- Biology Department, James Madison University, Harrisonburg, VA, United States
Skeleton plays a huge role in understanding how vertebrate animals have diversified in phylogeny, ecology and behavior. Recent evo-devo research has used ossification sequences to compare skeletal development among major groups, to identify conserved and labile aspects of a sequence within a group, to derive ancestral and modal sequences, and to look for modularity based on embryonic origin and type of bone. However, questions remain about how to detect and order bone appearances, the adaptive significance of ossification sequences and their relationship to adult function, and the utility of categorizing bones by embryonic origin and type. Also, the singular focus on bone appearances and the omission of other tissues and behavioral, ecological and life history events limit the relevance of such analyses. Amphibians accentuate these concerns because of their highly specialized biphasic life histories and the exceptionally late timing, and high variability of their ossification sequences. Amphibians demonstrate a need for a whole-animal, whole-ontogeny approach that integrates the entire ossification process with physiology, behavior and ecology. I discuss evidence and hypotheses for how hormone mediation and calcium physiology might elicit non-adaptive variability in ossification sequence, and for adaptive strategies to partition larval habitats using bone to offset the buoyancy created by lung use. I also argue that understanding plasticity in ossification requires shifting focus away from embryonic development and adult function, and toward postembryonic mechanisms of regulating skeletal growth, especially ones that respond directly to midlife environments and behaviors.
Introduction
Ossification sequence, meaning the order by which bones appear in a species, has recently become the subject of much evo-devo study and phylogenetic analysis. Amphibians feature prominently in this research owing to the large number of species for which these data have been collected (over 60 at last count) and their biphasic life cycles, which entail dramatic transformations in morphology and behavior midway through life and have supported remarkable behavioral and ecological diversification. While summarizing this research is beyond the scope of this paper, amphibians stand apart from other vertebrates for having exceptionally variable and late-occurring bone formation, as well as highly plastic rates of larval growth and development and high ranges in larval period. The goals here are 1. to summarize caveats about collecting and using ossification sequence data, 2. to use frogs and salamanders to question the prevailing adult function- and embryogeny-based approaches for interpreting ossification sequences, and 3. to present hypotheses and evidence that advocate for a new “whole-animal, whole-ontogeny” approach to understand the developmental and evolutionary significances of these data.
Caveats About Collecting and Interpreting Ossification Sequences
Determining when exactly a bone appears depends on how it is visualized, which has been done typically in Alizarin red-stained, glycerin-cleared whole mounts. Sectioned specimens (Hanken and Hall, 1988a) and calcein-stained whole mounts (Schreiber, 2006) can give earlier results, and micro-computed tomography (μCT) gives comparable results (Polachowski and Werneburg, 2013), but with the advantages of producing digital 3D images, and being faster, less size-limited and nondestructive, though caution must be taken to not dry out small specimens. However, μCT fails to resolve cartilage and small bones (Werneburg et al., 2015), and early appearing bones and portions of otherwise stained bones can also fail to stain with Alizarin red (Rieppel, 1993a; Cubbage and Mabee, 1996; Mabee and Trendler, 1996; Mitgutsch et al., 2011). One explanation for no staining is that the bone is still osteoid or not yet mineralized. Another is dissolution of the mineral by storage in formalin or by prior use of an acidic cartilage stain (Wagemans and Vandewalle, 2001; Grünbaum et al., 2003; Walker and Kimmel, 2007; Werneburg et al., 2015). Dissolution is more likely in specimens stored long term, especially in unbuffered or unstably buffered 10% formalin (Markle, 1984), or in 10% formaldehyde, as reported in one study reviewed for this paper. Distinguishing between the two requires fresh material that has been fixed for hours and not months or years, skipping the cartilage stain for some specimens or using a neutral stain (Walker and Kimmel, 2007; Ma et al., 2019), and using a stain for bone that detects calcium (von Kossa, Alizarin red, calcein) and not just matrix protein.
Obtaining a well-resolved ossification sequence depends not only on having a large number of specimens that represent a complete ontogenetic series, but on how specimens are ordered. Commonly used metrics are developmental stage, which is based on external morphological changes; body size (length or weight), which measures growth; and chronological age, which could be relative to fertilization, oviposition, hatching or birth. A fourth, physiological age, remains hypothetical as it requires finding a molecular marker that shows a constant rate of measurable change throughout life, and not just adulthood (Reiss, 1989; Austad, 1993; Strauss, 1999). Having a metric as well as multiple specimens for each stage, size, or age allows one to identify ranges of appearance, i.e., from the lowest stage/size/age at which a bone is first present in one or more specimens to the lowest stage/size/age at which that bone is present in all specimens. The convention for reporting ossification sequences is to use the former (Sheil et al., 2014). However, while staging systems are invaluable for the developmental biology of model organisms, comparative studies are often hindered by the absence of universal staging systems, meaning a sequence of external changes that are general enough and conserved enough in their relative timing to apply to all members of a major group of vertebrates, let alone to all vertebrates. Also, age is often not available for field collected and museum specimens, and even if age and size are both available, intraspecific variation in growth or developmental rates makes it difficult to use either metric to resolve an ossification sequence (Mabee and Trendler, 1996). The lack of a universal metric for gauging postembryonic progress is not surprising given the capacity for environmental factors and natural selection to dissociate traits at most, if not all, phenotypic levels (molecular, morphological, and growth).
Thus, the relatively recent idea to treat bone appearances relative to each other, either in pairs (Mabee and Trendler, 1996; Smith, 1997; Velhagen, 1997; Jeffery et al., 2005) or from first to last (Strauss, 1990; Germain and Laurin, 2009; Harrington et al., 2013), opened the door for many kinds of evo-devo analyses (Yeh, 2002; Sánchez-Villagra et al., 2008; Germain and Laurin, 2009; Maxwell et al., 2010; Weisbecker and Mitgutsch, 2010; Harrington et al., 2013; Koyabu et al., 2014; Werneburg et al., 2015). It became possible to identify heterochronic shifts in suites of characters between major groups of vertebrates as well as timing differences within a major group between modules of bones defined by function, developmental type or origin. It also became possible to experiment with ossification sequence data in creating phylogenies, and to use molecular based phylogenies for major groups to expose the conserved and labile components of their sequences, to estimate the phylogenetic signal of a sequence (meaning the impact of phylogenetic affinity on its evolution), and to derive ancestral or modal ossification sequences.
However, treating bone appearances relative only to each other also has its methodological constraints. The omission of everything that is not a bone appearance precludes one from distinguishing between small and large differences in timing of bone appearance. Also, the likelihood of finding intra- and interspecific differences in sequence as well as the significance of a difference varies not only with the number of bones involved and how closely they appear relative to each other, but with the relative length of the ossification period. This means the length of time over which bones appear relative to the portion of postembryonic life when the bony skeleton has developed to the point of actually contributing to fitness. Though a pioneering study of ossification sequences included key events in muscle, central nervous system and later bone development (Smith, 1997), most subsequent analyses have omitted important life history events such as hatching or birth, the onsets of exogenous feeding and metamorphosis, and changes in musculoskeletal function, behavior and ecology. Such features could serve as useful markers in developmental, ecological and phylogenetic comparisons, and broaden the utility of such studies to more areas of biology.
How Are Amphibian Ossification Sequences Special?
As it is difficult to compare sequences across vertebrates, I will briefly review the ossification periods of the major non-amphibian groups as background for discussing amphibian ossification periods and sequences.
Studies on turtles, lizards, alligators, snakes, one dinosaur, altricial and precocial birds, and placental mammals show that most amniotes acquire all or almost all bones before hatching or birth (Rieppel, 1993a,b, 1994; Smith, 1997; Nunn and Smith, 1998; Sánchez-Villagra, 2002; Maxwell, 2008; Sánchez-Villagra et al., 2008; Maxwell and Larsson, 2009; Maxwell et al., 2010; Hautier et al., 2011; Mitgutsch et al., 2011; Polachowski and Werneburg, 2013; Koyabu et al., 2014; Werneburg et al., 2015; Chapelle et al., 2020). Marsupial mammals differ in acquiring three cranial bones for feeding a day or two before birth and the others by the end of the first quarter of pouch life (Smith, 1997; Nunn and Smith, 1998) The most complete studies for teleost fish show ossification starting right after hatching (and chondrification), and continuing for several weeks while the yolk is consumed (Cubbage and Mabee, 1996; Mabee and Trendler, 1996; Gluckmann et al., 1999; Wagemans and Vandewalle, 2001). Flatfish differ in that some (Brewster, 1987; Ma et al., 2019), but not all (Wagemans and Vandewalle, 2001; Schreiber, 2006) species delay most or all bone appearances to the onset of eye migration, at around 3 weeks post hatching. Basal actinopterygian fish and lungfish extend bone appearances past the yolk sac stage, and in the case of bichirs and two lungfish to the stage of external gill loss, at 6–8 weeks post hatching (Agar, 1906; Pehrson, 1940, 1944, 1949, 1958; Jollie, 1980, 1984a,b,c; Bartsch et al., 1997). All major groups have relatively conserved ossification sequences, coupled with small amounts of intraspecific variation and interspecific variation among closely related species, and a few timing shifts that can be correlated with other traits, e.g., the supraoccipital appearing earlier in larger brained mammals (Koyabu et al., 2014) and compressed ossification periods in rapidly developing mammals (Smith, 1997).
Amphibians ossification periods usually extend past metamorphosis, which generally occurs within 1 or 2 years of hatching, but can be as early as eight days and 6 weeks post-hatching in frogs and salamanders respectively, and as late as 4 and 5 years in these groups (Blanchard, 1923; Bruce, 1980, 1988; Newman, 1989; Petranka, 1998; Bury and Adams, 1999). Salamanders, which are carnivorous as both larvae and adults, acquire bones for feeding around the time of hatching, and others in mid to late larval stages and metamorphosis (Rose, 2003a). The most variable in timing are the maxilla, nasal and septomaxilla, which can appear before or during metamorphosis (Rose, 1996). In contrast, frogs, which typically have herbivorous larvae, are marked by late starts for ossification, and highly variable ossification sequences. The first three bones, usually the exoccipital, parasphenoid, and frontoparietal (Figure 1), can be acquired at an early larval stage (Haas and Richards, 1998; Senevirathne et al., 2017), midlarval stage (Wild, 1997, 1999), during metamorphosis (Maglia, 2003), and afterwards (Trueb, 1966). One species acquires its first 10 cranial bones in larval stages (Haas, 1999), whereas another acquires its first two in late metamorphosis, and the next 16 in juvenile growth (Trueb, 1966). Functionally important bones like the premaxilla, maxilla, angulosplenial, dentary, and nasal (Figure 1) can appear in tadpoles (Trueb and Hanken, 1992), froglets (Trueb, 1966; Gaudin, 1973), or in between (Maglia, 2003). Such variability defies easy characterization and draws into question the meaning of a modal or average ossification period or sequence for frogs.
