- Department of Biology, University of Padova, Padova, Italy
In many arthropods, the appearance of new segments and their differentiation are not completed by the end of embryogenesis but continue, in different form and degree, well after hatching, in some cases up to the last post-embryonic molt. Focusing on the segmentation process currently described as post-embryonic segment addition (or, anamorphosis), we revise here the current knowledge and discuss it in an evolutionary framework which involves data from fossils, comparative morphology of extant taxa and gene expression. We advise that for a better understanding of the developmental changes underlying the evolution of arthropod segmentation, some key concepts should be applied in a critical way. These include the notion of the segment as a body block and the idea that hatching represents a well-defined divide, shared by all arthropods, between two contrasting developmental phases, embryonic vs. post-embryonic. This eventually reveals the complexity of the developmental processes occurring across hatching, which can evolve in different directions and with a different pace, creating the observed vagueness of the embryonic/post-embryonic divide.
Introduction
In many arthropods, production and differentiation of new segments are not completed by the end of embryogenesis but continue, in different form and degree, well after hatching, in some cases up to the last post-embryonic molt.
The post-embryonic addition of new segments is called anamorphosis and the taxa that present this mode of development are said to exhibit anamorphic development. Alternative to this developmental mode is epimorphic development, where the number of segments remains constant throughout the whole post-embryonic life. Completing the spectrum of options for the ontogenetic variation in the number of segments, there is the much less common process of desegmentation (or regressive segmentation), where the number of segments decreases at some point of the post-embryonic development; this is limited to a few holometabolous insects (Minelli and Fusco, 2013). Post-embryonic segment addition is not necessarily limited to a reproductively immature condition or to a larval phase, when present.
Anamorphosis: Numbers and Modes
Segmentation is a combination of multiple developmental processes that span from the first expression of segmentation genes to the complete display of all the morphological features of a mature segmental body unit. Since segmental units undergo developmental patterning (which may involve size, shape, limb formation, etc.), the “segmental stage” at which a segment can be considered “laid down” is an arbitrary choice. For instance, in the anostracan crustacean Artemia, this was identified either with the “segmental stage c,” at which the segment has the shape of a short cylinder (Weisz, 1946), or with the appearance of a stripe of Engrailed protein at the prospective posterior boundary of the segment (Williams et al., 2012).
For our comparative purposes, we count as developmental addition of a new segment the first morphological appearance of a segmental unit as traditionally recognized by descriptive morphology (not necessarily the same for all taxa), irrespective of how close it is to its final morphology (e.g., disregarding presence/absence of limb buds). We calculated a degree of anamorphosis as the percentage of segments that are added during post-embryonic life, from 0% in epimorphic taxa, to >95% in the longest millipedes (see Supplementary Table 1 for details on segment count).
Independent from the degree of anamorphosis, three main modes of anamorphosis are recognized, as first proposed by Enghoff et al. (1993) for millipedes. In euanamorphosis, segment number increases at each molt throughout the whole post-embryonic life, to terminate only with the death of the animal. In teloanamorphosis, segment number also increases throughout the animal's life, but both the number of molts and the schedule of segment addition at each molt are fixed for a given species and sex. Finally, in hemianamorphosis, the post-embryonic development includes a first anamorphic phase, through a first batch of stages (instars) separated by molts, followed by an epimorphic phase where molts take place without further increase in the number of body segments.
Taxonomic Survey
Anamorphosis in Extant Arthropods
The distribution of anamorphosis and epimorphosis in the main groups is shown in Figure 1 (reference to source data in Supplementary Table 1).
