- 1Department of Archaeology, Vilnius University, Vilnius, Lithuania
- 2Department of Archaeology, Max Planck Institute for the Science of Human History, Jena, Germany
The morphology of ancient cereal grains in Central Asia has been heavily discussed as an indicator of specific genetic variants, which are often linked to cultural factors or distinct routes of dispersal. In this paper, we present the largest currently existing database of barley (n = 631) and wheat (n = 349) measurements from Central Asia, obtained from two different periods at the Chap site (ca. 3,500 to 1,000 BC), located in the Tien Shan Mountains of Kyrgyzstan at 2,000 masl. The site is situated at the highest elevation ecocline for successful cereal cultivation and is, therefore, highly susceptible to minor climatic fluctuations that could force gradients up or down in the foothills. We contrast the Chap data with measurements from other second and first millennia BC sites in the region. An evident increase in average size over time is likely due to the evolution of larger grains or the introduction of larger variants from elsewhere. Additionally, site- or region-specific variation is noted, and we discuss potential influences for the formation of genetic varieties, including possible pleiotropic linkages and/or developmental responses to external factors, such as environmental fluctuations, climate, irrigation inputs, soil nutrients, pathologies, and seasonality. External factors acting on developmental or acclamatory responses in plants can be either natural or cultural. We argue that the study of long-term changes in grain morphology on the edges of crop-growing ranges can be informative regarding cultural and environmental constraints in the past.
Introduction
Targeted sickle harvesting of wild barley (Hordeum vulgare ssp. spontaneum) stands in the eastern Mediterranean by aceramic foragers appears to date back to at least 23,000 years ago (Groman-Yaroslavski et al., 2016). Although recent scholarship has questioned the long-standing narrative of a link between the sickle and the evolution of rachis toughness (Maeda et al., 2016), increasingly, this scholarship is pointing toward unconscious, possibly secondary or pleiotropic, drivers for the earliest evolutionary changes under cultivation (Jones et al., 2021). By 10,000 years ago, several genetically isolated populations of barley began to evolve in response to heavy human seed predation, associated with seed saving and dispersal behaviors, leading to a marked loss of the natural seed-dispersal mechanism and resulting in obligate domestication (Willcox, 2004; Willcox and Stordeur, 2012; Asouti and Fuller, 2013; Fuller and Weisskopf, 2014; Li and Olsen, 2016). Simultaneously, seed length and width gradually increased across the cultivated populations in southwest Asia and continued to increase as the crops dispersed into Anatolia. Barley rapidly spread with the Founder Crops across Europe and West Asia, reaching southern Central Asia by 6,500 BC (Harris, 2010), the eastern edge of the Iranian Plateau at Mehrgarh by 6,000 BC (Costantini, 1984), into the Ganges Plain (Liu et al., 2017) and north all the way to the Altai Mountains by 3000 BC (Zhou et al., 2020). Barley entered the economic system in Japan by the fifth century AD (Leipe et al., 2017) and developed into a monocropping system in the Tibetan highlands by the early first millennium BC (Tang et al., 2020). Barley remained the most important grain crop across Europe and West Asia from the earliest cultivation systems until the intensification of irrigation and crop-rotation cycles around the mid-first millennium BC, when free-threshing wheat (Triticum aestivum) started to increase in prominence across both Europe and West Asia and hulled varieties largely replaced naked forms of barley (Lister and Jones, 2013; Spengler, 2015). In the high-elevation mountain regions of the Tien Shan or the Himalaya, naked barley has been the dominant crop for nearly four millennia (Motuzaite Matuzeviciute et al., 2020a, 2021; Tang et al., 2020).
There are many potential reasons for the prominent role of barley in mixed agropastoral systems across such a wide span of time and space, including its tolerance to environmental constraints, notably salinity, aridity, frost (Fuller and Weisskopf, 2014; Riehl, 2019; Spengler, 2019), and low-nutrient soils (Zohary and Hopf, 2000). As with most crops, barley has proven to express rapid acclamatory, developmental, and evolutionary responses to extreme stressors. Despite claims to the contrary (Abbo and Gopher, 2020), wild barley expresses the same traits of weediness that characterize most crop progenitors, allowing it to: (1) express a highly plastic range of phenotypes without genetic change; (2) lack dormancy, even in the progenitor form and, presumably, rapidly evolve seasonal synchronization and self-compatibility; and (3) evolve a suite of derived traits for adaptation to an anthropogenic environment, i.e., a non-brittle rachis (btr1 and btr2) (Pourkheirandish et al., 2015), naked morphotype (nud), six-rowed forms (vrs1 and int-c), shutting off of photoperiod sensitivity (Ppd-H1 allele) (Liu et al., 2017; Lister et al., 2018), larger grains, frost tolerance, reduced tillering, reduced lodging, greater yields, etc. The high degree of plasticity in barley allowed the crop to move with farming beyond the growing ranges of its progenitor. Greater rates of evolutionary adaptation tend to occur at the margins of a species' range (Mayr, 1942). One example of this range edge effect for barley comes from the rapid adaptation of qingke barley to high elevation Tibet (Tang et al., 2020), often argued to have occurred through hybridization and gene transfer from native wild barleys (Dai et al., 2012; Zeng et al., 2015), although recent research is suggesting rapid evolution of a specific ecotype, and the subsequent feralization to brittle-rachis forms outside cultivation (Zeng et al., 2018).
In this paper, we discuss three plausible drivers for population-scale morphological change: (1) evolutionary adaptation (i.e., ecotypes or landraces); (2) pleiotropic linkages to other evolved adaptations; and (3) developmental plasticity (non-heritable adaptation). Looking at population-scale variations in cereals from Central Asia provides an important complement to the studies already conducted on diachronic grain size changes in Europe and southwest Asia (Asouti and Fuller, 2013; Fuller and Weisskopf, 2014; Fuller et al., 2017). In most cases, gradual increases in grain size over time are assumed to be a continuation of the domestication process in the form of parallel evolution of larger seeds. Evolutionary changes in grain structure may represent the continuation of the domestication process, which consists of unconscious selective processes, presumably associated with seed saving among other cultivation practices, and often (not always) result in size increase over time (Jones et al., 2021). However, the details of how these evolutionary processes play out and what the selection pressures were remain topics of debate among scholars (Abbo and Gopher, 2020; Spengler, 2020). For example, pleiotropic linkages have been implicated in certain cases of shifting grain dimensions in Central Asia, along with selective adaptations for the anthropogenic niche, such as a breaking of photoperiod sensitivity (Fuller and Allaby, 2009) or a reduction in lodging (Cheng et al., 2020). Likewise, adaptive divergence in isolated populations facing differing selection pressures can lead to ecotypes of differing grain sizes (Murphy, 2007).
Given the high level of plasticity tied to grain size, a phenotype, especially of a crop growing at the margins of its range, may not directly reflect its genotype. In our interpretations, we exclude any heterogeneous developmental responses in grains, such as differing seasonal stress or uneven conditions across a cultivated field. The archaeobotanical record is a palimpsest of annual seasons and a mingling of grains from across a large catchment area. The plastic responses to heterogeneous factors would simply show up as a greater range of variation, rather than a directional shift in features, as seen in varying archaeobotanical assemblages at the sites of the central Tien Shan (Motuzaite Matuzeviciute et al., 2018). However, developmental plasticity could, theoretically, resemble population-scale evolutionary changes in seed dimensions if the factors causing the developmental constraints were constant over many plant generations and across the entire cultivated population. These developmental constraints or releases from constraints include both natural and cultural factors, such as soil nutrients, irrigation, or extreme cold at higher elevation. Scholars have discussed the highly compact nature of second millennium BC wheat in Central Asia, but no consensus has been drawn regarding the causes of the compact forms. Little is known about variation in grain size and changes in forms of barley through time and space, as the crop spread outwards from its natural habitat of initial domestication. We refer to compact grains as those smaller and plumper than standard barley grains, size expressed as a shorter length, and plumpness as a higher length/breadth and length/thickness ratio.
We present a dataset of barley grain measurements (n = 631) from one high-elevation site spanning 1,500 years. Our data from Chap illustrate the ongoing process of adaptation as plants dispersed into the margins of cereal cultivation. In the light of a wider regional overview of published barley grain measurements, we discuss possible reasons for the barley caryopsis size shift, considering multiple processes that could have affected size and shape. In addition to barley, wheat caryopsis measurements (n = 349) are presented for Chap I to better understand the morphological change through time in relation to barley. Ultimately, we argue that (1) average grain size increased over time, as did distinct changes in morphology; (2) dimensional differences between hulled and naked varieties are clear; and (3) regional differences in morphology and variability are tied into a suite of developmental and genetic responses to both environmental and cultural factors.
