview showing how odors are detected by the olfactory pathway. Odors are detected by hair-like sensilla on the antennae and the maxillary palps. Panel (A) exemplifies how the antennae of Drosophila are covered with the basiconic (blue), trichoid (magenta), and coeloconic (green) sensilla types. These sensilla contain olfactory receptor neurons (ORN) that express olfactory receptors (ORs) and ionotropic receptors (IRs). Panel (B) exemplifies a trichoid sensilla in which the dendrite of the ORN (magenta) is covered with ORs (purple). In Drosophila, the ORNs can express one to four olfactory receptors, but as a simplification only one is shown. The odorant binding proteins (OBP) (blue) are secreted by the accessory cells (dark gray) into the sensillar lymph and carry the odorants (orange) from the pores to the dendrites. Panel (C) shows a simplified antennal lobe, found in the insect brain, in which odors picked up by the ORN activate specific glomeruli (magenta) that it converges into. The glomeruli that were not activated by the odor are illustrated in dark gray.
Consistent with their role in mediating contact between animals and their environment, the genes encoding chemosensory proteins evolve fast (Arguello et al., 2016). Selection operates on standing variation at chemosensory loci. This variation may be particularly easy to maintain because individual chemosensory proteins rarely have large phenotypic effects. These chemosensory genes properties may facilitate rapid evolutionary change when confronted with a new ecological niche.
Question 4: Which Trait Allows the Consumer to Successfully Exploit the Food Resource
While the detection and identification of resources are important steps toward exploiting them, many food resources possess a wide range of toxic defenses or antifeedant secondary metabolites to protect their tissues (Kessler and Baldwin, 2001; Moraes et al., 2001; Ballaré, 2011; Mithöfer and Boland, 2012). The interaction between noni fruits and D. sechellia offers a good example of a consumer that not only has chemosensory adaptations to detect its resource but also possesses the ability to develop on the resource. Genetic studies identified a chromosomal region with strong effects on fatty acid octanoic acid (OA) tolerance (Jones, 1998), which mapped to a 170 kb region on the third chromosome, harboring several OBPs and Osiris genes (a family of putative transmembrane proteins of unknown function) (Hungate et al., 2013). To establish a causal relationship between the genes identified by Hungate et al. (2013) and OA tolerance, Andrade López et al. (2017) used RNAi in D. melanogaster to knock down each of these 18 genes and test the phenotypic effects. They observed that three genes in the Osiris family (Osi6, Osi7, and Osi8) significantly influenced OA resistance. They also found that Osi6 and Osi7 were expressed at significantly higher levels in D. sechellia than in intolerant sibling species, indicating that these two genes in particular affect OA resistance. Lanno et al. (2017) subsequently implicated Osi6, but also identified many other differentially expressed genes through RNA seq (104 genes in total). Thus, there is a complex genetic architecture to tolerance, which also involves genetic loci not involved in chemosensory perception.
Similar to D. sechellia, the leaf-mining fruit fly S. flava has a specialized olfactory system alongside a tolerance to compounds that are otherwise lethal to Drosophilids. This species stands out from other Drosophilids by ovipositing and developing on leaves of the Brassicaceae family (Goldman-Huertas et al., 2015; Matsunaga et al., 2019). Gloss et al. (2019) suggested that the shift from ancestral yeast feeding Drosophila to the herbivorous Scaptomyza is the result of higher turnover rates of the heme-binding cytochrome P450 monooxygenases (CYPs) enzymes that are involved in the detoxification of harmful environmental chemicals. For two Cyp genes, cyp6g1 and GstE5-8, there is an increase in the number of copies in the S. flava genome compared to its ancestors. Cyp6g1 is involved in the evolution of resistance in other insect species through cis-regularly mutations and gene duplication events (Goff and Hilliou, 2017), and GstE5-8 underlies the production of enzymes that detoxify mustard oils (isothiocyanates). As mustard oils are the main toxic compound in plants of the Brassicaceae family, the tolerance for these toxins may have allowed ancestral Scaptomyza species to evade competition for fruits and fungi with other Drosophila species by adapting to a novel leaf mining niche. Interestingly, another Cyp gene, Cyp6as, has been suggested to facilitate the shift from carnivory to florivory in florivorous bees from the Apidae family compared to carnivorous species from the Vespidae family, by allowing the metabolism of flavonols in nectar and honey (Johnson et al., 2018). Together, these patterns suggest that detoxification genes can play a crucial role in the successful exploitation of food resources.
Question 5: Are Exploitation and Sensory Detection Genetically Linked?
An organism can only successfully adapt to a novel food resource when the ability to digest the food coincides with the ability to detect it (or even the tendency to be attracted to it). This implies that the genetic architecture of these traits, and how tightly they are linked, strongly influence the likelihood and speed of resource-mediated divergence. Specifically, a shared genetic basis or a genetic correlation between detection and digestion would greatly facilitate niche expansion, and possibly lead to ecological speciation (Lande, 1979; Jaenike, 1990; Fry et al., 1996; Grosjean et al., 2011; Janz, 2011). As reviewed above, both attraction and tolerance have been extensively studied in D. sechellia. The introgression lines between D. sechellia and D. simulans that Hungate et al. (2013) used to map OA tolerance, were also used to explore genetic linkage: flies were subjected to behavioral tests to quantify both tolerance and preference. Contrary to expectation, genotypes that scored high on tolerance did not also express preference for noni fruit over control food. Thus, the genetic region that confers tolerance does not include loci influencing preference–in fact, other studies suggest that factors influencing preference are located on another chromosome (Yassin et al., 2016). A similar situation is observed in the cactophilic D. mojavensis, which has evolved metabolic pathways to detoxify the toxic compounds in cactus necroses. Different populations express different detoxification genes to utilize their own cactus hosts. For instance, Baja California flies express high levels of the detoxification gene Cyp6g1 for detoxifying Agria, while mainland Sonoran flies mostly depend on the Ugt86Dd gene for Organ pipe detoxification (Matzkin, 2012). The same goes for attraction preferences. All four geographically isolated D. mojavensis populations use volatiles emitted from fermenting cactus necroses to identify their host plant and differ in preferences and sensitivity to the volatiles of these four cactus species (Newby and Etges, 1998; Date et al., 2013; Crowley-Gall et al., 2016). For example, the Mojave population which inhabits Santa Catalina Island displays increased olfactory responses to aromatics (Date et al., 2013), the main volatile of its host plant, barrel cactus, and upregulates two chemosensory genes Or67b and Or71a to detect aromatics (Crowley-Gall et al., 2016). Yet, even though both gene families for attraction and detoxification are paramount in cactus host adaptation, there is no direct genetic linkage between attraction and detoxification (Matzkin et al., 2013; Matzkin, 2014; Allan and Matzkin, 2019).
The evidence presented above, albeit coming from a limited number of species, suggests that physical genetic linkage between resource-specific digestive abilities (including toxin tolerance) and sensory responses toward that same resource (i.e., detection and attraction) does not actually dominate in nature. Instead, the two traits tend to evolve sequentially. Successful exploitation of a new resource may start with a single evolutionary innovation (e.g., the ability to detect a particular chemical compound), which is only maintained if it is quickly followed by the second (e.g., the ability to tolerate an associated toxin). This raises the question as to what comes first in general, tolerance or preference? Island populations of Drosophila yakuba (Diptera: Drosophilidae), which recently specialized on M. citrifolia as well, carry the same genetic changes that underlie octanoic acid tolerance in D. sechellia–while their preference for the toxic fruit appears to be largely mediated by other loci (Yassin et al., 2016). These findings suggest that attraction preceded tolerance. This is because once one of the two innovations has evolved, the second one is expected to emerge very rapidly by strong selection on the existing genetic variation within the population. Given that a substantial proportion of genetic variation will be shared between the ancestral D. sechellia and the derived D. yakuba, this leads to the rapid fixation of the same tolerance-conferring alleles (Yassin et al., 2016). However, we need more data from other species to see how consistent this novel food adaptation pattern is, and whether genetic linkage between sensory detection and exploitation is a major determiner of speciation dynamics.
Question 6: How Does Phenotypic Plasticity Contribute to the Successful Exploitation of a Novel Food Resource?