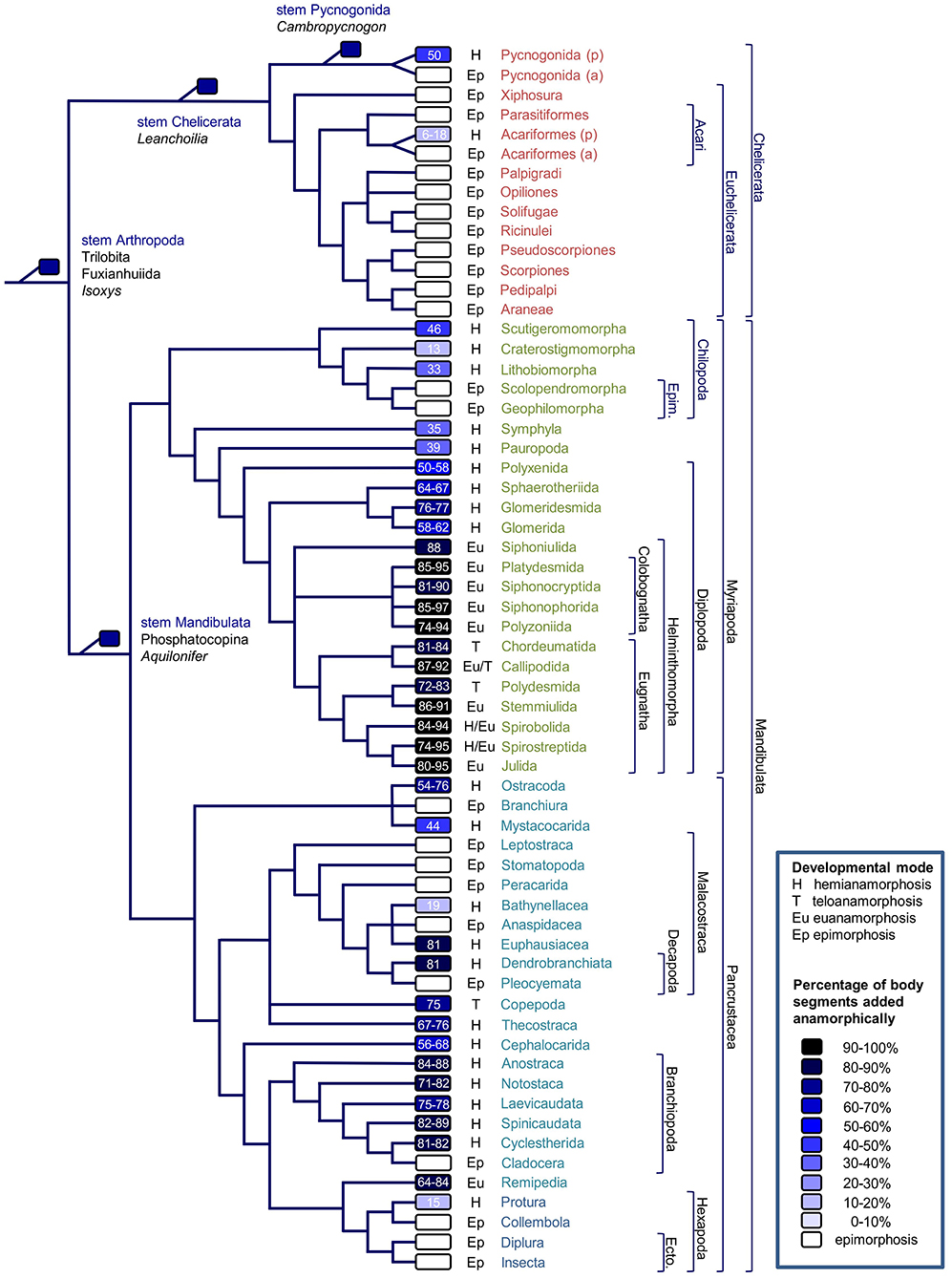
Figure 1. Phylogenetic distribution of segmentation modes in arthropods. Phylogeny based on Giribet and Edgecombe (2019), Chipman and Edgecombe (2019) (fossils), Howard et al. (2020) (Chelicerata), Fernández et al. (2016) (Myriapoda), and Schwentner et al. (2018) (Branchiopoda and Malacostraca). p, plesiomorphic condition; a, apomorphic condition; H, hemianamorphosis; T, teloanamorphosis; Eu, eunanamorphosis; Ep, epimorphosis; Epim., Epimorpha; Ecto., Ectognatha. Color of boxes and figures inside each box (percentage of body segments added post-embryonically) express the degree of anamorphosis (quantified only for extant taxa). In case of variation at lower taxonomic level, data refers to the most common or to the hypothesized plesiomorphic condition in the taxon. Details in Supplementary Table 1.
In Chelicerata, hemianamorphosis is found among the Pycnogonida, which are sister to all the other Chelicerata, the Euchelicerata; these are all epimorphic to the exclusion of the Acariformes. Within the Pycnogonida and Acariformes, a few lineages have independently evolved epimorphic development (Lindquist, 1984; Brenneis and Arango, 2019).
Most myriapod lineages are hemianamorphic. Epimorphic development only occurs in the centipede clade rightly named Epimorpha, which includes the Scolopendromorpha and Geophilomorpha. Euanamorphosis and teloanamorphosis are found among the Helminthomorpha millipedes exclusively, where both modes may have evolved once or several times independently (Miyazawa et al., 2014).
Within the Pancrustacea, hemianamorphosis is the most common developmental mode among the “crustacean” (non-Hexapoda) lineages, but epimorphic development has evolved in some lineages, in association with direct development, whereas teloanamorphosis has possibly evolved in Copepoda (Huys, 2014) and euanamorphosis in Remipedia (Koenemann et al., 2009). Within the Hexapoda, only the Protura are hemianamorphic, while the Collembola, Diplura, and Insecta are epimorphic.