The Chap Site
Chap II is located in the central Tien Shan Mountains of Kyrgyzstan at 2,000 masl (Figure 1). Archaeological excavations were conducted during the summers of 2018 and 2019, resulting in the discovery of two distinct occupation horizons separated into Chap II and Chap I phases. Chap II is dated to between 2,465 and 2,047 cal BC, while Chap I dates between 1,065 and 825 cal BC. The archaeobotanical investigations of both phases yielded large assemblages of domesticated grains and chaff, which reflect agricultural activities by the inhabitants of the site. Chap II may represent the first wave of farmers dispersing east into the Central Asian mountains around 4,500 years ago, who then left the site for 1,500 years and later picked up occupation again 3,000 years ago (Motuzaite Matuzeviciute et al., 2018, 2020a,b). Chap II consists of only lenses of ash containing carbonized cereal grains, while Chap I represents a typical farmstead with discarded crop processing waste found together with grinding stones, pottery sherds, and animal bones.
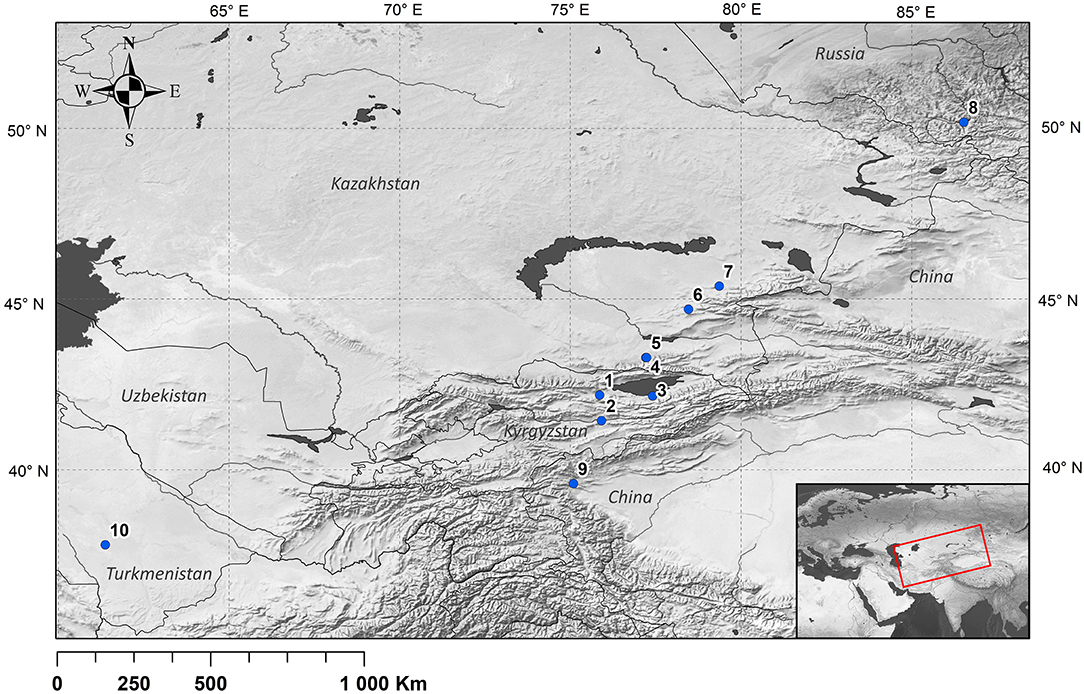
Figure 1. Archaeological sites mentioned in the paper: 1, Chap; 2, Aigyrzhal; 3, Uch-Kurbu; 4, Tuzusai; 5, Tseganka; 6, Begash; 7, Tasbas; 8, Tongtian cave; 9, Wupaer; 10, Adji-Kui.
Methods
Length, width, and breadth measurements of wheat and barley grains from two different occupation horizons at Chap were made using a Zeiss stereomicroscope, allowing for precise measurements of up to 0.01 mm. Only well-preserved, fully intact cereal grains were suitable for measuring all three parameters (length, width, and depth) at the widest areas of the caryopsis excluding the radicle (Motuzaite Matuzeviciute et al., 2021). In total, 631 barley grains were measured: 375 grains from phase I and 256 from phase II. Not all barley grains were divided into naked or hulled varieties, yet this criterion and possible variation in size was taken into consideration while analyzing the data (see below). Six- and two-row barleys were not separated due to morphological overlap between the two forms and a lack of rachises. Additionally, 38 wheat grains were measured from Chap I, and 311 wheat grains were measured from Chap II. Scatter plots of individual grains were built to compare Chap II and I data with other sites, testing for the relationship between them. In addition, we considered a scatter plot of the mean value of barley length and width from other archaeological sites in Central Asia. Building scatter plots, chronological trends have been taken into consideration embracing the period from the beginning of the fourth to the first millennia BC. Additional measurements used in this study were collected from already published research (Spengler, 2013; Spengler et al., 2014a, 2018; Motuzaite Matuzeviciute et al., 2018, 2020a, 2021; Zhou et al., 2020). A statistical test (Mann–Whitney U test) was used to contrast the naked barley and free-threshing wheat grains from the two different periods. The threshold for the p-value applied in this study was 0.05. We used MATLAB-based software to calculate and plot the ratios of the naked barley grains from Chap I and Chap II: length (L) to width (W), length (L) to depth, and width (W) to depth (D) all relative to the length, in order to evaluate seed size and shape change through time.
Results
Chap Barley Measurements
The Chap II (2,465–2,047 cal BC) assemblage predominantly contains naked morphotypes of barley (n = 256), which fits with other observations in Central Asia that hulled forms only became prominent during the first millennium BC. The average dimensions of these grains were 4.3 mm in length, width was 3.0 mm, and thickness was 2.3 mm. The barley grains from Chap I (1,065–825 cal BC) consist of 100 hulled, 200 naked, and 75 grains that could not be clearly differentiated (Motuzaite Matuzeviciute et al., 2018). The grains of naked barley had an average length of 5.1 mm, width of 3.0 mm, and depth of 2.2 mm. The average length of hulled barley was 5.4 mm, width was 2.7 mm, and depth was 2.1 mm (SI 1) (Figure 2). Comparing the naked barley grains from the early (Chap II) and late (Chap I) periods, there is a significant difference in grain length, while width and depth ranges are similar (Table 1). Consequently, it is clear that the grains of naked barley became more elongated before the first millennium BC. Yet, the statistical test comparing differences in width and depth between Chap I and Chap II sites does not capture the compact nature of the Chap II barley grains and the morphotypical difference between the two assemblages, due to the wide distribution of caryopses sizes. Therefore, we plotted the ratios of length to width (L/W) (Figure 3A), length to depth (L/D) (Figure 3B), and width to depth (W/D) (Figure 3C), relative to length of all naked barleys caryopses from Chap I and Chap II, in order to explore the possibility of differences in these ratios exhibited between the two data sets.
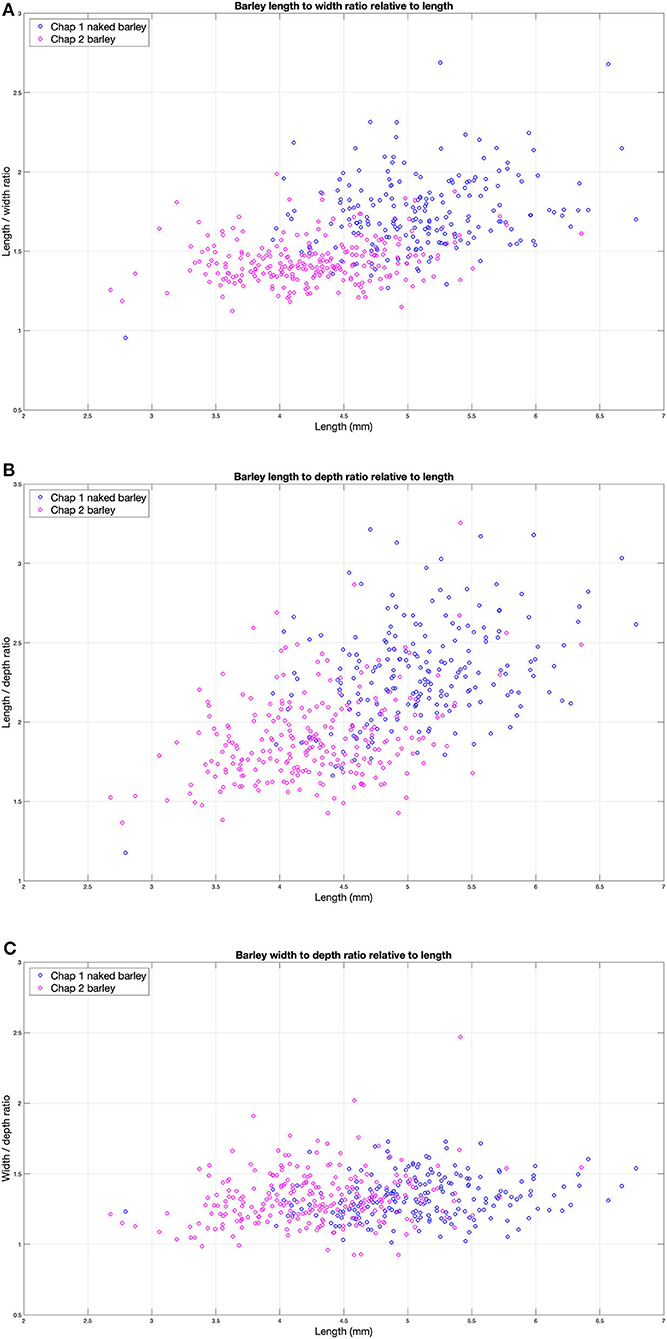
Figure 3. The ratios of the naked barley grains from Chap I and Chap II: (A) length (L) to width (W), (B) length (L) to depth, and (C) width (W) to depth Q20 (D) all relative to the length for evaluation the characteristics in seed size change through time.