The previous sections illustrate that adaptation to a food resource relies on a chemosensory system that is tuned to locate the resource and on detoxifying enzymes that neutralize toxic compounds (Scriber and Slansky, 1981; Gloss et al., 2016; Webster and Cardé, 2017). The important role of genetically encoded proteins that directly interact with the food resource suggests that these traits may be hard wired during early development. Evolution thus proceeds through classical selection of alleles that confer adaptation. However, some degree of plasticity may allow consumers to adjust their responses through experience. Neural plasticity occurs throughout the olfactory pathway, from olfactory receptor neurons to higher-order neurons, undergoing experience-based changes in synaptic efficacy to neurogenesis and apoptosis (Wilson et al., 2004; Gadenne et al., 2016). This plasticity might promote niche expansion, exposing organisms to new selective pressures that actually favor specialization. On the other hand, olfactory plasticity may interfere with the evolution of resource specialization: if plasticity allows successful detection and exploitation of a (novel) resource, then there is no selective advantage for alleles conferring certain specialist abilities or for assortative mating (i.e., individuals mating more often with individuals possessing similar phenotypes). Understanding how preference for certain resources and the ability to exploit it develop, is thus critical to understanding how divergent resource specialization evolves.
In the example of D. sechellia, chemosensory genes are obviously important for the attraction to noni fruit, but they do not solely account for the behavioral differences with its sibling species D. simulans. First, while D. sechellia develops twice as many Or22a-expressing neurons during pupal development compared to its sibling species, this higher number of neurons is not sufficient to explain host attraction. Auer et al. (2020) showed that substituting D. sechellia’s Or22a receptors with those from D. melanogaster, while maintaining their higher number of neurons, does not result in the same level of attraction to noni fruit as wild-type flies. Second, additional differences are found in D. sechellia’s lateral horn, a higher-order brain region that processes innate responses to olfactory cues (Jefferis et al., 2007), in which projection neurons connecting to Or22a-expressing neurons have a prominent branch innervating an area that is not targeted by the homologous neurons in its sibling species (Auer et al., 2020). These differences in projection neuron axon innervations suggest that changes in central-circuit connectivity also allowed for the adaptation to noni fruit. These examples illustrate that although chemosensory gene families evolve fast, additional traits shaping detoxifying enzymes, central-circuit connectivity and projection neuron innervations also account for the evolution of resource detection.
For many insects, resource detection and exploitation are subject to behavioral plasticity resulting from feeding and mating. Hunger generally increases sensitivity toward food odors and decreases sexual receptivity in many insect species (Edgecomb et al., 1994; Lightle et al., 2010; Luo et al., 2013; Ko et al., 2015; Lebreton et al., 2017). In fruit flies, this is controlled through the release of insulin, which leads to sensitization of OR neurons tuned to juvenile food sources like yeasts (Ribeiro and Dickson, 2010; Root et al., 2011). The increased sensitivity to yeast may, in turn, increase the sexual receptivity of these mated females, leading them to remate at the juvenile food resources (Gorter et al., 2016). In addition, feeding status and food-derived odors influence female responses to male pheromones (Lebreton et al., 2015; Das et al., 2017). Thus, food-induced behavioral changes may indirectly affect mating opportunities. Many species also show behavioral plasticity after mating. The so-called “post-mating switch” lowers responses to pheromones, while increasing responses to host odors or oviposition sites (Anderson and Anton, 2014; Gadenne et al., 2016). For example, mated female fruit flies show a strong preference for yeast (Ribeiro and Dickson, 2010) and need to locate yeast that allows for the development of their offspring (Becher et al., 2012). Hussain et al. (2016) showed that mated females show an increased expression in the olfactory system of a receptor of myoinhibitory peptides, resulting in a higher sensitivity to the smell of polyamines, which are indicative of substrates that enhance larval development. Both sexes of the cotton leafworm Spodoptera littoralis (Lepidoptera: Noctuidae) also exhibit a post-mating response to sex pheromones and host odors. In males, the post-mating switch results in reduced responses to sex pheromones and host leaf odors signaling mating sites, but not diminished attraction to odors signaling adult food (Kromann et al., 2015). In females, mating abolishes attraction to lilac flowers Syringa vulgaris (L.) (Oleaceae), but causes attraction to green-leaf odor of the larval host plant cotton, Gossypium hirsutum (L.) (Malvaceae) (Saveer et al., 2012). Thus, behavioral plasticity enables consumers to interact with food resources in a way that matches their physiological states and needs.
Question 7: How Do Individuals That Adapted to an Alternative Food Resource Become Reproductively Isolated?
A critical step in any ecological speciation scenario is the evolution of some level of reproductive isolation, which can be mediated by various evolutionary mechanisms (Kirkpatrick and Ravigné, 2002). In some of these scenarios divergent adaptation immediately causes reproductive isolation as a by-product. More specifically, when mating takes place at a specific resource, while alternative resources are available, assortative mating can emerge as a by-product of divergence in foraging behavior. This is one of the most straightforward routes to reproductive isolation, as it does not require the evolution of additional changes in traits involved in mate selection (Kirkpatrick and Ravigné, 2002; Servedio and Boughman, 2017; Hood et al., 2020). This mechanism contributed to the origin of the apple maggot fly, where colonization of apple trees reduced gene flow through spatiotemporal segregation from the ancestral population (Bush, 1969; Dambroski et al., 2005; Hood et al., 2020). Similar spatial or temporal segregation occurs in many other species, including several of the examples mentioned in the sections above, making it one of the major drivers of species diversity in insects (Matsubayashi et al., 2010). Establishing the contribution of spatiotemporal isolation to speciation requires quantifying the degree of overlap in resource distribution, as well as behavioral studies into the dispersal tendencies and abilities of the consumer organism.
Answers to many of the questions outlined in this review exemplify that divergent adaptation to alternative resources often involves changes in chemosensory perception. Given the importance of chemical signals in sexual communication, such changes may also affect mating probabilities between individuals exploiting different resources. This can constitute another fairly direct route to reproductive isolation, but it requires alignment between the sensory adaptations involved in resource detection, identification and processing, together with adaptations in sexual communication. For several insect species, such alignment occurs when dietary compounds influence the cuticular hydrocarbons (CHC) involved in mate recognition (Etges et al., 2006; Smadja and Butlin, 2009; Sharon et al., 2010; Fedina et al., 2012). When these changes in CHC profiles coincide with changes in mate selection, resource-based assortative mating can evolve, as seen for the cactophilic D. mojavensis (Etges, 1992; Brazner and Etges, 1993; Khallaf et al., 2020). Additionally, gut microbiota obtained by fruit flies through feeding can strongly affect their mate choice, as they select individuals harboring specific microbiota (Sharon et al., 2010, 2011; Brucker and Bordenstein, 2012; Engel and Moran, 2013; Shropshire and Bordenstein, 2016). Consequently, selection for microbiota can be expected to lead to the divergence of host lineages that feed on different substrates, ultimately leading to speciation. It is important to note, however, that these changes are often phenotypically plastic, which modulates their impact on reproductive isolation and species divergence (Otte et al., 2018).
Another caveat concerns the association between signal divergence and mate selection, as changes in chemical signals must align with changes in mating preferences in order to generate reproductive isolation. This is easily achieved when mating depends on the similarity of chemical signals [i.e., “self-referent phenotype matching” (Weddle et al., 2013)], but when mate selection targets specific trait values, assortative mating requires that preferences change in accordance with the chemical signals. This is not always the case, and several examples of chemical mismatches have been documented in Drosophila (e.g., Kwan and Rundle, 2010; Xue et al., 2018). Even without changes in chemical signals themselves, the combination with different food odors might modulate sexual communication. As discussed above, female responses to male pheromones can be affected by food-derived odors (e.g., Das et al., 2017). To establish whether such effects could impact the extent of assortative mating, future research should explore whether synergistic effects of food-derived and fly-derived compounds are resource-specific.