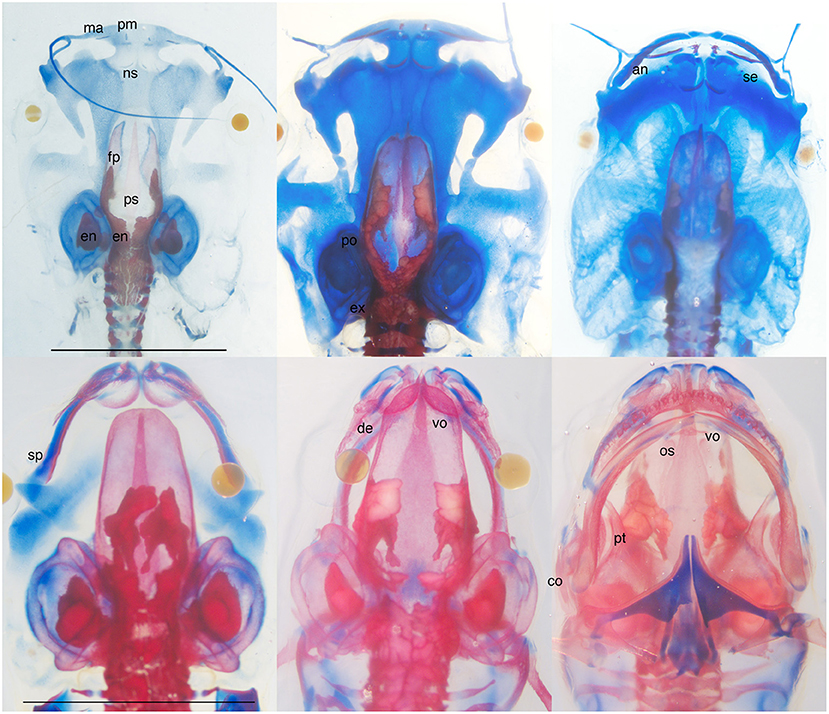
Figure 1. Skeletally stained Xenopus laevis at larval (top L to R, NF stages 56, 57, 59) and metamorphic (bottom L to R, NF 63, 65, 66) stages; bottom right is a ventral view, others are dorsal views; NF 56 and 58 specimens have lower jaw and hyobranchial skeletons removed; blue is cartilage and red is bone; scale bars are 5 mm. Cranial bones appear as faintly stained thin plates (ps and fp), small splints (pm, ma, ns, se, sp, de, vo, an), and small zones of replacement (ex, os, po), and progress gradually and directly toward their adult shapes and sizes. The major changes in skull architecture (shrinkage of the snout, nasal capsule formation, lower jaw lengthening, and shape change, posterior rotation of the jaw suspension) occur in cartilage between NF 59 and 66. They make the head narrower and more pointed, the braincase overlap with the nasal capsule, the snout protrude beyond the jaw, and the corner of the mouth move from in front of the eye to behind the eye. Whereas the braincase roof is closed by the end of metamorphosis (NF 66), the dentary and angulosplenial remain separate in the lower jaw as do the facial processes of premaxilla and maxilla in the snout. Endolymphatic deposits, which have white interiors and are irregularly shaped and distributed within the braincase, ear capsule, and vertebral canal, appear before the first bone and persist after metamorphosis. Bone labels are placed next to bone rudiments and on top of larger bones; an, angular part of angulosplenial; co, columella; de, dentary; en; endolymphatic deposits; ex, exoccipital; fp, frontoparietal; ma, maxilla; ns, nasal; op, operculum; os, orbitosphenoid; ps, parasphenoid; pm, premaxilla; po, prootic; pt, pterygoid; se, septomaxilla; sp, splenial part of angulosplenial; vo, vomer; bold fonted bones are endochrondral; not shown are the quadrate and squamosal; the angular, pterygoid, prootic, and columella as rudiments; and the quadratojugal, which appears after NF 66.
How is the Rest of Amphibian Development Special?
Ossification sequences are particularly easy to document and compare for metamorphosing amphibians because much of their postembryonic development is highly conserved. Most metamorphosing frogs exhibit very similar sequences of conspicuous change in the mouth, limbs, and tail, as well as of remodeling throughout the head and body (Fabrezi et al., 2010; Fabrezi, 2011), muscles (Alley, 1989; Fabrezi et al., 2014), gut (Schreiber et al., 2005; Ishizuya-Oka and Shi, 2007; Fabrezi et al., 2010), and skin (Fox, 1985; Lannoo, 1987; Regueira et al., 2016). Salamanders exhibit a less derived, less encompassing sequence of changes that differs from frogs externally by tail retention and earlier and more variable timing of hind limb development. The conservation of external changes allowed the creation of almost universal developmental staging systems for frogs (Gosner, 1960) and salamanders (Glücksohn, 1931; Rugh, 1962; Iwasawa and Kera, 1980; Norman, 1985; Shi and Boucaut, 1995) that extend from fertilization to the end of metamorphosis. The Gosner system for frogs, which consolidates two earlier systems (Taylor and Kollros, 1946; Limbaugh and Volpe, 1957), has been applied to almost all metamorphosing species studied so far (Altig and Johnston, 1989; Altig and McDiarmid, 2015), albeit with slight modifications for ones with exceptional larval growth (Moore and Townsend, 2003; Fabrezi et al., 2009). A similar, more detailed system for Xenopus and other pipids (Nieuwkoop and Faber, 1956) recognizes minor variability in the timing of external and internal characters, and points out the most reliable characters to use.
The conserved postembryonic development of amphibians derives from reliance upon one primary mediator, thyroid hormone (TH). TH production in frogs starts in early larval stages and increases through to metamorphosis (Etkin, 1935; Dodd and Dodd, 1976; White and Nicoll, 1981; Rose, 1999; Yaoita, 2019). The rise in plasma TH activates postembryonic changes through a combination of intracellular processes in tissues throughout the body that collectively determine if, when, and how each tissue and, for bone appearances, each population of precursor cells respond to TH (Gilbert et al., 1996; Shi, 2000). These processes include cytoplasmic enzymes converting T4 to the more active form, T3, and both to nonactive forms (Becker et al., 1997; Brown, 2005), regulation of TH receptor gene expression (Yaoita and Brown, 1990), interactions of TH with other hormones (Tata et al., 1991; Sachs and Buchholz, 2019; Sterner et al., 2020), and changes in TH-regulated gene expression (Buckbinder and Brown, 1992; Berry et al., 1998). Though these processes work together to control when and how tissues respond to TH, the tradition of referring to early tissue responses, which are activated at lower plasma TH levels, as being more sensitive to TH remains useful for discussing evolutionary changes in developmental timing. Whereas whole-body evolutionary trends like direct development and paedomorphosis likely involve changes in both TH production and TH sensitivity, the persistence of largely conserved postembryonic patterns among metamorphosing frogs and salamanders strongly suggests that heterochronic changes in individual tissue responses like bone appearances result largely from changes in TH sensitivity.
What Current Approaches Do not Tell Us About Ossification Sequence Evolution
Current efforts to understand evolutionary variation and modularity in ossification sequences are rooted in the historical traditions of functional and developmental morphology (alternative terms are externalist or adaptationist, and internalist) (Gould and Lewontin, 1979; Alberch, 1980, 1989; Hildebrand et al., 1985; Gould, 1986; Rieppel, 1988; Ashley-Ross and Gillis, 2002). The functional approach emphasizes the selective pressures that are directed at individual bones and bone regions to accommodate mechanical stress as the animal attains its adult size and functions (Trueb, 1993; Mabee and Trendler, 1996; Wagemans and Vandewalle, 2001; Simon and Marroig, 2017). The developmental approach emphasizes the influences on adult morphology that are imparted by the embryonic history of cells and tissues, e.g., cranial neural crest versus paraxial mesoderm and anterior versus posterior crest origins, and dermal vs. endochondral or perichondral bone formation (Hallgrímsson et al., 2009; Koyabu et al., 2014; Simon and Marroig, 2017; Felice and Goswami, 2018). While the two viewpoints fuel highly productive research programs, they leave unanswered several important questions about adult functions, embryonic influences, and whether both are sufficient to explain evolutionary patterns in ossification sequences.
A fundamental question for understanding the functional significance of ossification sequences is when does bone strength matter to the point that bone development is shaped by selection. Unlike other vertebrates, amphibians acquire many bones as fully differentiated animals that are already using their cartilage skeletons for feeding, breathing, support and locomotion. Their dermal bones typically appear as single grains of mineralized tissue alongside cylindrical cartilages like the lower jaw and jaw suspension or as multiple diffuse grains that coalesce in open spaces in the roof, floor and wall of the nasal capsule and braincase (Figure 1, Lebedkina, 1960a,b, 1964, 1968, 2004; Smirnov and Vassilieva, 2009). But when does a bone rudiment that gradually elongates along a cartilage or expands above a sense organ or brain start to impact fitness? A bony splint might be expected to allow the portion of cartilage adjacent to it to resist deformation. However, this would shift load to adjoining cartilage not adjacent to bone and increase its likelihood of deformation, especially in parts close to joints. Also, given the strength and weight differences between bone and cartilage, bone rudiments growing adjacent to cartilage might detract from the latter's ability to distribute load, as well as increase the energy required for its movement. The first stage at which one might expect dermal bone strength to definitely enhance fitness is when single elements articulate or fuse with each other to form a bony framework whose response to loading supercedes that of cartilage and unconnected bone rudiments. Examples would be the solidification of dermal bones in the regions of the mandible, palate and jaw suspension, and the abutting of left and right roofing bones to complete a cranial vault. Such configurations are usually not attained in frogs until the end of metamorphosis or later (Figure 2).