The L to W ratio (L/W) plot (Figure 3A) visually demonstrates a clear difference in the L/W ratio between the Chap I and Chap II datasets. The mean and standard deviation of the L/W, ratios for the two datasets are (μ = 1.748, σ = 0.2353) and (μ = 1.4416, σ = 0.1425), respectively. The seeds from Chap I are significantly longer in relation to their width in comparison to Chap II, demonstrating that the Chap II assemblage represents a much more compact seed shape, while the Chap I barley grains are more elongated. The cross-correlation coefficients for L/W vs. L for the two data sets are 0.258 and 0.212, respectively, indicating that there is a slight positive correlation of similar magnitude between the L/W ratio with respect to increasing seed length in both assemblages.
We observed a similar situation with the barley L to D ratio (L/D) relative to L (Figure 3B), where the mean and standard deviation of the L/D ratios for the two datasets are (μ = 2.3185, σ = 0.3266) and (μ = 1.9007, σ = 0.2718), respectively. As with the L/W ratio comparison, the seeds from Chap I are significantly longer in relation to their depth in comparison to Chap II, demonstrating the same Chap II assemblage compact seed shape with respect to Chap I. The cross-correlation coefficients for L/D vs. L for the two data sets are 0.3811 and 0.3392, respectively, indicating that there is an even stronger positive correlation of similar magnitude between the L/D ratio with respect to increasing seed length in both assemblages.
Finally, the width to depth ratio (W/D) relative to length for the two data sets was analyzed (Figure 3C). Interestingly, here, the W/D ratios between Chap I and Chap II are almost exactly the same, with the mean and standard deviation of the W/D ratios being (μ = 1.3332, σ = 0.1500) and (μ = 1.3243, σ = 0.1867), respectively. These values demonstrate convincingly that the W/D ratio characteristic of the barleys from Chap II and Chap I did not change over the timespan between the two assemblages. The cross-correlation coefficients for W/D vs. L for the two data sets are 0.1778 and 0.1949, respectively, indicating a very weak relationship between these two variables. The scatter plot in Figure 2 also illustrates the differences between naked and hulled varieties at Chap I and their differences from the Chap II barley. As has generally been shown from sites across Europe and West Asia, the hulled barley variety is slightly longer than the naked one. The hulled barley grains in this study express a slightly greater range of variability in length, but their width is generally similar to naked forms.
Chap Wheat Measurements
Wheat was the dominant crop at Chap II where 311 wheat grains were measured. The average length of these grains was 3.9 mm, the average width was 2.9 mm, and the average thickness was 2.2 mm. Only 38 wheat grains were measured from Chap I; the average grain size was 4.0 mm in length, 3.0 mm in width, and 3.0 mm in thickness. The statistical test shows no significant differences between wheat grains of the two periods in length and width (Table 2). The scatter plot (Figure 4) demonstrates that grains of different periods overlap considerably.
Early Compact Barley Forms and the Change in Morphotypes Through Time
The compact form of barley has been previously reported at the earliest prehistoric farming sites in the mountainous regions of Central Asia, such as Chap II in Tien Shan Mountains, dated to the mid-third millennium BC (Motuzaite Matuzeviciute et al., 2018, 2020a, 2021), Begash in Dhzungar Mountains, also dated to mid-third millennium BC (Spengler, 2013; Spengler et al., 2014a), and the late fourth millennium BC Tongtian Cave site in the Chinese Altai (Zhou et al., 2020). By the later period of the second and first millennia BC, compact forms of barley are less common from archaeobotanical assemblages, and larger lax-eared forms became dominant (Spengler, 2019), although in China, compact barley grains are reported from sites dating into the first millennium BC, such as Bangga (Tang et al., 2020).
The earliest reported compact naked barley grains come from the Tongtian Cave, located in the Altai Mountains, dating to the beginning of the fourth millennium BC (Zhou et al., 2020) containing a mean length to width ratio of barley grains (L/W) of 1.46. The Adji-Kui site, located in a natural depression in the Kara-Kum Desert on the Murghab Alluvial Fan of Turkmenistan, was occupied from 2,272 to 1,961 BC and had barley grains with a mean length to width ratio (L/W) of 1.46. The other third millennium BC site of Begash contained grains with a mean length to width of 1.55. All three sites contained compact forms similar to those of Chap II (L/W = 1.44).
During the second millennium BC, sites like Tasbas (L/W = 1.5), located in the Dhzungar Mountains of eastern Kazakhstan, and Uch–Kurbu (L/W = 1.56), located in the foothills of the Issyk-Kul region of Kyrgyzstan, still contain compact naked barley forms. Moreover, although there are no grain measurements available for Sarazm in Tadjikistan and Ojakly in Turkmenistan, the authors mention compact forms of naked barley (Spengler and Willcox, 2013; Spengler et al., 2014b) dating to the second millennium BC. In the early second millennium BC, Aigyrzhal, located in the central Tien Shan of Kyrgyzstan, barley, however, is less compact overall (L/W = 1.75) (Figure 4).
An increase in barley grain lengths was observed at first millennium BC sites across Central Asia; barley grains were longer and slightly narrower at Chap I (L/W = 1.75) and Tuzusai (L/W = 1.75). One exception can be seen in Tseganka, located along the mountain/steppe ecotone in the Tien Shan Mountains of eastern Kazakhstan (Spengler, 2013). While the small sample size from Tseganka may bias the interpretation, the grains were longer, wider, and more compact (L/W = 1.33).
Here, we can see that although the average seed sizes across Central Asia tends to increase, some sites retain compact barley forms throughout prehistory. Our data show, however, that changes from compact to elongated forms seem to be a wider phenomenon (Figures 5, 6). Additionally, much of the increase in length may be associated with the introduction of novel hulled forms during the first millennium BC that were not always differentiated from the naked forms in other Central Asian sites. The majority of grain measurements, except from territory of Kyrgyzstan, does not have grain depth data; therefore, we can only assume changes in depth through time relative to length in other regions of Central Asia.
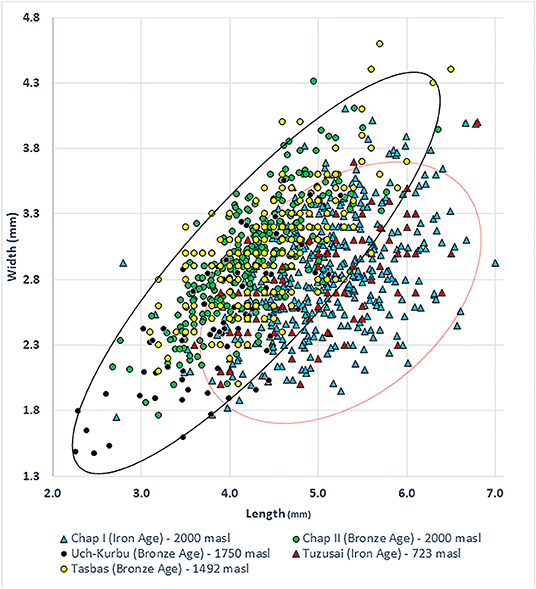
Figure 6. The mean values of barley lengths to widths ratios plotted against the length from archaeological sites in Central Asia.
Discussion
Plausible Drivers for Population-Scale Size Changes
We have presented the barley and wheat measurements from the earliest waves of Neolithization into this marginal zone of Central Asia, spanning the fourth millennium BC to the establishment of more intensive forms of farming during the first millennium BC (Figure 7). We discuss plausible drivers for population-scale size changes though time and the drivers of compact grain morphologies during the earliest wave of agriculture into Central Asian marginal geographic zones. We note that developmental plasticity may be responsible for non-heritable population-scale size changes if the selective constraints were relatively uniform across a population, as might be expected if the entire population was experiencing constraints to growth or developmental stress homogenously—possibly due to cold climatic spells or regular levels of aridity, resulting from being cultivated at the margins of their growing ranges. Selection constraints that may have affected development in grain size include uniform environmental factors of high salinity, low water availability, low nutrient availability, high solar insulation, and extreme temperature fluctuations. Note that any developmental stress that varies between seasons or across the population would show up as greater variability in the archaeobotanical assemblage rather than a directional change in morphology.