In the absence of direct effects of divergent adaptation on sexual communication, assortative mating may evolve as a consequence of selection for local adaptation. A special case concerns “reinforcement,” where assortative mating between individuals with the same adaptations evolves to avoid the production of maladapted hybrids (Craig et al., 1997; Caillaud and Via, 2000). In addition to reducing net gene flow, this generates selection for mechanisms that increase the probability of assortative mating, as observed for several Drosophilids (Yukilevich, 2012). For example, pre-mating isolation in several Drosophila sibling species is based on male responses to female CHC profiles (Coyne et al., 1994; Billeter et al., 2009; Seeholzer et al., 2018; Shahandeh et al., 2018). Based on quantitative trait locus (QTL) studies dissecting female CHC profiles (Gleason et al., 2005, 2009) and male courtship preferences (Shahandeh et al., 2018; Shahandeh and Turner, 2020), the most parsimonious scenario is that female CHC profiles diverged due to differences in microclimatic conditions, followed by divergence in male courtship preferences to avoid costly interspecific courtship and mating (Rundle and Dyer, 2015; Shahandeh and Turner, 2020).
Adaptation-assortative mating may also result from mating preferences for traits reflecting the adaptation of prospective mates to the local circumstances. In the most straightforward case, individuals prefer to mate with partners in good physical condition–thereby selecting those individuals that are best adapted. Somewhat more complex is the evolution of novel mate preferences, targeting traits that specifically reflect local performance. Sexual preferences for mates in good conditions is a well-documented phenomenon in behavioral ecology (Davies et al., 2012). In insects, chemical signals play a role in mate choice for unrelated or older mates (Kuo et al., 2012), but in the context of host race formation evidence for the contribution of sexual selection for local adaptation during divergence is scarce. Rundle et al. (2009) observed that experimental selection of Drosophila serrata (Diptera: Drosophilidae) on different food resources generated changes in both CHC profiles and CHC-based mating preferences, but failed to accelerate divergent adaptation through sexual selection. Currently, it seems unclear whether chemically mediated preference for locally adapted mating partners is an important contributor to assortative mating during resource-driven speciation in insects (Rundle et al., 2009).
Discussion
In this review, we presented seven questions to explore the processes leading up to resource-mediated ecological speciation, with a particular focus on chemosensory interactions in insects. For each of the seven questions, major insights have been generated using various taxa and approaches, documenting the critical resource properties that drive chemosensory adaptations in consumers, the molecular basis of these adaptations and the characterization of the behavioral responses that contribute to reproductive isolation. Our analysis also revealed important gaps in our knowledge. Notably, different approaches are needed for different questions: we need field ecology and chemical analyses to characterize environmental heterogeneity and its effects on consumer populations, neurobiology and physiology to examine how individuals detect and exploit food resources, developmental biology and genetics to assess the molecular basis of these traits, behavioral observation to document mechanisms underlying reproductive isolation, and evolutionary biology to link selective regimes to evolutionary change. There is rarely one model system in which all seven questions have been thoroughly answered; different organisms are generally employed to answer different questions. For example, D. mojavensis is used to explore resource heterogeneity and population differentiation, while laboratory strains of D. melanogaster are used to study receptors, olfactory pathways, neutral circuits, and genes involved in resource detection and exploitation. Niche specialists such as D. sechellia and D. mojavensis are used to examine host specialization and its underlying molecular determinants, while pea aphids and apple maggot flies are used to study the evolutionary ecology of incipient sympatric speciation. In order to extrapolate and generalize, it will be important to incorporate insights from one model system into studies of others. Certainly, all “model organism” and methods have their limitations. Drosophila has proven to be a powerful model in neurogenetics and evolutionary genetics because of its unique genetic tools (Bellen et al., 2010; Kazama, 2015; Castillo and Barbash, 2017). These tools however require the use of genetically homogenous or inbred strains so that the control and experimental groups only differ at the level of the mechanism studied, limiting “noise” coming from individual genetic variation. This is essential for data interpretation. However, these inbred Drosophila laboratory strains do not represent the diversity and complexity that ecologists aim to explain (Zuk et al., 2014) because ecological speciation relies on the existence of natural genetic variation between individuals, which can then be selected from. It is therefore important to combine the mechanistic insights acquired from the laboratory inbred strains with the study of the natural variants found in nature (Alfred and Baldwin, 2015).
One major knowledge gap concerns the nature of the heterogeneity that exerts divergent selection (Question 1). Environments are heterogeneous in many ways, but it is often not clear what kind of heterogeneity, or which aspect of a particular resource, will generate divergence in consumers. This restricts our predictive ability and hampers our understanding of the relative importance of intrinsic and extrinsic factors in speciation. As noted above, chemosensory genes evolve relatively fast, and this may be true for other sensory genes as well (Stevens, 2013). This may be explained by their specific genomic architectures and functioning, leading to high levels of redundancy and little evolutionary constraint. We may speculate that it is because of these properties that ecological speciation often involves divergence in sensory traits, which would suggest a major role for intrinsic organismal factors in explaining patterns of speciation. Alternatively, it is primarily the environment that governs the likelihood of speciation, and the nature and extent of environmental heterogeneity that determine the strength of divergent selection. Of course, both are important, but a major challenge for speciation research is to establish whether there are general rules across taxa in the contributions of specific intrinsic and extrinsic factors to explaining variation in speciation propensity.
Obviously, additional questions can be formulated. For instance, while we provided several examples of how the adaptation to a food resource relies on a chemosensory system that is tuned to specific substances, the incapacity to detect compounds that are repellent to closely related species may also be coupled to niche specialization (Bernays, 2001). It is also important to explore what happens after speciation has been initiated and some level of reproductive isolation has been achieved. The long-term persistence of new species will depend on the maintenance of environmental heterogeneity, the geographic setting, the dimension of selection and the genetic changes involved in divergence. Generally, in plant-insect interactions, the diversity and abundance of plants directly affect speciation and extinction in associated insect taxa (Nyman et al., 2012). For instance, the collapse of the host plants drives the extinction of specialized insect species (Wagner and Driesche, 2010). When host plants are segregated in space, persistence of two new herbivore species will be easily maintained because of the absence of gene flow (Nosil, 2012). In line with this, phylogenetic analyses reveal that geographic isolation is more important than host plant heterogeneity in maintaining new species (sawflies: Nyman et al., 2010; aphids: Jousselin et al., 2013). In addition to environmental heterogeneity itself, its dimensionality may affect the maintenance of incipient species. Divergence in multiple niche dimensions may not only promote diversification but also the maintenance of differentiation (Nosil, 2012). Finally, the genetic changes involved in divergence may influence the long-term persistence of incipient species. For example, the evolution of reproductive incompatibilities may take a long time (Coyne and Orr, 2004) but once present, greatly facilitates species persistence. It is still an open question whether the factors that promote speciation are also involved in maintaining diversity (Butlin et al., 2012).
One potentially important avenue for future research concerns the role of the consumer gut microbes in resource-mediated speciation. In addition to the microbes present in the substrate, consumer gut microbes also play a role in resource-mediated speciation by mediating resource exploitation ability (Brucker and Bordenstein, 2012; Shropshire and Bordenstein, 2016). For example, the elimination of an endosymbiont in natural Japanese populations of pea aphid Acyrthosiphon pisum significantly reduced populations’ exploitation of white clover Trifolium repens (L.) (Fabaceae) (Tsuchida et al., 2004). Gut microbiota may also influence mate choice of hosts. For example, D. melanogaster that were reared on different media acquired different microbiotas, which in turn influenced their cuticular hydrocarbon compositions and led to assortative mating among the flies reared on the same medium (Sharon et al., 2011; Heys et al., 2018). Therefore, microbes in the gut could be a promising direction for future research on resource-mediated ecological speciation.
Theoretical and methodological advances in both speciation biology and chemical ecology make today an exciting time for multidisciplinary speciation research. Speciation biologists have a wealth of methodological tools at their disposal that are increasingly applicable to other taxa beyond the traditional laboratory model organisms, making more taxa available for future comparative studies. We hope that these tools, together with a multidisciplinary perspective and the seven-question framework presented here, will contribute to a more integrated and complete understanding of resource-mediated speciation.
Author Contributions
All authors discussed, wrote, and revised the manuscript.
Funding
This research was supported by a scholarship of the China Scholarship Council (CSC) and the University of Groningen (awarded to XW) and grant VR-2018-00354 (awarded to TV) from the Swedish Research Council Vetenskapsrådet.