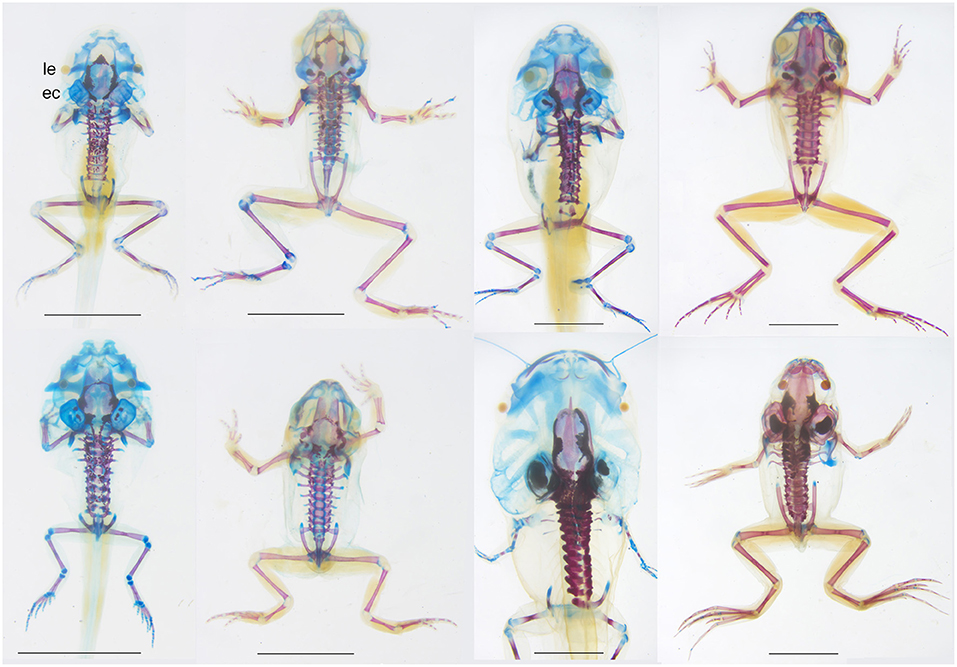
Figure 2. Dorsal views of skeletally stained specimens of four species of frogs, from top left to bottom right, Pseudacris crucifer (Hylidae), Lithobates sylvatica (Ranidae), Anaxyrus fowleri (Bufonidae), and Xenopus laevis (Pipidae) showing interspecific differences in bone size and shape in mature tadpoles (left) and recent postmetamorphs (right), i.e., at the start and end of the cartilage remodeling described in Figure 1 (GS 40-41 and GS 46 for the first three species; NF 59 and 66 for the fourth species). Mature tadpoles of all four species have endolymphatic deposits, one or more dermal bones lining the braincase, all vertebrae and rib ossifications, and all limb long bones and girdle bones excluding carpals and tarsals. Differences include the absence of the parasphenoid in Anaxyrus, the small size of limb bones in Xenopus, more bone rudiments in the snout of Xenopus, and narrow, widely separated frontoparietals in Pseudacris. Recent metamorphs all show general increases in cranial ossification; the braincase roof remains open and the ear capsule and snout remain poorly ossified in in Pseudacris and Anaxyrus, and Xenopus appears the most ossified of the four. ec, ear capsule; le, lens of eye. Scale bars are 5 mm. All specimens were collected and/or raised, prepared and DNA barcoded by the author using standard techniques, Virginia state collecting permits and AICUC approved protocols.
Also, amphibian metamorphosis marks one of the biggest postembryonic transformations in musculoskeletal function and structure in vertebrates and the changes in breathing and feeding behavior that define the metamorphic period are underlain by major changes in cartilage regions of the skull, particularly the lower jaw, jaw suspension, palate, nasal capsule, and hyobranchium (Figure 1, Rose and Reiss, 1993; Rose et al., 2015). The period of transformation is also one of high vulnerability and mortality, implying there is strong selection to keep the changes as brief and as coordinated as possible (Wassersug and Sperry, 1977; Wassersug and Hoff, 1982). That many bones appear outside of this period, that almost all bone rudiments develop directly to their adult size and shape (Figure 1)—only the salamander vomer and coronoid attain shapes designed specifically for larval functions—and that the major functional transformations at metamorphosis are effected more in cartilage than in bone underscores the primacy of cartilage in the origin and diversification of amphibian metamorphosis (Rose, 2014b). These points also accentuate the conclusion drawn from other vertebrates that timing of bone appearance is not strongly tied to, and therefore not predictive of, onset of bone function in load bearing (Mabee and Trendler, 1996; Maxwell, 2008; Maxwell and Larsson, 2009).
From a developmental perspective, the decision to analyze sequences of only bone appearances presupposes that the mechanisms controlling timing of bone appearance are somehow distinct from those controlling the timing of other tissue changes, and even that bone appearances are developmentally interdependent. The same could be said for grouping bone appearances into modules based on embryonic origin of the skeletal precursor cells or mode of bone formation. Yet, despite many postulations to the contrary, there has been no developmental evidence or mechanism proposed so far for how the appearance of one or more bones might activate, enable, inhibit, or otherwise affect the appearance of other bones. Endochondral and perichondral bone appearances obviously require the presence of pre-existing cartilage. The former also requires vascularization to supply the cells required for cartilage resorption and bone deposition, although cartilage cells transforming directly into bone cells remains a possibility (Ishizeki et al., 2010), as does bone cells transforming into secondary cartilage cells (Woronowicz and Schneider, 2019). All dermal bone appearances require interaction with epithelia, and certain bones interact with specific epithelia, e.g., lateral line organs in fish and amphibians and the nasolacrimal duct in amphibians, and possibly also with cartilage (Hall and Hanken, 1985; Rose and Reiss, 1993; Richman et al., 2006; Wada et al., 2010). Cartilage serving as a scaffold for dermal bone condensation is suggested by bones forming normally next to cartilage in ectopic grafts of facial primordia (Richman et al., 2006) and TH-induced bones appearing out of order alongside precociously induced nasal cartilage (Rose, 1995, 1996). That certain amphibian bones additionally rely upon thyroid hormone (discussed below) would imply that they also depend upon the establishment of a functioning circulatory system and thyroid gland.
Also, skeletal cell condensation and differentiation are the products of gene regulatory networks that involve bone- and cartilage-specific transcription factors and signaling pathways, and play out to similar ends in precursor cell populations that vary in size, shape, location, and embryonic origin (Hanken and Gross, 2005; Richman et al., 2006; Hall et al., 2014; Jandzik et al., 2014, 2015; Gilbert and Barresi, 2016; Schneider, 2018; Woronowicz and Schneider, 2019). Other than TH mediation and the dermal bone interactions mentioned above, there is no a priori reasoning for why gene regulatory networks or cell history would predispose certain bones or groups of bones to appear earlier or later than others. On the other hand, there are functional and physiological reasons for why certain bones appear when they do. The finding from across vertebrates (Mabee and Trendler, 1996; Wagemans and Vandewalle, 2001; Yeh, 2002; Maxwell et al., 2010; Koyabu et al., 2014; Ollonen et al., 2018) that dermal bones tend to appear earlier than endochondral bones need not reflect a developmental constraint (Koyabu et al., 2014). It could be simply that endochondral bones are not, and never have been, directly involved in the onset of feeding and breathing, and that the cartilage skeleton that precedes them in life (and probably evolution) suffices for support and locomotion in the aquatic environments occupied by amniote embryos and anamniote hatchlings and early larvae. Similarly, the late appearances of cranial bones in frogs and flatfish point to the sufficiency of a mostly cartilage skeleton for feeding and breathing throughout the larval period. Indeed, the supra- and infrarostral cartilages of tadpoles suffice for rasping algae and mouth closing even in the largest tadpoles (Alcalde and Barg, 2006; Downie et al., 2009a,b; Fabrezi and Goldberg, 2009). Also, delaying cranial bone appearances and growth to metamorphosis or afterwards allows for major reshaping of the skull without the physiological expense of having to resorb bone that has been shaped specifically for larval functions.
How Environmental Factors Impact Amphibian Ossification Sequences
Environmental factors can directly affect timing of bone appearances in both adaptive and non-adaptive ways. An example of adaptive plasticity is fin bone appearances being accelerated in a teleost raised in fast water (Cloutier et al., 2010). This implies that the gene regulatory networks for bone appearance can be configured epigenetically to activate cartilage replacement directly in response to mechanical stress, and that there is either a cost to early bone appearance or an adaptive advantage to later bone appearance; otherwise the early timing would become fixed genetically.
The most extreme case of variable bone appearances known for amphibians involves Ascaphus truei, a frog that spends 1–4 years as a tadpole sucking onto rocks in fast flowing mountain streams in temperate forests (Brown, 1990; Bury and Adams, 1999). Two of its three earliest bones (parasphenoid and frontoparietal) appear across a range of 11 Gosner stages, from the beginning of hind limb differentiation and growth at GS 30 to the beginning of metamorphosis at GS 41 (Moore and Townsend, 2003). Though the specimens were stored in formalin for about 20 years prior to Alizarin red staining, the authors took care to identify unstained bones. As 11 of the 14 other cranial bones appear across smaller ranges of stages, the number of possible ossification sequences exhibited by this species is immense. That a similar amount of variability was found in three populations from different parts of the species range argues for little, if any, adaptive significance to the exact stage of larval bone appearance. This conclusion is supported by studies on six distantly related species that report small ranges in all bone appearances for lab-raised animals, meaning that individuals are usually siblings, environmental and fixation conditions are controlled, and sources of variability are limited to differences in growth and developmental rates and feeding behavior (Kemp and Hoyt, 1969; Gaudin, 1978; Hanken and Hall, 1984; Smirnov, 1992; Trueb and Hanken, 1992; Haas, 1999). These differences, which could be genetic or induced by conspecifics (Wilbur and Collins, 1973), are indeed sufficient to produce variation in ossification sequence with lasting effects on adult bone shape (Smirnov, 1992).
How TH Mediation Impacts Amphibian Ossification Sequences
Since larval growth and developmental rates can vary independently of each other (Alford and Harris, 1988; Rose, 2005; Gomez-Mestre et al., 2010), whether both parameters can affect timing of bone appearance and ossification sequence remains unclear. However, some plasticity in early bone appearances might be expected as a non-adaptive consequence of being hormonally regulated. The earliest frog bones, which also appear to be the most variable, first arise around Gosner stage 30 (or Nieuwkoop Faber stage 53), which is the earliest stage at which plasma TH has been detected in frogs by radioimmunoassays (Leloup and Buscaglia, 1977; Miyauchi et al., 1977; Regard et al., 1978; Mondou and Kaltenbach, 1979; Suzuki and Suzuki, 1981; Weil, 1986). GS 30 is also approximately when frog postembryonic development is believed to become TH mediated, since tissues at earlier stages can be accelerated by exogenous TH, but not delayed by TH inhibitors or thyroid gland inactivation (Allen, 1918; Terry, 1918; Brown, 2005; Kerney et al., 2010; Smirnov and Vassilieva, 2014; Rose and Cahill, 2019). By the same criteria, the onset of TH mediation in salamander ossification occurs between mid and late larval stages (Smirnov et al., 2020). Cells that condense and mineralize around GS 30 might be expected to show accelerated or delayed activation due to the high plasticity in amphibian growth and developmental rates (Rose, 2005, 2014a), and the exceptionally low TH involved in their activation. The plasma T4 of larval frogs, when first detected near the start of metamorphosis, is at 0.3–0.6 nM (which is just above the detectable limit of the tests), and peaks in mid or late metamorphosis at 6–13 nM (references above). This contrasts with larval fish, whose plasma TH levels are typically above 20 nM, and amniotes near hatching or birth whose levels can be over 100 nM (Rose, 2003b). Also, all amphibian studies that measured single individuals found some with non-detectable TH at all larval and metamorphic stages. Thus, the TH signal of larval frogs is inherently low and variable, and the earliest and lowest part of the signal might be further destabilized by environmental fluctuations that switch the animal back and forth between development and growth. The bones that respond to the early signal also appear to be inherently variable based on their tendency to appear in variable order (Hanken and Hall, 1988b) and from a variable number of ossification centers (Smirnov and Vassilieva, 2009) when induced at a constant TH level. Whether mediation by TH makes amphibians uniquely susceptible to variably timed bone appearances depends on the extent to which other vertebrates rely upon circulating factors to mediate bone condensation in a concentration-dependent fashion. One might expect less variability from condensation events in embryogenesis that are mediated only by locally secreted paracrine factors.