Anamorphosis in Fossil Arthropods
Ontogenetic series are available for several fossil arthropods, both stem- and crown-group. Many of these show anamorphic development and hemianamorphosis seems to be the most common mode of segmentation among stem-group taxa (e.g., Fu et al., 2014, 2018). However, segmentation in these ancient forms also exhibits some distinctive features with respect to extant taxa. Many Phosphatocopina, interpreted either as stem-group Pancrustacea (Haug and Haug, 2015) or stem-group Mandibulata (Chipman and Edgecombe, 2019), were anamorphic with indirect development (Haug and Haug, 2015), hatching as so-called head larva. In contrast to modern anamorphic taxa, no segments were added with the first few molts, that is, anamorphosis was in some way delayed. Another peculiar feature of anamorphosis in these early forms was that, similar to trilobites, segments first emerged as dorsally non-articulated units forming a single shield, the pygidium. The most anterior pygidial segments developed articulation in successive stages, in a process that in trilobites is called segment release. Trilobita, variably assigned to stem-group arthropods, stem-group chelicerates or stem-group mandibulates (Giribet and Edgecombe, 2019), mostly developed hemianamorphically (Hughes et al., 2006). However, some Emuellidae, with more than 100 trunk segments as adults, were possibly euanamorphic (Paterson and Edgecombe, 2006), whereas Zhang and Clarkson (2009) made the case for an epimorphic eodiscoid species. Delayed anamorphosis might have characterized trilobite post-embryonic development as well. Evidence for an even earlier phase of cephalic segment addition (during the so-called phaselus stage, if this was actually a phase of trilobite ontogeny), is weak (Hughes et al., 2006).
Phylogenetic Patterns
Phylogenetic distribution of anamorphosis in extant taxa and information from extinct forms concur to indicate hemianamorphic development as the primitive condition in arthropods (Hughes et al., 2006; Minelli and Fusco, 2013; Miyazawa et al., 2014; Haug and Haug, 2015; Brenneis et al., 2017). Uncertainties on key nodes of arthropod phylogeny and incomplete information on post-embryonic segmentation in several taxa prevent a formal analysis of the evolution of this developmental character at the level of the whole clade. However, starting from the hypothesis of hemianamorphosis as the plesiomorphic condition and complementing the phylogenetic distribution of the character in Figure 1 with some available information at lower taxonomic level, four different evolutionary transitions can be recognized.
(i) Partial embryonization of segmentation (less anamorphic segments), with a consequent reduction in the degree of anamorphosis, seems to have occurred frequently. Millipedes usually have four trunk segments at hatching, but several species from different clades (Polyzoniida, Platydesmida, Julida, Stemmiulida, Spirobolida) hatch with more, up to 38 segments (Minelli, 2015; Supplementary Table 1). In centipedes, interpretation of the phylogenetic pattern crucially depends on the identity of the taxon that is sister to Epimorpha, either Lithobiomorpha or Craterostigmomorpha. In the first case, mainly supported by molecular data, from the primitive condition represented by Scutigeromorpha, there would have been a conspicuous embryonization of segmentation in Craterostigmomorpha firstly, followed by an opposite change in Lithobiomorpha and complete embryonization in Geophilomorpha. In the second case, mainly supported by morphological data (other than segmentation mode), a progressive embryonization from Scutigeromorpha to Epimorpha would have occurred. Among crustaceans, from a primitive condition of hatching as a nauplius larva, many lineages have independently evolved shorter anamorphic development, hatching as a more advanced-stage larva (e.g., metanauplius in Cephalocarida and Mystacocarida). This cannot generally be interpreted as a systemic heterochronic change, because different aspects of segmentation (segment appearance, segment patterning, or limb formation) and development of larval features (autonomous nutrition, locomotion, muscular, and nervous systems) are not necessarily associated (Fritsch et al., 2013; Haug and Haug, 2015; Jirikowski et al., 2015). Segmental patterning can even progress in the opposite direction with respect to segment addition, i.e., from posterior to anterior (Minelli, 2003, p. 162).
(ii) Complete embryonization of segmentation (epimorphosis) has evolved several times independently: at least in one trilobite species (Zhang and Clarkson, 2009), in some lineages of Pycnogonida (Brenneis et al., 2017), in Euchelicerata, in Epimorpha among the centipedes, in several lineages of Malacostraca (but see below), in Cladocera and twice among the Hexapoda, i.e., in Collembola and Ectognatha. In some cases, this process is associated with the evolution of direct from indirect development (many crustaceans) and a shortening of the metameric trunk (e.g., Branchiura and Cladocera). However, the opposite is observed in Geophilomorpha, where epimorphosis is associated with the most segment-rich trunks among the arthropods. It must also be noted that epimorphosis can evolve from anamorphosis not only by embryonization of the addition of most posterior segments, but also from the suppression of the addition of those segments (suppressed anamorphosis), as suggested for some lineages of Acariformes (Bochkov, 2009; Bolton et al., 2017).