Conflict of Interest
The authors declare that the research was conducted in the absence of any commercial or financial relationships that could be construed as a potential conflict of interest.
Acknowledgments
We thank Philip Kohlmeier and Daniel Shane Wright for insightful comments on earlier versions of the manuscript.
References
Alfred, J., and Baldwin, I. T. (2015). The natural history of model organisms: new opportunities at the wild frontier. eLife 4:e06956. doi: 10.7554/eLife.06956
Allan, C. W., and Matzkin, L. M. (2019). Genomic analysis of the four ecologically distinct cactus host populations of Drosophila mojavensis. BMC Genomics 20:732. doi: 10.1186/s12864-019-6097-z
Amlou, M., Moreteau, B., and David, J. R. (1998). Larval tolerance in the Drosophila melanogaster species complex toward the two toxic acids of the D. Sechellia host plant. Hereditas 129, 7–14. doi: 10.1111/j.1601-5223.1998.00007.x
Anderson, P., and Anton, S. (2014). Experience-based modulation of behavioural responses to plant volatiles and other sensory cues in insect herbivores. Plant Cell Environ. 37, 1826–1835. doi: 10.1111/pce.12342
Andrade López, J. M., Lanno, S. M., Auerbach, J. M., Moskowitz, E. C., Sligar, L. A., Wittkopp, P. J., et al. (2017). Genetic basis of octanoic acid resistance in Drosophila sechellia: functional analysis of a fine-mapped region. Mol. Ecol. 26, 1148–1160. doi: 10.1111/mec.14001
Anholt, R. R. H. (2020). Chemosensation and evolution of Drosophila host plant selection. iScience 23:100799. doi: 10.1016/j.isci.2019.100799
Arguello, J. R., Cardoso-Moreira, M., Grenier, J. K., Gottipati, S., Clark, A. G., and Benton, R. (2016). Extensive local adaptation within the chemosensory system following Drosophila melanogaster’s global expansion. Nat. Commun. 7:ncomms11855. doi: 10.1038/ncomms11855
Auer, T. O., Khallaf, M. A., Silbering, A. F., Zappia, G., Ellis, K., Álvarez-Ocaña, R., et al. (2020). Olfactory receptor and circuit evolution promote host specialization. Nature 579, 402–408. doi: 10.1038/s41586-020-2073-7
Ballaré, C. L. (2011). Jasmonate-induced defenses. A tale of intelligence, collaborators and rascals. Trends Plant Sci. 16, 249–257. doi: 10.1016/j.tplants.2010.12.001
Becher, P. G., Flick, G., Rozpędowska, E., Schmidt, A., Hagman, A., Lebreton, S., et al. (2012). Yeast, not fruit volatiles mediate Drosophila melanogaster attraction, oviposition and development. Funct. Ecol. 26, 822–828. doi: 10.1111/j.1365-2435.2012.02006.x
Bellen, H. J., Tong, C., and Tsuda, H. (2010). 100 years of Drosophila research and its impact on vertebrate neuroscience: a history lesson for the future. Nat. Rev. Neurosci. 11, 514–522. doi: 10.1038/nrn2839
Benton, R. (2008). Chemical sensing in Drosophila. Curr. Opin. Neurobiol. 18, 357–363. doi: 10.1016/j.conb.2008.08.012
Bernays, E. A. (2001). Neural limitations in phytophagous insects: implications for diet breadth and evolution of host affiliation. Annu. Rev. Entomol. 46, 703–727. doi: 10.1146/annurev.ento.46.1.703
Billeter, J.-C., Atallah, J., Krupp, J. J., Millar, J. G., and Levine, J. D. (2009). Specialized cells tag sexual and species identity in Drosophila melanogaster. Nature 461, 987–991. doi: 10.1038/nature08495
Brazner, J. C., and Etges, W. J. (1993). Pre-mating isolation is determined by larval rearing substrates in cactophilic Drosophila mojavensis. II. Effects of larval substrates on time to copulation, mate choice and mating propensity. Evol. Ecol. 7, 605–624. doi: 10.1007/BF01237824
Breitmeyer, C. M., and Markow, T. A. (1998). Resource availability and population size in cactophilic Drosophila. Funct. Ecol. 12, 14–21. doi: 10.1046/j.1365-2435.1998.00152.x
Bruce, T. J. A., Wadhams, L. J., and Woodcock, C. M. (2005). Insect host location: a volatile situation. Trends Plant Sci. 10, 269–274. doi: 10.1016/j.tplants.2005.04.003
Brucker, R. M., and Bordenstein, S. R. (2012). Speciation by symbiosis. Trends Ecol. Evol. 27, 443–451. doi: 10.1016/j.tree.2012.03.011
Bush, G. L. (1969). Sympatric host race formation and speciation in frugivorous flies of the genus Rhagoletis (Diptera, Tephritidae). Evolution 23, 237–251. doi: 10.2307/2406788
Butlin, R., Debelle, A., Kerth, C., Snook, R. R., Beukeboom, L. W., Castillo Cajas, R. F., et al. (2012). What do we need to know about speciation? Trends Ecol. Evol. 27, 27–39. doi: 10.1016/j.tree.2011.09.002
Caillaud, M. C., and Via, S. (2000). Specialized feeding behavior influences both ecological specialization and assortative mating in sympatric host races of pea aphids. Am. Nat. 156, 606–621. doi: 10.1086/316991
Campo, M. L. D., Via, S., and Caillaud, M. C. (2003). Recognition of host-specific chemical stimulants in two sympatric host races of the pea aphid Acyrthosiphon pisum. Ecol. Entomol. 28, 405–412. doi: 10.1046/j.1365-2311.2003.00524.x
Capy, P., Veuille, M., Paillette, M., Jallon, J.-M., Vouidibio, J., and David, J. R. (2000). Sexual isolation of genetically differentiated sympatric populations of Drosophila melanogaster in Brazzaville, Congo. The first step towards speciation? Heredity 84, 468–475. doi: 10.1046/j.1365-2540.2000.00711.x
Castillo, D. M., and Barbash, D. A. (2017). Moving speciation genetics forward. modern techniques build on foundational studies in Drosophila. Genetics 207, 825–842. doi: 10.1534/genetics.116.187120
Cloonan, K. R., Abraham, J., Angeli, S., Syed, Z., and Rodriguez-Saona, C. (2018). Advances in the chemical ecology of the spotted wing Drosophila (Drosophila suzukii) and its applications. J. Chem. Ecol. 44, 922–939. doi: 10.1007/s10886-018-1000-y
Coyne, J. A., Crittenden, A. P., and Maht, K. (1994). Genetics of a pheromonal difference contributing to reproductive isolation in Drosophila. Science 265, 1461–1464. doi: 10.1126/science.8073292
Craig, T. P., Horner, J. D., and Itami, J. K. (1997). Hybridization studies on the host races of Eurosta solidaginis: implications for sympatric speciation. Evolution 51, 1552–1560. doi: 10.1111/j.1558-5646.1997.tb01478.x
Crowley-Gall, A., Date, P., Han, C., Rhodes, N., Andolfatto, P., Layne, J. E., et al. (2016). Population differences in olfaction accompany host shift in Drosophila mojavensis. Proc. R. Soc. B 283:20161562. doi: 10.1098/rspb.2016.1562
Dambroski, H. R., Linn, C. Jr., Berlocher, S. H., Forbes, A. A., Roelofs, W., and Feder, J. L. (2005). The genetic basis for fruit odor discrimination in Rhagoletis flies and its significance for sympatric host shifts. Evolution 59, 1953–1964. doi: 10.1111/j.0014-3820.2005.tb01065.x
Das, S., Trona, F., Khallaf, M. A., Schuh, E., Knaden, M., Hansson, B. S., et al. (2017). Electrical synapses mediate synergism between pheromone and food odors in Drosophila melanogaster. Proc. Natl. Acad. Sci. U.S.A. 114, E9962–E9971. doi: 10.1073/pnas.1712706114
Date, P., Dweck, H. K. M., Stensmyr, M. C., Shann, J., Hansson, B. S., and Rollmann, S. M. (2013). Divergence in olfactory host plant preference in D. mojavensis in response to cactus host use. PLoS One 8:e70027. doi: 10.1371/journal.pone.0070027
Davies, N. B., Krebs, J. R., and West, S. A. (2012). An Introduction to Behavioural Ecology. Hoboken, NJ: John Wiley & Sons.