How Calcium Physiology Impacts Amphibian Ossification Sequences
Another source of plasticity in the appearance and growth of bone involves the availability of calcium and the utilization of mineral reserves. Frogs, which generally do not eat during metamorphosis or resorb bones, appear to draw the calcium required for their metamorphic bone appearances and growth from exceptionally large calcium carbonate deposits that are built up in the endolymphatic sacs, braincase, and anterior vertebral canal during larval growth (Figures 1, 2, Pilkington and Simkiss, 1966). Indeed, quantification of calcium, phosphate, and carbonate levels shows that as a ranid tadpole develops, it first deposits calcium carbonate as endolymphatic deposits, and then shifts to depositing calcium phosphate as bone as it approaches metamorphosis. Metamorphosis is characterized by both bone deposition and calcium carbonate resorption, implying that calcium mobilized from the carbonate reserves is redeposited as bone. A slight decrease in calcium content overall suggests that the calcium reserve is more than sufficient to supply the bone deposition (Pilkington and Simkiss, 1966). Both the calcium carbonate and bone deposits are larger in tadpoles raised in calcium-enriched water, and animals deprived of calcium do not grow bone during metamorphosis despite losing calcium carbonate. How such plasticity would impact skeletal anatomy and ultimately fitness would depend not only on calcium availability, but on how larval bone appearances are spaced relative to each other and to the buildup and dissolution of carbonate reserves.
How early mineral deposition affects later bone formation could also be influenced indirectly by environment as evidenced by early appearing bones growing larger in tadpoles with extended larval periods (Smirnov, 1992). In other words, bone deposition can proceed unabated in existing bones during growth and developmental slow-downs that delay the appearance of later arising bones. This suggests that ossification in tadpoles involves at least three separate rate-controlling mechanisms. TH appears to regulate, albeit with some variability, when bones appear relative to other tissue changes; bone growth is presumably regulated by the same circulating factors that keep most tissues on their species-specific growth curves despite differences in body size; and mineral deposition and resorption are regulated to allow the body to accumulate and mobilize calcium in different ways at different stages of life. The resilience of the third control mechanism to environmental factors that slow development and growth raises new hypotheses for why late appearing bones can become exceptionally small (Wake, 1980) or lost altogether (Trueb, 1985) in amphibian evolution. More generally, as other vertebrates also store and mobilize endolymphatic calcium carbonate (Polachowski and Werneburg, 2013), the pathways and environmental factors regulating calcium utilization present an altogether new front for exploring how ossification periods and sequences are shaped by differences in larval environment and growth trajectory, and how plasticity in the early part of a sequence impacts later ossification and adult morphology.
How Lung Use Might Impact Amphibian Ossification Sequences
When and where bone accumulates in the body might be adaptive in how bone weight rather than bone strength contributes to tadpole breathing, feeding, and locomotion. This derives from amphibian larvae having not one, but four respiratory organs: lungs, gills, skin, and the buccopharyngeal epithelium. The additional organs make lung use essentially optional for larval respiration, and while some amphibians have lost lungs altogether (Rose and James, 2013), others fall on a spectrum of larval lung use, with the extremes showing conspicuous differences in ossification, feeding and locomotion.
At one extreme, Xenopus tadpoles are suspension feeders that stay in the water column, and start lung breathing immediately after hatching (Rose and James, 2013). Their large lungs remain undivided, do little gas exchange under normal conditions (Burggren and West, 1982; Feder and Wassersug, 1984), and are not essential for life (Rose and James, 2013). Indeed, tadpoles denied access to air will metamorphose and live for years without inflating their lungs, and they can initiate lung use and resume lung development at any point in tadpole life (Rose and James, 2013). Xenopus tadpoles mostly likely use lungs to provide buoyancy as an energy saving measure for midwater living. The buoyancy is offset by beating a vertically flexed tail (Rose et al., 2018) and bone formation that starts at mid larval stages and produces a relatively well-ossified head and trunk by the start of metamorphosis (Figures 1, 2, Sedra and Michael, 1957; Trueb and Hanken, 1992). Xenopus tadpoles reduce activity to avoid predators (Kruger et al., 2019), but rarely settle on the bottom unless they have been air-deprived (Rose and James, 2013). Xenopus tadpoles thus appear committed to midwater living, and their lungs and larval bones collectively support a head-down, tail-up swimming posture.
At the other extreme, toad tadpoles have tiny lung buds that are not inflated until metamorphosis (Wassersug and Seibert, 1975). They spend much of their time feeding and lying on the bottom (Tejedo, 1993), and often fall to the bottom rather than actively swimming there (Rose, pers. obs.). Since toads do not modify their tail size, shape or color in response to predators (Benard and Fordyce, 2003), their delayed lung use supports benthic living and avoiding the water column where they are more prone to predation (Tejedo, 1993). Toads also have late ossification, especially in the skull, which acquires most bones during and after metamorphosis (Gaudin, 1978; Gómez et al., 2017; Figure 2, Dunlap and Sanchiz, 1996). An ossified trunk and unossified head means a more posterior center of gravity and less forward momentum when swimming, which would facilitate rapid, passive settlement and immobility as an escape response. At the same time, differences in larval ossification are likely to have consequence for the onset of postmetamorphic locomotion. Whereas Pseudacris treefrogs jump to and from horizontal and vertical surfaces the moment they leave the water, Anaxyrus toads are noticeably less agile, appearing to learn to walk before they learn to jump (Rose, pers. obs., Wassersug and Sperry, 1977). How larval lung use and bone appearances have co-evolved to support different styles of larval swimming and feeding, and early juvenile movement and habitat use (Altig and Johnston, 1989; Bruce et al., 1994) represents another avenue for analyzing ossification sequences that emphasizes midlife adaptation over embryonic influence and adult function.
Toward a More Inclusive Approach for Understanding the Evolution of Ossification
The two prevailing perspectives in evolutionary morphology are forward- and backward-looking in that one views early development through the lens of functional demands imposed by adulthood, and the other views adult morphology through the lens of embryonic events that influence postembryonic development. By virtue of their exceptional lateness, variability and plasticity, amphibian ossification sequences defy an explanation based strictly on governance by embryonic processes and compliance with adult functional demands. In addition to the two perspectives, amphibians call for a third, more “in-the-moment” perspective to embrace the interplay among environment, behavior, and physiology that directly shapes growth and development after embryogeny and before adulthood. This means implicitly a whole-animal approach that integrates bone appearances with other developmental events and traits such as using carbonate deposits as mineral reserves and lungs for buoyancy. It also means a whole-ontogeny approach that recognizes intermediate life history stages as being informative of how organisms diversify regardless of their impact on adult form and function. This would apply to all vertebrates and not just those whose larval and post-metamorphic bodies have already been shown to evolve independently of each other (Sherratt et al., 2017).
Both modern (Polachowski and Werneburg, 2013; Simon and Marroig, 2017) and classical (Miller, 1923; Tchernavin, 1938) studies of vertebrate skeletons have proposed that differences in bone growth explain more of the adult skeleton than differences in timing of bone appearance or bone origin. There has been ample research on how bone and cartilage growth is affected by mechanical stress for mammals and birds (Murray, 1936; Herring, 1993; Hall, 2005), but relatively little for anamniotes, especially ones with biphasic development. We are beginning to understand the cellular mechanisms that regulate skeletal shape during growth (Vandenberg et al., 2012; Rose, 2014b; Kaucka et al., 2017; Pinet et al., 2019) and that produce interspecific differences in long bone (Farnum et al., 2008; Cooper et al., 2013) and dermal bone shapes (Slater et al., 2009). We have yet to investigate how plasticity in skeletal growth is regulated by the environmental, behavioral, and physiological factors discussed here and elsewhere (Aubret and Shine, 2009; Serrat, 2014). Thanks to big data approaches, our knowledge of both the gene regulatory networks underlying embryonic morphogenesis and the evolutionary patterns exhibited by adult skeletons is expanding at phenomenal rates. At the same time, it is important to recall not only that “ontogeny [and not just embryogeny] creates phylogeny” (Garstang, 1922), but that the cellular, behavioral and physiological processes which create morphological differences play out over entire ontogenies and can be impacted directly by environment.
Data Availability Statement
The original contributions presented in the study are included in the article/supplementary material, further inquiries can be directed to the corresponding author/s.
Ethics Statement
The animal study was reviewed and approved by IACUC, James Madison University Office of Research Integrity.
Author Contributions
The author confirms being the sole contributor of this work and has approved it for publication.
Funding
This research was supported by the Biology Department, James Madison University.
Conflict of Interest
The author declares that the research was conducted in the absence of any commercial or financial relationships that could be construed as a potential conflict of interest.