(iii) Partial de-embryonization of sequential segmentation from an anamorphic condition (more segments produced by anamorphosis), with a consequent increase in the degree of anamorphosis, is apparently less common. Stem-group Pancrustacea hatched as head larvae of five segments, whereas the primitive condition for crown-group Pancrustacea is thought to be a four-segment nauplius (Haug and Haug, 2015). According to Scholtz (2000), Euphausiacea and Dendrobranchiata would have evolved a “new” nauplius secondarily (and in parallel) from primitive Malacostraca with shorter anamorphosis, but this has been questioned more recently (Akther et al., 2015; see also below). In centipedes, if Lithobiomorpha are actually sister to Epimorpha (see above), the former would have extended anamorphosis from a shorter Craterostigmomorpha-like condition.
(iv) Partial de-embryonization of embryonic sequential segmentation from epimorphosis (secondary anamorphosis), seems to be even more rare, and putative cases are uncertain. In Pycnogonida, some Nymphonidae might have returned to anamorphosis (Brenneis et al., 2017), but uncertainties on the phylogeny of epimorphic pycnogonids do not allow to resolve this transition with confidence. If Euchelicerata are primitively epimorphic, Acariformes would have evolved anamorphosis secondarily. However, due to the persisting instability of phylogenetic hypotheses about the major clades of Euchelicerata (Giribet and Edgecombe, 2019), it is not unparsimonious to hypothesize that the Acariformes simply retained the plesiomorphic chelicerate condition (Bochkov, 2009; Bolton et al., 2017). The phylogeny in Figure 1 would support epimorphosis as plesiomorphic for the Malacostraca, with secondary independent transition to anamorphosis in some derived taxa, compatible with the presence of a zoea-like larva as the plesiomorphic condition for the group (Jirikowski et al., 2015). However, in consideration of the similarities between the nauplii in anamorphic malacostracans and non-malacostracans and the differences in the direct development of epimorphic malacostracans, other authors have put forward the opposite hypothesis, i.e., the retention of the primitive condition of malacostracan anamorphic larval development in Bathynellacea, Euphausiacea, and Dendrobranchiata and its independent loss in the other malacostracan groups (Akther et al., 2015; Haug and Haug, 2015).
Anamophosis and epimorphosis are not fundamentally distinct developmental modes, the latter being only the lower extreme degree of the former. This is more than an arithmetic truism. In several clades, e.g., in decapod crustaceans, segment number is the same in anamorphic and epimorphic lineages. Among the most polymeric epimorphic clade, the Geophilomorpha, Brena and Akam (2013) discovered a minimal leftover of anamorphosis in the species Strigamia maritima, where 2–3 terminal segments (out of 48–54 trunk segments) are added after hatching, during the first embryoid stages (see below). However, the opposite evolutionary transitions, embryonization vs. de-embryonization of segment formation, might not have the same evolvability, the former having apparently occurred more often than the latter.
Genetics of Anamorphosis
In anamorphic development, as well as in embryonic sequential segmentation, the new segments appear sequentially in anteroposterior progression from a subterminal region referred to as “segment addition zone” (SAZ; Janssen et al., 2010). This is also often referred to as the proliferative (or generative, or growth) zone, but SAZ is to be preferred because it makes no assumption of localized and continuous cell proliferation in the posterior of the body (Clark et al., 2019; see also Fusco, 2005). However, information about morphogenesis and gene expression associated with anamorphosis is scarce, and current investigations are mainly concerned with the evolution of embryonic simultaneous segmentation from embryonic sequential segmentation in insects.
Evidence of a conserved role of the segment polarity gene engrailed during anamorphosis was found in the anostracan crustaceans Artemia and Thamnocephalus (Manzanares et al., 1993; Constantinou et al., 2020), in the thecostracan crustacean Sacculina (Gibert et al., 2000) and in the centipede Lithobius (Bortolin et al., 2011).
The involvement of Notch signaling is increasingly emerging as a common feature of sequential segmentation throughout the Bilateria. Williams et al. (2012) showed that blocking Notch signaling causes a specific, repeatable effect on segmentation in Artemia franciscana and Thamnocephalus platyurus, although the observation that loss-of-function Notch phenotypes differ significantly across arthropods suggests some variation in the role of Notch in the regulation of sequential segmentation.
Despite the paucity of experimental data on the developmental genetics of anamorphosis, some indirect information can be obtained from comparative studies on embryonic segmentation. In a certain way, the evolutionary embryonization of anamorphosis can be seen as a natural experiment, where post-embryonic segmentation, a process not easily accessible to current molecular methodologies, is brought under the eye of the investigator. The extended similarities found in embryonic sequential segmentation in lineages that independently evolved either complete or partial embryonization of segmentation can perhaps indicate a common basic mechanism among lineages with different degree of anamorphosis up to epimorphosis. This could be based on the same clock-and-wavefront mechanism inferred from data on embryonic segmentation in a small number of model species, and hypothesized to be ancestral and conserved among arthropods (Clark et al., 2019).
Anamorphosis in Context
Beyond the arbitrariness of what to count as the appearance of a new segment, the previous descriptions might suggest that anamorphosis is a well-defined phenomenon, and that its evolution can be confidently traced whenever reliable developmental and phylogenetic information is available. However, this is only a superficial view that can serve only broad comparative purposes. On a closer inspection, seeking for mechanistic explanations, anamorphosis remains surrounded by uncertainties that can be locally resolved only by overcoming the idealizations hidden in the traditional concepts of hatching, larva, and segment.
