- 1Chengdu Institute of Biology, Chinese Academy of Sciences, Chengdu, China
- 2School of Ecological and Environmental Sciences, East China Normal University, Shanghai, China
- 3School of Resource and Environment, Aba Teachers University, Aba, China
- 4Neijiang Academy of Agricultural Sciences, Neijiang, China
- 5International Centre for Integrated Mountain Development, Kathmandu, Nepal
Phenotypic plasticity among natural plant populations is a species-specific ecological phenomenon of paramount importance that depends on their life forms, development stages, as well as environmental factors. While this phenomenon is broadly understood, it has hardly been observed in nature. This study aimed at understanding phenotypic plasticity and ecological adaptability in three shrubs (Salix etosia, Rubus setchuenensis, and Hydrangea aspera) affected by potential environmental variables after deforesting in sparse Larix spp. forest and tall shrub mixed secondary forests. Soil organic carbon content, total nitrogen content, and available nitrogen content were greater outside the forests, contrary to other measured factors whose availability was higher in the forest interiors. In case of leaf traits and stoichiometric indicators, there were significant interactions of leaf area (LA), leaf dry matter (DW), specific leaf area (SLA), and leaf phosphorus content (LPC) between shrub species and heterogeneous environments (P < 0.05) but not for leaf C/N, N/P, and C/P. Principal components analysis (PCA) indicated that soil temperature, pH value, soil carbon content, soil nitrogen content, and MBC and MBN mainly constituted the first component. Summarized results indicated that TB and leaf C/P of S. etosia were significantly correlated with three principal components, but only marginal significant correlations existed between R/S and relevant components. SLA and R/S of R. setchuenensis had marginal significant relationships with independent variables. Both SLA and TB of H. aspera were significantly correlated with three principal components. Based on the pooled values of leaf functional traits and leaf stoichiometric indicators, R. setchuenensis (vining type) had better leaf traits plasticity to adapt to a heterogeneous environment. In descending order, the ranks of biomass allocation plasticity index of three shrubs were H. aspera (bunch type), R. setchuenensis (vining type), and S. etosia (erect type). The highest integrated plasticity values of leaf traits and biomass allocation was observed in H. aspera (bunch type), followed by R. setchuenensis, and by S. etosia with less adaptive plasticity in heterogeneous environments.
Introduction
Natural environments are heterogeneous at different scales for plants that not only affect their growth and development but also influence responses and adaptation of plants from functional traits to ecosystems (Stewart et al., 2000; Hutchings and John, 2004). Most knowledge of plant growth accumulated under homogenous conditions still cannot be universally applicable for the patchy resource availability in natural environments. It has been acknowledged that responses of plants to environmental heterogeneity are species specific and also depend on multiple aspects such as life forms, and development stages, among others. At global to local scales, deforestation can generate many environmental problems and reflect the spatio-temporal ecosystem complexity and interaction of biophysical, social, ecological, and human activity components (van Doorn and Bakker, 2007). Globally, nearly 6 million km2 of forest and woodland have been cleared for farming or are being extensively since 1850 (Ramankutty and Foley, 1999), which had serious repercussions on the natural habitats of many plant species due to habitat fragmentation, loss of plant populations, and therefore a decline in genetic variability including morphological, physiological, and behavioral traits, eventually reducing individual plant fitness (Grime, 1994). The spatial heterogeneity of essential resources and concerned environmental factors can affect allocation and growth of plant organs or different parts, the whole plants, even the yield and spatial structure of populations and communities (Grime, 1994; Stewart et al., 2000; King, 2003; Hutchings and John, 2004; Aguilar et al., 2006). The phenotypic plasticity of a plant is its ability to change in response to stress, disturbance, or inputs from the environment. It is critical to ascribe observed phenotypic variation in the field by experimental approach regarding phenotypic plasticity rather than genetic variation. Morphological and physiological responses of plants to environmental change have been well documented at both the organ level and organism level, including variation of growth patterns or biomass allocation. Deforestation results in an uneven distribution of environmental factors with stark differences of environmental conditions inside and outside the forests (Chen et al., 1996; Valladares et al., 2000; King, 2003; Rozendaal et al., 2006). This creates different land use types or land forms with heterogeneous habitat characteristics and forest gaps, which play a critical role in growth and development of plants, and affects community composition, structure, and functioning. For instance, an imminent regime shift toward an alternative tropical scrubland ecosystem state may result in warmer and drier conditions at the local scale (Lawrence and Vandecar, 2015).
Plant species may or may not adapt to changing surroundings by involvement of a confounding variation in morphology, physiological state, or behavior, or some combination of these, at a multi-level of organization. The phenotype can be all of the characteristics or performance of an organism other than its genes, whose nature has been influenced by both environment and genome (Grassi and Bagnaresi, 2001; Sih, 2004; Rozendaal et al., 2006). Performance and response of plants to changing environment are complicated and need to be understood in a proper way. Survival rate and competition capacity of plants in a community represent their adaptive ability to diverse habitats (Grassi and Bagnaresi, 2001). Plant functional traits are the reflection of adaptation to environmental variations, disturbances, and tradeoffs among different functions (Chen et al., 1996; Rozendaal et al., 2006). Leaves are the main site for photosynthesis, organic production, as well as the basic unit for energy exchange of the atmosphere–plant system, and are therefore the fundamental elements in maintaining terrestrial ecosystems (Chen et al., 1996; Cornelissen et al., 2003). Thus, leaf traits can directly reflect the survival strategies of plants under environmental change, which is also closely related to resource capture and utilization (Chen et al., 1996; Rozendaal et al., 2006). There exists a significant positive relationship between specific leaf area (SLA), potential relative growth rate, and photosynthetic rate, which reflects the plant characteristics for adaptation to different habitats and strategies of carbon acquisition (Reich et al., 1991, 1992; Vile et al., 2005; Rozendaal et al., 2006). Furthermore, carbon (C), nitrogen (N), and phosphorus (P) are the most essential elements for the structure and function of cells, and are correlated with plant metabolism (Reich et al., 1991; Rw and Jj, 2002). Leaf carbon content (LCC), leaf nitrogen content (LNC), leaf phosphorus content (LPC), and N/P ratio are important indicators of leaf traits as well. Thus, it is essential and meaningful to explore the response of leaf traits in heterogeneous environments, to enhance our understanding about fitness of plants in a changing world.
Biomass plays a critical role in the ecosystem since it directly influences key ecological processes such as hydrology, erosion, nutrient cycling, carbon storage, and biological diversity (Houghton et al., 2001; Costa et al., 2012). Biomass allocation is therefore an important factor in the characterization of plant physiological ecology. Moreover, it is also the result of the plants’ long-term adaption to different environmental conditions (Cairns et al., 1997). The aboveground and belowground biomass allocation affects plant growth as well as the overall function of the ecosystem and the biogeochemical cycles (Cairns et al., 1997; Yang et al., 2009). Therefore, the mechanism by which plants respond to variations in the availability of resources in their environment is a major question in plant ecology. Plants can optimize growth under a variety of environments by shifting their biomass allocation between non-photosynthesizing tissues and tissues associated with C-gain. For example, the greater root mass allocation or increased root length can improve water acquisition under drought conditions (Yang et al., 2010). The arrangement of mass within organs can vary substantially, independent of patterns of biomass allocation between organs. Variations in allocation within an organ can lead to patterns of biomass partitioning between different organs that show little relationship to the relative “functional strength” of these organs (Cairns et al., 1997; Yang et al., 2009, 2010). For example, relative biomass allocation between roots and shoots shows weak relationship with the relative amount of root length supported per unit leaf area (Körner and Renhardt, 1987; Archer, 1989; Larigauderie et al., 1991; Aerts et al., 1992). Biomass redistribution among plant parts is also significantly correlated with plant C and N status, such that the relative concentrations of C and N affect plant growth and biomass partitioning (Aerts et al., 1992; Eilts et al., 2011). In addition, C assimilation in plants depends on external resources’ availability, interacting with other environmental factors. For better investigating and understanding the underlying adaptive mechanism of plants and human activity-induced heterogeneous environmental conditions need to be identified from correlated abiotic factors in the experimental procedures.