References
Agar, W. E. (1906). The development of the skull and visceral arches in Lepidosiren and Protopterus. Trans. Roy. Soc. Edinburgh 45, 49–64. doi: 10.1017/S0080456800011637
Alberch, P. (1980). Ontogenesis and morphological diversification. Amer. Zool. 20, 653–667. doi: 10.1093/icb/20.4.653
Alberch, P. (1989). The logic of monsters: evidence for internal constraint in development and evolution. Geobios Mémoire Spécial 12, 21–57. doi: 10.1016/S0016-6995(89)80006-3
Alcalde, L., and Barg, M. (2006). Chondrocranium and cranial muscle morphology in Lysapsus and Pseudis tadpoles (Anura: Hylidae: Hylinae). Acta Zoolog. 87, 91–100. doi: 10.1111/j.1463-6395.2006.00205.x
Alford, R. A., and Harris, R. N. (1988). Effects of larval growth history on anuran metamorphosis. Amer. Nat. 131, 91–106. doi: 10.1086/284775
Allen, B. M. (1918). The results of thyroid removal in the larvae of Rana pipiens. J. Exp. Zool. A Ecol. Genetics Physiol. 24, 499–519. doi: 10.1002/jez.1400240303
Alley, K. E. (1989). Myofiber turnover is used to retrofit frog jaw muscles during metamorphosis. Amer. J. Anat. 184, 1–12. doi: 10.1002/aja.1001840102
Altig, R., and Johnston, G. F. (1989). Guilds of anuran larvae: relationships among developmental modes, morphologies, and habitats. Herpetol. Monogr. 3, 81–109. doi: 10.2307/1466987
Altig, R., and McDiarmid, R. W. (2015). Handbook of Larval Amphibians of the United States and Canada. Ithaca, NY: Cornell University Press. doi: 10.7591/9780801456084
Ashley-Ross, M. A., and Gillis, G. B. (2002). A brief history of vertebrate functional morphology. Int. Comp. Biol. 42, 183–189. doi: 10.1093/icb/42.2.183
Aubret, F., and Shine, R. (2009). Genetic assimilation and the postcolonization erosion of phenotypic plasticity in island tiger snakes. Curr. Biol. 19, 1932–1936. doi: 10.1016/j.cub.2009.09.061
Austad, S. N. (1993). Retarded senescence in an insular population of virginia opossums (Didelphis virginiana). J. Zool. 229, 695–708. doi: 10.1111/j.1469-7998.1993.tb02665.x
Bartsch, P., Gemballa, S., and Piotrowski, T. (1997). The embryonic and larval development of Polypterus senegalus cuvier, 1829: its staging with reference to external and skeletal features, behaviour and locomotory habits. Acta Zoolog. 78, 309–328. doi: 10.1111/j.1463-6395.1997.tb01014.x
Becker, K. B., Stephens, K. C., Davey, J. C., Schneider, M. J., and Galton, V. A. (1997). The type 2 and type 3 iodothyronine deiodinases play important roles in coordinating development in Rana catesbeiana tadpoles. Endocrinology 138, 2989–2997. doi: 10.1210/endo.138.7.5272
Benard, M. F., and Fordyce, J. A. (2003). Are induced defenses costly? Consequences of predator-induced defenses in western toads, Bufo boreas. Ecology 84, 68–78. doi: 10.1890/0012-9658(2003)084[0068:AIDCCO]2.0.CO;2
Berry, D. L., Rose, C. S., Remo, B. F., and Brown, D. D. (1998). The expression pattern of thyroid hormone response genes in remodeling tadpole tissues defines distinct growth and resorption gene expression programs. Dev. Biol. 203, 24–35. doi: 10.1006/dbio.1998.8975
Blanchard, F. N. (1923). The life-history of the four-toed salamander. Amer. Nat. 57, 262–268. doi: 10.1086/279920
Brewster, B. (1987). Eye migration and cranial development during flatfish metamorphosis: a reappraisal (Teleostei: Pleuronectiformes). J. Fish Biol. 31, 805–833. doi: 10.1111/j.1095-8649.1987.tb05281.x
Brown, D. D. (2005). The role of deiodinases in amphibian metamorphosis. Thyroid 15, 815–821. doi: 10.1089/thy.2005.15.815
Brown, H. A. (1990). Morphological variation and age-class determination in overwintering tadpoles of the tailed frog, Ascaphus truei. J. Zool. 220, 171–184. doi: 10.1111/j.1469-7998.1990.tb04301.x
Bruce, R. C. (1980). A model of the larval period of the spring salamander, Gyrinophilus porphyriticus based on size-frequency distributions. Herpetologica 36, 78–86.
Bruce, R. C. (1988). Life history variation in the salamander Desmognathus quadramaculatus. Herpetologica 44, 218–227.
Bruce, R. C., Beachy, C. K., Lenzo, P. G., Pronych, S. P., and Wassersug, R. J. (1994). Effects of lung reduction on rheotactic performance in amphibian larvae. J. Exp. Zool. 268, 377–380. doi: 10.1002/jez.1402680506
Buckbinder, L., and Brown, D. D. (1992). Thyroid hormone-induced gene expression changes in the developing frog limb. J. Biol. Chem. 267, 25786–25791. doi: 10.1016/S0021-9258(18)35678-3
Burggren, W. W., and West, N. H. (1982). Changing respiratory importance of gills, lungs and skin during metamorphosis in the bullfrog Rana catesbeiana. Respir. Physiol. 47, 151–164. doi: 10.1016/0034-5687(82)90108-6
Bury, R. B., and Adams, M. J. (1999). Variation in age at metamorphosis across a latitudinal gradient for the tailed frog, ascaphus truei. Herpetologica 55, 283–291.
Chapelle, K. E. J., Fernandez, V., and Choiniere, J. N. (2020). Conserved in-ovo cranial ossification sequences of extant saurians allow estimation of embryonic dinosaur developmental stages. Sci. Rep. 10:4224. doi: 10.1038/s41598-020-60292-z
Cloutier, R., Caron, A., Grünbaum, T., and Le François, N. R. (2010). Effect of water velocity on the timing of skeletogenesis in the arctic charr, Salvelinus alpinus (salmoniformes: Teleostei): an empirical case of developmental plasticity. Int. J. Zool. 2010:470546 doi: 10.1155/2010/470546
Cooper, K. L., Oh, S., Sung, Y., Dasari, R. R., Kirschner, M. W., and Tabin, C. J. (2013). Multiple phases of chondrocyte enlargement underlie differences in skeletal proportions. Nature 495, 375–378. doi: 10.1038/nature11940
Cubbage, C. C., and Mabee, P. M. (1996). Development of the cranium and paired fins in the zebrafish Danio rerio (Ostariophysi, Cyprinidae). J. Morphol. 229, 121–160. doi: 10.1002/(SICI)1097-4687(199608)229:2<121::AID-JMOR1>3.0.CO;2-4
Dodd, M. H. I., and Dodd, J. M. (1976). “The biology of metamorphosis,” in Physiology of the Amphibia, ed B. Lofts (New York, NY: Academic Press), 467–599. doi: 10.1016/B978-0-12-455403-0.50015-3
Downie, J. R., Ramnarine, I., Sams, K., and Walsh, P. T. (2009a). The paradoxical frog pseudis paradoxa: larval habitat, growth and metamorphosis. Herpetol. J. 19, 11–19.
Downie, J. R., Sams, K., and Walsh, P. T. (2009b). The paradoxical frog Pseudis paradoxa: larval anatomical characteristics, including gonadal maturation. Herpetol. J. 19, 1–10.
Dunlap, K. D., and Sanchiz, B. (1996). Temporal dissociation between the development of the cranial appendicular skeletons in Bufo bufo (Amphibia: Bufonidae). Herpetol 30, 506–513. doi: 10.2307/1565693
Etkin, W. (1935). The mechanisms of anuran metamorphosis: 1. Thyroxine concentration and the metamorphic pattern. J. Exp. Zool. 71, 317–340. doi: 10.1002/jez.1400710208
Fabrezi, M. (2011). Heterochrony in growth and development in anurans from the chaco of south america. Evol. Biol. 38, 390–411. doi: 10.1007/s11692-011-9128-5
Fabrezi, M., and Goldberg, J. (2009). Heterochrony during skeletal development of Pseudis platensis (Anura, Hylidae) and the early offset of skeleton development and growth. J. Morphol. 270, 205–220. doi: 10.1002/jmor.10680
Fabrezi, M., Manzano, A. S., Abdala, V., and Lobo, F. (2014). Anuran locomotion: ontogeny and morphological variation of a distinctive set of muscles. Evol. Biol. 41, 308–326. doi: 10.1007/s11692-014-9270-y
Fabrezi, M., Quinzio, S. I., and Goldberg, J. (2009). Giant tadpole and delayed metamorphosis of Pseudis platensis gallardo, 1961 (Anura, Hylidae). J. Herpetol. 43, 228–243. doi: 10.1670/08-028R3.1
Fabrezi, M., Quinzio, S. I., and Goldberg, J. (2010). The ontogeny of Pseudis platensis (Anura, Hylidae): heterochrony and the effects of larval development on postmetamorphic life. J. Morphol. 271, 496–510. doi: 10.1002/jmor.10815
Farnum, C. E., Tinsley, M., and Hermanson, J. W. (2008). Forelimb versus hindlimb skeletal development in the big brown bat, Eptesicus fuscus: functional divergence is reflected in chondrocytic performance in autopodial growth plates. Cells Tissues Organ. 187, 35–47. doi: 10.1159/000109962
Feder, M. E., and Wassersug, R. J. (1984). Aerial versus aquatic oxygen consumption in larvae of the clawed frog, Xenopus laevis. J. Exp. Biol. 108, 231–245.
Felice, R. N., and Goswami, A. (2018). Developmental origins of mosaic evolution in the avian cranium. PNAS 115:555. doi: 10.1073/pnas.1716437115
Fox, H. (1985). “Changes in amphibian skin during larval development and metamorphosis,” in Metamorphosis, ed M. Balls and M. Bownes (Oxford: Clarendon Press), 221–259.
Garstang, W. (1922). The theory of recapitulation: a critical re-statement of the biogenetic law. Zool. J. Linnean Soc. London 35, 81–101. doi: 10.1111/j.1096-3642.1922.tb00464.x
Gaudin, A. J. (1973). The development of the skull in the pacific tree frog, Hyla regilla. Herpetologica 29, 205–218.
Gaudin, A. J. (1978). The sequence of cranial ossification in the california toad, Bufo boreas (Amphibia, Anura, Bufonidae). J. Herpetol. 12, 309–318. doi: 10.2307/1563611
Germain, D., and Laurin, M. (2009). Evolution of ossification sequences in salamanders and urodele origins assessed through event-pairing and new methods. Evol. Dev. 11, 170–190. doi: 10.1111/j.1525-142X.2009.00318.x
Gilbert, L. I., Tata, J. R., and Atkinson, B. G. (1996). Metamorphosis: Postembryonic Reprogramming of Gene Expression in Amphibian and Insect Cells. San Diego: Academic Press.
Gilbert, S. F., and Barresi, M. J. F. (2016). Developmental Biology. Sunderland, MA: Sinauer Assoc., Inc.
Gluckmann, I., Huriaux, F., Focant, B., and Vandewalle, P. (1999). Postembryonic development of the cephalic skeleton in Dicentrarchus labrax (Pisces, Perciformes, Serranidae). Bull. Marine Sci. 65, 11–36.
Glücksohn, S. (1931). Äussere entwicklung der extremitäten und stadieneinteilung der larvenperiode von Triton taeniatus leyd. Und Triton cristatus laur. Wilhelm Roux' Archiv fur Entwicklungsmechanik der Organismen 125, 341–405. doi: 10.1007/BF00576359
Gómez, R., l., O., Regueira, E., O'Donohoe, M. E. A., and Hermida, G. N. (2017). Delayed osteogenesis and calcification in a large true toad with a comparative survey of the timing of skeletal ossification in anurans. Zool. Anzeiger 267, 101–110. doi: 10.1016/j.jcz.2017.03.002
Gomez-Mestre, I., Saccoccio, V. L., Iijima, T., Collins, E. M., Rosenthal, G. G., and Warkentin, K. M. (2010). The shape of things to come: linking developmental plasticity to post-metamorphic morphology in anurans. J. Evol. Biol. 23, 1364–1373. doi: 10.1111/j.1420-9101.2010.02016.x
Gosner, K. (1960). A simplified table for staging anuran embryos and larvae with notes on identification. Herpetologica 16, 183–190.
Gould, S. J., and Lewontin, R. C. (1979). The spandrels of San Marco and the panglossian paradigm: a critique of the adaptationist programme. Proc. R. Soc. Lond. B. 205, 581–598. doi: 10.1098/rspb.1979.0086
Grünbaum, T., Cloutier, R., and Dumont, P. (2003). “Congruence between chondrification and ossification sequences during caudal skeleton development: a moxostomatini case study,” in The big fish bang. Proceedings of the 26th annual larval fish conference, ed H. I. Browman and A. B. Skiftesvik (Bergen, Norway: Institute of Marine Research, Postboks), 161–176.