Dekker, T., Ibba, I., Siju, K. P., Stensmyr, M. C., and Hansson, B. S. (2006). Olfactory shifts parallel superspecialism for toxic fruit in Drosophila melanogaster sibling, D. sechellia. Curr. Biol. 16, 101–109. doi: 10.1016/j.cub.2005.11.075
Dicke, M. (2000). Chemical ecology of host-plant selection by herbivorous arthropods: a multitrophic perspective. Biochem. Syst. Ecol. 28, 601–617. doi: 10.1016/s0305-1978(99)00106-4
Edgecomb, R. S., Harth, C. E., and Schneiderman, A. M. (1994). Regulation of feeding behavior in adult Drosophila melanogaster varies with feeding regime and nutritional state. J. Exp. Biol. 197, 215–235.
Engel, P., and Moran, N. A. (2013). The gut microbiota of insects – diversity in structure and function. FEMS Microbiol. Rev. 37, 699–735. doi: 10.1111/1574-6976.12025
Enjin, A., Zaharieva, E. E., Frank, D. D., Mansourian, S., Suh, G. S. B., Gallio, M., et al. (2016). Humidity sensing in Drosophila. Curr. Biol. 26, 1352–1358. doi: 10.1016/j.cub.2016.03.049
Etges, W. J. (1992). Premating isolation is determined by larval substrates in cactophilic Drosophila mojavensis. Evolution 46, 1945–1950. doi: 10.2307/2410042
Etges, W. J., Veenstra, C. L., and Jackson, L. L. (2006). Premating isolation is determined by larval rearing substrates in cactophilic Drosophila mojavensis. VII. Effects of larval dietary fatty acids on adult epicuticular hydrocarbons. J. Chem. Ecol. 32, 2629–2646. doi: 10.1007/s10886-006-9187-8
Feder, J. L., and Filchak, K. E. (1999). It’s about time: the evidence for host plant-mediated selection in the apple maggot fly, Rhagoletis pomonella, and its implications for fitness trade-offs in phytophagous insects. Entomol. Exp. Appl. 91, 211–225.
Feder, J. L., Opp, S. B., Wlazlo, B., Reynolds, K., Go, W., and Spisak, S. (1994). Host fidelity is an effective premating barrier between sympatric races of the apple maggot fly. Proc. Natl. Acad. Sci. U.S.A. 91, 7990–7994. doi: 10.1073/pnas.91.17.7990
Fedina, T. Y., Kuo, T.-H., Dreisewerd, K., Dierick, H. A., Yew, J. Y., and Pletcher, S. D. (2012). Dietary effects on cuticular hydrocarbons and sexual attractiveness in Drosophila. PLoS One 7:e49799. doi: 10.1371/journal.pone.0049799
Fei, M., Gols, R., and Harvey, J. A. (2014). Seasonal phenology of interactions involving short-lived annual plants, a multivoltine herbivore and its endoparasitoid wasp. J. Anim. Ecol. 83, 234–244. doi: 10.1111/1365-2656.12122
Filchak, K. E., Roethele, J. B., and Feder, J. L. (2000). Natural selection and sympatric divergence in the apple maggot Rhagoletis pomonella. Nature 407, 739–742. doi: 10.1038/35037578
Fogleman, J. C., and Danielson, P. B. (2001). Chemical interactions in the cactus-microorganism- Drosophila model system of the Sonoran desert. Am. Zool. 41, 877–889. doi: 10.1093/icb/41.4.877
Fry, J. D., Heinsohn, S. L., and Mackay, T. F. C. (1996). The contribution of new mutations to genotype-environment interaction for fitness in Drosophila melanogaster. Evolution 50, 2316–2327. doi: 10.1111/j.1558-5646.1996.tb03619.x
Gadenne, C., Barrozo, R. B., and Anton, S. (2016). Plasticity in insect olfaction: to smell or not to smell? Annu. Rev. Entomol. 61, 317–333. doi: 10.1146/annurev-ento-010715-023523
Gleason, J. M., Jallon, J.-M., Rouault, J.-D., and Ritchie, M. G. (2005). Quantitative trait loci for cuticular hydrocarbons associated with sexual isolation between Drosophila simulans and D. sechellia. Genetics 171, 1789–1798. doi: 10.1534/genetics.104.037937
Gleason, J. M., James, R. A., Wicker-Thomas, C., and Ritchie, M. G. (2009). Identification of quantitative trait loci function through analysis of multiple cuticular hydrocarbons differing between Drosophila simulans and Drosophila sechellia females. Heredity 103, 416–424. doi: 10.1038/hdy.2009.79
Gloss, A. D., Dittrich, A. C. N., Lapoint, R. T., Goldman-Huertas, B., Verster, K. I., Pelaez, J. L., et al. (2019). Evolution of herbivory remodels a Drosophila genome. bioRxiv [Preprint]. doi: 10.1101/767160
Gloss, A. D., Groen, S. C., and Whiteman, N. K. (2016). A genomic perspective on the generation and maintenance of genetic diversity in herbivorous insects. Annu. Rev. Ecol. Evol. Syst. 47, 165–187. doi: 10.1146/annurev-ecolsys-121415-032220
Goff, G. L., and Hilliou, F. (2017). Resistance evolution in Drosophila: the case of CYP6G1. Pest Manag. Sci. 73, 493–499. doi: 10.1002/ps.4470
Goldman-Huertas, B., Mitchell, R. F., Lapoint, R. T., Faucher, C. P., Hildebrand, J. G., and Whiteman, N. K. (2015). Evolution of herbivory in Drosophilidae linked to loss of behaviors, antennal responses, odorant receptors, and ancestral diet. Proc. Natl. Acad. Sci. U.S.A. 112, 3026–3031. doi: 10.1073/pnas.1424656112
Gorter, J. A., Jagadeesh, S., Gahr, C., Boonekamp, J. J., Levine, J. D., and Billeter, J.-C. (2016). The nutritional and hedonic value of food modulate sexual receptivity in Drosophila melanogaster females. Sci. Rep. 6:19441. doi: 10.1038/srep19441
Grosjean, Y., Rytz, R., Farine, J.-P., Abuin, L., Cortot, J., Jefferis, G. S. X. E., et al. (2011). An olfactory receptor for food-derived odours promotes male courtship in Drosophila. Nature 478, 236–240. doi: 10.1038/nature10428
Günther, C. S., and Goddard, M. R. (2019). Do yeasts and Drosophila interact just by chance? Fungal Ecol. 38, 37–43. doi: 10.1016/j.funeco.2018.04.005
Haerty, W., Gibert, P., Capy, P., Moreteau, B., and David, J. R. (2003). Microspatial structure of Drosophila melanogaster populations in Brazzaville: evidence of natural selection acting on morphometrical traits. Heredity 91, 440–447. doi: 10.1038/sj.hdy.6800305
Hansson, B. S., and Stensmyr, M. C. (2011). Evolution of insect olfaction. Neuron 72, 698–711. doi: 10.1016/j.neuron.2011.11.003
Heed, W. B. (ed.) (1978). “Ecology and genetics of Sonoran desert Drosophila,” in Ecological Genetics: The Interface, ed. P. F. Brussard (New York, NY: Springer New York).