Phenotypic plasticity in plants is a crucial pathway to adapt to heterogeneous environments, which enables them to change their structure and function under changing environment (Matesanz et al., 2010). Plasticity of certain functional traits may be beneficial in new environmental conditions with better fitness (Tilman, 1982). The ecological consequences of deforestation have been widely investigated in theoretical research and empirical data. The research tendency has become from focusing on the relative isolated remnants of habitat to more realistic conditions in which the areas of altered habitat are not completely destroyed (Malanson et al., 2007). Wild species are severely affected by interactions with human’s deforestation, and species respond differently because of their different dispersal abilities (van Doorn and Bakker, 2007; Lawrence and Vandecar, 2015).
It is essential to investigate the adjustments of plant morphological traits and biomass allocation patterns, and to understand the adaptation of plants along with different environmental gradients. The morphological characteristics of different organs can respond to heterogeneous environments after anthropogenic activities and result in corresponding adjustments in phenotypic plasticity (Grime, 1994; Aguilar et al., 2006; King, 2003; Chen et al., 1996; Valladares et al., 2000; Grassi and Bagnaresi, 2001; Sih, 2004; Cornelissen et al., 2003). For specific ecological characteristics, plants can perform differently for adapting to changing environments (e.g., vining type, bunch type, erect type, etc.). Shrubs play a critical role not only in community succession but in various ecosystem functioning and services. In particular, they occupy a certain layer in the spatial distribution of forests, whose competition can be relived in underground environment of forests after human beings’ disturbance. In this study, we selected three shrub species with different life forms and survival strategies in Southern China, whose leaf traits and biomass allocation were observed and measured prior to and posterior to deforestation. Sparse Larix spp. comprise a large proportion of the secondary forests, whose stand age is nearly 20 years old, height is 10–15 m, and canopy density is between 0.7 and 0.8, associated with several tall shrubs with different life forms (3–5 m in height) and dense herbaceous plants (Dactylis glomerata, Lolium perenne, Imperata cylindrical with around 50–60% coverage) in different synusia, while logging or even deforesting in large amounts formed the so-called outside the forests, with scattered shrub individuals in secondary grass cluster. Last, the pooled phenotypic plasticity in spatially heterogeneous habitats and correlated environmental factors were demonstrated to test their response to changing environments. The study focused on addressing three questions as follows. (1) How are leaf traits and biomass partitioning of three shrubs different under heterogeneous environments after deforestation? (2) How do abiotic and biotic factors affect leaf traits and biomass allocation in the three shrubs due to many changed variables? (3) What are the variations of three shrubs’ phenotypic plasticity regarding leaf traits and biomass partitioning in two contrasting habitats?
Materials and Methods
Study Sites
The experiment was conducted at the Qishan Pasture (30°35’N,108o54’E, 1,300 m a.s.l.) in Yunyang County, Northwestern of Chongqing Municipality (Figure 1). According to the data from local mini-automatic meteorological station (HOBOWARE 3.0), the annual mean temperature, mean temperature in July and January are 10.7, 20.6, and 1.5°C, respectively. Mean annual precipitation is 1,300 mm, 80% of which is distributed from the months of April to November. The annual total photosynthetic active radiation and total photosynthetic radiation are 2,750 uE and 1,288 W/m2, respectively. Soil water content is about 30–35%. The study area is well known as Karst landform. The soil type is classified as mountain yellow soil mixed with gravel. According to the previous scoping study, the soil pH value was significantly greater outside than that inside the forests, while the soil organic carbon content (SOC), soil total nitrogen content (STN), and soil available nitrogen content (SAN) were significantly less outside the forests than those inside the forests. There were no apparent differences in soil available phosphorus (SAP), soil microbial biomass carbon (MBC), and soil microbial biomass nitrogen (MBN) between outside and inside the forests, although marginal significant differences existed for MBC (F = 6.78, P = 0.06). The dominant shrubs in the study area are Salix etosia, Rubus setchuenensis, Hydrangea aspera, and Cotoneaster dielsianus. Herbaceous plants are Dactylis glomerata, Lolium perenne, Imperata cylindrical, Artemisia roxburghiana, Trifolium repens, Hypericum monogynum, and Sanguisorba officinalis.
Experimental Design and Sampling
Experimental Design
The experiment was conducted with three shrubs (i.e., S. etosia, R. setchuenensis, and H. aspera) outside and inside the forests representing two contrasting environmental conditions after deforestation. At the end of 2011, some mosaic sites around 50 m × 50 m in the forests were logged to be open spaces. It fits to compare three understory species between outside and inside the forests influenced by multiple environmental factors. In May 2013, three paired blocks were chosen in both outside and inside the forests with five individuals of each shrub species. From each individual plant, three to five intact and healthy leaves were collected across the second to the fifth lateral branch (120 < N < 200 in each species). Since three shrubs included vining type, bunch type, and erect type, they basically represented common dominant shrub species at the study site. Inside the forests, it refers to sparse Larix spp. secondary forests and tall shrub mixed forests, while outside the forests it means scattered shrub individuals in secondary grass cluster. They were under full exposure outside the forest with 100% light transmittance but 30–50% on average inside the forests. Light densities outside and inside the forests were measured by an illuminometer (Digital Illuminance Miter, TES-1332A), and photosynthetically active radiation (PAR) was compared by LI-6400 (LI-COR Inc, Nebraska, U.S.A) with 60% relative humidity at 24°C, which were conducted in sunny days from 9:00 am to 11:00 am, 10 cm above the shrubs’ canopy.
Leaf Traits and Biomass Measurements
The whole plant biomass was collected by the excavation method after measuring the photosynthetic physiological indexes. Shoot and root were separated by secateurs with all root systems kept carefully. Biomass allocation of different plant parts were categorized as leaf biomass allocation (LBA), new branch biomass allocation (NBBA), aging branch biomass allocation (ABBA), root biomass allocation (RBA), ratio of leaf biomass account for aboveground biomass (LB/AGB), ratio of new branch biomass account for aboveground biomass (NBB/AGB), ratio of aging branch account for aboveground biomass (ABB/AGB) and Log10root to Log10shoot ratio (LgR/LgS). Sampled fresh leaves were scanned by Canon Scan Gear and calculated by Photoshop CS4 and Matlab 7.9 for their leaf area (LA). All the leaves were oven dried at 105°C for 30-min implement then at 80°C until the weight was constant for the dry weight (DW) by electronic balance. Specific leaf area was calculated using the methodology from Vile et al. (specific leaf area = leaf area/leaf dry weight) (Sih, 2004). All the leaf samples were milled to powder and then sieved through a 100-mesh sieve for nutrient content test. The collected plant samples were placed in paper envelopes and were oven dried at 65°C in the laboratory. Dry mass of all the samples was measured by electronic balance (accuracy 0.001 g) and were ground to be fine powder for further procedures of chemical analysis. C content and N content were determined by dry combustion with a CHNS autoanalyzer system (Elementar Analysen Systeme, Hanau, Germany) (Larigauderie et al., 1991). P content was obtained colorimetrically by the chloromolybdophosphoric blue color method after wet digested in a mixture of HNO3, H2SO4, and HClO4 solution.
Soil Sample Collecting
Soil temperature, soil water content, and soil bulk density were measured at a depth of 5 cm both inside the forests and outside the forests. Soil temperature was determined with a button thermometer (iButton-TMEX RTE). Soil bulk density was estimated using the cutting ring technique while oven-drying method for soil water content.