Haas, A. (1999). Larval and metamorphic skeletal development in the fast-developing frog Pyxicephalus adspersus (anura, ranidae). Zoomorphology 119, 23–35. doi: 10.1007/s004350050078
Haas, A., and Richards, S. J. (1998). Correlations of cranial morphology, ecology, and evolution in australian suctorial tadpoles of the genera Litoria and Nyctimystes (Amphibia: Anura: Hylidae: Pelodryadinae). J. Morphol. 238, 109–141. doi: 10.1002/(SICI)1097-4687(199811)238:2<109::AID-JMOR1>3.0.CO;2-#
Hall, B. K. (2005). Bones and Cartilage, Developmental and Evolutionary Skeletal Biology. San Diego: Elsevier Academic Press. doi: 10.1016/B978-0-12-319060-4.50065-8
Hall, B. K., and Hanken, J. (1985). “Forward,” in The Development of the Vertebrate Skull (Oxford: Oxford University Press), vii–xxviii.
Hall, J., Jheon, A. H., Ealba, E. L., Eames, B. F., Butcher, K. D., Mak, S. S., et al. (2014). Evolution of a developmental mechanism: species-specific regulation of the cell cycle and the timing of events during craniofacial osteogenesis. Dev. Biol. 385, 380–395. doi: 10.1016/j.ydbio.2013.11.011
Hallgrímsson, B., Jamniczky, H., Young, N. M., Rolian, C., Parsons, T. E., Boughner, J. C., et al. (2009). Deciphering the palimpsest: studying the relationship between morphological integration and phenotypic covariation. Evol. Biol. 36, 355–376. doi: 10.1007/s11692-009-9076-5
Hanken, J., and Gross, J. B. (2005). Evolution of cranial development and the role of neural crest: insights from amphibians. J. Anat.207, 437–446. doi: 10.1111/j.1469-7580.2005.00481.x
Hanken, J., and Hall, B. K. (1984). Variation and timing of the cranial ossification sequence of the oriental fire-bellied toad, Bombina orientalis (Amphibia, Discoglossidae). J. Morphol. 182, 245–255. doi: 10.1002/jmor.1051820302
Hanken, J., and Hall, B. K. (1988a). Skull development during anuran metamorphosis: I. Early development of the first three bones to form–the exoccipital, the parasphenoid, and the frontoparietal. J. Morphol. 195, 247–256. doi: 10.1002/jmor.1051950303
Hanken, J., and Hall, B. K. (1988b). Skull development during anuran metamorphosis. II. Role of thyroid hormone in osteogenesis. Anat. Embryol. 178, 219–227. doi: 10.1007/BF00318225
Harrington, S. M., Harrison, L. B., and Sheil, C. A. (2013). Ossification sequence heterochrony among amphibians. Evol. Dev. 15, 344–364. doi: 10.1111/ede.12043
Hautier, L., Weisbecker, V., Goswami, A., Knight, F., Kardjilov, N., and Asher, R. J. (2011). Skeletal ossification and sequence heterochrony in xenarthran evolution. Evol. Dev. 13, 460–476. doi: 10.1111/j.1525-142X.2011.00503.x
Herring, S. W. (1993). “Epigenetic and functional influences on skull growth,” in The Skull, ed J. Hanken and B. K. Hall (Chicago: University of Chicago Press), 153–206.
Hildebrand, M., Bramble, D. M., Liem, K. F., and Wake, D. B. (1985). Functional Vertebrate Morphology. Cambridge, MA: Harvard University Press.
Ishizeki, K., Kagiya, T., Fujiwara, N., Otsu, K., and Harada, H. (2010). Biological significance of site-specific transformation of chondrocytes in mouse meckel's cartilage. J. Oral Biosci. 52, 136–142. doi: 10.1016/S1349-0079(10)80042-8
Ishizuya-Oka, A., and Shi, Y. B. (2007). Regulation of adult intestinal epithelial stem cell development by thyroid hormone during Xenopus laevis metamorphosis. Dev. Dyn. 236, 3358–3368. doi: 10.1002/dvdy.21291
Iwasawa, H., and Kera, Y. (1980). Normal stages of development of the japanese lungless salamander, onychodactylus japonicus (houttuyn). Jap. J. Herpetol 8, 73–89. doi: 10.5358/hsj1972.8.3_73
Jandzik, D., Garnett, A. T., Square, T. A., Cattell, M. V., Yu, J. K., and Medeiros, D. M. (2015). Evolution of the new vertebrate head by co-option of an ancient chordate skeletal tissue. Nature 518, 534–537. doi: 10.1038/nature14000
Jandzik, D., Hawkins, M. B., Cattell, M. V., Cerny, R., Square, T. A., and Medeiros, D. M. (2014). Roles for fgf in lamprey pharyngeal pouch formation and skeletogenesis highlight ancestral functions in the vertebrate head. Development 141, 629–638. doi: 10.1242/dev.097261
Jeffery, J. E., Bininda-Emonds, O. R. P., Coates, M. I., and Richardson, M. K. (2005). A new technique for identifying sequence heterochrony. Systemat. Biol. 54, 230–240. doi: 10.1080/10635150590923227
Jollie, M. (1980). Development of head and pectoral fin girdle skeleton and scales in Acipenser. Copeia 1980, 226–249. doi: 10.2307/1444000
Jollie, M. (1984a). Development of cranial and pectoral girdle bones of Lepisosteus with a note on scales. Copeia 476–502. doi: 10.2307/1445204
Jollie, M. (1984b). Development of the head and pectoral skeleton of Amia with a note on scales. Gegenbaurs morphologisches Jahrbuch 130, 315–351.
Jollie, M. (1984c). Development of the head and pectoral skeleton of Polypterus with a note on scales (pisces: Actinoptergii). J. Zool. 204, 469–507. doi: 10.1111/j.1469-7998.1984.tb02382.x
Kaucka, M., Zikmund, T., Tesarova, M., Gyllborg, D., Hellander, A., Jaros, J., et al. (2017). Oriented clonal cell dynamics enables accurate growth and shaping of vertebrate cartilage. eLife 6:25902. doi: 10.7554/eLife.25902
Kemp, N. E., and Hoyt, J. A. (1969). Sequence of ossification in the skeleton of growing and metamorphosing tadpoles of Rana pipiens. J. Morphol. 129, 415–444. doi: 10.1002/jmor.1051290404
Kerney, R., Wassersug, R., and Hall, B. K. (2010). Skeletal advance and arrest in giant non-metamorphosing african clawed frog tadpoles (Xenopus laevis: Daudin). J. Anat. 216, 132–143. doi: 10.1111/j.1469-7580.2009.01176.x
Koyabu, D., Werneburg, I., Morimoto, N., Zollikofer, C. P. E., Forasiepi, A. M., Endo, H., et al. (2014). Mammalian skull heterochrony reveals modular evolution and a link between cranial development and brain size. Nat. Commun. 5:3625. doi: 10.1038/ncomms4625
Kruger, N., Measey, J., Herrel, A., and Secondi, J. (2019). Anti-predator strategies of the invasive african clawed frog, Xenopus laevis, to native and invasive predators in western france. Aquat. Invasions 14, 433–443. doi: 10.3391/ai.2019.14.3.03
Lannoo, M. J. (1987). Neuromast topography in anuran amphibians. J. Morphol. 191, 115–129. doi: 10.1002/jmor.1051910203
Lebedkina, N. S. (1960a). Development of the bones of the palatal arch in the caudate amphibia. Doklady 131, 218–220.
Lebedkina, N. S. (1960b). Development of the parasphenoid in the caudate amphibia. Doklady 133, 539–542.
Lebedkina, N. S. (1964). Development of the nasal bones in the caudate amphibia. Doklady 159, 787–790.
Lebedkina, N. S. (1968). “The development of bones in the skull roof of amphibia,” in Current Problems of Lower Vertebrate Phylogeny, ed T. Orvig (Stockholm: Interscience Publ.), 317–329.
Leloup, J., and Buscaglia, M. (1977). La triiodothyronine, hormone de la métamorphose des amphibiens. Comptes Rendus des séances de l'Academie des Sciences, Série D 284, 2261–2263.
Limbaugh, B. A., and Volpe, E. P. (1957). Early development of the gulf coast toad, Bufo valliceps wiegmann. Amer. Museum Novitates 1842, 1–32.
Ma, Q., Liu, S. F., Wang, X. X., Xiang, Z. L., and Zhuang, Z. M. (2019). Skeletal development of the chondrocranium in the tongue sole Cynoglossus semilaevis (Pleuronectiformes: Cynoglossidae). J. Fish Biol. 94, 223–230. doi: 10.1111/jfb.13870
Mabee, P. M., and Trendler, T. A. (1996). Development of the cranium and paired fins in Betta splendens (Teleostei: Percomorpha): intraspecific variation and interspecific comparisons. J. Morphol. 227, 249–287. doi: 10.1002/(SICI)1097-4687(199603)227:3<249::AID-JMOR1>3.0.CO;2-1
Maglia, A. M. (2003). Skeletal development of Pelobates cultripes and a comparison of the osteogenesis of pelobatid frogs (anura: Pelobatidae). Nat. Hist. Museum Univ. Kansas Sci. Pap. 30, 1–13. doi: 10.5962/bhl.title.10142
Markle, D. F. (1984). Phosphate buffered formalin for long term preservation of formalin fixed ichthyoplankton. Copeia 1984, 525–528. doi: 10.2307/1445208
Maxwell, E. E. (2008). Ossification sequence of the avian order anseriformes, with comparison to other precocial birds. J. Morphol. 269, 1095–1113. doi: 10.1002/jmor.10644
Maxwell, E. E., Harrison, L. B., and Larsson, H. C. E. (2010). Assessing the phylogenetic utility of sequence heterochrony: evolution of avian ossification sequences as a case study. Zoology 113, 57–66. doi: 10.1016/j.zool.2009.06.002
Maxwell, E. E., and Larsson, H. C. E. (2009). Comparative ossification sequence and skeletal development of the postcranium of palaeognathous birds (aves: Palaeognathae). Zool. J. Linnean Soc. 157, 169–196. doi: 10.1111/j.1096-3642.2009.00533.x
Miller, G. S. (1923). The telescoping of the cetacean skull (with eight plates). Smithson. Miscellan. Collect. 76, 1–68.