It is also likely that the observed size changes represent evolution through time. We define evolution as the change in allele frequencies within a population over time, which can result from either natural selection or gene flow. In this case, gene flow can include the introduction of novel varieties, and as many evolutionary biologists have pointed out, evolution is a population-scale phenomenon (Mayr, 1942). As has been illustrated for most grain and legume crops, unconscious selective pressures have continually led to the evolution of larger seeds and more complex inflorescences, with periods of stress resulting in smaller seeds; ultimately, cultivated grains today are much larger than those of a millennium ago, following a rough trend back to the Neolithic (Gepts, 2003; Asouti and Fuller, 2013; Fuller and Weisskopf, 2014; Kistler et al., 2018). In some cases, such as for the dispersal of glume wheats and hulled barley into northern Europe, evolutionary size increase either halted or reversed, and this has been explained as a result of ecological constraints altering directionality (Asouti and Fuller, 2013; Fuller et al., 2017). Considerable debate has gone into what the selective forces driving increased seed size in crops would have been, and we will not dive into these debates here, but we will note that sibling competition in densely packed fields and deep seed burial during sowing or tilling are readily cited drivers (Harlan, 1995; Zohary, 2004; Jones et al., 2021). Additionally, changes in seed size may be tied into pleiotropic linkages to evolutionary change in other plant parts that have been implemented in the development of highly compact wheat morphotypes. Pleiotropic linkages may have resulted in the evolution of more compact grain morphotypes in wheat and barley across Asia due to selection for (1) reduced tillering, (2) reduced lodging, (3) breaking of photoperiod sensitivity, or (4) a syndrome of architectural changes (e.g., spheroccocoidism).
Evolution of Ecotypes or Landraces
A likely explanation for a gradual seed size increase over time is that the evolution of domestication traits was part of the ongoing process of domestication, as grains dispersed north into Central Asia. A recent paper by Stevens et al. (2021) shows a gradual increase in broomcorn millet seed size though time as a part of the domestication process in selecting larger seed size for human consumption. Many parallel studies have illustrated the gradual process of seed size increase in cereals, a process that appears to occur in parallel with the evolution of non-shattering rachises (Asouti and Fuller, 2013; Fuller and Weisskopf, 2014; Fuller et al., 2017). The evolutionary selective pressures that have driven seed size increase and domestication broadly within cereals are still debated, with some leading theories being the deep burying of seeds during sowing or tilling, insularity linked to seed saving, and the releasing of evolutionary constraints to seed dispersal (Spengler, 2020). Additionally, developmental variation can become genetically coded for and may have led to larger seeds over time. Some evolutionary ecologists have suggested that plasticity more often than not paves the way for evolutionary change and the two routes that have been proposed for how this occurs are Baldwin's genetic accommodation (the Baldwin effect) or Waddington's genetic assimilation (Ancel, 2000; Badyaev, 2009). This latter possibility relies on the idea that the growth conditions in a cultivated field would favor either larger plants, which indirectly meant favoring larger seeds (Jones et al., 2021), or directly favoring larger seeds due to greater competition in highly dense fields (Harlan, 1995). The significant contrast between naked barley grain assemblages at Chap II and Chap I (Figure 8) could also indicate the wider presence of barley varieties. Barley varieties could constitute hulled or naked two-, six-, or four-rowed, compact, or hooded forms (Figure 9). Fuller et al. (2017) noted that the six-row barley tends to produce stubbier and plumper grains (p. 139), while two-row barleys tend to have fewer, larger grains relative to six-row barleys (Palmer et al., 2009). It is likely that the Chap I and Chap II sites contained different proportions of six- and two-row barley varieties. The dispersal of new varieties into Central Asia is an evolutionary process, as humans have an unparalleled ability to facilitate gene flow. The introgression of alleles for larger seeds or the replacement of one variety with another could drive seed size increases on a population scale.
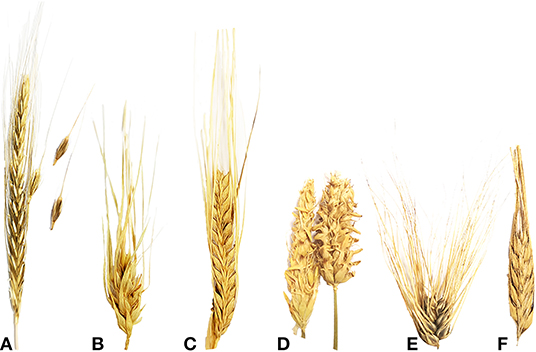
Figure 9. An example of highly diverse barley varieties: (A) wild two-row, (B) six-row naked, (C) two-row hulled, (D) hooded-naked, (E) six-row lax-eared naked, and (F) two-row hulled barleys.
The domestication process is compounding, and the selective pressures that humans impose on their cultivated crops are continually shifting. The variation in both natural and athropogenic selective pressures results in the formation of crop ecotypes or landraces. The evolutionary divergence of crops into specifically adapted forms tends to result from the genetic isolation of crops in a farmer's field over hundreds of plant generations. These plants can evolve to respond to colder clines in the north or differing elevations; they can also respond to differing cultivation practices. Thousands of landraces of barley were cultivated around the world prior to the past century. The variation in these landraces could express as regional forms in archaeobotanical assemblages or large-scale patterns across, for example, all of Central Asia. As one example, the shift from naked to hulled varieties of barley occurred across Central Asia during the first millennium BC (Spengler, 2015), a change in varieties that also resulted in an increase in seed size, as most hulled forms produce longer grains.
Another factor that may affect grain size change is agronomic selection. Silvertown (1989) highlighted four conditions for seed size under stabilizing selection: (1) mean seed size is constant; in other words, it is conservative for a given species and environment; (2) grain fitness is affected by its size; (3) seed size is heritable; and (4) there is a trade-off between seed number and its size. Sadras (2007) stated that the range in grain size could be a result of evolutionary and agronomic selection; in addition, environmental variability and genetic background of a given species also reflects seed size. Domestication, selection, and breeding is the result of fundamental plant transformation. Grain size plays a significant role in determining barley yield and malt quality; moreover, it is an important agronomic trait for human selection (Wang et al., 2019), as increasing grain size could increase the fitness of the plant (Sadras, 2007). Therefore, the fact that that the increase in grain size through time could be a natural agronomic selection and barley adaptation in highlands should be also taken into account.
Pleiotropy
Evolutionary selection for smaller grains as crops dispersed into novel ecologies may not necessarily have had anything to do with an advantage in possessing smaller seeds. For example, in order to withstand strong winds and rainstorms in the mountains, plant varieties with a more compact architecture may have inadvertently been selected due to their reduced rate of lodging, shorter stems, and ears that subsequently produce compact forms of grains. Knüpffer et al. (2003) noted that landraces of modern naked barleys collected from the remote high-mountain regions of Central Asia are short, with a thick, non-lodging straw, and grains that are more compact and spherical than those of taller barley varieties; likewise, in mountainous Japan, barley is small in order to withstand the monsoonal season of strong rains and winds. Interestingly, the earliest varieties of barley to disperse into Japan were not especially compact, potentially suggesting later adaptations (Leipe et al., 2017).
The argument that compact grains are a pleiotropic response to other evolutionary advantageous factors has already been made in Central Asia for wheat grains. This argument suggests that the highly compact morphotypes of wheat recovered from sites across Asia, dating to the second millennium BC, may be genetically linked to either Indian Dwarf Wheat or Japanese semidwarfing varieties (see Spengler, 2019 for a full discussion). While this remains an untested theory, a closer look at the evolutionary mechanisms leading to highly compact grain morphology in both landraces is informative to our discussion. The genetic coding for these highly compact mophotypes is different. Traditionally, the sphaerococcoid syndrome leading to the Indian and Pakistani populations of highly compact wheats was linked to the genotype ss (Koba, 1978; Salina et al., 2000), but recently, a mutation in the Tasg-D1-I gene leading to a single amino acid mutation has been implemented in a chain of pleiotropic changes across the plant (Cheng et al., 2020). While past research has shown that the rht alleles code for the Japanese and Korean historical semidwarfing wheat populations (Borojevic and Borojevic, 2005; Chen et al., 2012). These mutations are all different from the C allele that leads to compact wheats in some European landraces (Rao, 1972). Importantly, in neither case are the compact grains the evolutionarily selected trait. Historical observations suggest that the Indian Dwarf wheats were drought tolerant (Ellerton, 1939; Singh, 1946, 1987; Sears, 1947; Rao, 1977), and both populations of semidwarfing wheats expressed traits of increased tillering, more robust culms, and reduced lodging. In fact, much of the Green Revolution research conducted in Mexico and Italy focused on breeding varieties with compact vegetative architecture but without the highly compact grains (Reynolds and Borlaug, 2006; Salvi, 2013). Ultimately, the only feature of these compact wheat forms that archaeobotanists would be able to see is grain size, but natural or anthropogenic selection may have been driving overall changes in the plant with a reduction in grain size only representing a pleiotropic effect.