Data Analysis
The experiment data was conducted in one-way ANOVA to compare the environmental factors between inside and outside the forests in the scoping study. Besides, a general linear model (GLM) was adopted to ascertain the effects of species and two heterogeneous environments on plant leaf traits, biomass allocation, and phenotypic plasticity, as well as the interactions between the two factors. A comparative analysis was performed with one-way ANOVA statistics for the effects of changed environmental conditions after deforestation on the leaf traits of the three shrubs. Once there was a significant difference, the post hoc multiple comparisons were processed with Tukey’s test. Phenotypic plasticity index was calculated based on the Valladares et al. (2000) method where each indicator’s phenotypic plasticity index was presented with a ratio of the difference between maximum and minimum average to maximum under heterogeneous habitats (Chen et al., 1996). Phenotypic plasticity index of biomass allocation was the average of all biomass allocation indexes. Total plasticity index was computed as the mean of morphology plasticity (leaf traits) index and biomass allocation plasticity index, both of which are calculated by consisted indicators (leaf traits plasticity index: individual leaf mass, individual leaf area, specific leaf area, leaf carbon content, leaf nitrogen content, leaf phosphorus content, leaf carbon content/leaf nitrogen content, leaf carbon content/leaf phosphorus content, and leaf nitrogen content/leaf phosphorus content; biomass allocation plasticity index: LB/AGB, NBB/AGB, ABB/AGB, LBA, NBBA, ABBA, RBA, and lgR/lgS), respectively. The data were tested for normality and homogeneity of variances before comparison of means; otherwise, logarithmic transformations were conducted to meet the precondition. Last, the principal component analysis (PCA) was conducted to demonstrate the effects of abiotic factors and biotic factors on leaf trait and biomass allocation on the three shrubs. The data analysis was processed in the SPSS19.0 software package (SPSS Inc., Chicago, IL, United States). Figures were drawn and plotted with Origin 8.5.
Results
Leaf Traits of the Three Shrubs Outside and Inside the Forest
The SLA and LA of H. aspera presented significant differences outside and inside the forests. Quantiatively, the values for both SLA and LA were nearly twice inside the forests compared with those outside the forests (Figures 2A,C and Table 1; P < 0.05). There were significant differences in DW of R. setchuenensis under heterogeneous habitats (Figure 2B, P < 0.05). The SLA of S. etosia presented a decreasing trend from outside the forests (134.48 ± 22.90) cm2 g–1 to inside the forests (131.10 ± 22.63) cm2 g–1 with no significant difference. However, the SLAs of R. setchuenensis and H. aspera were significantly greater inside the forest than outside. In descending order, the SLA ranks of the three shrubs were H. aspera, R. setchuenensis, and S. etosia (Figure 2C). There was significant variation in LCC for H. aspera (496.74 ± 12.74 mg g–1 and 530.85 ± 20.00 mg g–1) but not for R. setchuenensis and S. etosia outside and inside the forests (Figure 2D). LNC and SLA had a similar trend outside and inside the forest (Figures 2C,E). The LNC amplitude of variation of S. etosia was just 4.8% and did not have a significant difference (P > 0.05). However, the LNC of H. aspera and R. setchuenensis significantly increased from outside to inside the forest with 24.07 and 12.54% variation amplitudes, respectively. The LPCs of R. setchuenensis and S. etosia were greater outside the forest than those inside, but H. aspera was just the opposite (Figure 2F).
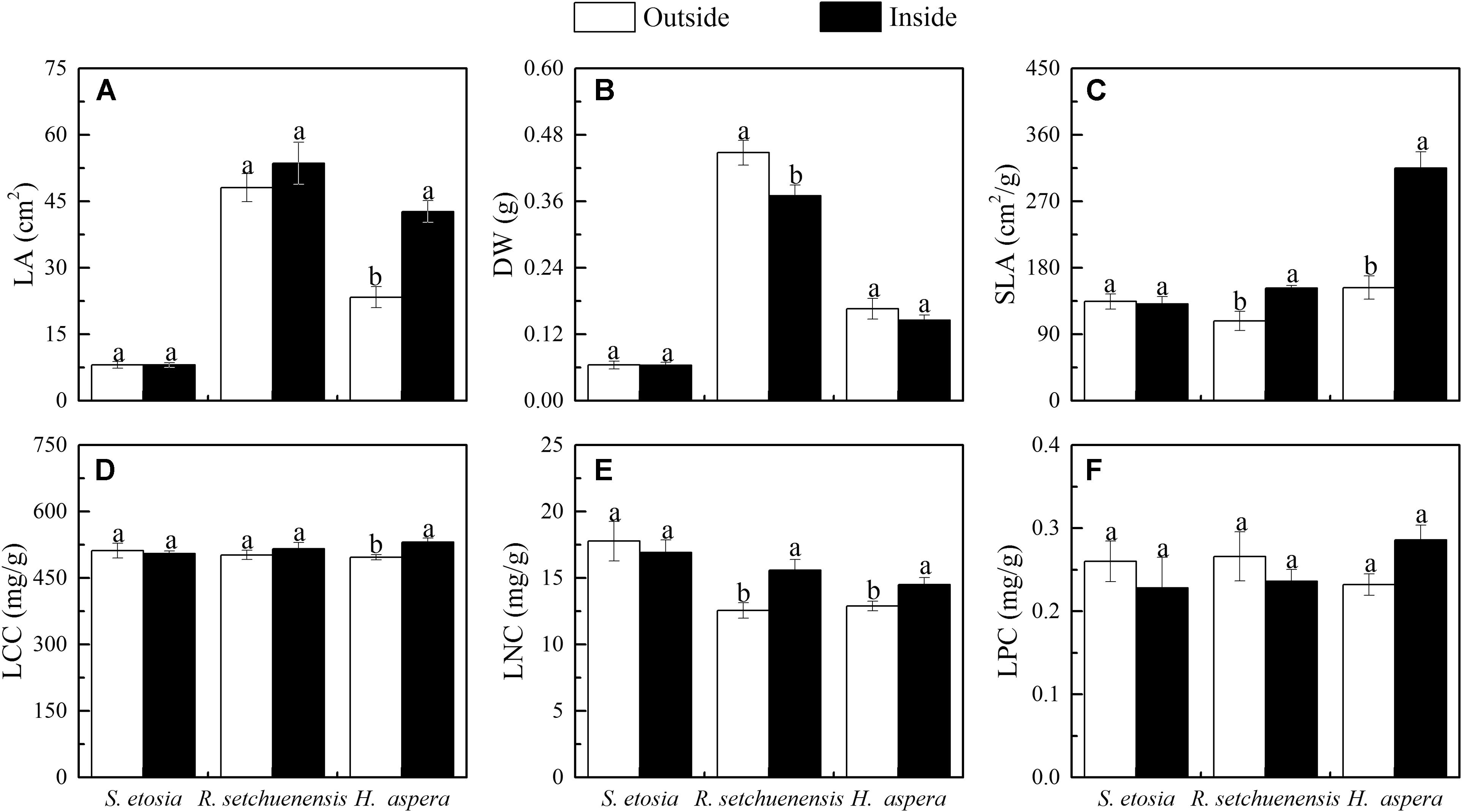
Figure 2. Comparison of leaf traits outside and inside; sample numbers, N: 120 < N < 200. Different lowercases indicate significant differences of leaf traits between two habitats, and the bars represent standard error. (A) LA, leaf area; (B) DW, dry weight; (C) SLA, specific leaf area; (D) LCC, leaf carbon content; (E) LNC, leaf nitrogen content; and (F) LPC, leaf phosphorous content (the same as below).