Heys, C., Lizé, A., Colinet, H., Price, T. A. R., Prescott, M., Ingleby, F., et al. (2018). Evidence that the microbiota counteracts male outbreeding strategy by inhibiting sexual signaling in females. Front. Ecol. Evol. 6:365. doi: 10.3389/fevo.2018.00029
Holzinger, F., Frick, C., and Wink, M. (1992). Molecular basis for the insensitivity of the Monarch (Danaus plexippus) to cardiac glycosides. FEBS Lett. 314, 477–480. doi: 10.1016/0014-5793(92)81530-y
Hood, G. R., Powell, T. H. Q., Doellman, M. M., Sim, S. B., Glover, M., Yee, W. L., et al. (2020). Rapid and repeatable host plant shifts drive reproductive isolation following a recent human-mediated introduction of the apple maggot fly, Rhagoletis pomonella. Evolution 74, 156–168. doi: 10.1111/evo.13882
Hungate, E. A., Earley, E. J., Boussy, I. A., Turissini, D. A., Ting, C.-T., Moran, J. R., et al. (2013). A locus in Drosophila sechellia affecting tolerance of a host plant toxin. Genetics 195, 1063–1075. doi: 10.1534/genetics.113.154773
Hussain, A., Üçpunar, H. K., Zhang, M., Loschek, L. F., and Grunwald Kadow, I. C. (2016). Neuropeptides modulate female chemosensory processing upon mating in Drosophila. PLoS Biol. 14:e1002455. doi: 10.1371/journal.pbio.1002455
Jaenike, J. (1990). Host specialization in phytophagous insects. Annu. Rev. Ecol. Evol. Syst. 21, 243–273. doi: 10.1146/annurev.ecolsys.21.1.243
Janz, N. (2011). Ehrlich and raven revisited: mechanisms underlying codiversification of plants and enemies. Annu. Rev. Ecol. Evol. Syst. 42, 71–89. doi: 10.1146/annurev-ecolsys-102710-145024
Jefferis, G. S. X. E., Potter, C. J., Chan, A. M., Marin, E. C., Rohlfing, T., Maurer, C. R., et al. (2007). Comprehensive maps of Drosophila higher olfactory centers: spatially segregated fruit and pheromone representation. Cell 128, 1187–1203. doi: 10.1016/j.cell.2007.01.040
Johnson, R. M., Harpur, B. A., Dogantzis, K. A., Zayed, A., and Berenbaum, M. R. (2018). Genomic footprint of evolution of eusociality in bees: floral food use and CYPome “blooms”. Insect. Soc. 65, 445–454. doi: 10.1007/s00040-018-0631-x
Jones, C. D. (1998). The genetic basis of Drosophila sechellia’s resistance to a host plant toxin. Genetics 149, 1899–1908.
Joseph, R. M., and Carlson, J. R. (2015). Drosophila chemoreceptors: a molecular interface between the chemical world and the brain. Trends Genet. 31, 683–695. doi: 10.1016/j.tig.2015.09.005
Jousselin, E., Cruaud, A., Genson, G., Chevenet, F., Foottit, R. G., and Cśur d’acier, A. (2013). Is ecological speciation a major trend in aphids? Insights from a molecular phylogeny of the conifer-feeding genus Cinara. Front. Zool. 10:56. doi: 10.1186/1742-9994-10-56
Kambach, S., Kühn, I., Castagneyrol, B., and Bruelheide, H. (2016). The impact of tree diversity on different aspects of insect herbivory along a global temperature gradient – a meta-analysis. PLoS One 11:e0165815. doi: 10.1371/journal.pone.0165815
Karageorgi, M., Bräcker, L. B., Lebreton, S., Minervino, C., Cavey, M., Siju, K. P., et al. (2017). Evolution of multiple sensory systems drives novel egg-laying behavior in the fruit pest Drosophila suzukii. Curr. Biol. 27, 847–853. doi: 10.1016/j.cub.2017.01.055
Karageorgi, M., Groen, S. C., Sumbul, F., Pelaez, J. N., Verster, K. I., Aguilar, J. M., et al. (2019). Genome editing retraces the evolution of toxin resistance in the monarch butterfly. Nature 574, 409–412. doi: 10.1038/s41586-019-1610-8
Kazama, H. (2015). Systems neuroscience in Drosophila: conceptual and technical advantages. Neuroscience 296, 3–14. doi: 10.1016/j.neuroscience.2014.06.035
Keesey, I. W., Knaden, M., and Hansson, B. S. (2015). Olfactory specialization in Drosophila suzukii supports an ecological shift in host preference from rotten to fresh fruit. J. Chem. Ecol. 41, 121–128. doi: 10.1007/s10886-015-0544-3
Kessler, A., and Baldwin, I. T. (2001). Defensive function of herbivore-induced plant volatile emissions in nature. Science 291, 2141–2144. doi: 10.1126/science.291.5511.2141
Khallaf, M. A., Auer, T. O., Grabe, V., Depetris-Chauvin, A., Ammagarahalli, B., Zhang, D.-D., et al. (2020). Mate discrimination among subspecies through a conserved olfactory pathway. Sci. Adv. 6:eaba5279. doi: 10.1126/sciadv.aba5279
Kirkpatrick, M., and Ravigné, V. (2002). Speciation by natural and sexual selection: models and experiments. Am. Nat. 159, S22–S35. doi: 10.1086/338370
Ko, K. I., Root, C. M., Lindsay, S. A., Zaninovich, O. A., Shepherd, A. K., Wasserman, S. A., et al. (2015). Starvation promotes concerted modulation of appetitive olfactory behavior via parallel neuromodulatory circuits. eLife 4:e08298. doi: 10.7554/eLife.08298.001
Kromann, S. H., Saveer, A. M., Binyameen, M., Bengtsson, M., Birgersson, G., Hansson, B. S., et al. (2015). Concurrent modulation of neuronal and behavioural olfactory responses to sex and host plant cues in a male moth. Proc. Biol. Sci. 282:20141884. doi: 10.1098/rspb.2014.1884
Kuo, T.-H., Yew, J. Y., Fedina, T. Y., Dreisewerd, K., Dierick, H. A., and Pletcher, S. D. (2012). Aging modulates cuticular hydrocarbons and sexual attractiveness in Drosophila melanogaster. J. Exp. Biol. 215, 814–821. doi: 10.1242/jeb.064980
Kwan, L., and Rundle, H. D. (2010). Adaptation to desiccation fails to generate pre- and postmating isolation in replicate Drosophila melanogaster laboratory populations. Evolution 64, 710–723. doi: 10.1111/j.1558-5646.2009.00864.x
Lande, R. (1979). Quantitative genetic analysis of multivariate evolution, applied to brain: body size allometry. Evolution 33, 402–416. doi: 10.1111/j.1558-5646.1979.tb04694.x
Lanno, S. M., Gregory, S. M., Shimshak, S. J., Alverson, M. K., Chiu, K., Feil, A. L., et al. (2017). Transcriptomic analysis of octanoic acid response in Drosophila sechellia using RNA-sequencing. G3 (Bethesda) 7, 3867–3873. doi: 10.1534/g3.117.300297
Larter, N. K., Sun, J. S., and Carlson, J. R. (2016). Organization and function of Drosophila odorant binding proteins. eLife 5:e20242.
