- 1Department of Biological Sciences, University of the Andes, Bogotá, Colombia
- 2Smithsonian Tropical Research Institute, Panama, Panama
- 3Coiba AIP, Clayton, Panama
- 4School of Natural Resources and the Environment, University of Arizona, Tucson, AZ, United States
- 5Department of Ecology and Evolutionary Biology, University of Arizona, Tucson, AZ, United States
- 6BC3 – Basque Centre for Climate Change, Scientific Campus of the University of the Basque Country, Leioa, Spain
- 7IKERBASQUE – Basque Foundation for Science, Bilbao, Spain
Páramos, tropical alpine ecosystems, host one of the world’s most diverse alpine floras, account for the largest water reservoirs in the Andes, and some of the largest soil carbon pools worldwide. It is of global importance to understand the future of this extremely carbon-rich ecosystem in a warmer world and its role on global climate feedbacks. This study presents the result of the first in situ warming experiment in two Colombian páramos using Open-Top Chambers. We evaluated the response to warming of several ecosystem carbon balance-related processes, including decomposition, soil respiration, photosynthesis, plant productivity, and vegetation structure after 3 years of warming. We found that OTCs are an efficient warming method in the páramo, increasing mean air temperature by 1.7°C and mean daytime temperature by 3.4°C. The maximum air temperature differences between OTC and control was 23.1°C. Soil temperature increased only by 0.1°C. After 3 years of warming using 20 OTC (10 per páramo) in a randomized block design, we found no evidence that warming increased CO2 emissions from soil respiration, nor did it increase decomposition rate, photosynthesis or productivity in the two páramos studied. However, total C and N in the soil and vegetation structure are slowly changing as result of warming and changes are site dependent. In Sumapaz, shrubs, and graminoids cover increased in response to warming while in Matarredonda we observed an increase in lichen cover. Whether this change in vegetation might influence the carbon sequestration potential of the páramo needs to be further evaluated. Our results suggest that páramos ecosystems can resist an increase in temperature with no significant alteration of ecosystem carbon balance related processes in the short term. However, the long-term effect of warming could depend on the vegetation changes and how these changes alter the microbial soil composition and soil processes. The differential response among páramos suggest that the response to warming could be highly dependent on the initial conditions and therefore we urgently need more warming experiments in páramos to understand how specific site characteristics will affect their response to warming and their role in global climate feedbacks.
Introduction
The Earth is warming at an accelerated rate. The temperature is projected to increase 1.0–4.8°C by the end of this century (IPCC, 2014), with mountains experiencing a higher rise in temperature than the low-lands (Bradley et al., 2004, 2006). A recent compilation from 302 European mountain sites and 145 years of observations by Steinbauer et al. (2018) clearly shows accelerated warming and associated vegetation changes in mountain summits with unpredictable consequences to the ecosystem function and services they provide. In the tropics, projected to experience large changes in climate (Williams et al., 2007), the data void from mountain sites precludes us from understanding how the climate and vegetation have been changing (Feeley, 2015). The Andean highlands are already getting warmer (Vuille et al., 2015) and are projected to warm by 3–5°C by the end of this century (Urrutia and Vuille, 2009; Buytaert et al., 2011). There is building evidence that some tree species are already migrating upward and shrinking their distribution range (Pauli et al., 1996; Feeley et al., 2011; Duque et al., 2015; Báez et al., 2016). However, since not all species move at the same pace (Root et al., 2003; Walther et al., 2005), we could expect to see new plant communities form with yet unforeseen consequences to the whole region’s biological diversity and ecosystem functioning (Báez et al., 2016). Whether the summits of the tropics are suffering floristic changes induced by the climate has yet to be detected through initiatives like GLORIA (The Global Observation Research Initiative in Alpine Environments) (Pauli et al., 2001), whose Andean branch has recently started to monitor floristic and climatic changes in the high tropical Andes (Cuesta et al., 2017). One piece of evidence that suggests vegetation is also shifting in the mountain summits of the tropics comes from the work by Cuesta et al. (2019) that documented important landscape transformations in the Andean highlands, including upward shifts of species and the formation of new communities in deglaciated forefronts (see also Zimmer et al., 2018). The consequences of these changes on species distribution, carbon storage, and ecosystem services across Andean alpine ecosystems are a pressing question that needs to be addressed.
Páramo ecosystems can be found at the top of several isolated mountains in the Northern Andean regions, Costa Rica, and Panamá and are considered biodiversity hotspots (Myers et al., 2000), important centers of speciation (Madriñán et al., 2013), crucial for local water supply, agriculture and hydropower (Buytaert et al., 2011), and important carbon sinks in the tropics (Hofstede, 1999; Hribljan et al., 2017). Páramos are usually found at elevations that range between 2800 and 4700 m above sea level and cover approximately an area of 41,700 km2 (Kappelle, 2005; Llambí and Cuesta, 2014; Sklenář et al., 2014). Páramos are characterized by low-temperature conditions during night time, sometimes below zero, and cool to warm conditions during the day. Some páramos are relatively dry, but many can be described as high-elevation wetlands, given that some areas are waterlogged throughout part of the year. These environmental conditions, e.g., low temperatures and waterlogged soils, have led to low decomposition rates and a large buildup of organic matter through centuries (Podwojewski et al., 2002; Poulenard et al., 2003). Consequently, páramos are one of the most important carbon sinks in the tropics, storing up to six-fold more carbon per area than tropical forests (Hofstede, 1999), and globally they contain some of the largest stocks of soil organic carbon (Curiel Yuste et al., 2017). However, climatic factors that have favored soil organic carbon accumulation are likely to change by the end of this century, potentially shifting páramos from sinks to greenhouse gas emissions sources. Even though there is plenty of evidence that warming and changes in precipitation are already affecting biodiversity, decomposition rates, and soil carbon turnover in other biomes such as forests, mesic grasslands, and tundra (Cregger et al., 2014; Chen et al., 2015; Xue et al., 2016), tropical alpine regions are only beginning to receive greater attention (Buytaert et al., 2011). Páramo ecosystems could play a disproportionate role in driving climate feedbacks resulting from increased CO2 release from their large soil carbon stores (Buytaert et al., 2011). Therefore, understanding how warming will impact carbon flux-related processes is of global importance.
Two recent publications highlight the potentially compounded aboveground and belowground challenges that would reduce soil carbon stocks. In the tropics, large carbon reservoirs could emit much carbon from soil respiration into the atmosphere with warming (Nottingham et al., 2020). Furthermore, there is evidence that accelerated growth in response to warming results in shorter tree lifespans, suggesting that increases in forest carbon stocks may be short-lived (Brienen et al., 2020). Results from a warming experiment in the tropical lowland forest of Panamá indicate that warming could increase the release of CO2 from the soil by as much as 55% and thereby become a potent source of CO2 in a warmer world (Nottingham et al., 2020). Likewise, there is a robust body of literature on the response of many carbon fluxes-related processes to changing temperatures that shows temperature increases soil respiration (Raich and Schlesinger, 1992; Bond-Lamberty and Thomson, 2010), litter decomposition (Hobbie, 1996; Cornelissen et al., 2001; Aerts, 2006), N mineralization and nitrification (MacDonald et al., 1995; Dalias et al., 2002; but see Emmett et al., 2004), plant nutrient uptake, and plant productivity and fine root dynamics (Bassirirad, 2000; Gill and Jackson, 2000). However, it is still unclear how whole ecosystems will respond to warming and which ecosystems will be more affected. The general notion is that high-latitude and high-elevation ecosystems will be more affected by warming than equatorial and low-elevation ecosystems (Pepin et al., 2015; Wang et al., 2016) and the ecosystem responses would depend on the initial conditions of the carbon and nitrogen stocks, the soil water content, the size, and longevity of plants and their turnover rate (Shaver et al., 2000).
The recognition of the necessity to understand ecosystem responses to global warming has fueled a series of temperature-manipulation experiments worldwide that have been mainly concentrated in high-latitude sites. Two flagship examples are: (1) the Network of Ecosystem Warming Studies, which aims to evaluate ecosystem-level effects of rising temperature using a variety of warming methods in the tundra, low tundra, grassland, and forests at high-latitudes and elevation and (2) the ITEX (International Tundra Experiment) a network that has been warming circumpolar and high-elevation ecosystems using a common design of open-top chambers (Marion, 1997). Data from these warming experiments have been synthesized in various meta-analysis (e.g., Arft et al., 1999; Rustad et al., 2001; Aerts, 2006; Elmendorf et al., 2012; Lu et al., 2013), and have shown that warming can significantly alter soil and plant processes and ecosystem carbon cycling and storage (Melillo et al., 2002; McGuire et al., 2009; Craine et al., 2010; Conant et al., 2011; Schaefer et al., 2011). The meta-analysis of high-latitude ecosystems by Rustad et al. (2001) shows that warming can increase soil respiration by 20%, N mineralization rate by 46%, and plant productivity by 19%. A later meta-analysis by Lu et al. (2013) showed that although warming can stimulate soil respiration and carbon emission processes, it can also stimulate plant-derived carbon influxes like gross ecosystem photosynthesis by 15.7%, net primary productivity (NPP) by 4.4%, and plant C pools from above- and belowground parts by around 7.0%. Those carbon influxes offset the increases in warming-induced carbon efflux, indicating that climate change, specifically warming, may not always trigger strong positive carbon-climate feedback from terrestrial ecosystems. None of those large networks and meta-analysis includes tropical sites, and in situ warming experiments are urgently needed in the tropics to have a better picture of carbon-climate feedbacks from all terrestrial ecosystems (Cavaleri et al., 2015).
In the tropics, responses could be quite different from what has been observed in higher latitudes (Wood et al., 2019). The continuous growing season and higher temperature of the tropics are ideal for decomposers (Schlesinger and Andrews, 2000). Although temperatures are not higher in tropical alpine environments than in temperate alpine environments, they experience strong temperature amplitude all year round because of the absence of snow (e.g., Vuille et al., 2018). Some of the greatest soil respiration rates are found in the tropics, where tropical forests can contribute around 30% of the total global soil-derived CO2 (Raich et al., 2002). However, because of the continuous growing season in moist and wet tropics, any negative effect of warming on decomposition and soil respiration could be offset if warming also increases plant productivity and carbon influxes all year around (Nemani et al., 2003). Therefore, the direction and magnitude of feedbacks could be different in the tropics. Two warming experiments were recently set in the tropical lowlands in Puerto Rico (Kimball et al., 2018; Kennard et al., 2020) and Panamá (Nottingham et al., 2020) and are starting to provide interesting data to understand how different the tropics may respond to warming. There are few in situ warming experiments running in Ecuador and Colombia in the tropical cold highlands, but no data have yet been published. However, translocation experiments set up along an elevation gradient in the Peruvian Andes (Salinas et al., 2011; Zimmermann and Bird, 2012; Nottingham et al., 2015) have provided some evidence of the temperature sensibility of soil organic matter decomposition in these high-elevation grasslands, which are drier than the páramos. Our previous work with páramos’ incubated soils also points to a strong soil microbial responses to artificial warming (Curiel Yuste et al., 2017). These findings suggest that the response of heterotrophic respiration to warming in tropical mountain ecosystems could be stronger than predicted. More studies are needed to fill the unexplored gap in our understanding of carbon dynamics in páramos, where large quantities of soil organic carbon are present (Hofstede, 1999; Curiel Yuste et al., 2017; Hribljan et al., 2017).
In this study, we evaluated how warming affected several processes related to carbon emission and sequestration. We followed ITEX protocol using hexagonal open-top chambers (OTC), a standardized passive warming methodology used at sites that are difficult to access and have no access to power (Marion, 1997). We evaluated two key components of the global carbon budget; decomposition rate and annual CO2 emissions from soil respiration, a process known to be one of the main sources of CO2 to the atmosphere. We also evaluated carbon influx processes like photosynthesis and plant productivity (below and aboveground); and soil properties that could eventually lead to changes in those processes like soil water content, soil pH, carbon, phosphorous, and nitrogen pools. Additionally, we studied the warming effect on the vegetation structure to evaluate whether warming could alter the carbon sequestration potential trough vegetation changes, as has been reported in other cold biomes in the artic where woody shrub dominance has increased (Tape et al., 2006), and with it the carbon sequestration potential of the ecosystems. We hypothesize that in these high-elevation páramo ecosystems limited by temperature and nutrients (Hofstede, 1995), the increasing temperature would increase rates of litter decomposition, rates of soil respiration, net N mineralization, resulting in greater soil nutrient availability, and consequently plant growth and greater biomass production and net primary productivity (NPP). Moreover, we hypothesize that warming will favor shrubs over other growth forms since this group dominates warmer sites in the sub-páramo (Buytaert et al., 2011), has a physiological tolerator behavior in other páramos (Rada et al., 2019), and climate-driven shrub expansion has been amply documented across tundra and temperate alpine ecosystems (McDougall, 2003; Tape et al., 2006; Anthelme et al., 2007; Brandt et al., 2013; Zhou et al., 2020). How much and in what directions will warming alter these different processes will be key to understanding the future role of the páramos as carbon reservoirs.
Materials and Methods
Study Site
The warming experiments were conducted at two sites in the North-eastern Andean range in Colombia (Figure 1). Both páramos are part of the Cruz Verde-Sumapaz complex and are surrounded by a matrix of forest, roads, and agriculture. One site is Matarredonda, located 3300 m above sea level, with annual precipitation of 1178 mm and a mean annual temperature of 8.8°C. The minimum temperature we have registered in Matarredonda is −2.0°C and the maximum is 35.0°C. The experimental site in Matarredonda is 1 km away from the road and 2.5 km from the closest agriculture field. The second site is in Sumapaz, near the military base “Batallón de Alta Montaña N° 1” at 3400 m above sea level with annual precipitation of 1469 mm and a mean annual temperature of 7.6°C. The minimum temperature we have registered in Sumapaz is −4.5°C and the maximum is 39.0°C. In Sumapaz there are no asphalted roads close to the experimental site, only gravel roads located >2 km from the OTC. The distance between both páramo sites is approximately 96 km (in a straight line), but 7 h driving. In both sites, the vegetation is dominated by tussock grasses, especially Calamagostri effusa, intermixed with forbs, stem, and basal rosettes, including the páramo’s endemic genus Espeletia, and sclerophyllous shrubs, with bryophytes and lichens commonly found in the prostrate vegetation strata. The soil type for both sites is Bh3-3b (FAO, 2009) with quaternary volcanic ashes and outflows, Paleozoic metamorphic and intrusive igneous rocks. In Matarredonda the soil has been classified as typic Hapludands while in Sumapaz as typic Dystrocryepts and typic Haplocryands (IGAC, 2000). A list of the most common species at the study sites are presented in Supplementary Table 1.