The Blurry Event of Hatching
It is not always the case that hatching separates embryonic from post-embryonic phases neatly. More or less embryo-like (embryoid) hatchlings are described for many arthropod groups, under a variety of taxon-specific terms (Minelli et al., 2006; Minelli and Fusco, 2013; Fritsch and Richter, 2015; Haug, 2020; Supplementary Table 1).
Focusing on taxonomic distribution and morphological and functional characteristics of these embryoid stages, three facts highlight the evolutionary flexibility of arthropod developmental schedules. First, conditions at hatching are often different between closely related taxa (e.g., in many spiders there is a pronymph with incompletely articulated appendages, but not in all). Second, this diversity is associated with a diversity in the number of molts the animal undergoes before and after the beginning of its active life. In most pterygote insects, three embryonic cuticles are shed before hatching, but only two in the cyclorrhaphous flies (Konopová and Zrzavý, 2005). Third, the condition at hatching is not necessarily correlated to segmentation schedule. For example, epimorphic hexapod hatchlings are anything between an active juvenile and a vermiform pronymph, while anamorphic myriapods hatch in conditions so different as the very active larva I of Lithobius and the motionless pupoid of Pauropus (Minelli et al., 2006).
Situated at one extreme of both embryonic and post-embryonic phases, where the methodologies used in the study of each phase are less effective, development around hatching time is little investigated, and recent work is disclosing unsuspected situations. For example, two embryoid stages were traditionally reported for the geophilomorph centipedes, whereas a recent closer scrutiny in Strigamia maritima revealed five stages (Brena, 2014).
The Multifaceted Larva
Many arthropods, in particular among the Pancrustacea, begin post-embryonic life as larvae. However, the term larva has been applied to immatures with very different, although non-mutually exclusive characteristics. These include forms that differ morphologically from the adult, have different ecological niches than the corresponding adult, or transform into an adult by a metamorphosis (see Haug (2020) for a detailed account), thus the qualification of development as either direct or indirect is somehow a matter of degree or requires qualitative specification (e.g., for some intermediate cases Fritsch et al. (2013) introduced the term pseudo-direct development). The evolution of post-embryonic segmentation, although potentially independent from other developmental features of juvenile stages, can be found to be variably associated to larval evolution, as for instance when the evolution of direct development coincides with a transition to epimorphosis.
The Complex Segment
Description and comparative analysis of anamorphosis assume that we are dealing with unambiguously countable units, the segments. However, not all putatively segmental structures (especially those of internal anatomy) are in register, as they can have different period or phase. Thus, a more realistic depiction of arthropod body organization is obtained by dissociating the serial homology of individual periodic structures (e.g., legs or sclerites), or segmentation, from the concept of the segment as a body module (e.g., Budd, 2001; Minelli and Fusco, 2004; Fusco, 2005, 2008; Fusco and Minelli, 2013; Hannibal and Patel, 2013). This accounts for the occurrence of so-called “segmental mismatch,” i.e., the discordance between different segmental series within the same animal, and of a number of segmental abnormalities (Leśniewska et al., 2009), but also for the high disparity in arthropod segmental patterns. The study of anamorphosis cannot disregard the complexity and the disparity of the segmentation process (Minelli, 2020).
Conclusions
We advise that for a better understanding of the developmental changes underlying the evolution of arthropod segmentation, some key concepts should be applied in a critical way.
The putative embryonic/post-embryonic divide suffers the same shortcomings shown by the traditional periodization of development (articulation in temporal units for comparative purposes) within each of the two main phases of arthropod development (Minelli et al., 2006). During embryonic development, periodization can either be based on absolute time from egg laying, on the fraction of elapsed embryonic time, or with reference to a series of events such as blastoderm formation, gastrulation, etc. During post-embryonic development, periodization is mainly based on temporal units delimited by molts, generally referred to as stages or instars. In both phases, some developmental events are employed to give temporal order to other events, but there is no biological foundation for one series of events to be recognized as “ordinator” and all other events as “ordered.” Periodization cannot be other than a relative framework, and the same is true for the passage from embryonic to post-embryonic life.
Evolutionary developmental biology seems to be over-preoccupied with boundaries, both in space (e.g., those between segments) and time (e.g., those between stages). However, these boundaries can easily hide both the continuity of many co-occurring developmental processes and the independence exhibited to a different degree by the same set of processes (Minelli et al., 2006). As an alternative, for instance, rather than defining embryonic development on the basis of its putative boundaries (fertilization, when the case, and hatching), it seems more sensible to define it based on “what it is,” that is as a special context for early developmental events, characterized by the fact that the latter run protected by the body of a parent (or a host) or by a shell, that are stabilized in physical parameters, occur in relatively small-size living systems, are supplied with energy and materials from the parent, etc. None of these features is necessary, nor sufficient for defining the embryonic phase, and each one can change in evolution with different direction and pace, creating the observed vagueness of the embryonic/post-embryonic divide. From this stance, recurrent embryonization and (although less frequently) de-embryonization of segmentation in evolution reveal the robustness of the developmental processes involved, able to work in contexts so different as an embryo and an active animal, where in many cases these processes can go on for years.