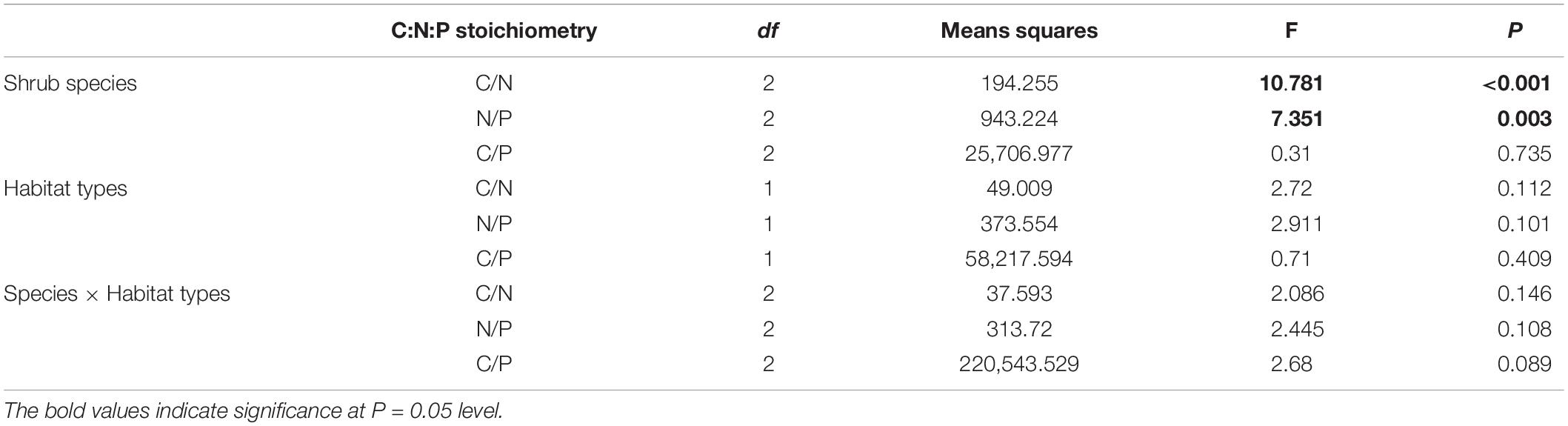
Table 2. Two-way ANOVA analysis of C:N:P stoichiometry between three shrubs under heterogeneous environments.
According to the information provided in Table 1, there were significant differences in LA, DW, SLA, and LNC among the three shrubs (P < 0.01). However the differences in LCC and LPC were not found to be significant. Environmental factors inside and outside the forest affected SLA, LA, and DW significantly, but they did not influence the LCC and LNC (P > 0.05). LA, DW, SLA, and LNC differed significantly between the three shrub species (F = 13.675, P < 0.001; F = 7.199, P < 0.013; F = 37.768, P < 0.001; F = 10.707, P < 0.001). There were also significant differences in the LA, DW, and SLA under two environmental conditions (F = 750.391, P < 0.001; F = 1,169.432, P < 0.001; F = 37.095, P < 0.001). There were significant interactions of LA, DW, SLA, and LPC between shrub species and habitats (P < 0.05).
C:N:P Stoichiometry of Three Shrubs Outside and Inside the Forests
There were no significant differences in leaf C/N, N/P, and C/P for the three shrub species outside and inside the forest. However, if only the variations between the three species are compared, there were significant differences of C/N (Figure 3A and Table. 2, F = 10.781, P < 0.001) and N/P (Figure 3C, F = 7.351, P < 0.05), but not for C/P (Figure 3B, P > 0.05). Both leaf N/P ratios of S. etosia and R. setchuenensis were greater outside the forest than those inside the forest, while H. aspera was in adverse.
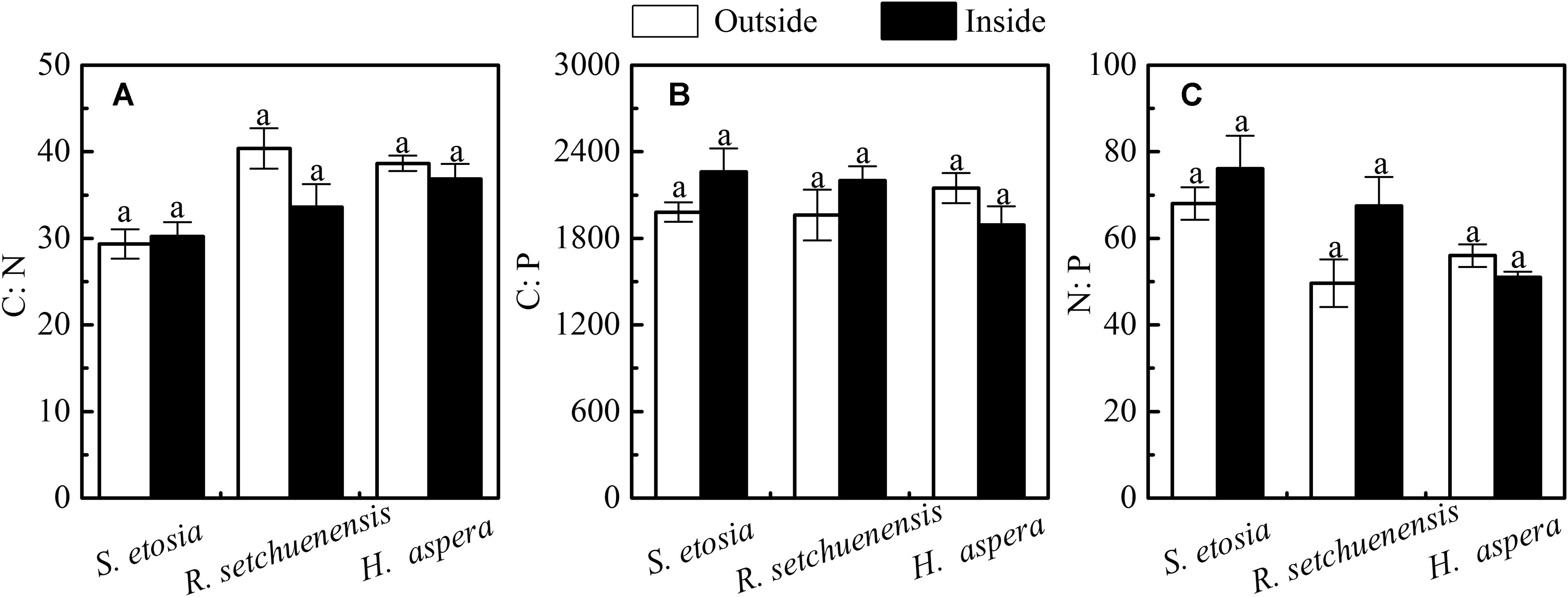
Figure 3. Leaf C:N:P stoichiometry of the three shrubs outside and inside; sample numbers, N: 120 < N < 200. (A–C) refer stoichiometric ratios leaf carbon content/leaf nitrogen content, leaf carbon content/leaf phosphorus content, and leaf nitrogen content/leaf phosphorus content, respectively.
Biomass Allocation of Different Parts of Shrubs Outside and Inside the Forests
There was no significant difference in the LB/AGB of S. etosia and R. setchuenensis inside and outside the forest, but the LB/AGB of H. aspera decreased significantly inside the forest (Table 3). Similarly, R. setchuenensis decreased (53% less) significantly from inside to outside (P < 0.05). It was the same for NBBA and ABBA of S. etosia and R. setchuenensis, but not for H. aspera, whose ABB/AGB and LBA significantly increased inside the forest 2.1 times and 1.5 times than those outside the forest, respectively. R. setchuenensis had increased RBA inside 3.9 times greater than those outside the forest. LgR/lgS of H. aspera decreased significantly under environment inside the forest (P < 0.05).