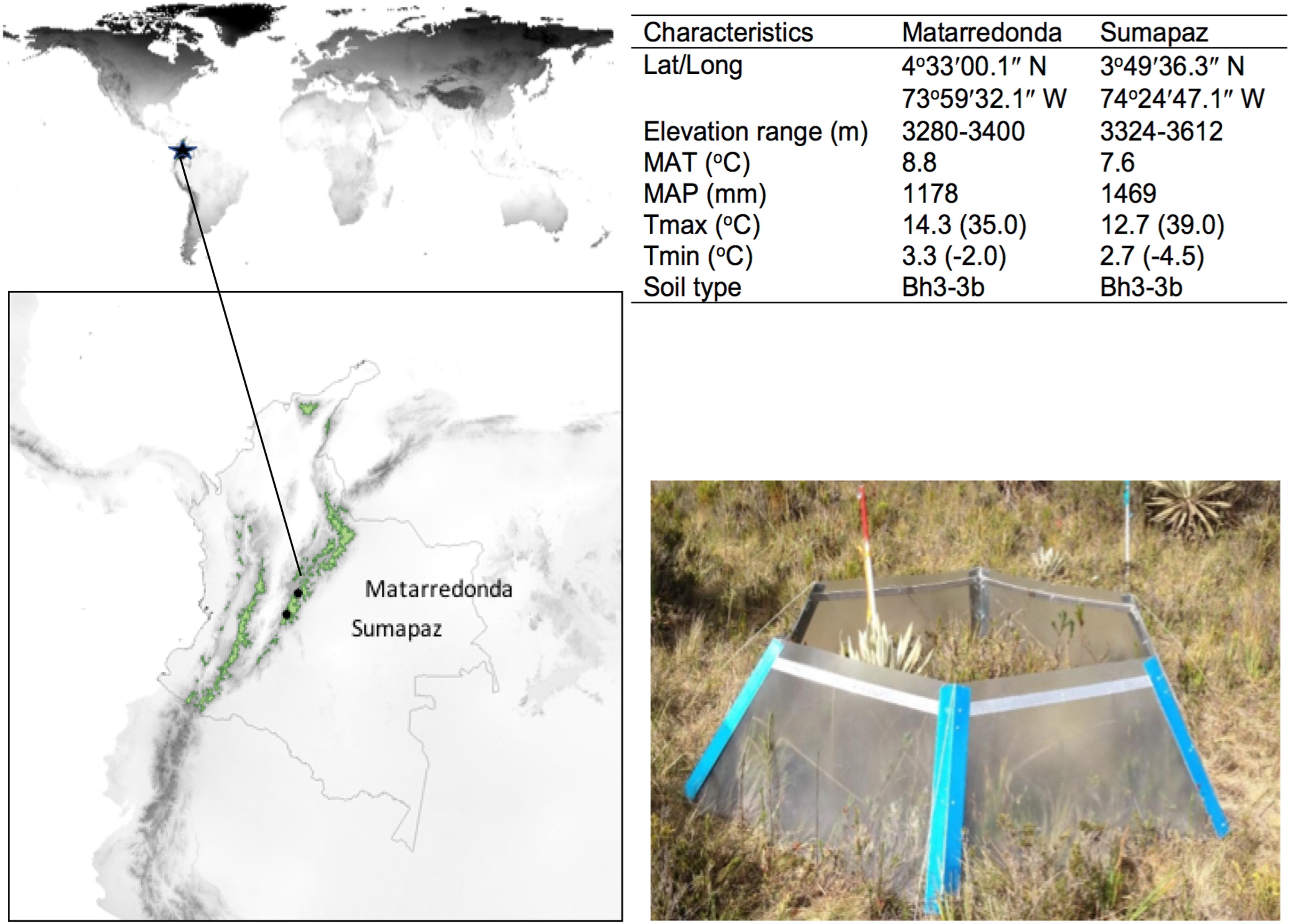
Figure 1. Location, characteristics of the study sites and OTC design. Páramos of Colombia are depicted in green. Climatic variables were downloaded from http://www.worldclim.org/bioclim and the maximum and minimum temperatures registered by our temperature sensors during 2017–2019 are shown in parenthesis.
Experimental Warming
Climatic warming was simulated using fiberglass hexagonal open-top chambers (OTC) following the ITEX (International Tundra Experiment) design (Figure 1). OTC act both as windshields and solar traps increasing ambient air and soil temperature (Marion, 1997). OTCs were constructed using six pieces of 0.040-inch-thick fiberglass Sun-Lite HP (Solar Components Corporation, Manchester, NH, United States) attached at 60° angles, with a side-to-side diameter of 2.08 m and 60 cm height, covering an area of 3.87 m2 and attached to the ground by UV-resistant wires. This warming method is the preferred warming method on remote places and high-mountain tops without access to electrical power and has been successfully used in more than 20 experiments by the ITEX group.
Before establishing the plots, we searched the páramo for flat sites (with less than 15° of inclination) with similar vegetation of less than 60 cm in height. We marked two similar sites within 1–2 m distance to assign them the treatment (OTC and non-manipulated plot as its control) within the same block following a complete randomized block design. The distance between adjacent blocks was about 4–8 m. We included in the plots the most common species of the páramo and at least one individual of Espeletia, an iconic dominant genus of the páramo. A preliminary analysis considering the species’ identity and its percentage of coverage showed that the plots assigned to each treatment within a block had similar vegetation at the beginning of the experiment (Supplementary Figure 2 and Supplementary Table 1). The average slope in all plots in Sumapaz was 10°, and the average aspect 125.9°. In Matarredonda, the average slope was 6.8°, and the average aspect of 166.6°. Slopes and aspects were estimated using GRASS GIS software and the digital elevation model at 30 m spatial resolution from the shuttle radar topography mission (Farr et al., 2007). The OTC installation was completed in June 2016.
Environmental Monitoring
To quantify whether the OTCs are modifying the environment, we monitored ambient air temperature, air humidity, soil temperature, and soil water potential in and out the OTC using an automatic recording systems in Matarredonda that consisted of an EM-50 datalogger (Decagon Devices, Inc., Pullman, WA, United States) connected to two VP-4 humidity and temperature sensors with a radiation shield (Decagon Devices, Inc., Pullman, WA, United States) and two MPS-6 soil temperature and water potential sensors (Decagon Devices, Inc., Pullman, WA, United States). The ambient air sensor was set at 30 cm above the soil surface, which is the height of many of the plants in the OTC, and the soil sensor at 10 cm belowground. Data were recorded every 30 min from December 2017 to December 2019. This monitoring was done in only one OTC and its control paired plot in Matarredonda. Simultaneous records of temperature, relative humidity, and atmospheric pressure were used to calculate air vapor pressure deficit (VPD) using the equation as in Ficklin and Novick (2017). VPD is a direct measure of the atmospheric drying power and is defined as the difference between the water vapor in the air at saturation and the actual amount of water vapor.
To confirm that all OTC are functioning as warming devices, we measured the leaf temperature of Espeletia species in all 40 plots. We took thermographic images with a Fluke® Ti32 infrared thermal camera (Fluke, Avery, WA, United States) from healthy mature leaves of two common endemic rosettes species from the páramo, Espeletia grandiflora (10 pairs of plots) in Sumapaz, Espeletia argentea (six pairs of plots), and Espeletia grandiflora (four pairs of plots) in Matarredonda. Photos were taken at midday on a sunny day between September and October 2016, 4 months after establishing the experiment. Using the software SmartView 3.15TM the temperature of the leaf was extracted from the thermal image of the whole leaf.
Effect of Warming in Processes Related to CO2 Emissions
Decomposition Rate
To evaluate whether warming could alter decomposition processes and lead to an increase in carbon losses from the páramo, we used the litterbag method proposed by Powers et al. (2009) where litter mass loss of one gram of substrate was followed for 2 years. We used two substrates; bay leaves, Laurus nobilis (Lauraceae) a standard substrate that has been used on litter decomposition experiments across the tropics (Powers et al., 2009), and “frailejón” leaves, Espeletia grandiflora (Asteraceae) an endemic páramo species belonging to one of the predominant genera in the South American páramo. E. grandiflora retain their dead leaves around the stem and they were cut from the plant avoiding collecting eaten or diseased leaves. Litterbags (15 cm × 10 cm) of 1 mm nylon mesh were filled with 1.0 ± 0.1 g of air-dried UV sterilized substrate. In July 2016, 1 month after establishing the OTC chambers, 12 bags were deployed randomly (six from each species) on each plot. Bags were anchored to the surface of the soil using metallic wire. One bag per species per plot was collected at 1, 3, 6, 12, 18, and 24 months after deployment. The litter samples were then cleaned up, dried (55°C, 72 h), and weighed. To compare our values with the pantropical study on decomposition rate of Powers et al. (2009), we calculated the annual decomposition rate (k) following their protocol. That is, k was calculated as the slope of the linear regression of the natural logarithm of percent mass remaining versus time, forcing the Y-intercepts through 100% mass remaining at time 0. k values are presented as absolute values for ease of interpretation, where high k indicate steeper slopes and therefore higher decomposition rates. The response variable decay rate (k) was log-transformed before the analysis to meet assumptions of homogeneity of variance.
Soil Respiration
To evaluate whether warming could increase carbon losses from páramos’ soil, we measured soil CO2 fluxes using an infrared gas analyzer (EGM-5, PP Systems, Amesbury, MA, United States), connected to its soil respiration chamber (SRC-2) and soil temperature probe (STP-2). Two PVC collars (11 cm diameter × 8 cm height) were installed in each plot in February 2017 on bare soil at a depth of 4 cm, after carefully scraping away litter. Fluxes were measured by attaching the respiration chamber to the soil collar every 2–3 months in the late morning early afternoon, between 10:00 AM and 2:00 PM, starting February 2017 until June 2019. Before every measurement, vegetation encroached in the collar was removed. The soil respiration values that we obtained represent the combined respiration of soil micro- and macro-organisms and plant roots. Air and soil temperatures were measured at the same time as the CO2 efflux. Measurements of CO2 built up within the system were performed during 60 s and respiration was calculated from the slope of the increase in CO2 concentration as a function of time. The volume of the PVC collar was added to the soil chamber’s volume to correct the final flux estimate.
Physical, Chemical and Biological Properties of the Soil
To evaluate whether warming changes soil properties like water content, bulk density, pH, total N, P, and C, we randomly collected, 36 months after establishing the experiment, two undisturbed soil cores at 20 cm depth per plot for a total of 40 cores per study site. Samples were then sieved to remove debris, roots, and soil particles >2 mm. Gravimetric water content was estimated from a subsample by weighting the sample before and after being oven-dried for 24 h at 105°C. For pH determination, 3 g of sieved soil were dissolved in 15 ml of distilled water for 30 min before reading was taken. The remaining soil sample was sent for total carbon and nitrogen (elemental analyzer), organic carbon (Walkley Black method and colorimetric), ammonia and nitrate (volumetric KCl 2M extraction), and phosphorous analysis (Bray II method) to the Soil and Water Laboratory from the Agricultural Sciences Faculty in Universidad Nacional de Colombia. Bulk density was calculated from the dry soil weight, divided by the core volume. We calculated soil organic carbon (SOC) stocks in the first 20 cm depth of soil by multiplying soil bulk density, soil depth, and % of organic carbon.
Effect of Warming on Processes Related to CO2 Capture
Photosynthesis
To evaluate whether warming can potentially change the carbon influxes through carbon assimilation by the vegetation, we measured maximum net photosynthetic rate (Amax) and dark respiration (Rd, a measure of the leaf level autotrophic respiration) with a portable infrared gas analyzer Li-COR 6400XT (Li-Cor Inc., Lincoln, NE, United States). We selected in both páramos one species of the Espeletia genus, which is the most iconic, abundant and diverse genus in the páramo (Monasterio and Sarmiento, 1991; Madriñán et al., 2013; see Supplementary Table 1) and considered one of the most vulnerable to climatic change (Buytaert et al., 2011; Peyre et al., 2020; Valencia et al., 2020). Additionally, we selected two other abundant forbs species, that were commonly found in most of the OTCs and their paired control plots that are also endemic to the páramo. Measurements were taken 1 year after the establishment of the OTC in Espeletia grandiflora Humb. & Bonpl. (n = 8) and Orthrosanthus chimboracensis (Kunth) Baker. (n = 8) in Sumapaz and in Espeletia argentea Humb. & Bonpl. (n = 7), and Valeriana pilosa Ruiz & Pav. (n = 7) in Matarredonda. Then again, after 2 years for E. grandiflora in Sumapaz. Measurements were taken in upper mature fully expanded healthy leaf, setting the IRGA at: 420 μmol mol–1 reference CO2, and a constant flow of 500 μmol s–1 at ambient humidity. For Amax leaves were exposed to 2000 μmol photons m–2 s–1 of light until stability was reached, and light curves were constructed with seven light points: 2000, 1500, 1000, 500, 250, 100, and 0 μmol photons m–2 s–1 of light. Values of Amax and Rd values were obtained from fitted curves after testing the best model (Lobo et al., 2013).
Net Ecosystem Exchange
Net ecosystem (CO2) exchange (NEE) was measured using the chamber technique in the third year of warming in two spots inside the OTC and in two spots in the control plots. Measurements were taken in low stature vegetation that could be fit inside the chamber, on spots with similar species and vegetation cover on each paired plot. Dominant vegetation included juveniles of Espeletia, prostrated shrubs like Pernettya prostrata, small upright shrubs like Diplostephium and Arcytophyllum, some mosses, sedges, and grasses in the genus Carex and Calamagrostis, and forbs like Valeriana, Orthrosanthus, Nertera, and Castratella. Species were selected based on high abundance, though species that grow larger than the chamber diameter were excluded (Supplementary Table 1).
The changes in CO2 concentration were measured using the EGM-5 Portable CO2 infrared gas analyzer and its transparent canopy assimilation chamber CPY-5 (PP Systems International, Inc., MA, United States). The chamber is made of polycarbonate and includes a photosynthetically active radiation sensor, an air temperature sensor, an air mixing fan and a manifold system that ensures uniform circulation within the chamber. The chamber is 145 mm in height × 146 mm diameter and covers a surface area of 167 cm2. At each spot, we took measurements in light and in darkness. In light, we measured net ecosystem production (NEE), which is the carbon sequestration by photosynthesis minus the carbon release from plant and soil microbial respiration. The dark measurement was taken after aeration of the chamber by covering it with a dark cloth that reduces the incoming light to zero; under this condition we measured the ecosystem (plant plus soil) respiration (Reco). The total uptake of carbon through photosynthesis or gross ecosystem production (GEP) was then calculated as GEP = NEE + Reco. Measurements were taken every second for 75 s. Initially, we worked on longer time series, but we had large variations in CO2 concentration at the end, suggesting that we started to see the effect of lateral diffusion. After several trials, 75 s was finally estimated to be the best time series to obtain a reliable concentration time series to fit a linear equation, and estimate the flux from the slope. In this study, positive values indicate an uptake of CO2 by the ecosystem from the atmosphere and negative values represent release of CO2 from the ecosystem to the atmosphere.
Above- and Below-Ground Biomass
Belowground productivity was estimated at the community level using the ingrowth core technique (Li et al., 2013), that is, intact soil cores were removed from the ground and replaced with an equivalent volume of root-free soil enclosed in a mesh cylinder. In August 2016, two soil cores (5 cm diameter × 20 cm deep) were randomly extracted from each plot for a total of 40 soil cores (20 in OTC and 20 in control plots) per study site. The cored holes were then filled with the polyethylene net cylinders (5 cm diameter × 15 cm depth; mesh size 3.5 mm) previously filled with sterilized root-free soil. Given the low productivity of the páramos, ingrowth cores were left in the sites for a whole year and retrieved afterward. Cores were brought back to the laboratory, and fine roots were manually removed and rinsed with water to remove soil particles. Cleaned roots were then oven dried at 70°C until constant weight. Estimates of fine root productivity are presented as mass (g) of fine roots produced in one square meter in 1 year.
To evaluate whether warming is affecting aboveground productivity, we harvested all the vegetation present in 1 m × 10 cm transect inside the OTCs and in their paired control plots after 3 years of warming. The transect was randomly assigned and established in one of the four outer borders of the central 1 m × 1 m permanent vegetation plot and away from the soil respiration PVC collars and other manipulations. All the vegetation in the transect was harvested, including any tall vegetation and branches within the transect, even if it was rooting outside the transect. The harvest was divided into vascular, non-vascular plant biomass and necromass. We consider as necromass all tissue and leaves that were 100% senesced and dry. When a leaf or tissue was not entirely dried, we split the tissue in two, the green tissue was added to the biomass value and the dry tissue to the necromass value. Additionally, we extracted all the roots present in the first 15 cm of the soil surface from two soil core extracted from each OTC and each control plot in the third year of warming. Soil cores were 15 cm long by 5 cm width.
Vegetation Changes
To evaluate whether the carbon sequestration potential of the páramo vegetation changes were due to changes in the dominance of growth forms, for example from herbaceous to woody species, we monitored changes in the plant community composition in the OTC and control plots. We sampled each plot’s vegetation using a 1 m × 1 m PVC frame with a grid with 100 squares (10 cm × 10 cm each square). Every square in the grid was identified with a combination of numbers (horizontally) and letters (vertically). To be able to relocate the grid on the same area every year, the grid was secured with four adjustable legs attached to four PVC tubes permanently anchored to the ground. The top center of the grid was always directed to the North and the 1 m × 1 m PVC frame occupied the center of the OTC, away from the walls. A stick of 3 mm in diameter was lowered through the upper left corner of each square until it reached the soil or lower lying vegetation and we registered each contact of the stick (a hit) with living and standing dead vegetation across all strata. Hits were recorded to the species level whenever possible or to genus level. Data were analyzed at the level of growth forms and all species were classified to one of the following forms: rosettes, shrubs, graminoids (grasses, sedges, and rushes), forbs, cushion plants, ferns, seedless vascular plants (ferns and club mosses), non-vascular plants (mosses, hornworts, and liverworts), and lichens. We also included bare soil cover and necromass (hits with standing dead vegetation) as additional categories. Vegetation was sampled upon the establishment of the OTC (in 2016) to obtain the base line vegetation cover to compare with, and then 3 years after establishing the experiment (June 2019). We obtained the cover of the major growth forms by dividing the number of hits for each species per plot by the total number of hits recorded for that plot each year. To evaluate how warming may have changed the vegetation, we calculated the change in cover as the differences in the cover found in 2019 from the cover found at the beginning of the experiment in 2016.