The pseudo-response regulator Ppd-H1 in barley is also associated with grain size but is likely to be an indirect effect through day-length sensitivity altering the developmental time of grain maturation (Fuller and Allaby, 2009). Therefore, one reason for the change in size in barley could, theoretically, be an association with the increase in Ppd-H1 non-sensitive barley normally associated with spring-sown varieties. Wild barley grasses are annuals, and they shed seeds after ripening in late summer or autumn, then regrow in the spring after responding to the increased day length and vernalization. When barley was moved to different latitudes and altitudes, the photoperiod sensitive genes, such as Ppd-H1, were silenced for better adaptation to novel climates allowing such barleys to be planted in the spring. Early Bronze Age barley in Central Asia could have been experiencing a selection process to becoming spring sown, while during the Iron Age, this proportion moved to a higher ratio in spring vs. autumn sown. Spring and autumn sowing have not been extensively discussed despite the fact that autumn-sown varieties normally do result in 20–30% higher yields in comparison to the spring barley, due to a longer developmental time (Špunar et al., 2002). During the early episodes of crop dispersal, early farmers might have planted their barley with or without Ppd-H1 gene silenced in spring. In the later periods, the ratio of winter to summer barleys might have changed, having an effect on average size increase in the caryopses.
Developmental Plasticity
Barley demonstrates wide-ranging ecological plasticity (a broad reaction norm) and tolerance to different stressors. Its wide range of ecological tolerance allows farmers to cultivate barley in very diverse environmental zones, from arid deserts to 4,500 masl (Knüpffer et al., 2003). Yet, change in environmental conditions induced by climate change may have had an important impact on agriculture development or caused farmers to move up or down in ecoclines (Hsu, 1998; Büntgen et al., 2011; Yang et al., 2020a). High-altitude ecosystems are highly sensitive to climate changes (Huang et al., 2014; Schwarz et al., 2017); thereby, the regional changes in precipitation and temperature patterns could directly affect the duration of the growing season or yields. Environmental conditions, including water availability and nutrients, directly influence the grain morphology in cereals (Giles, 1990; Blum, 1996, 2005; Knüpffer et al., 2003; Shah and Paulsen, 2003; Fuller et al., 2017; Motuzaite Matuzeviciute et al., 2018). Most crop plants express extreme developmental responses to factors, such as insufficient water supply or nutrients, through stunted growth and reduced yields, but unless the stress is extreme, the plant will not die (Bradshaw, 1965; Lacaze et al., 2009; Dahlin et al., 2020). Suboptimal growing conditions for cereal crops can result in smaller seeds (Seghatoleslami et al., 2008).
It has been shown that barley growing at higher altitudes in the mountains (between 2,400 and 3,400 masl) exhibits greater diversity in grain size than grains grown in regions where it is better adapted to specific sets of agroecological and microclimatic conditions (Asfaw, 2000; Motuzaite Matuzeviciute et al., 2020b). This greater range of variation, as we noted above, may be the result of highly variable seasons layered into a palimpsest of variation. An experimental study in Israel demonstrated links between grain size and water availability, by measuring gradual diversity along a rainfall gradient (Hübner et al., 2013). Likewise, experimental studies in rain-fed fields in northern Spain illustrated that barley grain weight was affected not only by drought stress but also by high temperature during the grain filling period; moreover, a plant might react only to one of these stresses or to both simultaneously (Voltas et al., 1999). In addition to known environmental variables such as precipitation, water availability, temperature, day length, nutrients, sunlight, altitude, and latitude, a plant's life cycle is influential for genotype responses (Voltas et al., 1999; Wiegmann et al., 2019; Dahlin et al., 2020). Smaller seed size in the early to mid-third millennium BC barley grains could have been affected by thermal and water stress at the stage of grains filling in the summer (Magliano et al., 2014).
Scholars have suggested that a reduction in the size of cereal grains in Europe after the first Neolithic wave may be linked to the biological constraints of the crops in more northerly climates (Fuller et al., 2017). These environmental constraints would have placed developmental pressures on cereal crops that might have uniformly resulted in small-statured plants and possibly smaller seeds. Developmental plasticity provides a buffer for plants as they disperse into novel environments or their local environment changes. Assuming their growth constraints are applied to the majority of the population and are felt relatively consistently across growing seasons, the palimpsest of the archaeological record could show these developmental responses as an overall seed size reduction. As Chap is located at the growing limits for modern cereal varieties, and we can assume prehistoric varieties were even less adapted to high elevations and altitudes, it is plausible to assume that the entire cultivated Chap population of the second millennium BC was expressing relatively uniform developmental constraints. Wiegmann et al. (2019) demonstrate that allelic variants of major barley flowering genes (Ppd-H1, Sdw1, Crn-H1, and Vrn-H3) react differently to different environmental cues. Barley phenotypic plasticity is common as well for plant elongation (plant height and spike length) as for grain characteristics (weight and size) (Hübner et al., 2013). Barley phenotypic plasticity can vary depending on what other crops or species are cultivated on the same field (Dahlin et al., 2020).
Agricultural development in the current territory of Kyrgyzstan is heavily dependent on irrigation due to insufficient precipitation during the summer months. Likewise, archaeobotanical research has shown the need for irrigation in the past (Motuzaite Matuzeviciute et al., 2021). The abundance of irrigation channels and terraced fields on the hilly slopes and mountain valleys of Kyrgyzstan shows the importance of artificially watering plants though irrigation systems (Rouse et al., 2021). Recent research at Wupaer (Yang et al., 2020b), located in the southwestern Tarim Basin, shows the importance of irrigation systems by 1,200 cal BC. Based on stable isotope analysis of the grains recovered at the site, water input could be one of the reasons for agricultural intensification in the Tarim Basin. Comparing archaeobotanical data from Chap I (Motuzaite Matuzeviciute et al., 2020b, 2021) and Wupaer (Yang et al., 2020b), crop diversification started at both sites at similar times during the end of the second to the first millennium BC. Crop diversification during the first millennium BC is also linked with the expansion and improvement of irrigation technologies (Miller et al., 2016). Therefore, it is hypothetically possible that the smaller and more compact barley varieties at Chap II during the third millennium BC uniformly received less water and possibly nutrients than barleys at Chap I during the early first millennium BC. Nonetheless, theoretically, irrigation would have served to mitigate environmental extremes and reduce variation due to plasticity among crops. Increases in grain size though time at Chap might indicate an increased reliance on irrigation or homogenously wetter climatic conditions. The studies on past climate in the Tien Shan region are not always helpful when trying to understand climatic differences between Chap II and Chap I sites as contrasting information is provided. Yet, some datasets tend to show dryer climate during the Chap II occupation and more humid during the Chap I (Hill, 2019; Leroy and Giralt, 2020; Tan et al., 2020).
How to Test Diachronic Seed Changes
There are several ways to clarify causes of diachronic seed size variation, especially if applied as multiproxy systems. In this paper, we only present measurement data, which could become a much more powerful tool with other supporting proxies. Stable isotope analysis of nitrogen and carbon could possibly indicate whether changes in the seeds are linked to changes in crop cultivation regimes connected with additional soil manuring, irrigation, or other factors, such as climatic change (Bogaard et al., 2007; Fraser et al., 2011; Vaiglova et al., 2014; Styring et al., 2017). Complementary evidence from zooarchaeological remains or cultural artifacts, such as cultivation tools, might imply that seeds were buried deeper to the ground and, by absorbing water and nutrients, could produce larger seedlings. Furthermore, archaeobotanical analysis and seed size measurements can also suggest changes in soil plowing and crop manuring regimes through time. Indeed, detailed archaeobotanical investigation at the Chap sites shows changes in agricultural strategies as seen from crop diversification, presence of water demanding legumes, and other plant hygrophilous species and the increase in weeds that flourish on nitrogen-rich soils and the identification of both summer and winter crop varieties (Motuzaite Matuzeviciute et al., 2020a, 2021, 2021).
If uncarbonized grains are found in the future, direct ancient DNA (aDNA) evidence for the factors causing seed change can be sought; in the meantime, better population-scale studies of distributions of semidwarfing forms of historical landraces across Asia could clarify some of these issues.
The detection of methylation signals in ancient DNA offers the opportunity to gain insight into the acclimation of past genomes to environments and to directly examine the evolutionary process of phenotypic plasticity in adapting to environmental conditions, while the identification of an ancient RNA virus genome in archaeological desiccated barley could indicate likely agent of stress (Smith et al., 2014).
Finally, while taking the measurements, most archaeobotanists just take the two variables length and width, while the grain depth is shown to be a major driver of shape change in domestication processes in grain (Hughes et al., 2019).