Evolution is about change, and to study evolutionary change we need flexible conceptual frameworks and data formats.
Author Contributions
Both authors listed have made a substantial, direct and intellectual contribution to the work, and approved it for publication.
Conflict of Interest
The authors declare that the research was conducted in the absence of any commercial or financial relationships that could be construed as a potential conflict of interest.
Acknowledgments
Jason Dunlop, Gregory D. Edgecombe, David A. Legg, Nigel C. Hughes, Jørgen Olesen, and Stefan Richter provided precious help in collecting and checking information on which the article is based. Jørgen Olesen, Stefan Richter, and the two reviewers provided useful comments on an earlier version of the ms.
Supplementary Material
The Supplementary Material for this article can be found online at: https://www.frontiersin.org/articles/10.3389/fevo.2021.622482/full#supplementary-material
References
Akther, H., Agersted, M. D., and Olesen, J. (2015). Naupliar and metanaupliar development of Thysanoessa raschii (Malacostraca, Euphausiacea) from Godthåbsfjord, Greenland, with a reinstatement of the ancestral status of the free-living nauplius in malacostracan evolution. PLoS ONE 10:e0141955. doi: 10.1371/journal.pone.0141955
Bochkov, A. V. (2009). A review of mites of the parvorder Eleutherengona (Acariformes: Prostigmata) – permanent parasites of mammals. Acarina 1, 1–149.
Bolton, S. J., Chetverikov, P. E., and Klompen, H. (2017). Morphological support for a clade comprising two vermiform mite lineages: Eriophyoidea (Acariformes) and Nematalycidae (Acariformes). Syst. Appl. Acarol. 22, 1096–1131. doi: 10.11158/saa.22.8.2
Bortolin, F., Benna, C., and Fusco, G. (2011). Gene expression during post-embryonic segmentation in the centipede Lithobius peregrinus (Chilopoda, Lithobiomorpha). Dev. Genes Evol. 221, 105–111 doi: 10.1007/s00427-011-0359-3
Brena, C. (2014). The embryoid development of Strigamia maritima and its bearing on post-embryonic segmentation of geophilomorph centipedes. Front. Zool. 11:58. doi: 10.1186/s12983-014-0058-9
Brena, C., and Akam, M. (2013). An analysis of segmentation dynamics throughout embryogenesis in the centipede Strigamia maritima. BMC Biol. 11:112. doi: 10.1186/1741-7007-11-112
Brenneis, G., and Arango, C. P. (2019). First description of epimorphic development in Antarctic Pallenopsidae (Arthropoda, Pycnogonida) with insights into the evolution of the four-articled sea spider cheliphore. Zool. Lett. 5:4. doi: 10.1186/s40851-018-0118-7
Brenneis, G., Bogomolova, E. V., Arango, C. P., and Krapp, F. (2017). From egg to no-body: an overview and revision of developmental pathways in the ancient arthropod lineage Pycnogonida. Front. Zool. 14:6. doi: 10.1186/s12983-017-0192-2
Budd, G. E. (2001). Why are arthropods segmented? Evol. Dev. 3, 332–342. doi: 10.1046/j.1525-142X.2001.01041.x
Chipman, A. D., and Edgecombe, G. D. (2019). Developing an integrated understanding of the evolution of arthropod segmentation using fossils and evo-devo. Proc. R. Soc. B 286:20191881. doi: 10.1098/rspb.2019.1881
Clark, E., Peel, A. D., and Akam, M. (2019). Arthropod segmentation. Development 146:dev170480. doi: 10.1242/dev.170480
Constantinou, S. J., Duan, N., Nagy, L. M., Chipman, A. D., and Williams, T. A. (2020). Elongation during segmentation shows axial variability, low mitotic rates, and synchronized cell cycle domains in the crustacean, Thamnocephalus platyurus. Evodevo 11:1. doi: 10.1186/s13227-020-0147-0
Enghoff, H., Dohle, W., and Blower, J. G. (1993). Anamorphosis in millipedes (Diplopoda) – the present state of knowledge with some developmental and phylogenetic considerations. Zool. J. Linn. Soc. 109, 103–234. doi: 10.1111/j.1096-3642.1993.tb00305.x
Fernández, R., Edgecombe, G. D., and Giribet, G. (2016). Exploring phylogenetic relationships within Myriapoda and the effects of matrix composition and occupancy on phylogenomic reconstruction. Syst. Biol. 65, 871–889. doi: 10.1093/sysbio/syw041
Fritsch, M., Bininda-Emonds, O. P., and Richter, S. (2013). Unraveling the origin of Cladocera by identifying heterochrony in the developmental sequences of Branchiopoda. Front. Zool. 10:35. doi: 10.1186/1742-9994-10-35