Statistical Analysis
For the analysis of all variables we checked for normality and homogeneity of variances using Shapiro–Wilk and Levene tests, respectively. Data were transformed before the analysis when needed. Most data were evaluated by a two-way ANOVA for a randomized block design with treatment (OTC and control) as main fixed factor and block as the second factor to control for the confounding effect of any spatial differences. Analyses were done separately for each páramo. We also evaluated the time effect on CO2 fluxes by two-way repeated measurement ANOVA for the soil respiration data. For each date, we evaluated if respiration differed between the OTCs and the control plots by a Holm–Sidak test. When normal distribution was not reached after data transformation, non-parametric statistics were applied (Mann–Whitney U-test). All analyses were performed using JMP software (JMP 9.01. SAS Institute Inc.) and SigmaPlot V.12.0 (Systat Software Inc.).
To explore general patterns on the effect of warming on all variables on both páramos we computed the effect size (d) from the means and standard deviations of the variable analyzed in OTC and control plots. The effect size was calculated as the difference between the two means (OTC and control), divided by the pooled estimate of standard deviation. The effect size was then corrected using a factor provided by Hedges and Olkin (1985) and the confidence interval for an effect size was also calculated as explained by Hedges and Olkin (1985). When 95% CI of d do not overlap with zero, the warming treatment has a significant effect; positive values indicate a positive effect of warming and negative values a negative effect of warming.
Results
Environmental Monitoring
The mean differences between the air temperature registered in the OTC with respect to the control plots was 1.7°C, the maximum air temperature differences between OTC and control was 23.1°C and the minimum air temperature differences was −1.0°C, evidencing a night-time cooling within the chambers (Figure 2 and Supplementary Figure 3) that reduced the daily mean differences. When we analyzed only daily temperatures (temperature registered between 6:00 AM and 6:00 PM) the differences in air temperature between OTCs and control was 3.4°C. Soil temperature registered at 10 cm depth was more resistant to change, with only a mean increase in the OTC with respect to the controls of 0.1°C with maximum differences of 1.6°C. The soil was more resistant to abrupt temperature changes, while the lowest air temperature registered during the study was −3°C, the lowest soil temperature registered was 8.4°C. OTCs are also slightly drying the soil, but only during the dry season with the lowest soil water potential values registered at the end of April. OTC also reduced air humidity resulting in higher values of VPD in the OTC in comparison to the control plots all year round but specially in the dry season when highest VPD values paralleled with lowest soil water potential values (Figure 2). Changes in the air temperature by the OTC also resulted in changes in the mean leaf temperature of the two species analyzed, which had warmer leaves by 3–4°C inside the OTC compared to the control plot (Supplementary Figure 1). The mean leaf temperature of Espeletia grandiflora in Sumapaz within the OTC was 29.5 ± 1.8°C, and outside the OTC was 25.6 ± 1.9°C (t = 2.81; df = 9; P = 0.01). The mean leaf temperature of Espeletia argentea in Matarredonda within the OTC was 23.1 ± 1.4°C, and outside the OTC was 19.1 ± 1.1°C (t = 3.77; df = 5; P = 0.006). The mean leaf temperature of Espeletia grandiflora in Matarredonda within the OTC was 23.6 ± 2.0°C, and outside the OTC was 18.7 ± 0.3°C (t = 2.79; df = 3; P = 0.03).
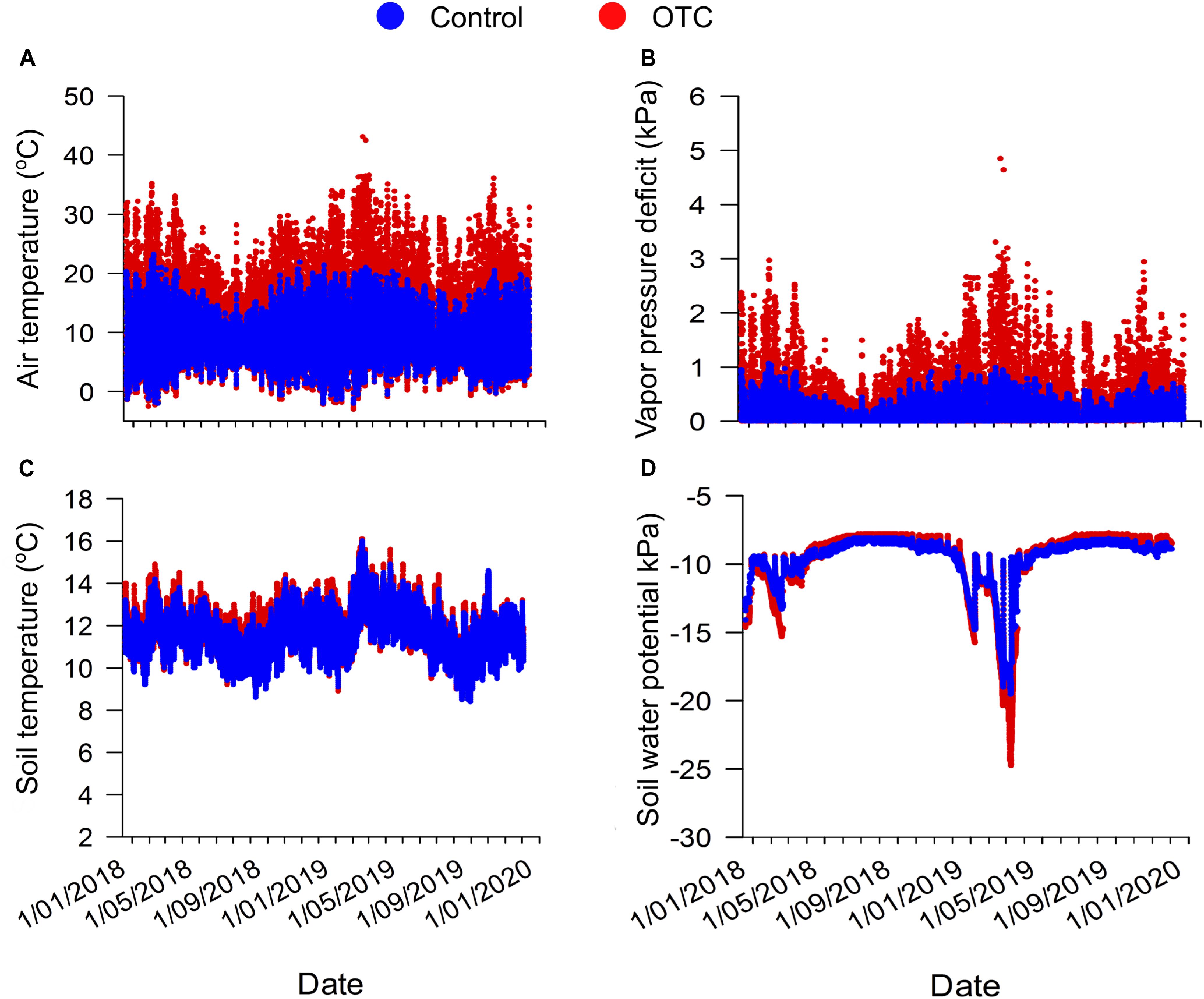
Figure 2. Climatic variables collected inside the OTC (red) and outside the OTC (blue). Air temperature (A), air vapor pressure deficit (B), soil temperature (C), and soil water potential (D) were collected every 30 min starting on December 19, 2017 until December 3, 2019 in Matarredonda.
Effect of Warming in Processes Related to CO2 Emissions
Decomposition Rate
Contrary to our initial expectation, the decomposition rates were similar or even lower in warmer plots than in control plots in both study sites (Table 1 and Figure 3). In Sumapaz, the mean decay rate for Espeletia’s substrate inside the OTC was lower than in the control plots (F = 13.4; df = 1; P = 0.005). Similarly, the decay rate for Laurel’s substrate was reduced inside the OTC (F = 15.2; df = 1; P = 0.004; Table 1). In Sumapaz, only 44% of the original material has been decomposed after 2 years in the OTC and 46–53% in the control plots (Figure 3). In Matarredonda the mean decay for Espeletia’s substrate was similar between treatments (F = 1.93; df = 1; P = 0.19; Table 1), while the decay rate for Laurel’s substrate was lower in the OTC (F = 6.48; df = 1; P = 0.03; Table 1). With this low decomposition rate, in Matarredonda, only 46–49% of the original material has been decomposed after 2 years in the OTC and 48–53% in the control plots (Figure 3).
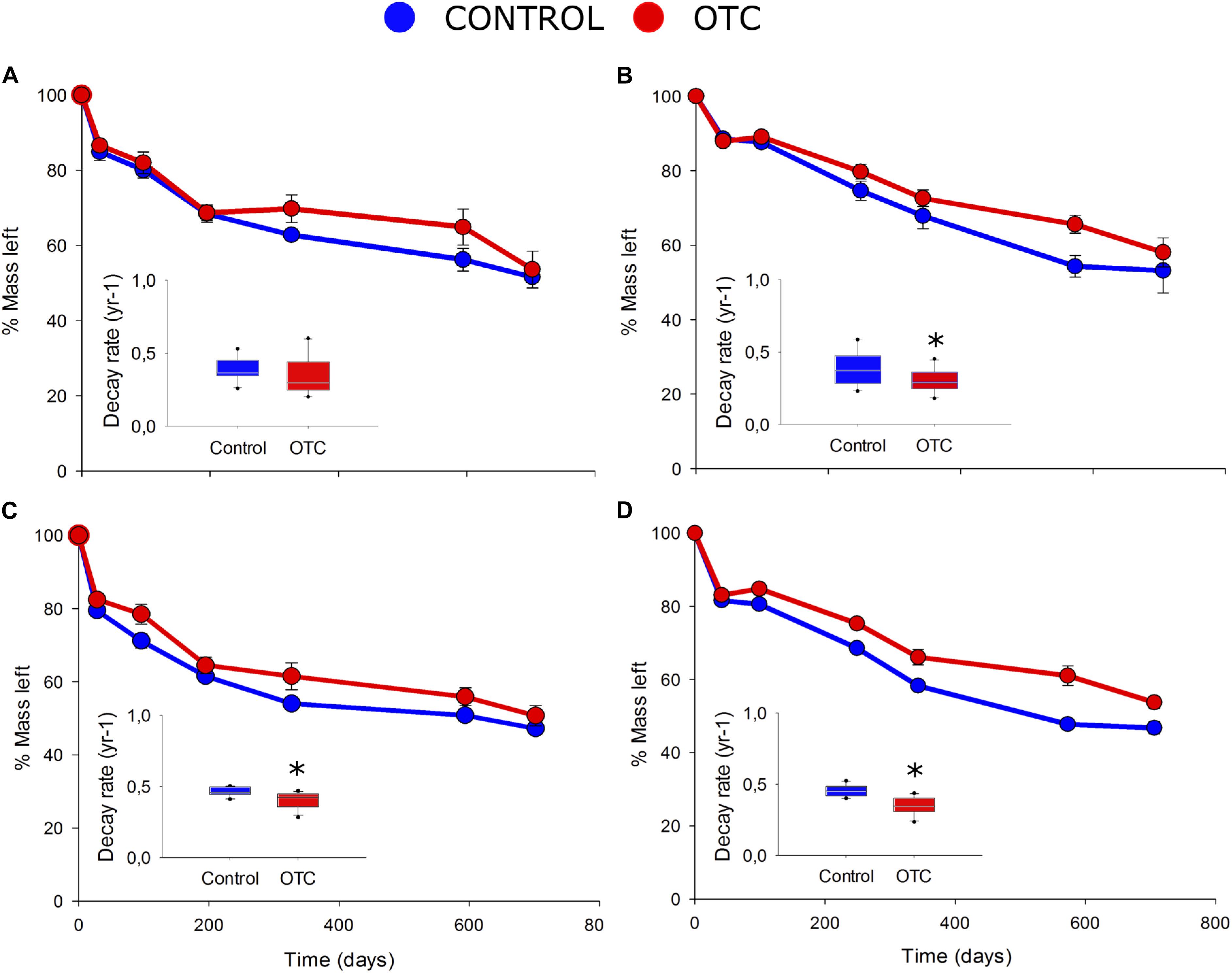
Figure 3. Percentage of litter mass left at the end of 2 years of incubation in the field (outer plot) and the decay rate k (inner plot) for Espeletia grandiflora in Matarredonda (A) and Sumapaz (B) and for Laurus nobilis in Matarredonda (C) and Sumapaz (D). Points are mean value and their error bars the standard error. Asterisk denote statistically significant differences between OTC and control plots at P < 0.05.
Soil Respiration
The regression between each of the measurements of soil respiration and its associated soil temperature shows that soil respiration increased with soil temperature in Matarredonda (temperature effect: t = 3.23; P = 0.001) and Sumapaz (temperature effect: t = 1.92; P = 0.05) but the slope of this relationship was no different between OTC and control plots in Matarredonda (t = 1.28; P = 0.20; Figure 4) and Sumapaz (t = −0.83; P = 0.40; Figure 4). Therefore, we did not find evidence that warming affects soil CO2 fluxes at any time of the year at both study sites (Figure 4). When we analyzed the seasonal pattern through a repeated ANOVA we found that in Sumapaz soil CO2 fluxes did not vary in response to warming (Treatment effect: F = 0.67; df = 1: P = 0.43), but varied seasonally (Date effect: F = 10.36; df = 4; P < 0.001; Figure 3), with the lower fluxes measured during the rainy season on June 2017 and June 2018. However, the seasonal effects on soil CO2 fluxes were not modified by warming (Treatment × date effect: F = 0.74; df = 4; P = 0.57). In Matarredonda a similar result was found where soil CO2 fluxes did not vary because of warming (Treatment effect: F = 0.09; df = 1: P = 0.77), but varied seasonally (Date effect: F = 11.58; df = 4; P < 0.001), with the lower fluxes also measured during the rainy season June 2017. Like in Sumapaz, we did not observe that warming modified seasonal CO2 fluxes (Treatment × date effect: F = 1.48; df = 4; P = 0.19).
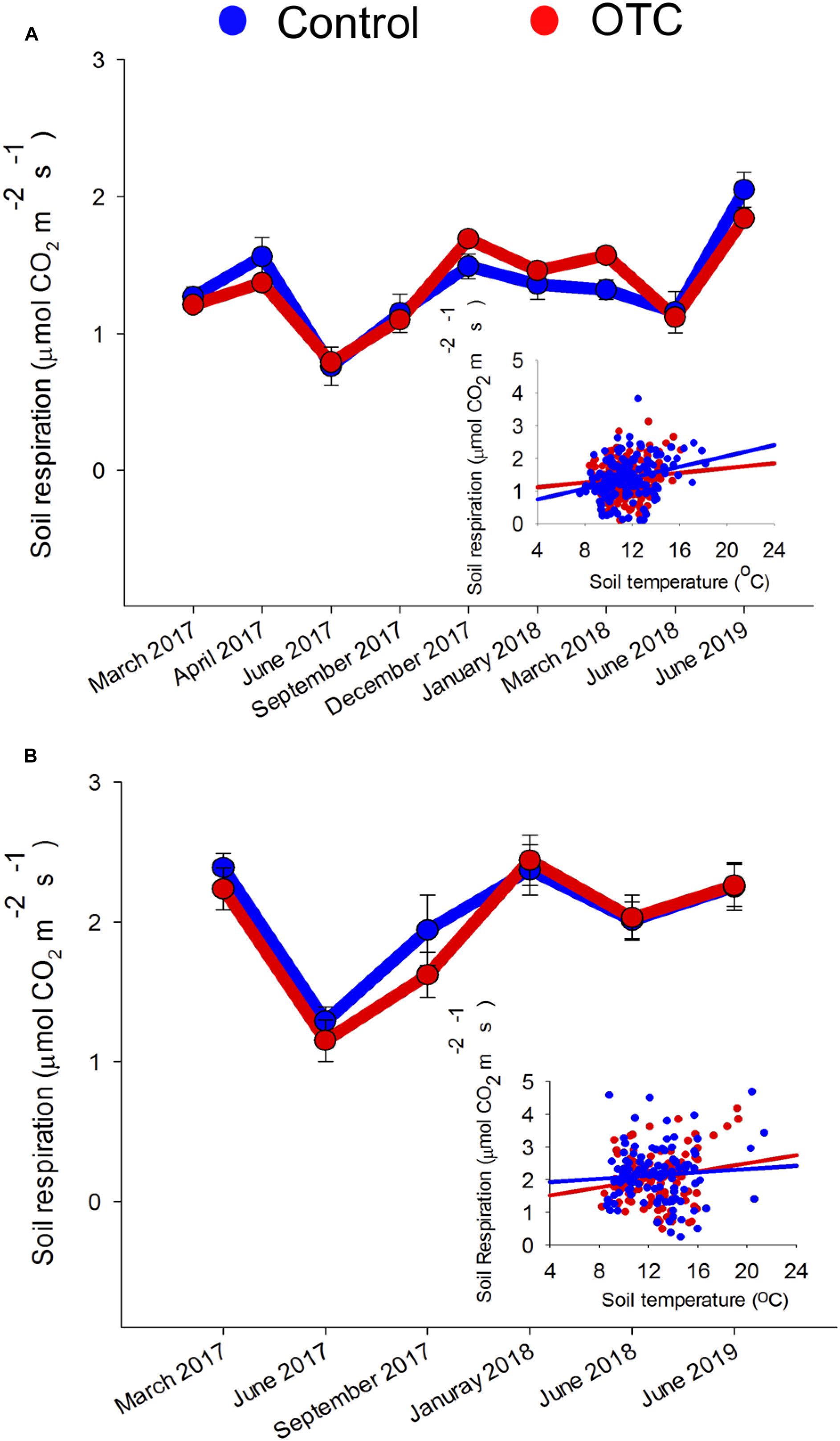
Figure 4. Average CO2 emissions from soil respiration measured inside the OTC and outside the OTC (Control) at Matarredonda (A) and Sumapaz (B). Mean values (points) and standard errors (error bars) are given for each time and warming treatment. The inner plot shows the relationship between each soil respiration measurement and its associated soil temperature and the slope of the regression in the OTC and control plots.