Conclusion
In our study, we present population-scale wheat and barley size data from Central Asia in an attempt to better contextualize the drivers that account for cereal grain size changes. Our data provide an important complement to European and southwest Asian databases, in that they represent a parallel and contemporaneous evolutionary trend in domesticated crops outside their natural habitat in the marginal regions of Central Asia. As considerable discussion regarding unique morphotypes in Central Asia and China has left unsolved archaeobotanical questions, we demonstrate that barley grains increased in size over time from compact to longer grained forms and that localized variation existed through time. While these data are not robust enough to pinpoint a single driving variable, we propose three plausible scenarios that could have led to the observed changes through time: (1) evolutionary adaptation (i.e., ecotypes or landraces), (2) pleiotropic linkages to other evolved adaptations, and (3) developmental plasticity (non-heritable adaptation). Evolutionary changes leading to localized adaptations can occur either through natural selection driving the formation of ecotypes or through gene flow leading to the introgression of genes from larger grained varieties introduced from southwest Asia in secondary waves of crop dispersal. The introduction of new crops may have been tied to different barley varieties of hulled, two-row and six-row, and spring and autumn sown. The formation of genetic varieties can occur through developmental responses to external factors, such as environmental fluctuations, climate, irrigation inputs, soil nutrients, pathologies, and seasonality. External factors acting on developmental or acclamatory responses in plants can be either natural or cultural. The trend of increasing grain size is seen across the wider regions of Central Asia in turn can be linked with better water and nutrient availability for plants during the first millennium BC that is indicative of advancing agricultural and irrigation technologies. The following factors could be further tested by conducting stable isotope analysis on measured seeds combining with the expanded analysis of archaeobotanical remains.
Data Availability Statement
The original contributions presented in the study are included in the article/Supplementary Material, further inquiries can be directed to the corresponding author/s.
Author Contributions
GM: researh design and writing, archaeological excavation, and archaeobotanical sample collection. BM-M: caryopses measurement, laboratory analysis, and writing the manuscript. RS: manuscript editing, contribution of caryopses measurements, and research design. All authors contributed to the article and approved the submitted version.
Funding
This project has received funding from European Social Fund (project No 09.3.3-LMT-712-01-0002) under grant agreement with the Research Council of Lithuania (LMTLT); The PI of the project is GM. Additional support provided by the European Research Council grant number 851102, FEDD.
Conflict of Interest
The authors declare that the research was conducted in the absence of any commercial or financial relationships that could be construed as a potential conflict of interest.
Supplementary Material
The Supplementary Material for this article can be found online at: https://www.frontiersin.org/articles/10.3389/fevo.2021.633634/full#supplementary-material
References
Abbo, S., and Gopher, A. (2020). Plant domestication in the Neolithic Near East: the humans-plants liaison. Quat. Sci. Rev. 242:106412. doi: 10.1016/j.quascirev.2020.106412
Ancel, L. W. (2000). Undermining the baldwin expediting effect: does phenotypic plasticity accelerate evolution? Theor. Popul. Biol. 58, 307–319. doi: 10.1006/tpbi.2000.1484
Asfaw, Z. (2000). “Barleys of Ethiopia,” in Genes in the field: on-farm conservation of crop diversity., ed. B. B. Stephen (Boca Raton/Ottawa: Lewis Publishers/International Plant Genetic Resources Institute), 77–108.
Asouti, E., and Fuller, D. Q. (2013). A contextual approach to the emergence of agriculture in Southwest Asia. Curr. Anthropol. 54, 299–345. doi: 10.1086/670679
Badyaev, A. V. (2009). Evolutionary significance of phenotypic accommodation in novel environments: an empirical test of the Baldwin effect. Philos. Trans. R. Soc. B Biol. Sci. 364, 1125–1141. doi: 10.1098/rstb.2008.0285
Blum, A. (1996). “Crop responses to drought and the interpretation of adaptation,” in Drought Tolerance in Higher Plants: Genetical, Physiological and Molecular Biological Analysis, ed. E. Belhassen (Dordrecht: Springer Netherlands), 57–70. doi: 10.1007/978-94-017-1299-6_8
Blum, A. (2005). Drought resistance, water-use efficiency, and yield potential—are they compatible, dissonant, or mutually exclusive? Aust. J. Agric. Res. 56, 1159–1168. doi: 10.1071/AR05069
Bogaard, A., Heaton, T. H. E., Poulton, P., and Merbach, I. (2007). The impact of manuring on nitrogen isotope ratios in cereals: archaeological implications for reconstruction of diet and crop management practices. J. Archaeol. Sci. 34, 335–343. doi: 10.1016/j.jas.2006.04.009
Borojevic, K., and Borojevic, K. (2005). The transfer and history of “reduced height genes” (Rht) in wheat from Japan to Europe. J. Hered. 96, 455–459. doi: 10.1093/jhered/esi060
Bradshaw, A. D. (1965). Evolutionary significance of phenotypic plasticity in plants. Adv. Genet. 13, 115–155. doi: 10.1016/S0065-2660(08)60048-6
Büntgen, U., Tegel, W., Nicolussi, K., McCormick, M., Frank, D., Trouet, V., et al. (2011). 2500 years of european climate variability and human susceptibility. Science 331, 578 −582. doi: 10.1126/science.1197175
Chen, G., Zheng, Q., Bao, Y., Liu, S., Wang, H., and Li, X. (2012). Molecular cytogenetic identification of a novel dwarf wheat line with introgressed Thinopyrum ponticum chromatin. J. Biosci. 37, 149–155. doi: 10.1007/s12038-011-9175-1
Cheng, X., Xin, M., Xu, R., Chen, Z., Cai, W., Chai, L., et al. (2020). A single amino acid substitution in STKc_GSK3 kinase conferring semispherical grains and its implications for the origin of triticum sphaerococcum. Plant Cell 32, 923–934. doi: 10.1105/tpc.19.00580
Costantini, L. (1984). “The beginning of agriculture in the Kachi Plain: the evidence of Mehrgarh. in South Asian archaeology,” in 1981 : proceedings of the Sixth International Conference of the Association of South Asian Archaeologists in Western Europe (New York, NY: Cambridge University Press), 29–33.
Dahlin, I., Kiær, L. P., Bergkvist, G., Weih, M., and Ninkovic, V. (2020). Plasticity of barley in response to plant neighbors in cultivar mixtures. Plant Soil 447, 537–551. doi: 10.1007/s11104-019-04406-1
Dai, F., Nevo, E., Wu, D., Comadran, J., Zhou, M., Qiu, L., et al. (2012). Tibet is one of the centers of domestication of cultivated barley. Proc. Natl. Acad. Sci. U.S.A. 109, 16969–16973. doi: 10.1073/pnas.1215265109
Ellerton, S. (1939). The origin and geographical distribution ofTriticum sphaerococcum perc. and its cytogenetical behaviour in crosses withT. vulgare VILL. J. Genet. 38, 307–324. doi: 10.1007/BF02982176
Fraser, R. A., Bogaard, A., Heaton, T., Charles, M., Jones, G., Christensen, B. T., et al. (2011). Manuring and stable nitrogen isotope ratios in cereals and pulses: towards a new archaeobotanical approach to the inference of land use and dietary practices. J. Archaeol. Sci. 38, 2790–2804. doi: 10.1016/j.jas.2011.06.024
Fuller, D., Colledge, S., Murphy, C., and Stevens, C. (2017). “Sizing up cereal variation: patterns in grain evolution revealed in chronological and geographical comparisons,” in Miscelánea en homenaje a Lydia Zapata Peña (1965-2015), eds C. Murphy and C. Stevens (Bilbao: Universidad Del País Vasco), 131–149.
Fuller, D. Q., and Weisskopf, A. (2014). “Barley: origins and development,” in Encyclopedia of Global Archaeology (New York, NY: Springer New York), 763–766. doi: 10.1007/978-1-4419-0465-2_2168
Fuller, D. Q., and Allaby, R. (2009). “Seed dispersal and crop domestication: shattering, germination and seasonality in evolution under cultivation,” in Fruit Development and Seed Dispersal (Oxford: Wiley-Blackwell), 238–295. doi: 10.1002/9781444314557.ch7
Gepts, P. (2003). “Crop domestication as a long-term selection experiment,” in Plant Breeding Reviews, ed. J. Janick (Oxford: John Wiley & Sons, Inc.), 1–44. doi: 10.1002/9780470650288.ch1
Giles, B. E. (1990). The effects of variation in seed size on growth and reproduction in the wild barley Hordeum vulgare ssp. spontaneum. Heredity (Edinb). 64, 239–250. doi: 10.1038/hdy.1990.29
Groman-Yaroslavski, I., Weiss, E., and Nadel, D. (2016). Composite sickles and cereal harvesting methods at 23,000-years-Old Ohalo II, Israel. PLoS ONE 11:e0167151. doi: 10.1371/journal.pone.0167151
Harlan, J. (1995). The Living Fields: Our Agricultural Heritage. Cambridge: Cambridge University Press.