Lavista-Llanos, S., Svatoš, A., Kai, M., Riemensperger, T., Birman, S., Stensmyr, M. C., et al. (2014). Dopamine drives Drosophila sechellia adaptation to its toxic host. eLife 3:e03785. doi: 10.7554/eLife.03785
Leal, W. S. (2013). Odorant reception in insects: roles of receptors, binding proteins, and degrading enzymes. Annu. Rev. Entomol. 58, 373–391. doi: 10.1146/annurev-ento-120811-153635
Lebreton, S., Carlsson, M. A., and Witzgall, P. (2017). Insulin signaling in the peripheral and central nervous system regulates female sexual receptivity during starvation in Drosophila. Front. Physiol. 8:685. doi: 10.3389/fphys.2017.00685
Lebreton, S., Trona, F., Borrero-Echeverry, F., Bilz, F., Grabe, V., Becher, P. G., et al. (2015). Feeding regulates sex pheromone attraction and courtship in Drosophila females. Sci. Rep. 5:13132. doi: 10.1038/srep13132
Lee, J. C., Bruck, D. J., Dreves, A. J., Ioriatti, C., Vogt, H., and Baufeld, P. (2011). In focus. spotted wing Drosophila, Drosophila suzukii, across perspectives. Pest Manag. Sci. 67, 1349–1351. doi: 10.1002/ps.2271
Legal, L., Chappe, B., and Jallon, J. M. (1994). Molecular basis of Morinda citrifolia (L.): toxicity on Drosophila. J. Chem. Ecol. 20, 1931–1943. doi: 10.1007/BF02066234
Legal, L., Jean, R. D., and Jallon, J. M. (1992). Toxicity and attraction effects produced by Morinda citrifolia fruits on the Drosophila melanogaster complex of species. Chemoecology 3, 125–129. doi: 10.1007/BF01370140
Liang, L., and Luo, L. (2010). The olfactory circuit of the fruit fly Drosophila melanogaster. Sci. China Life Sci. 53, 472–484. doi: 10.1007/s11427-010-0099-z
Lightle, D., Ambrosion, M., and Lee, J. C. (2010). Sugar in moderation: sugar diets affect short-term parasitoid behaviour. Physiol. Entomol. 35, 179–185. doi: 10.1111/j.1365-3032.2009.00718.x
Linz, J., Baschwitz, A., Strutz, A., Dweck, H. K. M., Sachse, S., Hansson, B. S., et al. (2013). Host plant-driven sensory specialization in Drosophila erecta. Proc. Biol. Sci. 280:20130626. doi: 10.1098/rspb.2013.0626
Luo, S., Michaud, J. P., Li, J., Liu, X., and Zhang, Q. (2013). Odor learning in Microplitis mediator (Hymenoptera. Braconidae) is mediated by sugar type and physiological state. Biol. Control 65, 207–211. doi: 10.1016/j.biocontrol.2013.02.010
Mansourian, S., Enjin, A., Jirle, E. V., Ramesh, V., Rehermann, G., Becher, P. G., et al. (2018). Wild african Drosophila melanogaster are seasonal specialists on marula fruit. Curr. Biol. 28, 3960–3968.e3. doi: 10.1016/j.cub.2018.10.033
Matsubayashi, K. W., Ohshima, I., and Nosil, P. (2010). Ecological speciation in phytophagous insects. Entomol. Exp. Appl. 134, 1–27. doi: 10.1111/j.1570-7458.2009.00916.x
Matsunaga, T., Reisenman, C. E., Goldman-Huertas, B., Brand, P., Miao, K., Suzuki, H., et al. (2019). Olfactory receptors tuned to volatile mustard oils in drosophilid flies. bioRxiv [Preprint]. doi: 10.1101/2019.12.27.889774
Matsuo, T., Sugaya, S., Yasukawa, J., Aigaki, T., and Fuyama, Y. (2007). Odorant-binding proteins OBP57d and OBP57e affect taste perception and host-plant preference in Drosophila sechellia. PLoS Biol. 5:e118. doi: 10.1371/journal.pbio.0050118
Matzkin, L. M. (2012). Population transcriptomics of cactus host shifts in Drosophila mojavensis. Mol. Ecol. 21, 2428–2439. doi: 10.1111/j.1365-294X.2012.05549.x
Matzkin, L. M. (2014). “Ecological genomics of host shifts in Drosophila mojavensis,” in Ecological Genomics, eds C. R. Landry, and A.-H. Nadia (Dordrecht: Springer), 233–247. doi: 10.1007/978-94-007-7347-9_12
Matzkin, L. M., Markow, T. A., and Michalak, P. (2013). “Transcriptional differentiation across the four subspecies of Drosophila mojavensis,” in Speciation: Natural Processes, Genetics and Biodiversity, ed. P. Michalak (New York: Nova Scientific Publishers), 119–135.
McBride, C. S., Baier, F., Omondi, A. B., Spitzer, S. A., Lutomiah, J., Sang, R., et al. (2014). Evolution of mosquito preference for humans linked to an odorant receptor. Nature 515, 222–227. doi: 10.1038/nature13964
Missbach, C., Dweck, H. K. M., Vogel, H., Vilcinskas, A., Stensmyr, M. C., Hansson, B. S., et al. (2014). Evolution of insect olfactory receptors. eLife 3:e02115.
Mithöfer, A., and Boland, W. (2012). Plant defense against herbivores: chemical aspects. Annu. Rev. Plant. Biol. 63, 431–450. doi: 10.1146/annurev-arplant-042110-103854
Montell, C. (2013). Gustatory receptors: not just for good taste. Curr. Biol. 23, R929–R932. doi: 10.1016/j.cub.2013.08.020
Moraes, C. M. D., Mescher, M. C., and Tumlinson, J. H. (2001). Caterpillar-induced nocturnal plant volatiles repel conspecific females. Nature 410, 577–580. doi: 10.1038/35069058
Moreau, J., Desouhant, E., Louâpre, P., Goubault, M., Rajon, E., Jarrige, A., et al. (2017). “How host plant and fluctuating environments affect insect reproductive strategies?,” in Advances in Botanical Research, eds N. Sauvion, D. Thiéry, and P.-A. Calatayud (Cambridge, MA: Academic Press), 259–287. doi: 10.1016/bs.abr.2016.09.008
Newby, B. D., and Etges, W. J. (1998). Host preference among populations of Drosophila mojavensis (Diptera. Drosophilidae) that use different host cacti. J. Insect Behav. 11, 691–712. doi: 10.1023/A:1022398809881
Nyman, T., Linder, H. P., Peña, C., Malm, T., and Wahlberg, N. (2012). Climate-driven diversity dynamics in plants and plant-feeding insects. Ecol. Lett. 15, 889–898. doi: 10.1111/j.1461-0248.2012.01782.x
Nyman, T., Veli, V., David, R., Smith, and Boevé, J. L. (2010). How common is ecological speciation in plant-feeding insects? A ‘Higher’ nematinae perspective. BMC Evol. Biol. 10:266. doi: 10.1186/1471-2148-10-266
Otte, T., Hilker, M., and Geiselhardt, S. (2018). Phenotypic plasticity of cuticular hydrocarbon profiles in insects. J. Chem. Ecol. 44, 235–247. doi: 10.1007/s10886-018-0934-4
Pelosi, P., Iovinella, I., Felicioli, A., and Dani, F. R. (2014). Soluble proteins of chemical communication: an overview across arthropods. Front. Physiol. 5:320. doi: 10.3389/fphys.2014.00320
Prieto-Godino, L. L., Rytz, R., Cruchet, S., Bargeton, B., Abuin, L., Silbering, A. F., et al. (2017). Evolution of acid-sensing olfactory circuits in Drosophilids. Neuron 93, 661–676.e6. doi: 10.1016/j.neuron.2016.12.024
Ramasamy, S., Ometto, L., Crava, C. M., Revadi, S., Kaur, R., Horner, D. S., et al. (2016). The evolution of olfactory gene families in Drosophila and the genomic basis of chemical-ecological adaptation in Drosophila suzukii. Genome Biol. Evol. 8, 2297–2311. doi: 10.1093/gbe/evw160
Revadi, S., Vitagliano, S., Rossi Stacconi, M. V., Ramasamy, S., Mansourian, S., Carlin, S., et al. (2015). Olfactory responses of Drosophila suzukii females to host plant volatiles. Physiol. Entomol. 40, 54–64. doi: 10.1111/phen.12088
Ribeiro, C., and Dickson, B. J. (2010). Sex peptide receptor and neuronal TOR/S6K signaling modulate nutrient balancing in Drosophila. Curr. Biol. 20, 1000–1005. doi: 10.1016/j.cub.2010.03.061
Rimal, S., and Lee, Y. (2018). The multidimensional ionotropic receptors of Drosophila melanogaster. Insect Mol. Biol. 27, 1–7. doi: 10.1111/imb.12347
Rio, B., Couturier, G., Lemeunier, F., and Lachaise, D. (1983). Evolution d’une spécialisation saisonnière chez Drosophila erecta (Dipt., Drosophilidae). Ann. Soc. Entomol. France 19, 235–248.