Physical, Chemical and Biological Properties of the Soil
Most soil physical and chemical properties have not changed as result of warming in Matarredonda, only bulk density has increased in the warmed plots (Table 1). However, in Sumapaz we saw some changes in the N and C pools resulting from warming; total carbon and total nitrogen are higher in the OTC (Table 1). Belowground productivity has not been affected by warming either, with only a marginally significant increase in the OTCs’ root production in both sites (Table 1). After 3 years or warming most soil related-processes and properties such as water content, organic carbon, SOC, pH, NH4, NO3, and soil respiration have remained the same, and show that both páramos can maintain their homeostasis with no evident short-term negative effect of warming (Table 1). If anything, warming (and the drying effect of the OTC) has slowed down decomposition rate and was slightly increasing root productivity.
Effect of Warming in Processes Related to CO2 Capture
Photosynthesis
Maximum net photosynthetic rate (Amax) and respiration in the dark (Rd), were not affected by the warming treatment in Espeletia grandiflora, Espeletia argentea, Orthrosanthus chimboracensis, and Valeriana pilosa (Table 2), suggesting that they are not modifying their photosynthetic machinery because of warming or being inhibited by the higher temperatures. Whether carbon fluxes related to photosynthesis and aboveground autotrophic respiration may change in future warming scenarios, may ultimately depend on the net assimilation rate at the community level.
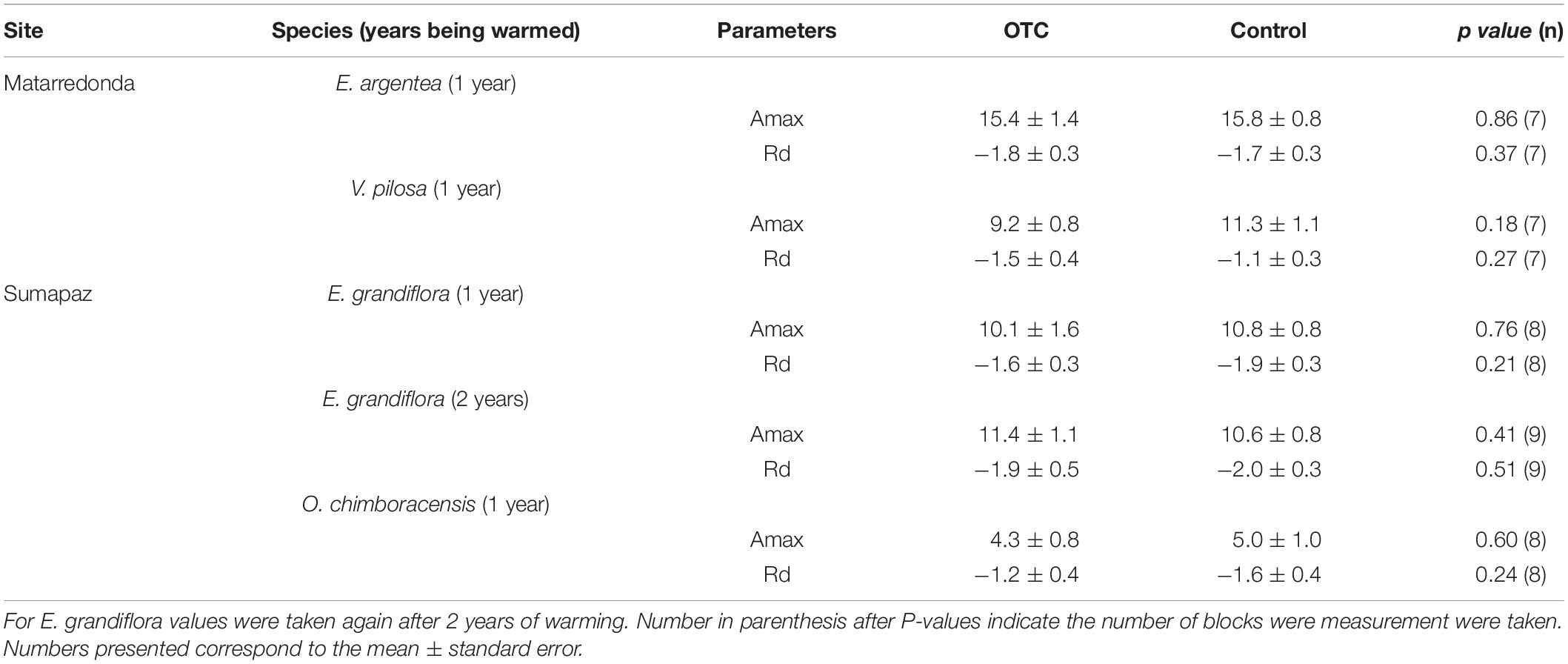
Table 2. Maximum net photosynthetic rate (Amax; μmol CO2 m–2 s–1) and dark respiration (Rd; μmol CO2 m–2 s–1), measured in leaves that developed in and out the OTC after 1 year of experimental warming.
Net Ecosystem Exchange
The CO2 fluxes measured at the small scale of the EGM chamber indicate that warming was not increasing the net carbon release from the ecosystem (Figure 5). Values of Gross primary production (GPP) or total uptake of carbon through photosynthesis after 3 years of warming were similar in the OTC and the control plots in Matarredonda (F = 0.02; df = 1; p = 0.89) and Sumapaz (F = 0.08; df = 1; P = 0.79). Values of total carbon dioxide release from autotrophic and heterotrophic respiration (Reco) were indistinguishable between OTC and controls plots in Matarredonda: (X2 = 1.42; df = 1; P = 0.23) but in Sumapaz carbon dioxide release was slightly lower in the OTC (X2 = 3.94; df = 1; P = 0.05), where less carbon was being released from respiration in the OTC in comparison to the control plots. The net ecosystem exchange (NEE) or final carbon dioxide effluxes from the ecosystem were not different between the OTC and control plots in Matarredonda (F = 1.35; df = 1; P = 0.25) nor in Sumapaz (F = 0.59; df = 1; P = 0.48).
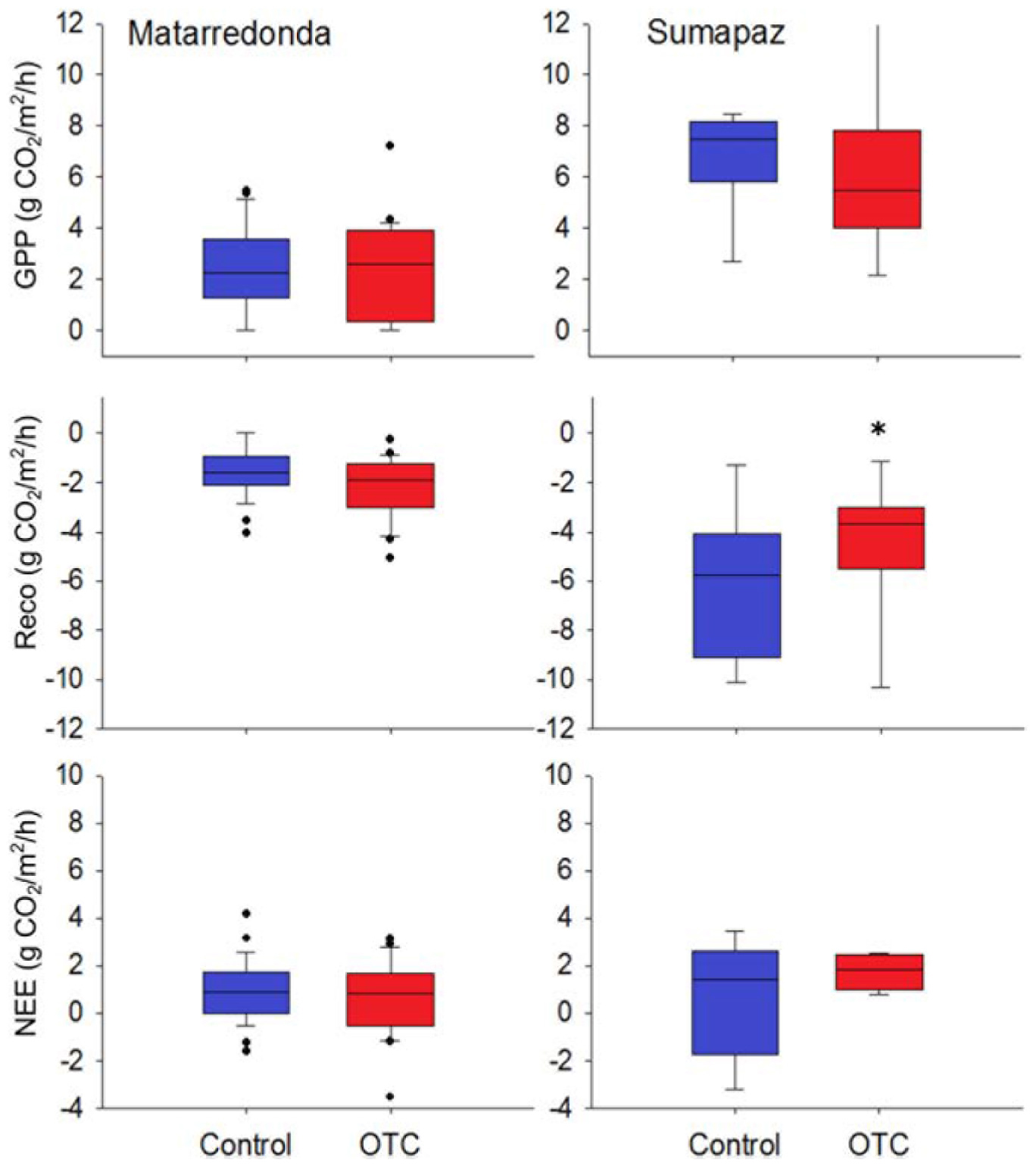
Figure 5. Gross primary production (GPP), autotrophic and heterotrophic respiration (Reco), and net ecosystem exchange (NEE) measured inside the OTC and in the control plots after 3 years of warming. All box plots show the median, 25/75th percentiles within the box, 10/90th percentiles shown within the whiskers and the outlier. Asterisk denote statistically significant differences between OTC and control plots at P < 0.05.
Above- and Below-Ground Biomass
After 3 years of warming we did not detect any effect of warming on the production of belowground biomass (Sumapaz: F = 0.59; df = 1; P = 0.45, and Matarredonda: F = 0.53; df = 1; P = 0.47), and aboveground biomass; neither from vascular (Sumapaz: X2 = 2.29; df = 1; P = 0.13, and Matarredonda: F = 0.39; df = 1; P = 0.55) nor from non-vascular plants (Sumapaz: X2 = 0.09; df = 1; P = 0.76, and Matarredonda: F = 0.03; df = 1; P = 0.88). However, the standing necromass has increased slightly in the OTC in comparison to the control plots in Sumapaz (X2 = 5.1; df = 1; P = 0.02) but not in Matarredonda (F = 1.5; df = 1; P = 0.25; Figure 6).
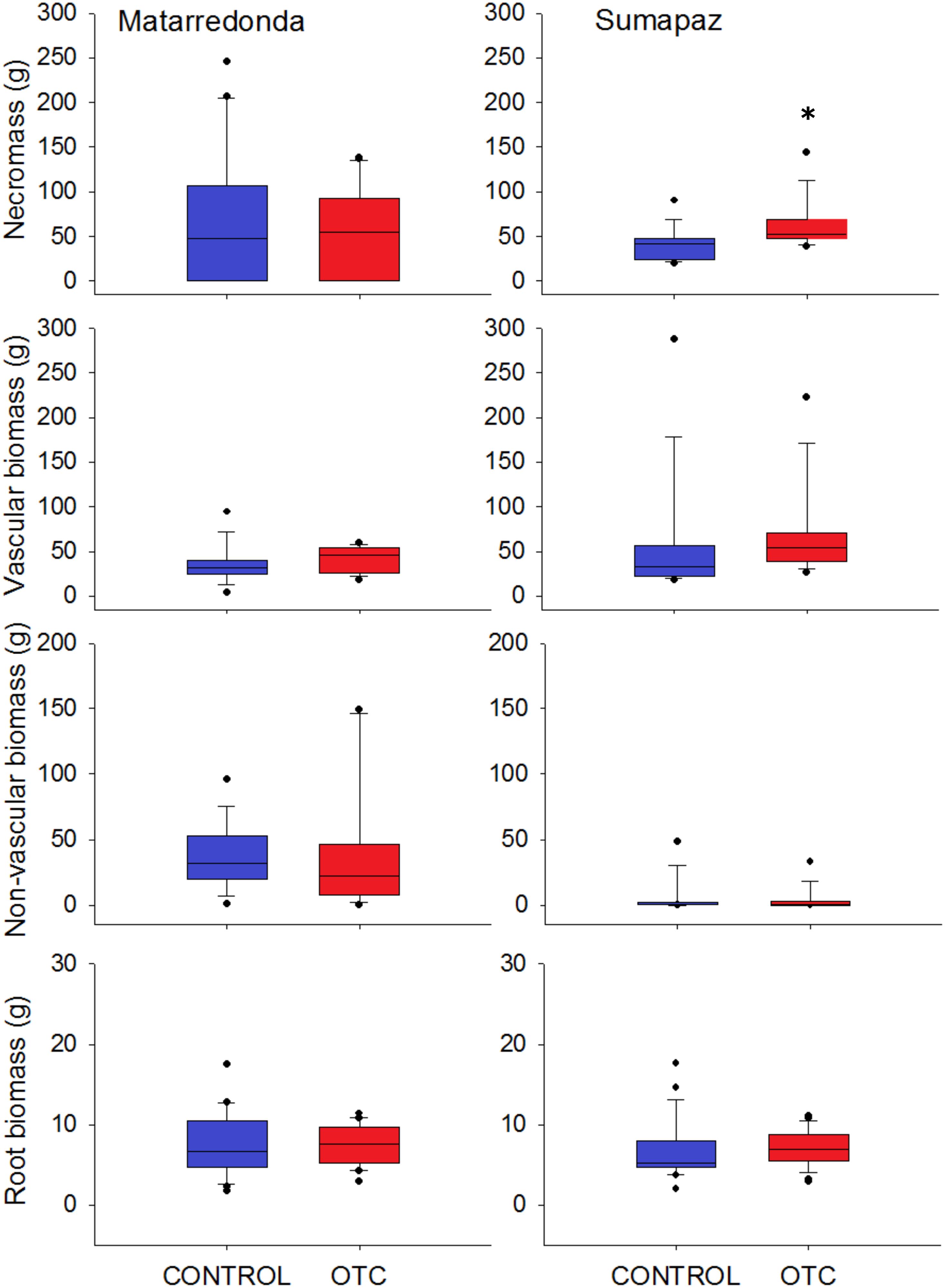
Figure 6. Aboveground biomass separated by vegetation type [vascular, non-vascular plants, and necromass found in a 1 m × 10 cm strip on each plot (n = 10) and below ground biomass collected from 2 soil cores of 15 cm × 5 cm extracted from each plot (n = 20)]. All box plots show the median, 25/75th percentiles within the box, 10/90th percentiles shown within the whiskers and the outlier. Asterisk denote statistically significant differences between OTC and control plots at P < 0.05.