Harris, D. (2010). Origins of Agriculture in Western Central Asia: An Environmental-Archaeological Study. Philadelphia, PA:University of Pennsylvania Museum of Archaeology and Anthropology
Hill, D. J. (2019). “Climate change and the rise of the central asian silk roads,” in Socio-Environmental Dynamics Along the Historical Silk Road, eds. Y. L., B. HR., F. X., and M. S. (Cham: Springer International Publishing), 247–259. doi: 10.1007/978-3-030-00728-7_12
Hsu, K. J. (1998). Sun, climate, hunger, and mass migration. Sci. China Ser. D Earth Sci. 41, 449–472. doi: 10.1007/BF02877737
Huang, X., Oberhänsli, H., von Suchodoletz, H., Prasad, S., Sorrel, P., Plessen, B., et al. (2014). Hydrological changes in western Central Asia (Kyrgyzstan) during the Holocene as inferred from a palaeolimnological study in lake Son Kul. Quat. Sci. Rev. 103, 134–152. doi: 10.1016/j.quascirev.2014.09.012
Hübner, S., Bdolach, E., Ein-Gedy, S., Schmid, K. J., Korol, A., and Fridman, E. (2013). Phenotypic landscapes: phenological patterns in wild and cultivated barley. J. Evol. Biol. 26, 163–174. doi: 10.1111/jeb.12043
Hughes, N., Oliveira, H. R., Fradgley, N., Corke, F. M. K., Cockram, J., Doonan, J. H., et al. (2019). μCT trait analysis reveals morphometric differences between domesticated temperate small grain cereals and their wild relatives. Plant J. 99, 98–111. doi: 10.1111/tpj.14312
Jones, G., Kluyver, T., Preece, C., Swarbrick, J., Forster, E., Wallace, M., et al. (2021). The origins of agriculture: intentions and consequences. J. Archaeol. Sci. 125:105290. doi: 10.1016/j.jas.2020.105290
Kistler, L., Maezumi, S. Y., Gregorio de Souza, J., Przelomska, N. A. S., Malaquias Costa, F., Smith, O., et al. (2018). Multiproxy evidence highlights a complex evolutionary legacy of maize in South America. Science 362, 1309–1313. doi: 10.1126/science.aav0207
Knüpffer, H., Terentyeva, I., Hammer, K., Kovaleva, O., and Sato, K. (2003). “Ecogeographical diversity – a Vavilovian approach,” in Developments in Plant Genetics and Breeding, 53–76. doi: 10.1016/S0168-7972(03)80006-3
Koba, T. (1978). Mapping of the s and Ch2 genes on chromosome 3D of common wheat. Wheat Inf Serv 48, 18–20.
Lacaze, X., Hayes, P. M., and Korol, A. (2009). Genetics of phenotypic plasticity: QTL analysis in barley, Hordeum vulgare. Heredity 102, 163–173. doi: 10.1038/hdy.2008.76
Leipe, C., Sergusheva, E. A., Müller, S., Spengler, R. N., Goslar, T., Kato, H., et al. (2017). Barley (Hordeum vulgare) in the Okhotsk culture (5th−10th century AD) of northern Japan and the role of cultivated plants in hunter–gatherer economies. PLoS ONE 12:e0174397. doi: 10.1371/journal.pone.0174397
Leroy, S. A., and Giralt, S. R. (2020). Humid and cold periods in the last 5600 years in Arid Central Asia revealed by palynology of Picea schrenkiana from Issyk-Kul. Holocene 31, 380–391. doi: 10.1177/0959683620972776
Li, L.-F., and Olsen, K. M. (2016). To have and to hold. Curr. Top. Dev. Biol. 119, 63–109. doi: 10.1016/bs.ctdb.2016.02.002
Lister, D. L., Jones, H., Oliveira, H. R., Petrie, C. A., Liu, X., Cockram, J., et al. (2018). Barley heads east: genetic analyses reveal routes of spread through diverse Eurasian landscapes. PLoS ONE 13:e0196652. doi: 10.1371/journal.pone.0196652
Lister, D. L., and Jones, M. K. (2013). Is naked barley an eastern or a western crop? The combined evidence of archaeobotany and genetics. Veg. Hist. Archaeobot. 22, 439–446. doi: 10.1007/s00334-012-0376-9
Liu, X., Lister, D. L., Zhao, Z., Petrie, C. A., Zeng, X., Jones, P. J., et al. (2017). Journey to the east: diverse routes and variable flowering times for wheat and barley en route to prehistoric China. PLoS ONE 12:e0187405. doi: 10.1371/journal.pone.0187405
Maeda, O., Lucas, L., Silva, F., Tanno, K.-I., and Fuller, D. Q. (2016). Narrowing the harvest: Increasing sickle investment and the rise of domesticated cereal agriculture in the Fertile Crescent. Quat. Sci. Rev. 145, 226–237. doi: 10.1016/j.quascirev.2016.05.032
Magliano, P. N., Prystupa, P., and Gutiérrez-Boem, F. H. (2014). Protein content of grains of different size fractions in malting barley. J. Inst. Brew. 120, 347–352. doi: 10.1002/jib.161
Mayr, E. (1942). Systematics and the Origin of Species from the Viewpoint of a Zoologist — Ernst Mayr. Cambridge: Cambridge University Press.
Miller, N. F., Spengler, R. N., and Frachetti, M. (2016). Millet cultivation across Eurasia: origins, spread, and the influence of seasonal climate. Holocene 26, 1566–1575. doi: 10.1177/0959683616641742
Motuzaite Matuzeviciute, G., Abdykanova, A., Kume, S., Nishiaki, Y., and Tabaldiev, K. (2018). The effect of geographical margins on cereal grain size variation: case study for highlands of Kyrgyzstan. J. Archaeol. Sci. Rep. 20, 400–410. doi: 10.1016/j.jasrep.2018.04.037
Motuzaite Matuzeviciute, G., Hermes, T. R., Mir-Makhamad, B., and Tabaldiev, K. (2020a). Southwest Asian cereal crops facilitated high-elevation agriculture in the central Tien Shan during the mid-third millennium BCE. PLoS ONE 15:e0229372. doi: 10.1371/journal.pone.0229372
Motuzaite Matuzeviciute, G., Mir-Makhamad, B., and Tabaldiev, K. (2021). First comprehensive archaeobotanical analysis of prehistoric agriculture in Kyrgyzstan. Veg. Hist. Archaeobot. doi: 10.1007/s00334-021-00827-0
Motuzaite Matuzeviciute, G., Tabaldiev, K., Hermes, T., Ananyevskaya, E., Grikpedis, M., Luneau, E., et al. (2020b). High-altitude agro-pastoralism in the kyrgyz tien shan: new excavations of the chap farmstead (1065–825 cal b.c.). J. F. Archaeol. 45, 29–45. doi: 10.1080/00934690.2019.1672128
Murphy, D. J. (2007). People, Plants and Genes: The Story of Crops and Humanity. Oxford: OUP Oxford.
Palmer, S. A., Moore, J. D., Clapham, A. J., Rose, P., and Allaby, R. G. (2009). Archaeogenetic evidence of ancient nubian barley evolution from six to two-row indicates local adaptation. PLoS ONE 4:e6301. doi: 10.1371/journal.pone.0006301
Pourkheirandish, M., Hensel, G., Kilian, B., Senthil, N., Chen, G., Sameri, M., et al. (2015). Evolution of the grain dispersal system in barley. Cell 162, 527–539. doi: 10.1016/j.cell.2015.07.002
Rao, P. (1977). Mapping of the sphaerococcum gene'S'on chromosome 3D of wheat. Cereal Res. Commun. 5, 15–17.