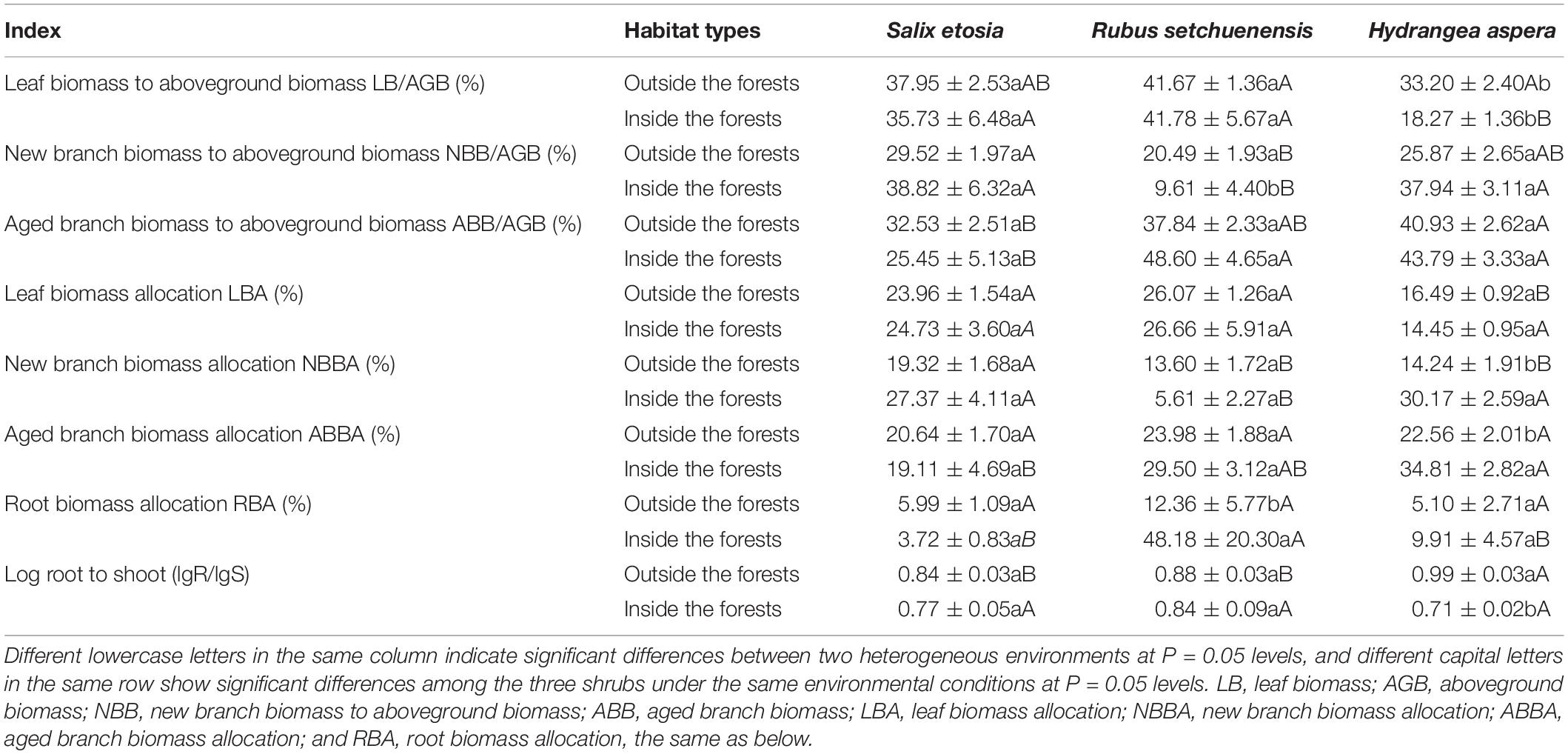
Table 3. Biomass allocation of different organs of three shrubs under two heterogeneous environments.
Effects of Abiotic and Biotic Factors on Leaf Traits and Biomass Allocation
There were three principal components with environmental eigenvalues greater than 1 for all three shrub species, whose cumulative variance proportions were 87.6% (S. etosia), 91.0% (R. setchuenensis), and 93.7% (H. aspera), respectively (Figure 4A). The first principal component (Z1, 57.3%) of S. etosia mainly consisted of pH value, soil carbon content, soil nitrogen content, and MBC and MBN. Its second principal component (Z2, 20.0%) was composed of SWC, SBD, PAR, and ST, and the third principal component (Z3, 10.3%) was formed from SAP and soil temperature (ST) (Figure 4B). Z1 (62.0%) of R. setchuenensis mainly consisted of ST, pH, soil carbon content, and soil nitrogen content, MBC, and MBN. Z2 (19.2%) of R. setchuenensis was composed of SWC, SBD, PAR, and ST, and Z3 (9.4%) was formed by SAP (Figure 4C). Z1 (64.3%) of H. aspera mainly consisted of ST, pH, soil carbon content, and soil nitrogen content, MBC, and MBN. Z2 (20.3%) was made up of SWC, SBD, MBN, and MNC. Z3 (9.2%) of H. aspera was also composed of SAP (Figure 4D).
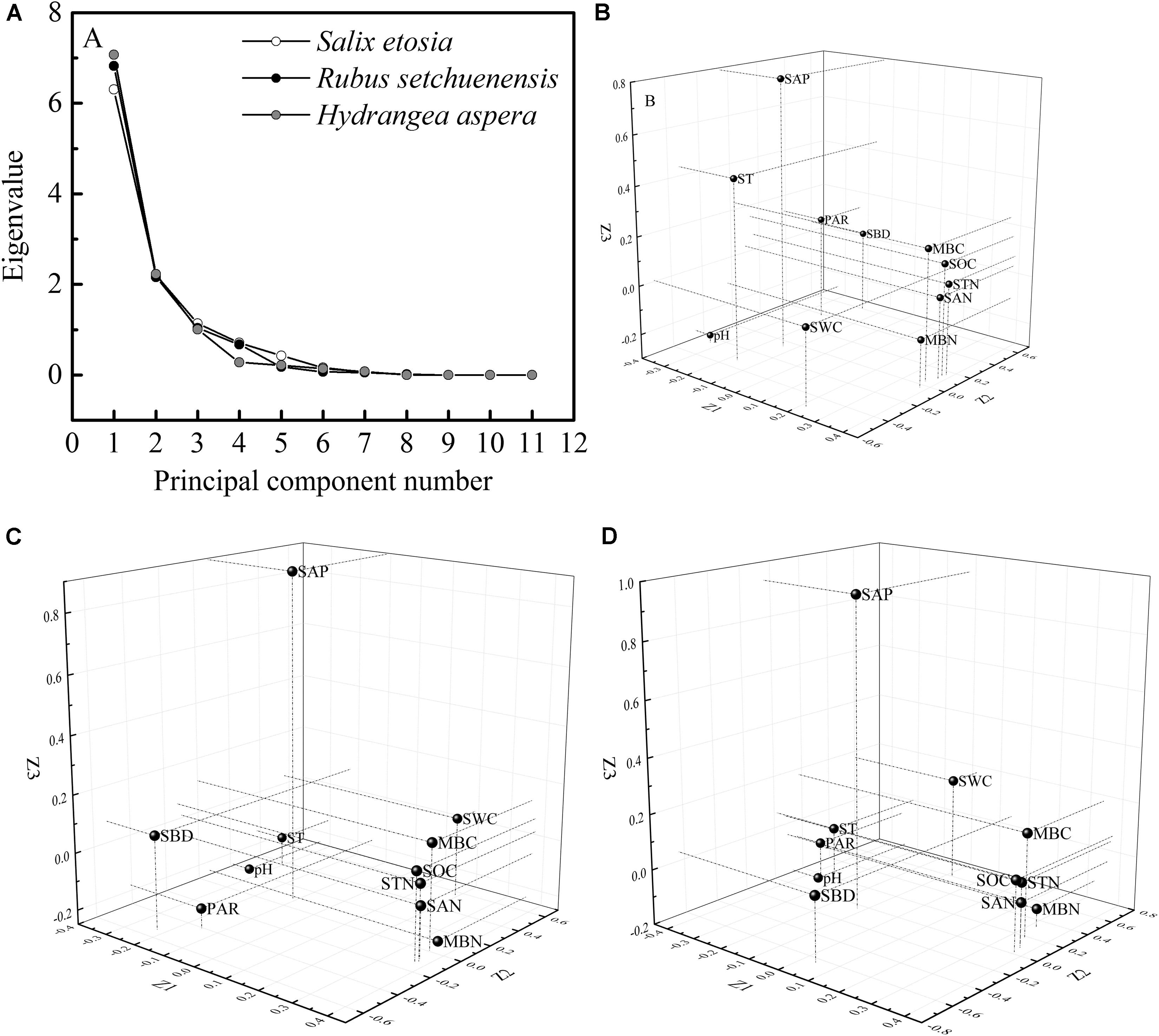
Figure 4. Principal component analysis (PCA) of abiotic factors and biotic factors on leaf trait and biomass allocation of three shrubs. (A) Scree plot of three species’ principal components; (B–D) load factor graph and PCA of Salix etosia, Rubus setchuenensis, and Hydrangea aspera, respectively.