Fritsch, M., and Richter, S. (2015). How the cladoceran heterogonic life cycle evolved — insights from gamogenetic reproduction and direct development in Cyclestherida. Evol. Dev. 17, 356–366. doi: 10.1111/ede.12163
Fu, D., Ortega-Hernández, J., Daley, A. C., Zhang, X., and Shu, D. (2018). Anamorphic development and extended parental care in a 520 million-year-old stem-group euarthropod from China. BMC Evol. Biol. 18:147. doi: 10.1186/s12862-018-1262-6
Fu, D., Zhang, X., Budd, G. E., Liu, W., and Pand, X. (2014). Ontogeny and dimorphism of Isoxys auritus (Arthropoda) from the Early Cambrian Chengjiang biota, South China. Gondwana Res. 25, 975–982. doi: 10.1016/j.gr.2013.06.007
Fusco, G. (2005). Trunk segment numbers and sequential segmentation in myriapods. Evol. Dev. 7, 608–617. doi: 10.1111/j.1525-142X.2005.05064.x
Fusco, G. (2008). “Morphological nomenclature, between patterns and processes: segments and segmentation as a paradigmatic case,” in Updating the Linnaean Heritage: Names as Tools for Thinking about Animals and Plants, eds A. Minelli, L. Bonato, and G. Fusco (Auckland: Zootaxa 1950, Magnolia Press), 96–102. doi: 10.11646/zootaxa.1950.1.9
Fusco, G., and Minelli, A. (2013). “Arthropod segmentation and tagmosis,” in Arthropod Biology and Evolution. Molecules, Development, Morphology, eds A. Minelli, G. Boxshall, and G. Fusco (Berlin Heidelberg: Springer), 197–221. doi: 10.1007/978-3-662-45798-6_9
Gibert, J.-M., Mouchel-Vielh, E., Quéinnec, E., and Deutsch, J. S. (2000). Barnacle duplicate engrailed genes: divergent expression patterns and evidence for a vestigial abdomen. Evol. Dev. 2, 1–9. doi: 10.1046/j.1525-142x.2000.00059.x
Giribet, G., and Edgecombe, G. D. (2019). The phylogeny and evolutionary history of arthropods. Curr. Biol. 29, R592–R602. doi: 10.1016/j.cub.2019.04.057
Hannibal, R. L., and Patel, N. H. (2013). What is a segment? Evodevo 4:35. doi: 10.1186/2041-9139-4-35
Haug, J. T. (2020). Why the term “larva” is ambiguous, or what makes a larva? Acta Zool. 101, 167–188. doi: 10.1111/azo.12283
Haug, J. T., and Haug, C. (2015). “Crustacea”: comparative aspects of larval development,” in Evolutionary Developmental Biology of Invertebrates 4: Ecdysozoa II: Crustacea, ed A. Wanninger (Wien: Springer), 1–37. doi: 10.1007/978-3-7091-1853-5_1
Howard, R. J., Puttick, M. N., Edgecombe, G. D., and Lozano-Fernandez, J. (2020). Arachnid monophyly: morphological, palaeontological and molecular support for a single terrestrialization within Chelicerata. Arthropod Struct. Dev. 59:100997. doi: 10.1016/j.asd.2020.100997
Hughes, N. C., Minelli, A., and Fusco, G. (2006). The ontogeny of trilobite segmentation: a comparative approach. Paleobiology 32, 602–627. doi: 10.1666/06017.1
Huys, R. (2014). “Copepoda,” in Atlas of Crustacean Larvae, eds J. W. Martin, J. Olesen, and J. T. Høeg (Baltimore: The Johns Hopkins University Press), 144–163.
Janssen, R., Le Gouar, M., Pechmann, M., Poulin, F., Bolognesi, R., Schwager, E. E., et al. (2010). Conservation, loss, and redeployment of Wnt ligands in protostomes: implications for understanding the evolution of segment formation. BMC Evol. Biol. 10:374. doi: 10.1186/1471-2148-10-374
Jirikowski, G. J., Wolff, C., and Richter, S. (2015). Evolution of eumalacostracan development – new insights into loss and reacquisition of larval stages revealed by heterochrony analysis. Evodevo 6:4. doi: 10.1186/2041-9139-6-4
Koenemann, S., Olesen, J., Alwes, F., Iliffe, T. M., Hoenemann, M., Ungerer, P., et al. (2009). The post-embryonic development of Remipedia (Crustacea)— additional results and new insights. Dev. Genes Evol. 219, 131–145. doi: 10.1007/s00427-009-0273-0
Konopová, B., and Zrzavý, J. (2005). Ultrastructure, development, and homology of insect embryonic cuticles. J. Morphol. 264, 339–362. doi: 10.1002/jmor.10338
Leśniewska, M., Bonato, L., Minelli, A., and Fusco, G. (2009). Trunk anomalies in the centipede Stigmatogaster subterranea provide insight into late-embryonic segmentation. Arthropod Struct. Dev. 38, 417–426. doi: 10.1016/j.asd.2009.05.001
Lindquist, E. E. (1984). “Current theories on the evolution of major groups of Acari and on their relationship with other groups of Arachnida, with consequent implications for their classification,” in Acarology. Proceedings of the 6th International Congess of Acarology, eds D. A. Griffiths and C. E. Bowman (Chichester: Ellis Horwood), 28–62.