Reynolds, M. P., and Borlaug, N. E. (2006). Impacts of breeding on international collaborative wheat improvement. J. Agric. Sci. 144, 3–17. doi: 10.1017/S0021859606005867
Riehl, S. (2019). “Barley in Archaeology and Early History,” in Oxford Research Encyclopedia of Environmental Science (Oxford: Oxford University Press). doi: 10.1093/acrefore/9780199389414.013.219
Rouse, L. M., Tabaldiev, K., and Motuzaite Matuzeviciute, G. (2021). Exploring landscape archaeology and UAV-based survey in the Kochkor Valley, Kyrgyzstan: Results from the Kok-Sai alluvial slope. J. F. Archaeol
Sadras, V. O. (2007). Evolutionary aspects of the trade-off between seed size and number in crops. F. Crop. Res. 100, 125–138. doi: 10.1016/j.fcr.2006.07.004
Salina, E., Börner, A., Leonova, I., Korzun, V., Laikova, L., Maystrenko, O., et al. (2000). Microsatellite mapping of the induced sphaerococcoid mutation genes in Triticum aestivum. Theor. Appl. Genet. 100, 686–689. doi: 10.1007/s001220051340
Salvi, S. (2013). Ipotesi sulle origini del “grano di Rieti (Hypothesis on the origins of the “grain of Rieti”). Propos. e Ric. 36 71, 233–238
Schwarz, A., Turner, F., Lauterbach, S., Plessen, B., Krahn, K. J., Glodniok, S., et al. (2017). Mid- to late Holocene climate-driven regime shifts inferred from diatom, ostracod and stable isotope records from Lake Son Kol (Central Tian Shan, Kyrgyzstan). Quat. Sci. Rev. 177, 340–356. doi: 10.1016/j.quascirev.2017.10.009
Seghatoleslami, M. J., Kafi, M., and Majidi, E. (2008). Effect of drought stress at different growth stages on yield and water use efficiency of five proso millet (Panicum miliaceum L.) genotypes. Pakistan J. Bot. 40, 1427–1432
Shah, N. H., and Paulsen, G. M. (2003). Interaction of drought and high temperature on photosynthesis and grain-filling of wheat. Plant Soil 257, 219–226. doi: 10.1023/A:1026237816578
Silvertown, J. (1989). The paradox of seed size and adaptation. Trends Ecol. Evol. 4, 24–26. doi: 10.1016/0169-5347(89)90013-X
Smith, O., Clapham, A. J., Rose, P., Liu, Y., Wang, J., and Allaby, R. G. (2014). Genomic methylation patterns in archaeological barley show de-methylation as a time-dependent diagenetic process. Sci. Rep. 4, 5559. doi: 10.1038/srep05559
Spengler, R. N. (2013). Botanical resource use in the Bronze and Iron Age of the Central Eurasian mountain/steppe interface: decision making in multiresource pastoral economies
Spengler, R. N. (2015). Agriculture in the Central Asian Bronze Age. J. World Prehistory 28, 215–253. doi: 10.1007/s10963-015-9087-3
Spengler, R. N. (2019). Fruit from the Sands. Oakland, California: University of California Press Available at: http://www.jstor.org/stable/10.2307/j.ctvh1dx4s
Spengler, R. N. (2020). Anthropogenic Seed Dispersal: Rethinking the Origins of Plant Domestication. Trends Plant Sci. 25, 340–348. doi: 10.1016/j.tplants.2020.01.005
Spengler, R. N., Cerasetti, B., Tengberg, M., Cattani, M., and Rouse, L. M. (2014a). Agriculturalists and pastoralists: Bronze Age economy of the Murghab alluvial fan, southern Central Asia. Veg. Hist. Archaeobot. 23, 805–820. doi: 10.1007/s00334-014-0448-0
Spengler, R. N., de Nigris, I., Cerasetti, B., Carra, M., and Rouse, L. M. (2018). The breadth of dietary economy in Bronze Age Central Asia: Case study from Adji Kui 1 in the Murghab region of Turkmenistan. J. Archaeol. Sci. Reports 22, 372–381. doi: 10.1016/j.jasrep.2016.03.029
Spengler, R. N., Frachetti, M., Doumani, P., Rouse, L., Cerasetti, B., Bullion, E., et al. (2014b). Early agriculture and crop transmission among Bronze Age mobile pastoralists of Central Eurasia. Proc. R. Soc. B Biol. Sci. 281, 20133382. doi: 10.1098/rspb.2013.3382
Spengler, R. N., and Willcox, G. (2013). Archaeobotanical results from Sarazm, Tajikistan, an Early Bronze Age Settlement on the edge: Agriculture and exchange. Environ. Archaeol. 18, 211–221. doi: 10.1179/1749631413Y.0000000008
Špunar, J., Vaculová, K., Špunarov,á, M., and Nesvadba, Z. (2002). Comparison of important parameters of spring and winter barley cultivated in sugar beet production area of Czech Republic. Plant, Soil Environ. 48, 237–242. doi: 10.17221/4233-PSE
Stevens, C. J., Shelach-Lavi, G., Zhang, H., Teng, M., and Fuller, D. Q. (2021). A model for the domestication of Panicum miliaceum (common, proso or broomcorn millet) in China. Veg. Hist. Archaeobot. 30, 21–33. doi: 10.1007/s00334-020-00804-z
Styring, A. K., Charles, M., Fantone, F., Hald, M. M., McMahon, A., Meadow, R. H., et al. (2017). Isotope evidence for agricultural extensification reveals how the world's first cities were fed. Nat. Plants 3, 17076. doi: 10.1038/nplants.2017.76
Tan, L., Dong, G., An, Z., Lawrence Edwards, R., Li, H., Li, D., et al. (2020). Megadrought and cultural exchange along the proto-silk road. Sci. Bull. 66, 603–611. doi: 10.1016/j.scib.2020.10.011
Tang, L., Lu, H., Song, J., Wangdue, S., Chen, X., Zhang, Z., et al. (2020). The transition to a barley-dominant cultivation system in Tibet: First millennium BC archaeobotanical evidence from Bangga. J. Anthropol. Archaeol. 61, 101242. doi: 10.1016/j.jaa.2020.101242
Vaiglova, P., Bogaard, A., Collins, M., Cavanagh, W., Mee, C., Renard, J., et al. (2014). An integrated stable isotope study of plants and animals from Kouphovouno, southern Greece: a new look at Neolithic farming. J. Archaeol. Sci. 42, 201–215. doi: 10.1016/j.jas.2013.10.023
Voltas, J., van Eeuwijk, F. A., Araus, J. L., and Romagosa, I. (1999). Integrating statistical and ecophysiological analyses of genotype by environment interaction for grain filling of barley II. F. Crop. Res. 62, 75–84. doi: 10.1016/S0378-4290(99)00007-6
Wang, Q., Sun, G., Ren, X., Du, B., Cheng, Y., Wang, Y., et al. (2019). Dissecting the Genetic Basis of Grain Size and Weight in Barley (Hordeum vulgare L.) by QTL and Comparative Genetic Analyses. Front. Plant Sci. 10. doi: 10.3389/fpls.2019.00469
Wiegmann, M., Maurer, A., Pham, A., March, T. J., Al-Abdallat, A., Thomas, W. T. B., et al. (2019). Barley yield formation under abiotic stress depends on the interplay between flowering time genes and environmental cues. Sci. Rep. 9, 6397. doi: 10.1038/s41598-019-42673-1
Willcox, G. (2004). Measuring grain size and identifying Near Eastern cereal domestication: evidence from the Euphrates valley. J. Archaeol. Sci. 31, 145–150. doi: 10.1016/j.jas.2003.07.003
Willcox, G., and Stordeur, D. (2012). Large-scale cereal processing before domestication during the tenth millennium cal BC in northern Syria. Antiquity 86, 99–114. doi: 10.1017/S0003598X00062487
Yang, L., Shi, Z., Zhang, S., and Lee, H. F. (2020a). Climate Change, Geopolitics, and Human Settlements in the Hexi Corridor over the Last 5,000 Years. Acta Geol. Sin. - English Ed. 94, 612–623. doi: 10.1111/1755-6724.14529
Yang, Q., Zhou, X., Spengler, R. N., Zhao, K., Liu, J., Bao, Y., et al. (2020b). Prehistoric agriculture and social structure in the southwestern Tarim Basin: multiproxy analyses at Wupaer. Sci. Rep. 10, 14235. doi: 10.1038/s41598-020-70515-y
Zeng, X., Guo, Y., Xu, Q., Mascher, M., Guo, G., Li, S., et al. (2018). Origin and evolution of qingke barley in Tibet. Nat. Commun. 9, 5433. doi: 10.1038/s41467-018-07920-5
Zeng, X., Long, H., Wang, Z., Zhao, S., Tang, Y., Huang, Z., et al. (2015). The draft genome of Tibetan hulless barley reveals adaptive patterns to the high stressful Tibetan Plateau. Proc. Natl. Acad. Sci. 112, 1095–1100. doi: 10.1073/pnas.1423628112
Zhou, X., Yu, J., Spengler, R. N., Shen, H., Zhao, K., Ge, J., et al. (2020). 5,200-year-old cereal grains from the eastern Altai Mountains redate the trans-Eurasian crop exchange. Nat. Plants 6, 78–87. doi: 10.1038/s41477-019-0581-y
Zohary, D. (2004). Unconscious Selection and the Evolution of Domesticated Plants. Econ. Bot. 58, 5–10. doi: 10.1663/0013-0001(2004)058[0005:USATEO]2.0.CO;2
Keywords: cereal morphology, archaeobotany (palaeoethnobotany), grain size, high elevation agriculture, developmental plasticity, Central Asia, geographical margins, barley grain measurements
Citation: Motuzaite Matuzeviciute G, Mir-Makhamad B and Spengler RN III (2021) Interpreting Diachronic Size Variation in Prehistoric Central Asian Cereal Grains. Front. Ecol. Evol. 9:633634. doi: 10.3389/fevo.2021.633634
Received: 27 November 2020; Accepted: 18 February 2021;
Published: 29 April 2021.
Edited by:
Gianluca Piovesan, University of Tuscia, ItalyReviewed by:
Anna Maria Mercuri, University of Modena and Reggio Emilia, ItalyMark Nesbitt, Royal Botanic Gardens, Kew, United Kingdom
Copyright © 2021 Motuzaite Matuzeviciute, Mir-Makhamad and Spengler. This is an open-access article distributed under the terms of the Creative Commons Attribution License (CC BY). The use, distribution or reproduction in other forums is permitted, provided the original author(s) and the copyright owner(s) are credited and that the original publication in this journal is cited, in accordance with accepted academic practice. No use, distribution or reproduction is permitted which does not comply with these terms.
*Correspondence: Basira Mir-Makhamad, bWlybWFraGFtYWRAc2hoLm1wZy5kZQ==