As interpreted in Table 4, TB and leaf C/P of S. etosia were significantly correlated with the three principal components (R2 = 0.75, P = 0.03; R2 = 0.812, P = 0.014). There was marginal significant correlations between R/S and relevant components (R2 = 0.662, P = 0.073). All the dependent variables of R. setchuenensis were not significantly correlated with the three principal components except for SLA and R/S with marginal significant correlations with independent variables (R2 = 0.636, P = 0.090, and R2 = 0.665, P = 0.071, respectively). Both SLA and TB of H. aspera had significant relationships with the three principal components (R2 = 0.894, P = 0.003; R2 = 0.807, P = 0.015).
Plasticity Comparison of the Three Shrubs Calculated From Leaf Traits and Biomass Allocation
Salix etosia had lower SLA plasticity for its weak LA plasticity though it had greater DW. H. aspera was in contrast with S. etosia, and R. setchuenensis was medium (Table 5). For leaf carbon content, leaf nitrogen content, and leaf phosphorus content, S. etosia had greater LCC but lower LPC compared with the others. H. aspera had lower LCC, but the greatest LPC compared with the other two species. R. setchuenensis had the greatest LNP compared with the others. Based on the pooled values of leaf functional traits and leaf stoichiometry indicators, R. setchuenensis had better leaf trait plasticity. S. etosia had the lowest plasticity values of NBBA and RBA. Plasticity values of LB/AGB, LBA, and lgR/lgS of R. setchuenensis were lower than the other two species, but its plasticity values of NBB/AGB, ABB/AGB, NBBA, and RBA were the maximum. H. aspera had greater LB/AGB, LBA, ABBA, and lgR/lgS than the others but presented the lowest ABB/AGB among the three species. In descending order, the ranks of biomass allocation plasticity index of the three shrubs were H. aspera, R. setchuenensis, and S. etosia. After integrating the plasticity values of the leaf traits and biomass allocation, H. aspera reached the first place of total plasticity and was close to R. setchuenensis, followed by S. etosia.
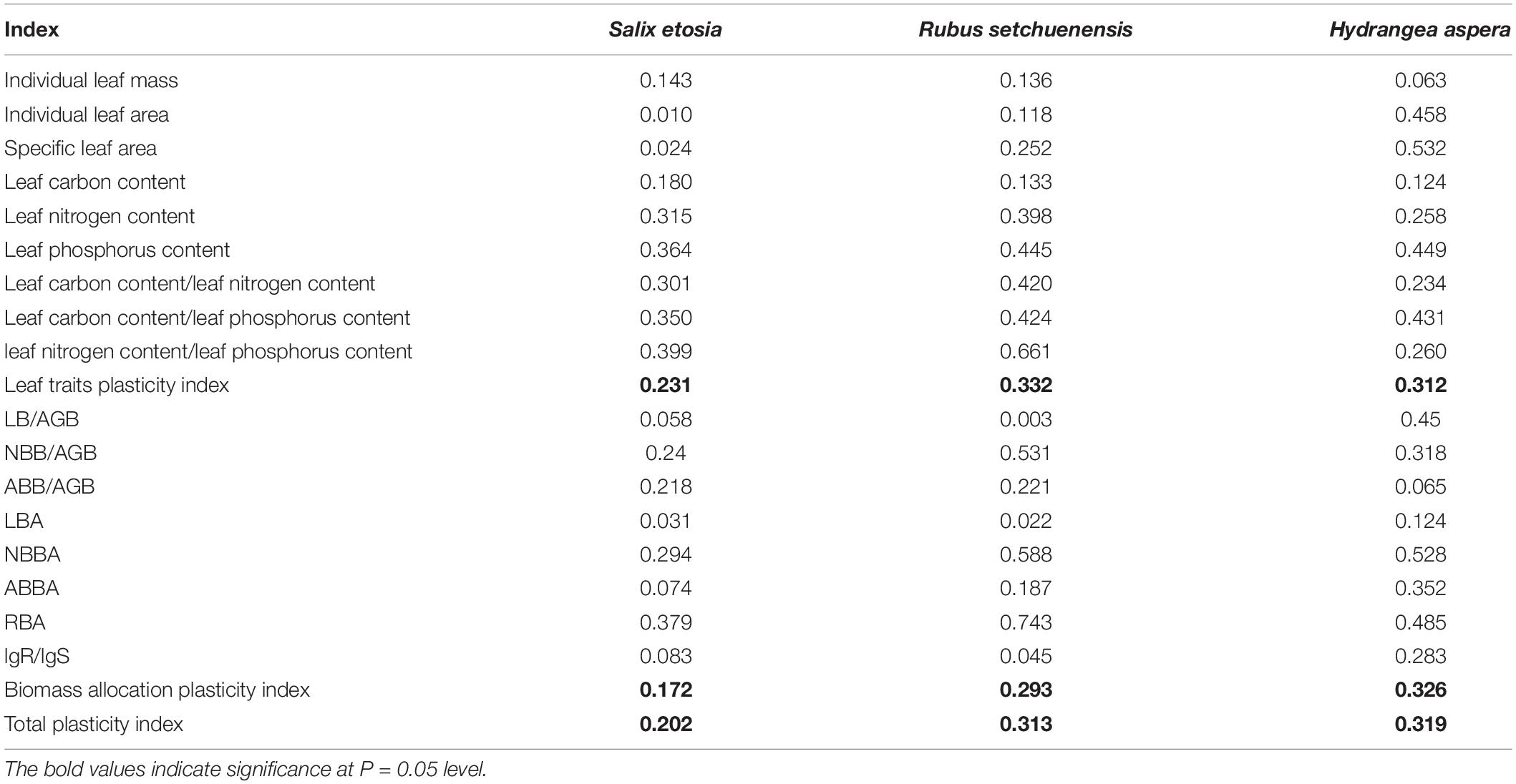
Table 5. Plasticity index comparison of the three shrubs based on leaf traits and biomass allocation.
Discussion
Environmental heterogeneity is among the most important factors in natural systems, which not only influences population dynamics but also regulates community structure (Archer, 1989; Oliver et al., 2010; Yang et al., 2015). In our study, Salix etosia, Rubus setchuenensis, and Hydrangea aspera, three typical shrubs with different life forms in southern China, were selected to demonstrate their adjustments in morphological traits and biomass allocation patterns to changed habitats (outside the forest versus inside the forest). Various studies suggest that species from more heterogeneous and changing environments have better plasticity (Archer, 1989). The differences in plasticity and its mechanisms allow us to improve our understanding of why plant species grow where they do. Besides, it also enables the projection of their most likely range in climate change scenarios (Salisbury and Ross, 1991; Walther et al., 2002; de Mazancourt et al., 2008). Photosynthetic responses of sciophytes, shade-loving plants, are naturally easily saturated at lower irradiance than heliophytes and sun plants (Salisbury and Ross, 1991). Phenotypic plasticity is a crucial pathway for plants to adapt to the heterogeneous environments. It is essential to investigate the adjustments of plant morphological traits and biomass allocation patterns, which contribute to understanding of the adaptation of plants to different environmental gradients.
Carbon content indicates carbon sequestration and storage capacity of living plants (Osada et al., 2003). The greater SLA reduces diffusive resistance of chloroplast to CO2, and lowers cost and allocation to investment and maintains leaves. Moreover, exposure area of leaf increased for more light, which favored the plants to survive under weak light environment (Chapin et al., 1987), while reduced self-shading was increased with greater leaf overlap to relieve hurt from intensified light or extreme heat (Wilson, 1988; Malavasi and Malavasi, 2001; de Groot et al., 2002). There was greater N content inside the forests probably due to sufficient substrates (i.e., soil organic matter) and greater mineralization. The habitat experienced warmer temperature outside the forests, but its SWC had no significant differences with habitat inside the forests, which probably resulted from improved transpiration of plants at a community level. For R. setchuenensis and H. aspera, individual leaf area and SLA increased significantly inside the forests than those outside, but no significant difference for those of S. etosia, which was in accordance with specific leaf mass positively correlating with lignin but negatively related to nitrogen content. Shading effects of surrounded trees induced greater LNC of plant leaf inside the forests since LNC refers to N content in protein of all photosynthetic organs closely related with photosynthesis (Yang et al., 2009). Plants can improve their photosynthetic efficiency for greater dry matter production with more N content and allocation in leaf cost for adapting environments inside the forests. Moreover, there existed a lower pH value and a stronger acidity in the soil inside the forests. R. setchuenensis and H. aspera are probably more sensitive to soil available nitrogen content. The LNC of the three shrubs based on descending order was H. aspera, R. setchuenensis, and S. etosia, which showed a consistent sorting with their SLA. In general, there were no significant differences of stoichiometric characteristics inside and outside the forest in our study, which indicated relative stable inherent characteristics of the three species (Figure 3 and Table 3). The differences in N:P ratios can be caused by variances of climate conditions, soil properties, and phytogenic development of plants (Reich et al., 1999; Gusewell, 2004; Townsend et al., 2007). The N:P ratios of the leaves of the three shrubs were 68.33, 47.20, and 55.53 outside the forest, while the NP ratios were 74.18, 66.01, and 50.69 inside the forest, respectively, which were greater than those shrub communities from the grassland in China (He et al., 2008). Only the leaf N:P ratio of H. aspera, the same with their variations of LPC, was higher outside the forest than that inside the forest. It was probably because H. aspera increased P investment to enhance the ability of cell division, since a greater SLA could adapt to a lower light environment better.