Manzanares, M., Marco, R., and Garesse, R. (1993). Genomic organization and developmental pattern of expression of the engrailed gene from the brine shrimp Artemia. Development 118, 1209–1219.
Minelli, A. (2003). The Development of Animal Form. Cambridge: Cambridge University Press. doi: 10.1017/CBO9780511541476
Minelli, A. (2020). Arthropod segments and segmentation – lessons from myriapods, and open questions. Opusc. Zool. Budapest 51, 7–21. doi: 10.18348/opzool.2020.S2.7
Minelli, A. (ed). (2015). Treatise on Zoology— Anatomy, Taxonomy, Biology. The Myriapoda, Vol 2. Leiden: Brill. doi: 10.1163/9789004188273
Minelli, A., Brena, C., Deflorian, G., Maruzzo, D., and Fusco, G. (2006). From embryo to adult. Beyond the conventional periodization of arthropod development. Dev. Genes Evol. 216, 373–383. doi: 10.1007/s00427-006-0075-6
Minelli, A., and Fusco, G. (2004). Evo-devo perspectives on segmentation: model organisms and beyond. Trends Ecol. Evol. 19, 423–429. doi: 10.1016/j.tree.2004.06.007
Minelli, A., and Fusco, G. (2013). “Arthropod post-embryonic development,” in Arthropod Biology and Evolution. Molecules, Development, Morphology, eds A. Minelli, G. Boxshall, and G. Fusco (Berlin Heidelberg: Springer), 91–122. doi: 10.1007/978-3-662-45798-6_5
Miyazawa, H., Ueda, C., Yahata, K., and Zhu, Z.-H. (2014). Molecular phylogeny of Myriapoda provides insights into evolutionary patterns of the mode in post-embryonic development. Sci. Rep. 4:4127. doi: 10.1038/srep04127
Paterson, J. R., and Edgecombe, G. D. (2006). The Early Cambrian trilobite family Emuellidae Pocock, 1970: systematic position and revision of Australian species. J. Paleontol. 80, 496–513. doi: 10.1666/0022-3360(2006)80[496:TECTFE]2.0.CO;2
Scholtz, G. (2000). Evolution of the nauplius stage in malacostracan crustaceans. J. Zool. Syst. Evol. Res. 38, 175–187. doi: 10.1046/j.1439-0469.2000.383151.x
Schwentner, M., Richter, S., Rogers, D. C., and Giribet, G. (2018). Tetraconatan phylogeny with special focus on Malacostraca and Branchiopoda: highlighting the strength of taxon-specific matrices in phylogenomics. Proc. R. Soc. B Biol. Sci. 285:20181524. doi: 10.1098/rspb.2018.1524
Weisz, P. B. (1946). The space-time pattern of segment formation in Artemia salina. Biol. Bull. 91, 119–140. doi: 10.2307/1538255
Williams, T., Blachuta, B., Hegna, T. A., and Nagy, L. M. (2012). Decoupling elongation and segmentation: Notch involvement in anostracan crustacean segmentation. Evol. Dev. 14, 372–382. doi: 10.1111/j.1525-142X.2012.00555.x
Keywords: anamorphosis, comparative analysis, development, evolvability, fossils, hatching, gene expression, phylogeny
Citation: Fusco G and Minelli A (2021) The Development of Arthropod Segmentation Across the Embryonic/Post-embryonic Divide – An Evolutionary Perspective. Front. Ecol. Evol. 9:622482. doi: 10.3389/fevo.2021.622482
Received: 28 October 2020; Accepted: 06 January 2021;
Published: 28 January 2021.
Edited by:
Nico Posnien, University of Göttingen, GermanyReviewed by:
Ariel D. Chipman, Hebrew University of Jerusalem, IsraelAnna Schoenauer, Oxford Brookes University, United Kingdom
Copyright © 2021 Fusco and Minelli. This is an open-access article distributed under the terms of the Creative Commons Attribution License (CC BY). The use, distribution or reproduction in other forums is permitted, provided the original author(s) and the copyright owner(s) are credited and that the original publication in this journal is cited, in accordance with accepted academic practice. No use, distribution or reproduction is permitted which does not comply with these terms.
*Correspondence: Giuseppe Fusco, Z2l1c2VwcGUuZnVzY29AdW5pcGQuaXQ=