Vegetation Structure Changes (Carbon Sequestration Potential)
After 3 years of warming, we observed some natural changes in the plant community, some related to warming but most of them occurring regardless of the warming treatment, and interestingly those changes were páramo dependent (Figure 7). We only observed changes related to warming on the dominance of shrubs, graminoids, and lichens and they were completely different in the two páramos (Figure 7). In Matarredonda only lichens have increased as result of warming (X2 = 5.14; df = 1; P = 0.02), increasing their cover by 2% in the OTC while in the control plots only by 0.36%. In Sumapaz, on the other hand, shrubs have increased in dominance in both the OTC (23%) and the control plots (19%) (F = 3.9; df = 1; P = 0.08); while warming is reducing graminoids losses, although they have decreased in both the OTC (0.9%) and the control plots (6%) the reduction was smaller in the OTC (X2 = 5.14; df = 1; P = 0.02). For other groups, we did not find evidence that warming was changing their cover; forbs, rosettes, cushion plants, ferns, and club mosses (seedless vascular) and mosses and liverwort (non-vascular plants) have changed in similar ways in all treatments. In Sumapaz, we also observed that the bare soil cover has decreased and the standing necromass is increasing in similar ways in both treatments (Treatment effect: F = 0.09; df = 1; P = 0.76), while in Matarredonda we registered the opposite pattern, a reduction in necromass and increase of bare soil cover in all plots, regardless of treatment (F = 1.21; df = 1; P = 0.29).
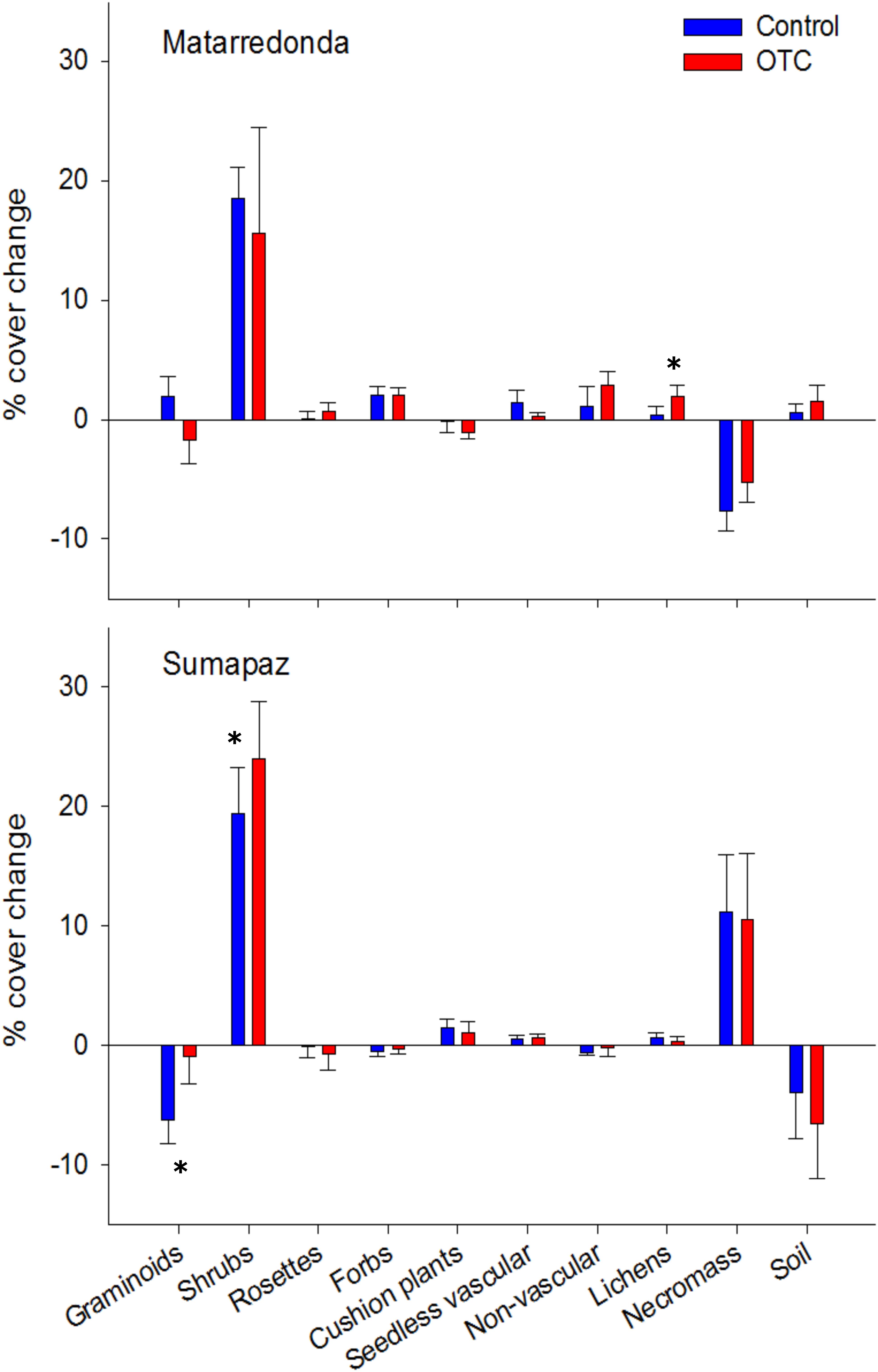
Figure 7. Changes in vegetation cover of the main growth forms in the páramo and of the bare soil and necromass cover after 3 years of warming. We present the percentage cover change that has occurred during 3 years of warming. Positive values denote an increase in cover since the start of the experiment and negative values a reduction in cover. Errors bars represent the standard error. Asterisk denote statistically significant differences between OTC and control plots at P < 0.05.
Effect Size
Values of effect size indicate no experimental effect of warming on most measured variables (Figure 8). The largest effect size was observed for the decay rate of Laurus nobilis in Sumapaz and Matarredonda, −1.96 and −1.27, respectively, and for the decay rate of Espeletia grandiflora in Sumapaz, −0.83. In this case, warming was significantly slowing down litter decomposition rate (Figure 8). Another large effect size observed was for the soil total N and C in Sumapaz (d = 0.80 and d = 0.76, respectively) where warming was significantly increasing the availability of C and N. Other large and medium effect size but whose confidence intervals were overlapping with 0 that may indicate a developing trend, are a change in graminoids cover observed in Sumapaz (d = 0.81) indicating that warming was slightly increasing graminoids cover, while in Matarredonda warming has the opposite effect (d = −0.64) of decreasing graminoids cover. Medium effect sizes were observed for the change in lichen cover (d = 0.59) and root productivity (d = 0.54) in Matarredonda only.
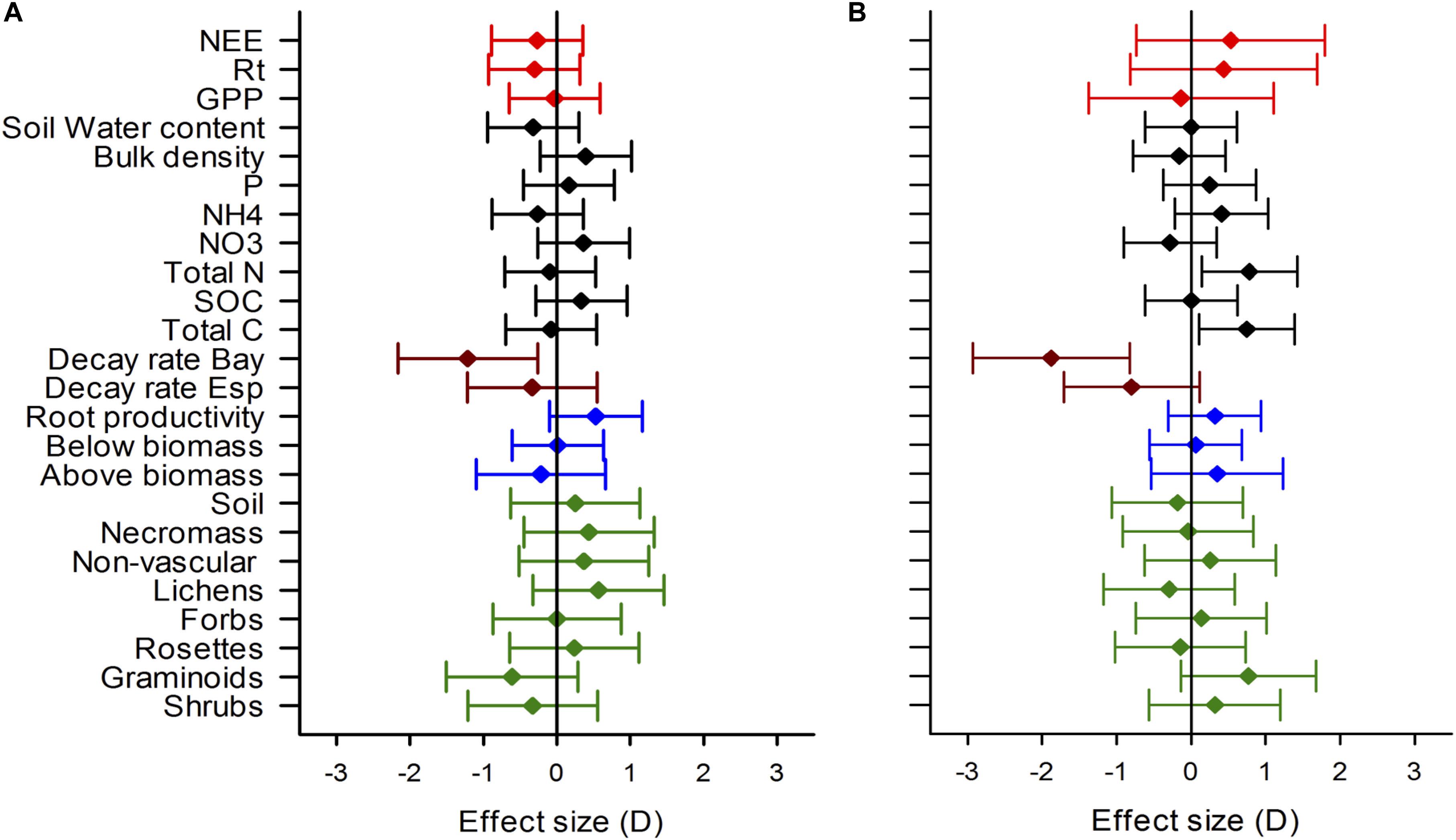
Figure 8. Effects size of experimental warming on CO2 fluxes (red symbols), soil related properties (black symbols), decomposition rate (brown symbols), productivity and biomass (blue symbols), and on vegetation cover (green symbols) in Matarredonda (A) and Sumapaz (B). Diamond symbols show the warming effect size and the error lines the 95% CI. When 95% CI do not overlap with zero the warming treatment has a significant effect, positive values indicate a positive effect of warming and negative values a negative effect of warming. The effect size for most variables are for measurements taken in June 2019 after 3 years of the establishments of the experiment, except for decomposition and root productivity that were taken during the first 2 years of warming. Decay rate “Bay” refers to the estimate for Laurus nobilis and decay rate “Esp” for the estimates for Espeletia grandiflora.
Discussion
Results from 3 years of in situ experimental warming using open-top chambers (OTC) at two páramo sites in Colombia shows that warming has not so far significantly increased soil respiration, litter decomposition, aboveground and belowground biomass, leaf-level photosynthesis, net ecosystem exchange or gross primary productivity, indicating that the páramo has some resilience to warming at least in the short-term. The few changes observed in response to warming were páramo dependent. In Sumapaz, warming increased shrub, and graminoid cover, necromass, and total C and N in the soil while barren soil cover was reduced in the warmed plots. In Matarredonda, warming has resulted in an increase in lichen cover, a slightly higher fine-root productivity, and a small increase in soil bulk density with no other changes. With this result we conclude that the tropical high-elevation ecosystems of the Andes show an homeostatic response to warming at least in the short term and are less sensitive to warming as expected initially given their low temperatures (Lloyd and Taylor, 1994; Carey et al., 2016). However, changes observed in the vegetation suggest that changes could be slow, and that warming could affect those ecosystem processes indirectly and in the long run through changes in the vegetation and soil community. Site-specific differences underscore that the sensitivity of carbon-related processes to global drivers like warming depend on the background climate and ecosystem conditions. Earth system models should be evaluated on those underrepresented regions for an adequate forecasting of future terrestrial carbon-climate feedback (Song et al., 2019).
Application of OTC to Simulate Future Warming Scenarios in the Páramo
Since global climate change was recognized as a major threat, scientists have tried to determine how much carbon can different ecosystems soak up from the atmosphere and how much of the carbon that already resides in the vegetation and soil could be lost as temperatures increases. One realistic way to address these questions is through in situ warming experiments that include the vegetation, the soil community and their interactions. Here we show that hexagonal OTCs constructed following ITEX protocol are a suitable method to mimic future warming scenarios and to explore questions related to páramos’ functioning in a future warmer world, especially giving the inaccessibility of some of these high-elevation sites with no access to power. Most of the experience using OTC come from temperate ecosystems, including tundra, and temperate alpine systems (Arft et al., 1999; Rustad et al., 2001; Aerts, 2006; Lu et al., 2013). Our knowledge of how warming will affect the cold tropics, where the climatic conditions are dramatically different without marked seasons and where vegetation can grow all year round, is scarce.
A series of warming experiments in the high alpine ecosystems of the tropics in Colombia, Ecuador, and Costa Rica are running under the IPPEX network (International Network of Páramo and Puna experimental warming sites) with the goal of integrating and fostering research in ecosystem level effects of rising temperatures in the páramo; and in this special issue we present the first reports of two of these in situ warming experiments. We show that OTC is also an efficient warming method in the páramo capable of increasing mean air temperature by 1.7°C and soil temperature by 0.1°C, rising the air temperatures within the range predicted in the future warming scenarios (IPCC, 2014). This environmental data comes from 2-years monitoring but from only one site/block, limiting our ability to assess spatial variation in the ability of OTCs to increase temperature. However, this increase by 1.7°C, is within the general range (1.2–3.0°C) reported in many tundra and high-latitudes mountain warming experiments using OTCs (Marion, 1997; Hollister and Webber, 2000; Alatalo et al., 2017) which suggest that it is unlikely that all OTCs are not functioning within those ranges. Moreover, leaf temperature data that comes from all plots and sites consistently shows that OTC are increasing leaf temperature by at least 3°C. On the other hand, changes in soil temperature were smaller but consistent, in line with what is expected with air warming faster than the soil, and with vegetation and soils buffering abrupt temperature variations it is expected that changes in belowground processes will lag changes in aboveground processes (Pregitzer et al., 2000; Radville et al., 2016). All temperature enhancing systems, active or passive, have potential unexpected ecological effects such as: altering temperature extremes, light, moisture, gas concentration, wind, and animal visitants, so they invariably will alter other factors besides just temperature. However, they have provided insightful information on how warming might alter the vegetation and other ecosystem processes and have been used for more than 20 years. Here, by using OTC with the hexagonal more open design, we reduced some of those unwanted effects, for example the large opening in the top allows for direct solar radiation to the plants, allow for some wind flow and excess heat to dissipate through the top by free convection and reduces any significant difference in CO2 concentrations between controls and OTC chambers (Marion, 1997). Minimum air temperatures are sometimes lower in the OTC than in the control plots, but differences are small, and these events are infrequent. The large opening in the roof and the fact that the base is not buried in the ground allows air flow also through the base and the free entry of both flying and crawling insects. We have seen larvae, caterpillars, bees, and bumblebees inside the OTC, which tells us that the walls are not blocking the arrival of animals. Outside of these limitations, we believe that OTC can give us valuable information about how the páramo will respond to rising temperatures in places where it is difficult to get power to use other active heating methods.
Physical, Chemical and Biological Properties of the Soil
Páramos are carbon sinks, storing larger carbon quantities per hectare than tropical forests in their soil organic layer, which can extend from 1 m depth to 9.4 m in some peatlands (Hofstede et al., 2014; Hribljan et al., 2017). How much soil organic carbon (SOC) is stored depends on the climatic conditions and the land use history of the páramo (Gutiérrez et al., 2020) and in Colombia, SOC values reported range between 22 and 338 tons per hectare in the first 30 cm of the soil profile (Gutiérrez et al., 2020). Estimates from Sumapaz indicates values of SOC of 188.2 ± 41.2 ton/ha in the first 25 cm of the soil profile (Montes-Pulido et al., 2017) and values between 119 and 397 tons per hectare (t/ha) in the first 40 cm has been reported in other páramos (Castañeda-Martín and Montes-Pulido, 2017). Given the different sampling depth it is challenging to compare where our data stands in compared to other páramos but our values between 102.1 and 149.4 ton/ha from the first 20 cm of the soil, are within ranges reported for others carbon-rich Colombian and Ecuadorian páramos. Here we do not see evidence of a warming-induced reduction on SOC nor changes in most soil-related processes and properties such as water content, pH, NH4 and NO3 and soil respiration. However, in Sumapaz we see an increase in total carbon and total nitrogen in the OTC that suggest an increase in N mineralization rate is taking place in response to warming as reported in other cold biomes (Rustad et al., 2001). Although we have not measured N mineralization rate per se, we do have instantaneous measurements of ammonium and nitrate and total N that suggest that mineralization may have increased as result of warming. If warming increases the mineralization rate, it could potentially increase plant productivity. A similar response was observed in a warming experiment in Alaska, where a 3-year lag between the initiation of treatment and ecosystem response was observed, probably related to changes in mineralization and an increase in nutrient availability (Chapin et al., 1995). If the trend holds and N increases trough time, any possible future negative effect of warming in decomposition and soil respiration could be offset if warming increases productivity, which in the unseasonal tropics with a continuous growing season, could be quite high. Moreover, the differential response among páramo sites indicate that páramo response to warming would depend on the site-specific conditions of the páramo; C and N pools and turnover rate, soil water content and precipitation regime, the disturbance history, the chemical composition of plants, the longevity, and population turnover rates of dominant species (Shaver et al., 2000).