Biomass allocation pattern plays an essential role in plant life history (Weiner, 2004) and is associated with metabolism, CO2 assimilation, and carbon sequestration. A specific pattern of plant biomass allocation reflects diversified ecological adaptation strategies (Enquist and Niklas, 2002; Weiner, 2004; Niinemets, 2006; Roa-Fuentes et al., 2012). H. aspera had greater new branch biomass allocation and aging branch biomass allocation inside the forests for its bunch type in this typical life form, while there were no significant differences of that for S. etosia and R. setchuenensis. In addition, root biomass allocation of R. setchuenensis increased significantly inside the forests, while neither S. etosia and H. aspera had significant differences between the two heterogeneous environments. In accordance with the results of de Groot et al., more photosynthesis production was allocated to stem biomass and SLA while there was nearly constant or even less leaf biomass (Malavasi and Malavasi, 2001). H. aspera adapted better to environment inside the forests than S. etosia and R. setchuenensis by investing greater biomass to sustain itself and support structure (i.e., branch) to enhance competitive ability with shading or surrounding other plants (Roa-Fuentes et al., 2012). H. aspera has stronger tolerance to shading and better phenotypic plasticity with self-regulation of biomass partitioning. Such instances of plasticity have been commonly observed in perennial plants due to aboveground resource limitation.
Aboveground biomass and belowground biomass allocation has been extensively studied for the critical role of root-to-shoot (R/S) ratio in carbon cycle models (Enquist and Niklas, 2002). The variance of R/S reflects the strategy of plants in response to the environment. Given that plant biomass allocation is largely genetically regulated for certain species, environmental factors can still flexibly shift investments toward needed structures (Patty et al., 2010). A higher logarithm of root-to-shoot ratio (R/S) of H. aspera was observed inside the forests, but not for S. etosia and R. setchuenensis. Moreover, R. setchuenensis and H. aspera had isometric relationships between above- and belowground biomass (P > 0.05), but S. etosia had an allometric growth relationship with being prone to allocate more biomass belowground (Figure 5, F = 7.993, P = 0.008). Plasticity index refers to the adaptation ability of plants in heterogeneous environments. Usually, plants allocate more biomass aboveground with limited space and interspecies competition while distributing greater biomass belowground with water and nutrients being deficient (Osada et al., 2003; Bloom and Mallik, 2004; Rozendaal et al., 2006). Inside the forests, plants are prone to invest more resources to aboveground parts to improve photosynthetic capacity with allometric partitioning.
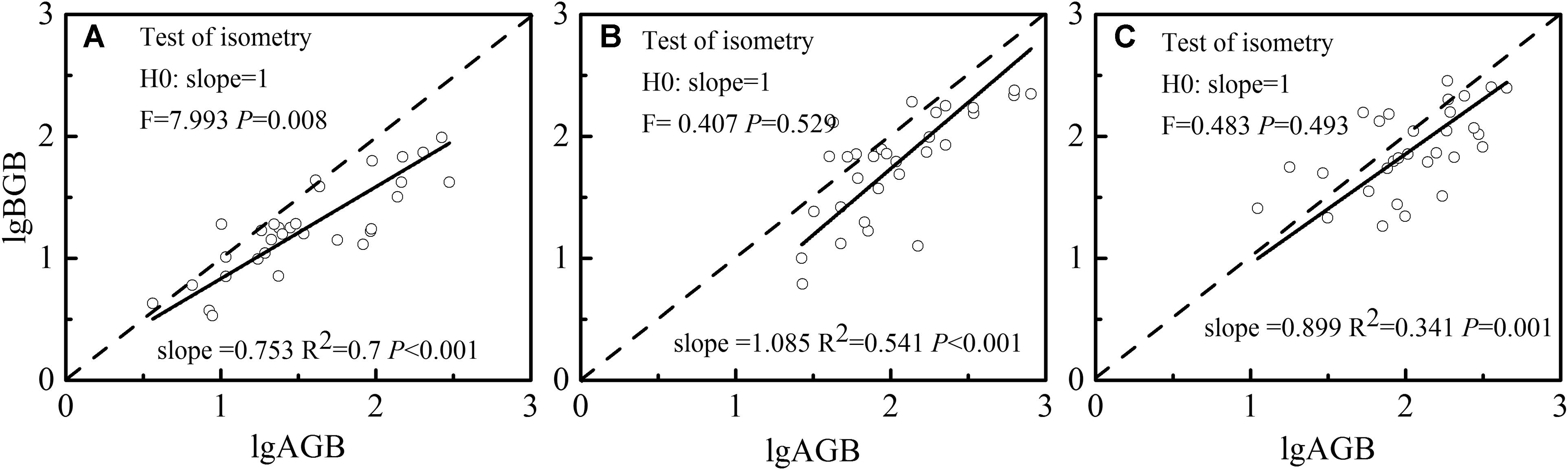
Figure 5. Growth relationship and isometric test between pooled above- and belowground biomass of S. etosia (A), R. setchuenensis (B), and H. aspera (C), respectively.
In general, most studies presenting adaptive plastic responses are usually conducted in simple environments with mainly variation of one or two abiotic factors (Matesanz et al., 2010). A shade-enduring plant has greater phenotypic plasticity and adaptation ability inside the forests. A shade-enduring shrub has lower photosynthesis capacity but with relatively greater individual leaf area and belowground biomass partitioning for understory environment. It has been said that the effects of chloroplasts might be greater than the variation of SLA or leaf thickness (Oguchi et al., 2008). Nevertheless, it has been also acknowledged that there exist a large series of variables going on inside vs. outside the forests, which has been also addressed in this study (light, temperature, N, SOC, and pH). Based on the PCA of abiotic and biotic factors on leaf trait and biomass allocation of the three shrubs, the three principal components were sorted out with cumulative variance proportions of the three shrubs greater than 85%. The correlations of leaf traits or biomass had been tested with relevant components. Morphological and total phenotypic plasticities of the bunch shrub H. aspera and vining shrub R. setchuenensis were significantly greater than those of the erect shrub S. etosia. The former two species performed better whatever deforestation happened for the bunch shrub and vining shrub’s stronger phenotypic plasticity. Because of lower photosynthesis ability, both species mostly adjust the leaves and branches to adapt to external environmental change. However, the erect shrub S. etosia has a relatively weak morphological phenotypic plasticity, which had no significant differences of morphology and biomass allocation with the adaptive root system spatial distribution to variation of environmental conditions. It is also essential to testify whether those plants with better plastic responses can be also adaptive by assessing fitness consequences or in future studies. An extensive approach to the genotypic basis of phenotypic responses may contribute to the grasping of the ecological significance of phenotypic plasticity, which needs to focus on the typical genotypic sample, together with the recognition that any environmentally induced phenotypic change is legitimate plasticity or natural selection’s potential target.