R’Kha, S., Capy, P., and David, J. R. (1991). Host-plant specialization in the Drosophila melanogaster species complex: a physiological, behavioral, and genetical analysis. Proc. Natl. Acad. Sci. U.S.A. 88, 1835–1839. doi: 10.1073/pnas.88.5.1835
Root, C. M., Ko, K. I., Jafari, A., and Wang, J. W. (2011). Presynaptic facilitation by neuropeptide signaling mediates odor-driven food search. Cell 145, 133–144. doi: 10.1016/j.cell.2011.02.008
Rundle, H. D., Chenoweth, S. F., and Blows, M. W. (2009). The diversification of mate preferences by natural and sexual selection. J. Evol. Biol. 22, 1608–1615. doi: 10.1111/j.1420-9101.2009.01773.x
Rundle, H. D., and Dyer, K. A. (2015). Reproductive character displacement of female mate preferences for male cuticular hydrocarbons in Drosophila subquinaria. Evolution 69, 2625–2637. doi: 10.1111/evo.12761
Rundle, H. D., and Nosil, P. (2005). Ecological speciation. Ecol. Lett. 8, 336–352. doi: 10.1111/j.1461-0248.2004.00715.x
Saveer, A. M., Kromann, S. H., Birgersson, G., Bengtsson, M., Lindblom, T., Balkenius, A., et al. (2012). Floral to green: mating switches moth olfactory coding and preference. Proc. Biol. Sci. 279, 2314–2322. doi: 10.1098/rspb.2011.2710
Schluter, D. (2009). Evidence for ecological speciation and its alternative. Science (New York, N.Y.) 323, 737–741. doi: 10.1126/science.1160006
Scott, K. (2018). Gustatory processing in Drosophila melanogaster. Annu. Rev. Entomol. 63, 15–30. doi: 10.1146/annurev-ento-020117-043331
Scriber, J. M., and Slansky, F. (1981). The nutritional ecology of immature insects. Annu. Rev. Entomol. 26, 183–211. doi: 10.1146/ANNUREV.EN.26.010181.001151
Seeholzer, L. F., Seppo, M., Stern, D. L., and Ruta, V. (2018). Evolution of a central neural circuit underlies Drosophila mate preferences. Nature 559, 564–569. doi: 10.1038/s41586-018-0322-9
Servedio, M. R., and Boughman, J. W. (2017). The role of sexual selection in local adaptation and speciation. Annu. Rev. Ecol. Evol. Syst. 48, 85–109. doi: 10.1146/ANNUREV-ECOLSYS-110316-022905
Shahandeh, M. P., Pischedda, A., and Turner, T. L. (2018). Male mate choice via cuticular hydrocarbon pheromones drives reproductive isolation between Drosophila species. Evolution 72, 123–135. doi: 10.1111/evo.13389
Shahandeh, M. P., and Turner, T. L. (2020). The complex genetic architecture of male mate choice evolution between Drosophila species. Heredity 124, 737–750. doi: 10.1038/s41437-020-0309-9
Sharon, G., Segal, D., Ringo, J. M., Hefetz, A., Zilber-Rosenberg, I., and Rosenberg, E. (2010). Commensal bacteria play a role in mating preference of Drosophila melanogaster. Proc. Natl. Acad. Sci. U.S.A. 107, 20051–20056. doi: 10.1073/pnas.1009906107
Sharon, G., Segal, D., Zilber-Rosenberg, I., and Rosenberg, E. (2011). Symbiotic bacteria are responsible for diet-induced mating preference in Drosophila melanogaster, providing support for the hologenome concept of evolution. Gut Microbes 2, 190–192. doi: 10.4161/gmic.2.3.16103
Shropshire, J. D., and Bordenstein, S. R. (2016). Speciation by symbiosis: the microbiome and behavior. mBio 7:e01785. doi: 10.1128/mBio.01785-15
Silbering, A. F., and Benton, R. (2010). Ionotropic and metabotropic mechanisms in chemoreception. ‘chance or design’? EMBO Rep. 11, 173–179. doi: 10.1038/embor.2010.8
Singer, M. C., and Parmesan, C. (2010). Phenological asynchrony between herbivorous insects and their hosts: signal of climate change or pre-existing adaptive strategy? Philos. Trans. R. Soc. Lond. Ser. B 365, 3161–3176. doi: 10.1098/rstb.2010.0144
Smadja, C., and Butlin, R. (2009). On the scent of speciation: the chemosensory system and its role in premating isolation. Heredity 102, 77–97. doi: 10.1038/hdy.2008.55
Tomioka, S., Aigaki, T., and Matsuo, T. (2012). Conserved cis-regulatory elements of two odorant-binding protein genes, Obp57d and Obp57e, in Drosophila. Genes Genet. Syst. 87, 323–329. doi: 10.1266/ggs.87.323
Tsuchida, T., Koga, R., and Fukatsu, T. (2004). Host plant specialization governed by facultative symbiont. Science 303:1989. doi: 10.1126/science.1094611
Verschut, T. A., and Hambäck, P. A. (2018). A random survival forest illustrates the importance of natural enemies compared to host plant quality on leaf beetle survival rates. BMC Ecol. 18:33. doi: 10.1186/s12898-018-0187-7
Vogt, R. G., Prestwich, G. D., and Lerner, M. R. (1991). Odorant-binding-protein subfamilies associate with distinct classes of olfactory receptor neurons in insects. J. Neurobiol. 22, 74–84. doi: 10.1002/neu.480220108
Vosshall, L. B., Amrein, H., Morozov, P. S., Rzhetsky, A., and Axel, R. (1999). A spatial map of olfactory receptor expression in the Drosophila antenna. Cell 96, 725–736. doi: 10.1016/S0092-8674(00)80582-6
Wagner, D. L., and Driesche, R. G. V. (2010). Threats posed to rare or endangered insects by invasions of nonnative species. Annu. Rev. Entomol. 55, 547–568. doi: 10.1146/annurev-ento-112408-085516
Webster, B., and Cardé, R. T. (2017). Use of habitat odour by host-seeking insects. Biol. Rev. 92, 1241–1249. doi: 10.1111/brv.12281
Weddle, C. B., Steiger, S., Hamaker, C. G., Ower, G. D., Mitchell, C., Sakaluk, S. K., et al. (2013). Cuticular hydrocarbons as a basis for chemosensory self-referencing in crickets. A potentially universal mechanism facilitating polyandry in insects. Ecol. Lett. 16, 346–353. doi: 10.1111/ele.12046
Wetzel, W. C., Kharouba, H. M., Robinson, M., Holyoak, M., and Karban, R. (2016). Variability in plant nutrients reduces insect herbivore performance. Nature 539, 425–427. doi: 10.1038/nature20140
Wilson, D. A., Best, A. R., and Sullivan, R. M. (2004). Plasticity in the olfactory system: lessons for the neurobiology of memory. Neuroscientist 10, 513–524. doi: 10.1177/1073858404267048
Xue, H.-J., Segraves, K. A., Wei, J., Zhang, B., Nie, R.-E., Li, W.-Z., et al. (2018). Chemically mediated sexual signals restrict hybrid speciation in a flea beetle. Behav. Ecol. 29, 1462–1471. doi: 10.1093/beheco/ary105
Yassin, A., Debat, V., Bastide, H., Gidaszewski, N., David, J. R., and Pool, J. E. (2016). Recurrent specialization on a toxic fruit in an island Drosophila population. Proc. Natl. Acad. Sci. U.S.A. 113, 4771–4776. doi: 10.1073/pnas.1522559113
Yukilevich, R. (2012). Asymmetrical patterns of speciation uniquely support reinforcement in Drosophila. Evolution 66, 1430–1446. doi: 10.1111/j.1558-5646.2011.01534.x
Keywords: ecological speciation, resource heterogeneity, food, adaptation, reproductive isolation, insect
Citation: Wang X, Verschut TA, Billeter J-C and Maan ME (2021) Seven Questions on the Chemical Ecology and Neurogenetics of Resource-Mediated Speciation. Front. Ecol. Evol. 9:640486. doi: 10.3389/fevo.2021.640486
Received: 11 December 2020; Accepted: 28 January 2021;
Published: 18 February 2021.
Edited by:
Sebastien Lebreton, Institut de Recherche en Sémiochimie et Ethologie Appliquée (IRSEA), FranceReviewed by:
Mohammed A. Khallaf, Max Planck Institute for Chemical Ecology, GermanySuzan Mansourian, Lund University, Sweden
Sofía Lavista Llanos, Max Planck Institute for Chemical Ecology, Germany
Copyright © 2021 Wang, Verschut, Billeter and Maan. This is an open-access article distributed under the terms of the Creative Commons Attribution License (CC BY). The use, distribution or reproduction in other forums is permitted, provided the original author(s) and the copyright owner(s) are credited and that the original publication in this journal is cited, in accordance with accepted academic practice. No use, distribution or reproduction is permitted which does not comply with these terms.
*Correspondence: Xiaocui Wang, eGlhb2N1aS53YW5nQHJ1Zy5ubA==