Effect of Warming in Processes Related to CO2 Emissions
Decomposition Rate
Plant litter decomposition is a key component of the global carbon budget (Couteaux et al., 1995; Aerts, 1997; Robinson, 2002) contributing approximately 70% to the total annual carbon flux (Raich and Schlesinger, 1992) and acting as critical feedback to climate change by simultaneously influencing CO2 flux from the soil and productivity. Thus, any changes in factors that control litter decomposition rates may have significant repercussions for the global carbon budget. Given the sensitivity of decomposition to temperature, especially in cold biomes, it has been hypothesized that global warming will lead to increased litter decomposition rates, both through direct temperature effects and through indirect effects on litter quality and soil organisms. Here we did not find evidence that warming will increase litter decomposition and the loss of carbon from this process in the páramo. Evidence from different warming experiments in other cold biomes is still inconclusive. The analysis by Aerts (2006) of 34 warming experiments showed that warming resulted in slightly increased decomposition rates only when heating lamps were used, but in OTC decomposition rates was consistently reduced as we show here. OTC walls might be reducing UV light and therefore reduce decomposition. Alternatively, OTC may lead to stronger drought than heating lamps, and decomposition is moisture-limited, as suggested by Aerts (2006). This last possibility is further supported by the meta-analysis by Rustad et al. (2001), which shows that soil moisture was significantly lower in the heated plots than in the control plot across 14 experimental warming sites. If this is the case, global warming will increase litter decomposition rates only if there is sufficient soil moisture. For the Northern part of the high tropical Andes, temperature and precipitation are expected to change in the future (Urrutia and Vuille, 2009; Buytaert et al., 2011), and we could expect an increase in decomposition with the concomitant higher contribution to the total annual carbon fluxes (Salinas et al., 2011; Gutiérrez-Salazar and Medrano-Vizcaíno, 2019) in those future scenarios. However, in the current conditions, páramos’ decomposition rate values are extremely low compared to what has been reported for other tropical ecosystems (Cusack et al., 2009; Powers et al., 2009). The decay rate for Espeletia’s substrate was between 0.30 and 0.39 year–1, and for the bay leaf was between 0.35 and 0.46 year–1. With this low decomposition rate, 1 g of litter has lost <50% of its mass after 2 years in the field, which strongly contrasts with the values reported for other tropical forests where values of decay rates ranged from 0.47 to 15.10 year–1 resulting in 95% of mass lost in just 1 year (Powers et al., 2009). The low decomposition rate in the páramo is not only related to the cold temperature of the páramo but also to the conditions of the soil, the clay-loamy soil texture, the high acidity that also induces a high content of aluminum, all factors that inhibit the degradation of the organic matter by the soil community (Gonzalez-Prieto et al., 1996; Bottner et al., 2006; Hofstede et al., 2014) so the effect of warming could be more intricate and deserves future evaluation.
Soil Respiration
Soil respiration is one of the main sources of CO2 loss to the atmosphere, representing 40–90% of the whole CO2 production in forest ecosystems (Schlesinger and Andrews, 2000), and warming is hypothesized to increase rates of soil respiration, potentially stimulating further intensification in global temperatures. Although warming has been shown to stimulate soil respiration in the tundra and other northern cold ecosystems (Melillo et al., 2002; McGuire et al., 2009; Conant et al., 2011; Chen et al., 2015) and in a tropical forest (Nottingham et al., 2020) we have not found evidence that the same is happening in the páramo. Our result contrasts with what should be expected in colder climates, which are supposed to be more responsive to increased ambient temperature than warmer climates (Lloyd and Taylor, 1994; Carey et al., 2016), but in agreement with the hypothesis that predicts that warming should cause only modest carbon loss from tropical soils relative to those at higher latitudes (Davidson and Janssens, 2006; Carey et al., 2016). This is not the first report of a neutral response to warming. Some studies have shown even negative responses to warming, often attributed to moisture limitation (Suseela et al., 2012), to the depletion of labile C pools (Melillo et al., 2002; Kirschbaum, 2004; Hartley et al., 2007) or shifts in the microbial composition and their physiological response (Luo et al., 2001; Crowther and Bradford, 2013).
While soil respiration in incubated soil from the páramo suggested a high sensitivity of CO2 emissions to changes in soil temperature (Curiel Yuste et al., 2017), our findings from this in situ long-term warming experiment indicates that processes are more complicated once the local vegetation and soil community become part of the story. It is clear that the vegetation is buffering changes in soil temperature, and the soil system per se is also buffering against air temperature fluctuations. Consequently, the soil system seems not to react to the 0.1°C net increase in temperature brought on by the OTC. The soil metabolism might have undergone a process of acclimation to the small increase in temperature (Allison et al., 2010; Wei et al., 2014) under OTC’s that reflect a homeostatic behavior of the system. Another possibility is that this apparent acclimation reflects depletion of the readily decomposable substrate under warming (Kirschbaum, 2004), but our soil organic carbon data suggest otherwise. We have no evidence that the microbial community has changed during the first year of the experiment, where the bacterial, archaeal, and fungal communities measured through amplicon sequencing of the 16S rRNA and ITS1 rRNA gene regions remains similar in the OTC and control plots (Gallery and Lasso, 2017). However, given that páramo plants have shown surprisingly high heat tolerance (Leon-Garcia and Lasso, 2019), microbes may also be adapted to deal with the dramatic daily variation in temperature typical of the páramo, where temperatures can range from −2 to 30°C in one single day. Consequently, we do see a response to seasonal temperature fluctuation, but we do not see a strong response to the OTC’s warming. Research on the soil microbial communities’ thermal adaptation is still needed for the tropics, especially for the cold tropics (Bradford et al., 2008; Curiel Yuste et al., 2010; Wood et al., 2019).
Effect of Warming in Processes Related to CO2 Capture and Net Ecosystem Exchange
The net effects of future warming on carbon fluxes in the páramo would depend on whether warming could boost up photosynthesis and primary productivity and offset any warming-induced CO2 release thorough soil autotrophic and heterotrophic respiration. We did not found evidence that the higher temperatures are inhibiting species or that warming is modifying their photosynthetic machinery, all species had similar leaf level maximum photosynthetic rate and respiration in the OTC and the control plots. Consistent with this observation, we also saw that warming is not strongly affecting the production of below- and above-ground biomass, and we only see a trend in warming-induced fine-root production, which is a key component of carbon sequestration in soil (Matamala et al., 2003), accounting for 50–90% of primary production (Ruess et al., 2003; Steinaker and Wilson, 2008). So, warming could increase carbon sequestration through root production. Likewise, total uptake of carbon at the community level through photosynthesis (GPP) and total carbon dioxide release from autotrophic and heterotrophic respiration were indistinguishable between OTC and control plots in Matarredonda, while in Sumapaz we found that less carbon is being released from autotrophic and heterotrophic respiration in the OTC in comparison to the control plots. In both sites the net ecosystem exchange (NEE) or final carbon dioxide effluxes from the ecosystem were not different between the OTC and control plots. Our values of NEE range between 1.14 and 0.87 g CO2 m2/h and are slightly larger than the values reported for an undisturbed páramo in Coca National Park in Northern Ecuador with NEE = 0.69 g CO2 m2/h, but slightly smaller than values found in a grazed páramo in Antisana Ecuador with NEE = 1.25 g CO2 m2/h (Sánchez et al., 2017). Based on these data, which does not include night time respiration, gross primary productivity still exceeded respiration by around 17–30% in our sites and by 43–51% in Ecuador (Sánchez et al., 2017) and suggest that those páramos are still working as carbon sinks, absorbing more carbon than liberating it. However, the only study using eddy covariance technique following diurnal and nocturnal exchanges in the páramos, found that the páramo at Zhurucay in Northern Ecuador behaved as a source of CO2 most of the time and only 34% of the time was acting as a weak carbon sink; respiration was exceeding gross primary productivity by 6–11% (Carrillo-Rojas et al., 2018). Until more eddy covariance towers have been installed in other páramos we would not know to what extent páramos have lost their potential for carbon sequestration or if these results are specific to that Ecuadorian páramo.
Vegetation Structure Changes (Carbon Sequestration Potential)
Vegetation responses to warming were completely different between the two páramo sites, in Sumapaz, shrubs, and graminoids increased in response to warming while, in Matarredonda, we observed an increase in lichen cover and a slight increase (not significant) in moss cover. While we need to wait to see the long-term effect of warming in the páramo, these first 3 years of experimental warming indicate that warming has the potential to change the structure and function of the plant communities by increasing the dominance of shrubs, graminoids, and lichens. These changes could have several implications. For one, an increase in the dominance of woody shrubs has the potential to alter the C-sequestration of the páramo vegetation over the long-term if páramo’s woody shrubs have greater C-sequestration capacity than other growth forms as found in the tundra (Shaver et al., 1998), and these changes could have in turn a positive feedback on the climate (Shaver and Jonasson, 1999; Shaver et al., 2000; Walker et al., 2006). This response is congruent with observations for the tundra where warming increased shrub cover (Sturm et al., 2001; Walker et al., 2006; Molau, 2010). The increase in woody shrubs in one of the páramo sites, together with the accumulation of standing dead necromass in the OTC, a response also registered in some warming experiment in the tundra (Hollister et al., 2005), implies a larger residence time for carbon in the vegetation. In Matarredonda, however, lichens have increased because of warming, if this trend is confirmed, it may imply a reduction in the complexity of the vegetation and on the carbon storage potential. The increase in lichens contrasts with the results of warming experiments in the tundra where warming resulted in a decreased cover of mosses and lichens (Graglia et al., 2001; Hollister et al., 2005; Jónsdóttir et al., 2005; Walker et al., 2006). Lichen and mosses in the páramo may not be particularly sensitive to warming and N2 fixation may be maintained even in a warmer climate, where both groups might account for up to 50% of total N inputs as shown in the tundra cold biomes (Rousk and Michelsen, 2017). The greater stability of the vegetation observed in Matarredonda in response to warming could be connected to the larger presence of mosses and Sphagnum in this site, a group that buffered and stabilized the vegetation response to warming also in the Arctic tundra (Hollister et al., 2005; Klanderud, 2008; Keuper et al., 2011). Longer warming times, extended monitoring and more warming sites would be needed for a good prediction of long-term changes in the páramo vegetation in response to warming as it has been shown with a 20 years-long vegetation monitoring in a warming experiment in the tundra (Elmendorf et al., 2012). To fully understand how the vegetation changes connects with the carbon sequestration potential of the páramo we need more data on the carbon content and carbon sequestration potential of different growth forms in the páramo.
Conclusion
Understanding the sensitivity of páramo vegetation to climate warming is critical to forecasting future biodiversity and vegetation feedbacks to climate. Our results suggest that the carbon sequestration potential of the páramo will not be reduced by warming in the short term since none of the processes that could increase carbon release, neither soil or plant respiration nor the rate of decomposition are increasing in the OTC. However, such results must be interpreted cautiously because the large differences among sites suggest that not all páramos are going to respond in the same way. Moreover, there could be a tipping point after which the changes become irreversible and we don’t know what this tipping point is going to be for the páramos. Here we are manipulating a single factor, temperature, and we are not accounting for all the complex interactions between environmental factors that limit growth and decomposition in the páramo, which are likely also constrained by water and/or nutrients. Long-term studies in different páramos will be crucial for resolving how all these factors interact with warming and will affect the vegetation and ecosystem services.
Data Availability Statement
The raw data supporting the conclusions of this article will be made available by the authors, without undue reservation.
Author Contributions
EL, JC, and RG conceived the ideas and designed methodology. EL, RG, JC, PM-A, CG-L, MC, IL-G, AA-P, and LA collected the data. EL and JC analyzed the data. EL led the writing of the manuscript. All authors contributed critically to the drafts and gave final approval for publication.
Funding
Funding for this research comes from the “Patrimonio Autónomo Fondo Nacional de Financiamiento para la Ciencia, la Tecnología y la Innovación Francisco José de Caldas–Colciencias,” grant number 120471451294, granted by Colciencias (today’s Colombian Ministry of Science, Technology and Innovation), from Eloisa Lasso’s FAPA (Fondo de Apoyo para Profesores Asistentes) grant number P12.160422.001 from the Universidad de los Andes, and from the Research Fund to support faculty programs at the Faculty of Sciences at the Universidad de los Andes grant number INV-2019-84-1805.
Conflict of Interest
The authors declare that the research was conducted in the absence of any commercial or financial relationships that could be construed as a potential conflict of interest.
Acknowledgments
Special thanks to David Campos, Fabian Salgado, Luis Fernando Rojas, Ewen Dano, Jorge Acosta, Juliana Portilla, and Michelle Guevara that helped with field and lab work. We would like to thank the Sabogal family for allowing the establishment of the OTC and collection of samples in the páramo “Parque Ecológico Matarredonda” and to the Lieutenant Colonel Edgar Riveira, Commander of the High Mountain Battalion No. 1 in Sumapaz and all the commanders that follow him and who provided us with accommodation and food in the battalion as well as logistic support in all our field campaigns. Thanks to all the soldiers who always enthusiastically helped us in the field work in Sumapaz. This research was also supported by the Basque Government through the BERC 2018–2021 program, and by the Spanish Ministry of Science, Innovation and Universities through the BC3 María de Maeztu excellence accreditation (MDM-2017-0714).
Supplementary Material
The Supplementary Material for this article can be found online at: https://www.frontiersin.org/articles/10.3389/fevo.2021.615006/full#supplementary-material
References
Aerts, R. (1997). Climate, leaf litter chemistry and leaf litter decomposition in terrestrial ecosystems: a triangular relationship. Oikos 79, 439–449. doi: 10.2307/3546886
Aerts, R. (2006). The freezer defrosting: global warming and litter decomposition rates in cold biomes. J. Ecol. 94, 713–724. doi: 10.1111/j.1365-2745.2006.01142.x
Alatalo, J.M., Jägerbrand, A.K., Chen, S., and Molau, U. (2017). Responses of lichen communities to 18 years of natural and experimental warming. Ann. Bot. 120, 159–170. doi: 10.1093/aob/mcx053
Allison, S., Wallenstein, M. and Bradford, M. (2010). Soil-carbon response to warming dependent on microbial physiology. Nature Geosci. 3, 336–340. doi: 10.1038/ngeo846
Anthelme, F., Villaret, J.C., and Brun, J.J. (2007). Shrub encroachment in the alps gives rise to the convergence of sub-alpine communities on a regional scale. J. Veg. Sci. 18, 355–362. doi: 10.1111/j.1654-1103.2007.tb02547.x
Arft, A.M., Walker, M.D., Gurevitch, J., Alatalo, J.M., Bret-Harte, M.S., Dale, M. et al. (1999). Response patterns of tundra plant species to experimental warming: a meta-analysis of the international tundra experiment. Ecol. Monogr. 69, 491–511.