Mitgutsch, C., Wimmer, C., Sánchez-Villagra, M. R., Hahnloser, R., and Schneider, R. A. (2011). Timing of ossification in duck, quail, and zebra finch: lntraspecific variation, heterochronies, and life history evolution. Zool. Sci. 28, 491–500. doi: 10.2108/zsj.28.491
Miyauchi, H., LaRochelle, F. T. Jr., Suzuki, M., Freeman, M., and Frieden, E. (1977). Studies on thyroid hormones and their binding in bullfrog tadpole plasma during metamorphosis. Gen. Comp. Endocrinol. 33, 254–266. doi: 10.1016/0016-6480(77)90250-7
Mondou, P. M., and Kaltenbach, J. C. (1979). Thyroxine concentrations in blood serum and pericardial fluid of metamorphosing tadpoles and of adult frogs. Gen. Comp. Endocrinol. 39, 343–349. doi: 10.1016/0016-6480(79)90131-X
Moore, M. K., and Townsend, V. R. Jr. (2003). Intraspecific variation in cranial ossification in the tailed frog, ascaphus truei. J. Herpetol. 37, 714–717. doi: 10.1670/246-01N
Murray, P. D. F. (1936). Bones. A Study of the Development and Structure of the Vertebrate Skeleton. Cambridge: Cambridge University Press.
Newman, R. A. (1989). Developmental plasticity of Scaphiopus couchii tadpoles in an unpredictable environment. Ecology 70, 1775–1787. doi: 10.2307/1938111
Nieuwkoop, P. D., and Faber, J. (1956). Normal table of Xenopus laevis (daudin). A systematical and chronological survey of the development from the fertilized egg till the end of metamorphosis. Amsterdam: North-Holland Publishing Company.
Norman, M. F. (1985). A practical method for staging metamorphis in the tiger salamander Ambystoma tigrinum. Anat. Rec. 211, 102–109. doi: 10.1002/ar.1092110115
Nunn, C. L., and Smith, K. K. (1998). Statistical analyses of developmental sequences: the craniofacial region in marsupial and placental mammals. Amer. Natur. 152, 82–101. doi: 10.1086/286151
Ollonen, J., Da Silva, F. O., Mahlow, K., and Di-Poï, N. (2018). Skull development, ossification pattern, and adult shape in the emerging lizard model organism Pogona vitticeps: a comparative analysis with other squamates. Front. Physiol. 9:278. doi: 10.3389/fphys.2018.00278
Pehrson, T. (1940). The development of dermal bones in the skull of Amia calva. Acta Zool. 21, 1–50. doi: 10.1111/j.1463-6395.1940.tb00338.x
Pehrson, T. (1944). Some observations on the development and morphology of the dermal bones in the skull of Acipenser and Polyodon. Acta Zool. 25, 27–48. doi: 10.1111/j.1463-6395.1944.tb00343.x
Pehrson, T. (1949). The ontogeny of the lateral line system in the head of dipnoans. Acta Zool. 30, 153–182. doi: 10.1111/j.1463-6395.1949.tb00505.x
Pehrson, T. (1958). The early ontogeny of the sensory lines and the dermal skull in Polypterus. Acta Zool. 39, 241–258. doi: 10.1111/j.1463-6395.1958.tb00387.x
Petranka, J. W. (1998). Salamanders of the United States and Canada. Washington: Smithsonian Institution Press.
Pilkington, J. B., and Simkiss, K. (1966). The mobilization of the calcium carbonate deposits in the endolymphatic sacs of metamorphosing frogs. J. Exp. Biol. 45:329.
Pinet, K., Deolankar, M., Leung, B., and McLaughlin, K. A. (2019). Adaptive correction of craniofacial defects in pre-metamorphic Xenopus laevis tadpoles involves thyroid hormone-independent tissue remodeling. Development 146:dev175893. doi: 10.1242/dev.175893
Polachowski, K. M., and Werneburg, I. (2013). Late embryos and bony skull development in Bothropoides jararaca (Srpentes, Viperidae). Zoology 116, 36–63. doi: 10.1016/j.zool.2012.07.003
Regard, E., Taurog, A., and Nakashima, T. (1978). Plasma thyroxine and triiodothyronine levels in spontaneously metamorphosing Rana catesbeiana tadpoles and in adult anuran amphibia. Endocrinology 102, 674–684. doi: 10.1210/endo-102-3-674
Regueira, E., Dávila, C., and Hermida, G. N. (2016). Morphological changes in skin glands during development in Rhinella arenarum (Anura: Bufonidae). Anat. Rec. 299, 141–156. doi: 10.1002/ar.23284
Reiss, J. O. (1989). The meaning of developmental time: a metric for comparative embryology. Amer. Nat. 134, 170–189. doi: 10.1086/284974
Richman, J. M., Buchtová, M., and Boughner, J. C. (2006). Comparative ontogeny and phylogeny of the upper jaw skeleton in amniotes. Dev. Dyn. 235, 1230–1243. doi: 10.1002/dvdy.20716
Rieppel, O. (1993a). Studies on skeleton formation in reptiles: patterns of ossification in the skeleton of Chelydra serpentina (Reptilia, Testudines). J. Zool. 231, 487–509. doi: 10.1111/j.1469-7998.1993.tb01933.x
Rieppel, O. (1993b). Studies on skeleton formation in reptiles. V. Patterns of ossification in the skeleton of Alligator mississippiensis daudin (Reptilia, Crocodylia). Zool. J. Linnean Soc. 109, 301–325. doi: 10.1111/j.1096-3642.1993.tb02537.x
Rieppel, O. (1994). Studies on skeleton formation in reptiles: patterns of ossification in the skeleton of Lacerta agilis exigua eichwald (Reptilia, Squamata). J Herpetol. 28, 145–153. doi: 10.2307/1564613
Rose, C. S. (1995). Skeletal morphogenesis in the urodele skull: III. Effect of hormone dosage in TH-induced remodelling. J. Morphol. 223, 243–261. doi: 10.1002/jmor.1052230303
Rose, C. S. (1996). An endocrine-based model for developmental and morphogenetic diversification in metamorphic and paedomorphic urodeles. J. Zool. 239, 253–284. doi: 10.1111/j.1469-7998.1996.tb05451.x
Rose, C. S. (1999). “Hormonal control in larval development and evolution—Amphibians,” in The Origin and Evolution of Larval Forms, eds B. K. Hall and M. H. Wake (San Diego: Academic Press), 167–216. doi: 10.1016/B978-012730935-4/50007-9
Rose, C. S. (2003a). “The developmental morphology of salamander skulls,” in Amphibian Biology, vol. 5. Osteology, eds H. Heatwole and M. Davies (Chipping Norton, Australia: Surrey Beatty and Son Pty. Ltd.), 1686–1783.
Rose, C. S. (2003b). “Thyroid hormone-mediated development in vertebrates: What makes frogs unique?” in Environment, Development and Evolution, eds G. B. Müller, B. K. Hall and R. D. Pearson (Boston: MIT), 197–237.
Rose, C. S. (2005). Integrating ecology and developmental biology to explain the timing of frog metamorphosis. TREE 20, 129–134. doi: 10.1016/j.tree.2005.01.005
Rose, C. S. (2014a). Caging, but not air deprivation, slows tadpole growth and development in the amphibian Xenopus laevis. J. Exp. Zool. A Ecol. Genetics Physiol. 321, 365–375. doi: 10.1002/jez.1867
Rose, C. S. (2014b). The importance of cartilage to amphibian development and evolution. Int. J. Dev. Biol. 58, 917–927. doi: 10.1387/ijdb.150053cr
Rose, C. S., Aleagha, O., Modolo, C., and Hoguet, N. (2018). Xenopus tails are unique in combining whip-like lateral undulations and vertical extension and flexion. Int. Comp. Biol. 58:E409.
Rose, C. S., and Cahill, J. W. (2019). How thyroid hormones and their inhibitors affect cartilage growth and shape in the frog xenopus laevis. J. Anat. 234, 89–105. doi: 10.1111/joa.12897
Rose, C. S., and James, B. (2013). Plasticity of lung development in the amphibian, Xenopus laevis. Biol. Open 2, 1324–1335. doi: 10.1242/bio.20133772
Rose, C. S., Murawinski, D., and Horne, V. (2015). Deconstructing cartilage shape and size into contributions from embryogenesis, metamorphosis, and tadpole and frog growth. J. Anat. 226, 575–595. doi: 10.1111/joa.12303
Rose, C. S., and Reiss, J. O. (1993). “Metamorphosis and the vertebrate skull: ontogenetic patterns and developmental mechanisms,” in The Skull, eds J. Hanken and B. K. Hall (Chicago: University of Chicago Press), 289–346.
Rugh, R. (1962). Experimental Embryology Techniques and Procedures. Minneapolis, MN: Burgess Publishing Co. doi: 10.5962/bhl.title.6412
Sachs, L. M., and Buchholz, D. R. (2019). Insufficiency of thyroid hormone in frog metamorphosis and the role of glucocorticoids. Front. Endocrinol. 10:287. doi: 10.3389/fendo.2019.00287
Sánchez-Villagra, M. R. (2002). Comparative patterns of postcranial ontogeny in therian mammals: an analysis of relative timing of ossification events. J. Exp. Zool. 294, 264–273. doi: 10.1002/jez.10147
Sánchez-Villagra, M. R., Goswami, A., Weisbecker, V., Mock, O., and Kuratani, S. (2008). Conserved relative timing of cranial ossification patterns in early mammalian evolution. Evol. Dev. 10, 519–530. doi: 10.1111/j.1525-142X.2008.00267.x
Schneider, R. A. (2018). Neural crest and the origin of species-specific pattern. Genesis 56, 1–33. doi: 10.1002/dvg.23219
Schreiber, A. M. (2006). Asymmetric craniofacial remodeling and lateralized behavior in larval flatfish. J. Exp. Biol. 209, 610–621. doi: 10.1242/jeb.02056
Schreiber, A. M., Cai, L., and Brown, D. D. (2005). Remodeling of the intestine during metamorphosis of Xenopus laevis. PNAS 102, 3720–3725. doi: 10.1073/pnas.0409868102
Sedra, S. N., and Michael, M. I. (1957). The development of the skull, visceral arches, larynx and visceral muscles of the south african clawed toad, Xenopus laevis (daudin) during the process of metamorphosis (from stage 55 to stage 66). Verhandelingen der Koninklijke Nederlandse Akademie van Wetenschappen, Afdeling Natuurkunde. Tweede Reeks 51, 1–80.
Senevirathne, G., Kerney, R., and Meegaskumbura, M. (2017). Comparative postembryonic skeletal ontogeny in two sister lineages of old world tree frogs (Rhacophoridae: Taruga, polypedates). PLoS ONE 12:167939. doi: 10.1371/journal.pone.0167939
Serrat, M. A. (2014). Environmental temperature impact on bone and cartilage growth. Comp. Physiol. 4, 621–655. doi: 10.1002/cphy.c130023
Sheil, C. A., Jorgensen, M., Tulenko, F., and Harrington, S. (2014). Variation in timing of ossification affects inferred heterochrony of cranial bones in lissamphibia. Evol. Dev. 16, 292–305. doi: 10.1111/ede.12092
Sherratt, E., Vidal-García, M., Anstis, M., and Keogh, J. S. (2017). Adult frogs and tadpoles have different macroevolutionary patterns across the australian continent. Nat. Ecol. Evol. 1, 1385–1391. doi: 10.1038/s41559-017-0268-6
Shi, D., and Boucaut, J. (1995). The chronological development of the urodele amphibian Pleurodeles waltl (michah). Int. J. Dev. Biol. 39, 427–441.