Báez, S., Jaramillo, L., Cuesta, F., and Donoso, D.A. (2016). Effects of climate change on andean biodiversity: a synthesis of studies published until 2015. Neotrop. Biodivers. 2, 181–194. doi: 10.1080/23766808.2016.1248710
Bassirirad, H. (2000). Kinetics of nutrient uptake by roots: responses to global change. New Phytol. 147, 155–169. doi: 10.1046/j.1469-8137.2000.00682.x
Bond-Lamberty, B., and Thomson, A. (2010). Temperature-associated increases in the global soil respiration record. Nature 464, 579–582. doi: 10.1038/nature08930
Bottner, P., Pansu, M., Sarmiento, L., Hervé, D., Callisaya–Bautista, R., Metselaar, K. et al. (2006). Factors controlling decomposition of soil organic matter in fallow systems of the high tropical andes: a field simulation approach using 14C–and 15N–labelled plant material. Soil Biol. Biochem. 38, 2162–2177. doi: 10.1016/j.soilbio.2006.01.029
Bradford, M.A., Davies, C.A., Frey, S.D., Maddox, T.R., Melillo, J.M., and Mohan, J.E., et al., (2008). Thermal adaptation of soil microbial respiration to elevated temperature. Ecol. Lett. 11, 1316–1327. doi: 10.1111/j.1461-0248.2008.01251.x
Bradley, R.S., Keimig, F. and Diaz, H. (2004). Projected temperature changes along the American cordillera and the planned GCOS network, geophys. Res. Lett. 31:L16210. doi: 10.1029/2004GL020229
Bradley, R.S., Vuille, M., Diaz, H. and Vergara, W. (2006). Threats to water supplies in the tropical andes. Science. 312, 1755–1756. doi: 10.1126/science.1128087
Brandt, J. S., Haynes, M. A., Kuemmerle, T., Waller, D. M., and Radeloff, V. C. (2013). Regime shift on the roof of the world: alpine meadows converting to shrublands in the southern Himalayas. Biol. Conserv. 158, 116–127. doi: 10.1016/j.biocon.2012.07.026
Brienen, R.J.W., Caldwell, L., Duchesne, L., Voelker, S., Barichivich, J., Baliva, M., et al., (2020). Forest carbon sink neutralized by pervasive growth-lifespan trade-offs. Nat. Commun. 11:4241. doi: 10.1038/s41467-020-17966-z
Buytaert, W., Cuesta, F., and Tobon, C. (2011). Potential impacts of climate change on the environmental services of humid tropical alpine regions. Glob. Ecol. Biogeogr. 20, 19–33. doi: 10.1111/j.1466-8238.2010.00585.x
Carey, J.C., Tang, J., Templer, P.H., Kroeger, K.D., Crowther, T.W., Burton, A.J., et al. (2016). Limited acclimation of soil respiration to warming. Proc. Natl. Acad. Sci. 113, 13797–13802. doi: 10.1073/pnas.1605365113
Carrillo-Rojas, G., Silva, B., Rütger, R., Célleri, R. and Jörg, B. (2018). The breathing of the andean highlands: net ecosystem exchange and evapotranspiration over the páramo of southern ecuador. Agric. For. Meteorol. 265, 30–47. doi: 10.1016/j.agrformet.2018.11.006
Castañeda-Martín, A.E. and Montes-Pulido, C.R. (2017). Carbono almacenado en páramo andino. Entramado 13, 210–221. doi: 10.18041/entramado.2017v13n1.25112
Cavaleri, M.A., Reed, S.C., and Smith, W.K. and Wood, T.E. (2015). Urgent need for warming experiments in tropical forests. Glob. Change Biol. 21, 2111–2121. doi: 10.1111/gcb.12860
Chapin, F.S., III, Shaver, G.R., Giblin, A.E., Nadelhoffer, K.J. Laundre, J.A. (1995). Responses of arctic tundra to experimental and observed changes in climate. Ecology. 76, 694–711. doi: 10.2307/1939337
Chen, J., Luo, Y., Xia, J., Jiang, L., Zhou, X., Lu, M., and Cao, J. (2015). Stronger warming effects on microbial abundances in colder regions. Sci. Rep. 5:18032. doi: 10.1038/srep18032
Conant, R.T., Ryan, M.G., Ågren, G.I., Birge, H.E., Davidson, E.A., Eliasson, P.E., et al., (2011). Temperature and soil organic matter decomposition rates – synthesis of current knowledge and a way forward. Glob. Change Biol. 17, 3392–3404. doi: 10.1111/j.1365-2486.2011.02496.x
Cornelissen, J.H.C., Callaghan, T.V., Alatalo, J.M., Michelsen, A., Graglia, E., Hartley, A.E. et al., (2001). Global change and arctic ecosystems: is lichen decline a function of increases in vascular plant biomass? J. Ecol. 89, 984–994. doi: 10.1111/j.1365-2745.2001.00625.x
Couteaux, M.M., Bottner, P. Berg, B. (1995). Litter decomposition, climate and litter quality. Trends Ecol. Evol. 10, 63–66. doi: 10.1016/S0169-5347(00)88978-8
Craine, J., Fierer, N., and McLauchlan, K. (2010). Widespread coupling between the rate and temperature sensitivity of organic matter decay. Nature Geosci. 3, 854–857. doi: 10.1038/ngeo1009
Cregger, M.A., Sanders, N.J., Dunn, R.R., and Classen, A.T. (2014). Microbial communities respond to experimental warming, but site matters. PeerJ. 2:e358. doi: 10.7717/peerj.358
Crowther, T.W. and Bradford, M.A. (2013). Thermal acclimation in widespread heterotrophic soil microbes. Ecol. Lett. 16, 469–477. doi: 10.1111/ele.12069
Cuesta, F., Llambí, L.D., Huggel, C., Drenkhan, F., Gosling, W.D., Muriel, P., et al., (2019). New land in the neotropics: a review of biotic community, ecosystem, and landscape transformations in the face of climate and glacier change. Reg. Environ. Change 19, 1623–1642. doi: 10.1007/s10113-019-01499-3
Cuesta, F., Muriel, P., Llambí, L.D., Halloy, S., Aguirre, N., Beck, S., et al., (2017). Latitudinal and altitudinal patterns of plant community diversity on mountain summits across the tropical andes. Ecography 40, 1381–1394. doi: 10.1111/ecog.02567
Curiel Yuste, J., Hereş, A.M., Ojeda, G., Paz, A., Pizano, C., García-Angulo, D., et al. (2017). Soil heterotrophic CO2 emissions from tropical high-elevation ecosystems (páramos) and their sensitivity to temperature and moisture fluctuations. Soil Biol. Biochem. 110, 8–11. doi: 10.1016/j.soilbio.2017.02.016
Curiel Yuste, J., Ma, S., Baldocchi, D.D. (2010). Plant-soil interactions and acclimation to temperature of microbial-mediated soil respiration may affect predictions of soil CO2 efflux. Biogeochemistry 98, 127–138 doi: 10.1007/s10533-009-9381-1
Cusack, D.F., Chou, W.W., Yang, W.H., Harmon, M.E. and Silver, W.L. (2009). Controls on long-term root and leaf litter decomposition in neotropical forests. Glob. Change Biol. 15, 1339–1355. doi: 10.1111/j.1365-2486.2008.01781.x
Dalias, P., Anderson, J.M., Bottner, P. and Coûteauxa, M.M. (2002). Temperature responses of net nitrogen mineralization and nitrification in conifer forest soils incubated under standard laboratory conditions. Soil Biol. Biochem. 34, 691–701. doi: 10.1016/S0038-0717(01)00234-6
Davidson, E.A. and Janssens, I.A. (2006). Temperature sensitivity of soil carbon decomposition and feedbacks to climate change. Nature 440, 165–173. doi: 10.1038/nature04514
Duque, A., Stevenson, P.R., Feeley, K.J. (2015). Thermophilization of andean forests. Proc. Natl. Acad. Sci. 112, 10744–10749. doi: 10.1073/pnas.1506570112
Elmendorf, S.C., Henry, G.H.R., Hollister, R.D., Björk, R.G., Bjorkman, A.D., Callaghan, T.V., et al., (2012). Global assessment of experimental climate warming on tundra vegetation: heterogeneity over space and time. Ecol. Lett. 15, 164–175. doi: 10.1111/j.1461-0248.2011.01716.x
Emmett, B., Beier, C., Estiarte, M., Tietema, A., Kristensen, H.L., Williams, D., et al. (2004). The response of soil processes to climate change: results from manipulation studies of shrublands across an environmental gradient. Ecosystems 7, 625–637. doi: 10.1007/s10021-004-0220-x
Farr, T.G., Rosen, P.A., Caro, E., Crippen, R., Duren, R., Hensleym S. et al. (2007), The shuttle radar topography mission. Rev. Geophys. 45:RG2004. doi: 10.1029/2005RG000183
Feeley, K. (2015). Are we filling the data void? an assessment of the amount and extent of plant collection records and census data available for tropical south america. PLoS One 10:e0125629. doi: 10.1371/journal.pone.0125629
Feeley, K.J., Silman, M.R., Bush, M.B., Farfan, W., Cabrera, K.G., Malhi, Y., et al., (2011). Upslope migration of andean trees. J. Biogeogr. 38, 783–791. doi: 10.1111/j.1365-2699.2010.0244.x
Ficklin, D.L., and Novick, K.A. (2017), Historic and projected changes in vapor pressure deficit suggest a continental-scale drying of the united states atmosphere. J. Geophys. Res. Atmos. 122, 2061–2079. doi: 10.1002/2016JD025855
Gallery, R. and Lasso, E. (2017). Soil microbial response to climate warming in the Northern Andean alpine ecosystems. In proceedings AGU Fall Meeting B53I-04. Available online at: https://ui.adsabs.harvard.edu/abs/2017AGUFM.B53I..04G (accessed October 6, 2020).
Gill, R.A., and Jackson, R.B. (2000). Global patterns of root turnover for terrestrial ecosystems. New Phytol. 147, 13–31. doi: 10.1046/j.1469-8137.2000.00681.x
Gonzalez-Prieto, S.J., Cabaneiro, A., Villar, M.C., Carballas, M. and Carballas, T. (1996). Effect of soil characteristics on N mineralization capacity in 112 native and agricultural soils. Biol. Fertil. Soils. 22, 252–260. doi: 10.1007/BF00382521
Graglia, E., Jonasson, S., Michelsen, A., Schmidt, I.K., Havström, M. and Gustavsson, L. (2001). Effects of environmental perturbations on abundance of subarctic plants after three, seven and ten years of treatments. Ecography. 24, 5–12. doi: 10.1034/j.1600-0587.2001.240102.x
Gutiérrez, J., Ordoñez, N., Bolívar, A., Bunning, S., Guevara, M., Medina, E., et al., (2020). Estimación del carbono orgánico en los suelos de ecosistema de páramo en Colombia. Ecosistemas 29:1855. doi: 10.7818/ECOS.1855
Gutiérrez-Salazar, P. and Medrano-Vizcaíno, P. (2019). The effects of climate change on decomposition processes in andean páramo ecosystem-synthesis, a systematic review. Appl. Ecol. Environ. Res. 17, 4957–4970. doi: 10.15666/aeer/1702_49574970
Hartley, I.P., Heinmeyer, A., Ineson, P. (2007). Effects of three years of soil warming and shading on the rate of soil respiration: Substrate availability and not thermal acclimation mediates observed response. Glob. Change Biol. 13, 1761–1770. doi: 10.1111/j.1365-2486.2007.01373.x
Hobbie, S.E. (1996). Temperature and plants species controls over litter decomposition in alaskan tundra. Ecol. Monogr. 66, 503–522. doi: 10.2307/2963492
Hofstede, R. (1995). Effects of livestock farming and recommendations for management and conservation of páramo grasslands (Colombia). Land Degrad. Dev. 6, 133–147. doi: 10.1002/ldr.3400060302
Hofstede, R. (1999). “El páramo como espacio para la fijación de carbono atmosférico” in El Páramo Como Espacio De Mitigación De Carbono Atmosférico. eds G. Medina, and P. Mena (Ecuador, Quito: Ediciones Abya-Yala).3–6.
Hofstede, R., Calles, J., López, V.V., Polanco, R., Torres, F., Ulloa, J., et al. (2014). Los Páramos Andinos ¿Qué Sabemos? Estado De Conocimiento Sobre El Impacto Del Cambio Climático En El Ecosistema Páramo. Quito, Ecuador: UICN.
Hollister, R.D., and Webber, P.J. (2000). Biotic validation of small open-top chambers in a tundra ecosystem. Glob. Change Biol. 6, 835–842. doi: 10.1046/j.1365-2486.2000.00363.x
Hollister, R.D., Webber, P.J. and Tweedie, C.E. (2005). The response of alaskan arctic tundra to experimental warming: differences between short- and long-term responses. Glob. Change Biol. 11, 525–536. doi: 10.1111/j.1365-2486.2005.00926.x
Hribljan, J.A., Suarez, E., Bourgeau-Chavez, L., Endres, S., Lilleskov, E.A., Chimbolema, S. et al. (2017). Multi-date, multi- sensor remote sensing reveals high density of carbon-rich mountain peatlands in the páramo of Ecuador. Glob. Change Biol. 23, 5412–5425. doi: 10.1111/gcb.13807
IGAC (2000). Estudio General De Suelos Del Departamento De Cundinamarca. Bogotá, Colombia: Instituto Geográfico Agustín Codazzi.
IPCC (2014). Climate change 2014: synthesis report. Core Writing Team, R.K. Pachauri and L.A. Meyer (eds.). Contribution Of Working Groups I, Ii And Iii To The Fifth Assessment Report Of The Intergovernmental Panel On Climate Change. Geneva, Switzerland: IPCC.
Jónsdóttir, I.S., Magnússon, B., Gudmundsson, J., and Elmarsdóttir, A. and Hjartarson, H. (2005). Variable sensitivity of plant communities in Iceland to experimental warming. Glob. Change Biol. 11, 553–563. doi: 10.1111/j.1365-2486.2005.00928.x
Kappelle, M. (2005). “Hacia una breve descripción del concepto páramo”. eds M. Kappelle, and S.P. Horn. in Páramos De Costa Rica. (Costa Rica: Instituto Nacional de Biodiversidad.). 30.
Kennard, D.K., Matlaga, D., Sharpe, J., King, C., Alonso-Rodriguez, A.M., Reed, S.C., et al., (2020). Tropical understory herbaceous community responds more strongly to hurricane disturbance than to experimental warming. Ecol. Evol. 10, 8906–8915. doi: 10.1002/ece3.6589
Keuper, F., Dorrepaal, E., Van Bodegom, P.M., Aerts, R., Van Logtestijn, R.S.P., Callaghan, T.V. et al., (2011). A race for space? How Sphagnum fuscum stabilizes vegetation composition during long-term climate manipulations. Glob. Change Biol. 17, 2162–2171. doi: 10.1111/j.1365-2486.2010.02377.x
Kimball, B.A., Alonso-Rodríguez, A.M., Cavaleri, M.A., Reed, S.C., González, G. Wood, T.E. et al. (2018). Infrared heater system for warming tropical forest understory plants and soils. Ecol. Evol. 8, 1932–1944. doi: 10.1002/ece3.3780
Kirschbaum, M.U.F. (2004), Soil respiration under prolonged soil warming: are rate reductions caused by acclimation or substrate loss? Glob. Change Biol. 10, 1870–1877. doi: 10.1111/j.1365-2486.2004.00852.x
Klanderud, K. (2008). Species-specific responses of an alpine plant community under simulated environmental change. J. Veg. Sci. 19, 363–372. doi: 10.3170/2008-8-18376
Leon-Garcia, I.V. and Lasso, E. (2019). High heat tolerance in plants from the andean highlands: implications for páramos in a warmer world. PLoS One 14:e0224218. doi: 10.1371/journal.pone.0224218
Li, X., Zhu, J., Lange, H., and Han, S. (2013). A modified ingrowth core method for measuring fine root production, mortality and decomposition in forests. Tree Physiol. 33, 18–25. doi: 10.1093/treephys/tps124
Llambí, L.D., and Cuesta, F. (2014). “La diversidad de los páramos andinos en el espacio y en el tiempo.”, eds F. Cuesta, J. Sevink, L.D. Llambí, B. De Bievre, J. Posner. In: Avances En Investigación Para La Conservación En Los Páramos Andinos. Peru: Quito, Ecuador, Proyecto Páramo Andino-CONDESAN. 7–40.
Lloyd, J., and Taylor, J.A. (1994). On the temperature dependence of soil respiration. Funct. Ecol. 8, 315–323. doi: 10.2307/2389824
Lobo, F., Barros, M. P., Dalmagro, H., Dalmolin, A. C., Pereira, W. E., Souza, C. et al. (2013). Fitting net photosynthetic light-response curves with microsoft excel — a critical look at the models. Photosynthetica 51, 445–456. doi: 10.1007/s11099-013-0045-y
Lu, M., Zhou, X., Yang, Q., Li, H., Luo, Y., Fang, C., et al., (2013). Responses of ecosystem carbon cycle to experimental warming: a meta-analysis. Ecology. 94, 726–738. doi: 10.1890/12-0279.1
Luo, Y., Wan, S., Hui, D. and Wallace, L.L. (2001). Acclimatization of soil respiration to warming in a tall grass prairie. Nature. 413, 622–625. doi: 10.1038/35098065
MacDonald, N.W., Zak, D.R. and Pregitzer, K.S. (1995), Temperature effects on kinetics of microbial respiration and net nitrogen and sulfur mineralization. Soil Sci. Soc. Am. J. 59, 233–240. doi: 10.2136/sssaj1995.03615995005900010036x
Madriñán, S., Cortés, A. J., and Richardson, J. E. (2013). Páramo is the world’s fastest evolving and coolest biodiversity hotspot. Front. Genet. 4:192. doi: 10.3389/fgene.2013.00192
Marion, G.M. (1997). “Temperature enhancement experiments”. eds U. Molau and P. Mølgaard. in Itex Manual Second Edition. (Denmark: International Tundra Experimen, Danish Polar Center). 17-22.
Matamala, R., Gonzàlez-Meler, M.A., Jastrow, J.D., Norby, R.J., and Schlesinger, W.H. (2003). Impacts of fine root turnover on forest NPP and soil C sequestration potential. Science. 302, 1385–1387. doi: 10.1126/science.1089543
McDougall, K. L. (2003). Aerial photographic interpretation of vegetation changes on the Bogong high plains, victoria, between 1936 and 1980. Aus. J. Bot. 51:251. doi: 10.1071/bt02079
McGuire, A.D., Anderson, L.G., Christensen, T.R., Dallimore, S., Guo, L., Hayes, D.J., et al., (2009). Sensitivity of the carbon cycle in the arctic to climate change. Ecol. Monogr. 79, 523–555. doi: 10.1890/08-2025.1
Melillo, J.M., Steudler, P.A., Aber, J.D., Newkirk, K., Lux, H., Bowles, F.P., et al., (2002). Soil warming and carbon-cycle feedbacks to the climate system. Science. 298, 2173–2176. doi: 10.1126/science.1074153
Molau, U. (2010). Long-term impacts of observed and induced climate change on tussock tundra near its southern limit in northern Sweden. Plant Ecol. Divers. 3, 29–34. doi: 10.1080/17550874.2010.487548
Monasterio, M., and Sarmiento, L. (1991). Adaptive radiation of Espeletia in the cold andean tropics. Trends Ecol. Evol. 6, 387–391. doi: 10.1016/0169-5347(91)90159-u
Montes-Pulido, C.R., Ramos-Miras, J.J., and San José-Wery, A.M. (2017). Estimation of soil organic carbon (SOC) at different soil depths and soil use in the Sumapaz páramo, Cundinamarca - Colombia. Acta Agron. 66, 95–101. doi: 10.15446/acag.v66n1.53171
Myers, N., Mittermeier, R.A., Mittermeier, C.G., and da Fonseca, G.A.B. (2000). Biodiversity hotspots for conservation priorities. Nature. 403, 853–858. doi: 10.1038/35002501
Nemani, R.R. Keeling, C.D., Hashimoto, H., Jolly, W.M., Piper, S.C., Tucker, C.J., et al., (2003). Climate-driven increases in global terrestrial net primary production from 1982 to 1999. Science. 300, 1560–1563. doi: 10.1126/science.1082750
Nottingham, A.T., Meir, P., Velasquez, E., and Turner, B. (2020). Soil carbon loss by experimental warming in a tropical forest. Nature. 584, 234–237 doi: 10.1038/s41586-020-2566-4
Nottingham, A.T. Whitaker, J., Turner, B.L., Salinas, N., Zimmermann, M., Malhi, Y., et al., (2015). Climate warming and soil carbon in tropical forests: Insights from an elevation gradient in the Peruvian Andes. Bioscience 65, 906–921. doi: 10.1093/biosci/biv109
Pauli, H., Gottfried, M., Reiter, K., and Grabherr, G. (2001). “High mountain summits as sensitive indicators of climate change effects on vegetation patterns: the “multi summit-approach” of gloria (global observation research initiative in alpine environments)”. eds G. Visconti, M. Beniston, E.D. Iannorelli, and D. Barba. in Global Change and Protected Areas. Advances in Global Change Research. (Dordrecht: Springer).
Pauli, H., Gottfried, M. and Grabherr, G. (1996). Effects of climate change on mountain ecosystems – upward shifting of alpine plants. World Res. Rev. 8, 382–390.
Pepin, N., Bradley, R.S., Diaz, H.F., Baraer, M., Caceres, E.B., Forsythe, N., et al., (2015). Elevation-dependent warming in mountain regions of the world. Nat. Clim. Change. 5, 424–430. doi: 10.1038/nclimate2563
Peyre, G., Lenoir, J., Karger, D.N., Gomez, M., Gonzalez, A., Broennimann, O., et al., (2020). The fate of páramo plant assemblages in the sky islands of the northern andes. J. Veg. Sci. 31, 967–980. doi: 10.1111/jvs.12898
Podwojewski, P., Poulenard, J., Zambrana, T. and Hofstede, R. (2002). Overgrazing effects on vegetation cover and properties of volcanic ash soil in the páramo of llangahua and la esperanza (tungurahua, ecuador). Soil Use Manage. 18, 45–55. doi: 10.1111/j.1475-2743.2002.tb00049.x
Poulenard, J., Podwojewski, P., and Herbillon, A. J. (2003). Characteristics of non-allophanic andisols with hydric properties from the ecuadorian páramos. Geoderma 117, 267–281. doi: 10.1016/S0016-7061(03)00128-9
Powers, J.S., Montgomery, R.A., Adair, E.C., Brearley, F.Q., DeWalt, S.J., Castanho, C.T., et al., (2009). Decomposition in tropical forests: a pan-tropical study of the effects of litter type, litter placement and mesofaunal exclusion across a precipitation gradient. J. Ecol. 97, 801–811. doi: 10.1111/j.1365-2745.2009.01515.x
Pregitzer, K.S., King, J.S., Burton, A.J. and Brown, S.E. (2000). Responses of tree fine roots to temperature. New Phytol. 147, 105–115. doi: 10.1046/j.1469-8137.2000.00689.x
Rada, F., Azócar, A. and García-Núñez, C. (2019). Plant functional diversity in tropical Andean páramos. Plant Ecol. Divers. 12, 1–15. doi: 10.1080/17550874.2019.1674396
Radville, L., Post, E. and Eissenstat, D.M. (2016). Root phenology in an arctic shrub-graminoid community: the effects of long-term warming and herbivore exclusion. Clim. Chang. Resp. 3:4. doi: 10.1186/s40665-016-0017-0
Raich, J.W., Potter, C.S. and Bhagawati, D. (2002). Interannual variability in global soil respiration, 1980-94. Glob. Change Biol. 8, 800–812. doi: 10.3334/CDIAC/lue.ndp081
Raich, J.W. and Schlesinger, W.H. (1992). The global carbon dioxide flux in soil respiration and its relationship to vegetation and climate. Tellus 44, 81–99. doi: 10.3402/tellusb.v44i2.15428
Robinson, C.H. (2002). Controls on decomposition and soil nitrogen availability at high latitudes. Plant Soil 242, 65–81. doi: 10.2307/24120905
Root, T.L., Price, J.T., Hall, K.R., Schneider, S.H., Rosenzweigh, C. Pounds, J.A. et al. (2003). Fingerprints of global warming on wild animals and plants. Nature 421, 57–60. doi: 10.1038/nature01333
Rousk, K., and A. Michelsen. (2017). Ecosystem nitrogen fixation throughout the snow-free period in subarctic tundra: effects of willow and birch litter addition and warming. Glob. Change Biol. 23, 1552–1563. doi: 10.1111/gcb.13418
Ruess, R.W., Hendrick, R.L., Burton, A.J., Pregitzer, K.S., Sveinbjornssön, B., Allen, M.F., et al. (2003). Coupling fine root dynamics with ecosystem carbon cycling in Black spruce forests of interior alaska. Ecol. Monogr. 73, 643–662. doi: 10.1890/02-4032
Rustad, L.E., Campbell, J.L., Marion, G.M., Norby, R.J., Mitchell, M.J., Hartley, A.E. et al., (2001). A meta-analysis of the response of soil respiration, net nitrogen mineralization, and aboveground plant growth to experimental ecosystem warming. Oecologia 126, 543–562. doi: 10.1007/s004420000544
Salinas, N., Malhi, Y., Meir, P., Silman, M., Roman-Cuesta, R., Huaman, J., et al. (2011). The sensitivity of tropical leaf litter decomposition to temperature: results from a large-scale leaf translocation experiment along an elevation gradient in peruvian forests. New Phytol. 189, 967–977. doi: 10.1111/j.1469-8137.2010.03521.x
Sánchez, M.E., Chimner, R.A., Hribljan, J.A., Lilleskov, E.A., and Suárez, E. (2017). Carbon dioxide and methane fluxes in grazed and undisturbed mountain peatlands in the ecuadorian andes. Mires Peat 19, 1–18. doi: 10.19189/MaP.2017.OMB.277
Schaefer, K. Zhang, T., Bruhwiler, L., and Barrett, A. P. (2011). Amount and timing of permafrost carbon release in response to climatewarming. Tellus B Chem. Phys. Meteorol. 63, 168–180. doi: 10.1111/j.1600-0889.2011.00527.x
Schlesinger, W.H. and Andrews, J.A. (2000). Soil respiration and the global carbon cycle. Biogeochemistry 48:7. doi: 10.1023/A:1006247623877
Shaver, G.R., Canadell, J., Chapin, F. S., Gurevitch, J., Harte, J., and Henry, G., et al., (2000). Global warming and terrestrial ecosystems: a conceptual framework for analysis. Bioscience 50, 871–882. doi: 10.1641/0006-3568(2000)050[0871:gwatea]2.0.co;2
Shaver, G.R., Johnson, L.C., Cades, D.H., Murray, G., Laundre, J.A., Rastetter, E.B., et al., (1998). Biomass and CO2 flux in wet sedge tundra: responses to nutrients, temperature, and light. Ecol. Monogr. 68, 75–97. doi: 10.2307/2657144
Shaver, G.R. and Jonasson, S. (1999). Response of arctic ecosystems to climate change: results of long-term field experiments in sweden and alaska. Polar Res. 18, 245–252. doi: 10.1111/j.1751-8369.1999.tb00300.x
Sklenář, P., and Hedberg, I. and Cleef, A.M. (2014). Island biogeography of tropical alpine floras. J. Biogeogr. 41, 287–297. doi: 10.1111/jbi.12212
Song, J., Wan, S., Piao, S. Knapp, A.K., Classen, A.T., Vicca, S., et al., (2019). A meta-analysis of 1,119 manipulative experiments on terrestrial carbon-cycling responses to global change. Nat. Ecol. Evol. 3, 1309–1320. doi: 10.1038/s41559-019-0958-3
Steinaker, D.F. and Wilson, S.D. (2008). Phenology of fine roots and leaves in forest and grassland. J. Ecol. 96, 1222–1229. doi: 10.1111/j.1365-2745.2008.01439.x
Steinbauer, M.J., Grytnes, J., Jurasinski, G., Kulonen, A., Lenoir, J. Pauli, H., et al. (2018). Accelerated increase in plant species richness on mountain summits is linked to warming. Nature 556, 231–234. doi: 10.1038/s41586-018-0005-6
Sturm, M., Racine, C., and Tape, K. (2001). Climate change—Increasing shrub abundance in the arctic. Nature. 411, 546–547. doi: 10.1038/35079180
Suseela, V., Conant, R.T., Wallenstein, M.D., and Dukes, J.S. (2012). Effects of soil moisture on the temperature sensitivity of heterotrophic respiration vary seasonally in an old-field climate change experiment. Glob. Change Biol. 18, 336–348. doi: 10.1111/j.1365-2486.2011.02516.x
Tape, K., Sturm, M. and Racine, C. (2006). The evidence for shrub expansion in northern alaska and the pan-arctic. Glob. Change Biol. 12, 686–702. doi: 10.1111/j.1365-2486.2006.01128.x
Urrutia, R. and Vuille, M. (2009). Climate change projections for the tropical andes using a regional climate change model: temperature and precipitation simulations for the 21st century. J. Geophys. Res. 114:D02108. doi: 10.1029/2008JD011021
Valencia J.B., Mesa J., León J.G., Madriñán S., and Cortés A.J. (2020). Climate vulnerability assessment of the espeletia complex on páramo sky islands in the northern andes. Front. Ecol. Evol. 8:309. doi: 10.3389/fevo.2020.565708
Vuille, M., Carey, M., Huggel, C., Buytaert, W., Rabatel, A., Jacobsen, D., et al., (2018). Rapid decline of snow and ice in the tropical andes – Impacts, uncertainties and challenges ahead. Earth-Sci. Rev. 176, 195–213. doi: 10.1016/j.earscirev.2017.09.019
Vuille, M., Franquist, E., Garreaud, R., Lavado Casimiro, W.S., and Cáceres, B. (2015). Impact of the global warming hiatus on andean temperature. J. Geophys. Res. Atmos. 120, 3745–3757. doi: 10.1002/2015JD023126
Walker, M.D., Wahren, C.H., Hollister, R.D., Henry, G.H.R., Ahlquist, L.E., Alatalo, J.M. et al., (2006). Plant community responses to experimental warming across the tundra biome. Proc. Natl. Acad. Sci. 103, 1342–1346. doi: 10.1073/pnas.0503198103
Walther, G-R., Beißner, S. and Burga, C.A. (2005). Trends in the upward shift of alpine plants. J. Veg. Sci. 16, 541–548. doi: 10.1111/j.1654-1103.2005.tb02394.x
Wang, Q., Fan, X. and Wang, M. (2016). Evidence of high-elevation amplification versus arctic amplification. Sci. Rep. 6:19219. doi: 10.1038/srep19219
Wei, H., Guenet, B., Vicca, S., Nunan, N., AbdElgawad, H., Pouteau, V., et al., (2014). Thermal acclimation of organic matter decomposition in an artificial forest soil is related to shifts in microbial community structure. Soil Biol. Biochem. 71, 1–12. doi: 10.1016/j.soilbio.2014.01.003
Williams, J.W., Jackson, S.T. and Kutzbach, J.E. (2007). Projected distributions of novel and disappearing climates by 2100 AD. Proc. Natl. Acad. Sci. 104, 5738–5742. doi: 10.1073/pnas.0606292104
Wood, T.E., Cavaleri, M.A., Giardina, C.P., Khan, S., Mohan, J.E., and Nottingham, A., et al., (2019). “Soil warming effects on low-latitude forests with highly-weathered soils”, ed. J.E. Mohan. in Ecosystem Consequences of Soil Warming. (London: Elsevier, Academic Press). 385–439. doi: 10.1016/b978-0-12-813493-1.00015-6
Xue, K., Xie, J., Zhou, A., Liu, F., Li, D., Wu, L., et al., (2016). Warming alters expressions of microbial functional genes important to ecosystem functioning. Front. Microbiol. 7:668. doi: 10.3389/fmicb.2016.00668
Zhou, J, Tape, KD, Prugh, L, Kofinas, G, Carroll, G, Kielland, K. et al. (2020). Enhanced shrub growth in the Arctic increases habitat connectivity for browsing herbivores. Glob Change Biol. 26, 3809–3820. doi: 10.1111/gcb.15104
Zimmer, A., Meneses, R.I., Rabatel, A., Soruco, A., Dangles, O., Anthelme, F. et al. (2018). Time lag between glacial retreat and upward migration alters tropical alpine communities. Perspect. Plant Ecol. Evol. Syst. 30, 89–102. doi: 10.1016/j.ppees.2017.05.003
Keywords: carbon emission, climate change, litter decomposition, IPPEX, OTC, tropical alpine ecosystems, carbon sinks, soil respiration
Citation: Lasso E, Matheus-Arbeláez P, Gallery RE, Garzón-López C, Cruz M, Leon-Garcia IV, Aragón L, Ayarza-Páez A and Curiel Yuste J (2021) Homeostatic Response to Three Years of Experimental Warming Suggests High Intrinsic Natural Resistance in the Páramos to Warming in the Short Term. Front. Ecol. Evol. 9:615006. doi: 10.3389/fevo.2021.615006
Received: 07 October 2020; Accepted: 22 January 2021;
Published: 17 February 2021.
Edited by:
Luis Daniel Llambi, Universidad de Los Andes, VenezuelaReviewed by:
Fabien Anthelme, Research Institute for Development, FranceXiaoli Hu, Nanjing University, China
Copyright © 2021 Lasso, Matheus-Arbeláez, Gallery, Garzón-López, Cruz, Leon-Garcia, Aragón, Ayarza-Páez and Curiel Yuste. This is an open-access article distributed under the terms of the Creative Commons Attribution License (CC BY). The use, distribution or reproduction in other forums is permitted, provided the original author(s) and the copyright owner(s) are credited and that the original publication in this journal is cited, in accordance with accepted academic practice. No use, distribution or reproduction is permitted which does not comply with these terms.
*Correspondence: Eloisa Lasso, ZS5sYXNzb0B1bmlhbmRlcy5lZHUuY28=