Shi, Y.-B. (2000). Amphibian Metamorphosis: From Morphology to Molecular Biology. New York, NY: John Wiley & Sons.
Simon, M. N., and Marroig, G. (2017). Evolution of a complex phenotype with biphasic ontogeny: contribution of development versus function and climatic variation to skull modularity in toads. Ecol. Evol. 7, 10752–10769. doi: 10.1002/ece3.3592
Slater, B. J., Liu, K. J., Kwan, M. D., Quarto, N., and Longaker, M. T. (2009). Cranial osteogenesis and suture morphology in Xenopus laevis: a unique model system for studying craniofacial development. PLoS ONE 4:e3914. doi: 10.1371/journal.pone.0003914
Smirnov, S. V. (1992). The influence of variation in larval period on adult cranial diversity in Pelobates fuscus (Anura: Pelobatidae). J. Zool. 226, 601–612. doi: 10.1111/j.1469-7998.1992.tb07503.x
Smirnov, S. V., Merkulova, K. M., and Vassilieva, A. B. (2020). Skull development in the iberian newt, Pleurodeles waltl (Salamandridae: Caudata: Amphibia): timing, sequence, variations, and thyroid hormone mediation of bone appearance. J. Anatomy 237, 543–555. doi: 10.1111/joa.13210
Smirnov, S. V., and Vassilieva, A. B. (2009). Number of ossification centers in the anuran cranial bones depends upon the rate of development: experimental evidence. Russian J. Herptol. 16, 167–176.
Smirnov, S. V., and Vassilieva, A. B. (2014). Thyroid hormones in the skeletogenesis and accessory sources of endogenous hormones in Xenopus laevis (Amphibia; Anura) ontogeny: experimental evidence. Doklady Biol. Sci. 455, 136–138. doi: 10.1134/S0012496614020185
Smith, K. K. (1997). Comparative patterns of craniofacial development in eutherian and metatherian mammals. Evolution 51, 1663–1678. doi: 10.1111/j.1558-5646.1997.tb01489.x
Sterner, Z. R., Shewade, L. H., Mertz, K. M., Sturgeon, S. M., and Buchholz, D. R. (2020). Glucocorticoid receptor is required for survival through metamorphosis in the frog Xenopus tropicalis. Gen. Comp. Endocrinol. 291:113419. doi: 10.1016/j.ygcen.2020.113419
Strauss, R. E. (1990). Heterochronic variation in the developmental timing of cranial ossifications in poeciliid fishes (Cyprinodontiformes). Evolution 44, 1558–1567. doi: 10.1111/j.1558-5646.1990.tb03846.x
Strauss, R. E. (1999). Brain-tissue accumulation of fluorescent age pigments in four poeciliid fishes (Cyprinodontiformes) and the estimation of “biological age.” Growth Dev. Aging 63, 151–170.
Suzuki, S., and Suzuki, M. (1981). Changes in thyroidal and plasma lodine compounds during and after metamorphosis of the bullfrog, Rana catesbeiana. Gen. Comp. Endocrinol. 45, 74–81. doi: 10.1016/0016-6480(81)90171-4
Tata, J. R., Kawahara, A., and Baker, B. S. (1991). Prolactin inhibits both thyroid hormone-induced morphogenesis and cell death in cultured amphibian larval tissues. Dev. Biol. 146, 72–80. doi: 10.1016/0012-1606(91)90447-B
Taylor, A. C., and Kollros, J. J. (1946). Stages in the normal development of Rana pipiens larvae. Anat. Rec. 94, 7–23. doi: 10.1002/ar.1090940103
Tchernavin, V. (1938). Changes in the salmon skull. Trans. Zool. Soc. London 24, 103–184. doi: 10.1111/j.1096-3642.1938.tb00390.x
Tejedo, M. (1993). Size-dependent vulnerability and behavioral responses of tadpoles of two anuran species to beetle larvae predators. Herpetologica 49, 287–294.
Terry, G. S. (1918). Effects of the extirpation of the thyroid gland upon ossification in Rana pipiens. J. Exp. Zool. 24, 567–587. doi: 10.1002/jez.1400240306
Trueb, L. (1966). Morphology and development of the skull in the frog Hyla septentrionalis. Copeia 1966, 562–573. doi: 10.2307/1441083
Trueb, L. (1985). A summary of osteocranial development in anurans with notes on the sequence of cranial ossification in Rhinophrynus dorsalis (Anura: Pipoidea: Rhinophrynidae). S. African J. Sci. 81, 181–185.
Trueb, L. (1993). “Patterns of cranial diversity among the lissamphibia,” in The Skull eds J. Hanken and B. K. Hall (Chicago: University of Chicago Press), 255–343.
Trueb, L., and Hanken, J. (1992). Skeletal development in Xenopus laevis (Anura: Pipidae). J. Morphol. 214, 1–41. doi: 10.1002/jmor.1052140102
Vandenberg, L. N., Adams, D. S., and Levin, M. (2012). Normalized shape and location of perturbed craniofacial structures in the xenopus tadpole reveal an innate ability to achieve correct morphology. Dev. Dyn. 241, 863–878. doi: 10.1002/dvdy.23770
Velhagen, W. A. Jr. (1997). Analyzing developmental sequences using sequence units. System. Biol. 46, 204–210. doi: 10.1093/sysbio/46.1.204
Wada, H., Ghysen, A., Satou, C., Higashijima, S.-,i., Kawakami, K., Hamaguchi, S., et al. (2010). Dermal morphogenesis controls lateral line patterning during postembryonic development of teleost fish. Dev. Biol. 340, 583–594. doi: 10.1016/j.ydbio.2010.02.017
Wagemans, F., and Vandewalle, P. (2001). Development of the bony skull in common sole: brief survey of morpho-functional aspects of ossification sequence. J. Fish Biol. 59, 1350–1369. doi: 10.1111/j.1095-8649.2001.tb00197.x
Wake, D. B. (1980). Evidence of heterochronic evolution: a nasal bone in the olympic salamander, Rhyacotriton olympicus. J. Herpetol. 14, 292–295. doi: 10.2307/1563553
Walker, M. B., and Kimmel, C. B. (2007). A two-color acid-free cartilage and bone stain for zebrafish larvae. Biotech. Histochem. 82, 23–28. doi: 10.1080/10520290701333558
Wassersug, R. J., and Hoff, K. (1982). “Developmental changes in the orientation of the anuran jaw suspension,” in Evolutionary Biology, eds M. K. Hecht, B. Wallace and G. T. Prance (New York, NY: Plenum), 223–246. doi: 10.1007/978-1-4615-6968-8_5
Wassersug, R. J., and Seibert, E. A. (1975). Behavioral responses of amphibian larvae to variation in dissolved oxygen. Copeia 1975, 86–103. doi: 10.2307/1442410
Wassersug, R. J., and Sperry, D. G. (1977). The relationship of locomotion to differential predation of Pseudacris triseriata (Anura: Hylidae). Ecology 58, 830–839. doi: 10.2307/1936218
Weil, M. R. (1986). Changes in plasma thyroxine levels during and after spontaneous metamorphosis in a natural population of the green frog, Rana clamitans. Gen. Comp. Endocrinol. 62, 8–12. doi: 10.1016/0016-6480(86)90088-2
Weisbecker, V., and Mitgutsch, C. (2010). A large-scale survey of heterochrony in anuran cranial ossification patterns. J. Zool. Systematics Evolut. Res. 48, 332–347. doi: 10.1111/j.1439-0469.2010.00570.x
Werneburg, I., Polachowski, K. M., and Hutchinson, M. N. (2015). Bony skull development in the argus monitor (Squamata, Varanidae, Varanus panoptes) with comments on developmental timing and adult anatomy. Zoology 118, 255–280. doi: 10.1016/j.zool.2015.02.004
White, B. A., and Nicoll, C. S. (1981). “Hormonal control of amphibian metamorphosis,” in Metamorphosis: A Problem in Developmental Biology, eds L. I. Gilbert and E. Frieden (New York, NY: Plenum Press), 363–396. doi: 10.1007/978-1-4613-3246-6_11
Wilbur, H. M., and Collins, J. P. (1973). Ecological aspects of amphibian metamorphosis. Science 182, 1305–1314. doi: 10.1126/science.182.4119.1305
Wild, E. R. (1997). Description of the adult skeleton and developmental osteology of the hyperossified horned frog, Ceratophrys cornuta (Anura: Leptodactylidae). J. Morphol. 232, 169–206. doi: 10.1002/(SICI)1097-4687(199705)232:2<169::AID-JMOR4>3.0.CO;2-5
Wild, E. R. (1999). Description of the chondrocranium and osteogenesis of the chacoan burrowing frog, Chacophrys pierotti (Anura: Leptodactylidae). J. Morphol. 242, 229–246. doi: 10.1002/(SICI)1097-4687(199912)242:3<229::AID-JMOR3>3.0.CO;2-N
Woronowicz, K. C., and Schneider, R. A. (2019). Molecular and cellular mechanisms underlying the evolution of form and function in the amniote jaw. EvoDevo 10:8. doi: 10.1186/s13227-019-0131-8
Yaoita, Y. (2019). Tail resorption during metamorphosis in Xenopus tadpoles. Front. Endocrinol. 10:143. doi: 10.3389/fendo.2019.00143
Yaoita, Y., and Brown, D. D. (1990). A correlation of thyroid hormone receptor gene expression with amphibian metamorphosis. Genes Dev. 4, 1917–1924. doi: 10.1101/gad.4.11.1917
Keywords: amphibians, bone, skeleton, thyroid hormone, metamorphosis, ossification sequence, plasticity, variability
Citation: Rose CS (2021) Amphibian Hormones, Calcium Physiology, Bone Weight, and Lung Use Call for a More Inclusive Approach to Understanding Ossification Sequence Evolution. Front. Ecol. Evol. 9:620971. doi: 10.3389/fevo.2021.620971
Received: 24 October 2020; Accepted: 11 January 2021;
Published: 01 February 2021.
Edited by:
Fernando Casares, Andalusian Center for Development Biology (CABD), SpainReviewed by:
Ivan Gomez-Mestre, Estación Biológica de Doñana (EBD), SpainAlexander Schreiber, St. Lawrence University, United States
Copyright © 2021 Rose. This is an open-access article distributed under the terms of the Creative Commons Attribution License (CC BY). The use, distribution or reproduction in other forums is permitted, provided the original author(s) and the copyright owner(s) are credited and that the original publication in this journal is cited, in accordance with accepted academic practice. No use, distribution or reproduction is permitted which does not comply with these terms.
*Correspondence: Christopher S. Rose, cm9zZWNzJiN4MDAwNDA7am11LmVkdQ==