Plants can adapt to various external heterogeneous environments with multiple species-specific strategies (Niinemets, 2006; Cheng and Niklas, 2007; Portsmuth and Niinemets, 2007; Valladares and Niinemets, 2008). Plants addressed the responses of utilization of soil available nutrients resulting from plant organs, species, and ecosystem for up-scaling (Walther et al., 2002; Montgomery, 2004). Also, if we consider ecotypes (heliophyte or ombrophyte), the relationships between different leaf traits can be changed accordingly, and their adaptation strategies are also species specific given that the leaf is a secondary functional structure unit with relative stability. Plants can only benefit from morphological plasticity when it harvests more resources with adaptable variation of morphology. Besides, there always is some wastage along with morphological plasticity given that the adjustment of phenotypic plasticity can obviously enhance photosynthesis and improve self-competitive ability. Plants have plasticity throughout the world, to the new environmental conditions, but it is overlooked that the plants are possibly evolving new plastic responses, which can be a potentially important component of their responding strategies to environmental change. Thus, in the future, it is crucial to understand the mechanisms that underlie plasticity, such as non-genetic factors that determine the growth and development of a plant, and how this impacts the plants’ biological efficacy. Being a self-organized organism, plants perform with not just a single trait for adaptation and response to the external environment but synergistically adapt by coupling with other traits in a changing world.
Data Availability Statement
The raw data supporting the conclusions of this article will be made available by the authors, without undue reservation.
Author Contributions
JW, YW, and NW raised scientific questions and designed the experiments. JW, BX, HZ, and FS conducted the experiments. JW, JG, and BX analyzed the experimental data. JW, JG, YW, NW, and NB wrote the manuscript. All authors discussed the results and approved the final manuscript and contributed substantially to this research work.
Funding
This study was financially supported by the China Postdoctoral Science Foundation (2014M552385), National Natural Science Foundation of China (31400389 and 31971436), International Science and Technology Cooperation Program of China (2013DFR90670), and International Cooperation Project of Science and Technology Department of Sichuan Province (2014HH0056).
Conflict of Interest
The authors declare that the research was conducted in the absence of any commercial or financial relationships that could be construed as a potential conflict of interest.
References
Aerts, R., Decaluwe, H., and Konings, H. (1992). Seasonal allocation of biomass and nitrogen in 4 Carex species from mesotrophic and eutrophic fens as affected by nitrogen supply. J. Ecol. 80, 653–664. doi: 10.2307/2260857
Aguilar, R., Ashworth, L., Galetto, L., and Aizen, M. A. (2006). Plant reproductive susceptibilityto habitat fragmentation: review and synthesis through ameta-analysis. Ecol. Lett. 9, 968–980. doi: 10.1111/j.1461-0248.2006.00927.x
Archer, S. (1989). Have southern texas savannas been converted to woodlands in recent history. Am.Nat. 134, 545–561. doi: 10.1086/284996
Bloom, R. G., and Mallik, A. U. (2004). Indirect effects of black spruce (Picea mariana) cover on community structure and function in sheep laurel (Kalmia angustifolia) dominated heath of eastern Canada. Plant Soil 265, 279–293. doi: 10.1007/s11104-005-0508-4
Cairns, M. A., Brown, S., Helmer, E. H., and Baumgardner, G. A. (1997). Root biomass allocation in the world’s upland forests. Oecologia 111, 1–11. doi: 10.1007/s004420050201
Chapin, F. S., Bloom, A. J., Field, C. B., and Waring, R. H. (1987). Plant-responses to multiple environmental-factors. Bioscience 37, 49–57. doi: 10.2307/1310177
Chen, H. Y. H., Klinka, K., and Kayahara, G. J. (1996). Effects of light on growth, crown architecture, and specific leaf area for naturally established Pinus contorta var. latifolia and Pseudotsuga menziesii var. glauca saplings. Can.J. Forest Res. 26, 1149–1157. doi: 10.1139/x26-128
Cheng, D. L., and Niklas, K. J. (2007). Above- and below-ground biomass relationships across 1534 forested communities. Ann. Bot. 99, 95–102. doi: 10.1093/aob/mcl206
Cornelissen, J. H. C., Lavorel, S., Garnier, E., Díaz, S., Buchmann, N., Gurvich, D. E., et al. (2003). A handbook of protocols for standardised and easy measurement of plant functional traits worldwide. Aust. J. Bot. 51, 335–380. doi: 10.1071/BT02124
Costa, L. G. S., Miranda, I. S., Grimaldi, M., Silva, M. L., Mitja, D., and Lima, T. T. S. (2012). Biomass in different types of land use in the Brazil’s ‘arc of deforestation’. Forest. Ecol. Manag. 278, 101–109. doi: 10.1016/j.foreco.2012.04.007
de Groot, C. C., Marcelis, L. F. M., van den Boogaard, R., and Lambers, H. (2002). Interactive effects of nitrogen and irradiance on growth and partitioning of dry mass and nitrogen in young tomato plants. Funct. Plant Biol. 29, 1319–1328. doi: 10.1071/FP02087
de Mazancourt, C., Johnson, E., and Barraclough, T. G. (2008). Biodiversity inhibits species’ evolutionary responses to changing environments. Ecol. Lett. 11, 380–388. doi: 10.1111/j.1461-0248.2008.01152.x
Eilts, J. A., Mittelbach, G. G., Reynolds, H. L., and Gross, K. L. (2011). Resource heterogeneity, soil fertility, and species diversity: effects of clonal species on plant communities. Am.Nat. 177, 574–588. doi: 10.1086/659633
Enquist, B. J., and Niklas, K. J. (2002). Global allocation rules for patterns of biomass partitioning in seed plants. Science 295, 1517–1520. doi: 10.1126/science.1066360
Grassi, G., and Bagnaresi, U. (2001). Foliar morphological and physiological plasticity in Picea abies and Abies alba saplings along a natural light gradient. TreePhysiol. 21, 959–967. doi: 10.1093/treephys/21.12-13.959
Grime, J. P. (1994). “The role of plasticity in exploiting environmental heterogeneity,” in Exploitation of Environmental Heterogeneity by Plants: Ecophysiological Processes Above- and Belowground, eds J. Roy, M. M. Caldwell, and R. P. Pearce (London: Academic Press). doi: 10.1016/B978-0-12-155070-7.50006-8
Gusewell, S. N. (2004). P ratios in terrestrial plants: variation and functional significance. New Phytol. 164, 243–266. doi: 10.1111/j.1469-8137.2004.01192.x
He, J. S., Wang, L., Flynn, D. F. B., Wang, X. P., Ma, W. H., and Fang, J. Y. (2008). Leaf nitrogen: phosphorus stoichiometry across chinese grassland biomes. Oecologia 155, 301–310. doi: 10.1007/s00442-007-0912-y
Houghton, R. A., Lawrence, K. L., Hackler, J. L., and Brown, S. (2001). The spatial distribution of forest biomass in the Brazilian Amazon: a comparison of estimates. Global Change Biol. 7, 731–746. doi: 10.1046/j.1365-2486.2001.00426.x
Hutchings, M. J., and John, E. A. (2004). The effects of environmental heterogeneity on root growth and root/shoot partitioning. Ann. Bot. 94, 1–8. doi: 10.1093/aob/mch111
King, D. A. (2003). Allocation of above-ground growth is related to light in temperate deciduous saplings. Funct. Ecol 17, 482–488. doi: 10.1046/j.1365-2435.2003.00759.x
Körner, C., and Renhardt, U. (1987). Dry-matter partitioning and root length leaf-area ratios in herbaceous perennial plants with diverse altitudinal distribution. Oecologia 74, 411–418. doi: 10.1007/BF00378938
Larigauderie, A., Ellis, B. A., Mills, J. N., and Kummerow, J. (1991). The effect of root and shoot temperatures on growth of Ceanothus-greggii seedlings. Ann. Bot. 67, 97–101. doi: 10.1093/oxfordjournals.aob.a088121
Lawrence, D., and Vandecar, K. (2015). Effects of tropical deforestation on climate and agriculture Nat. Clim. Chang. 5, 27–36. doi: 10.1038/nclimate2430
Malanson, G. P., Wang, Q., and Kupfer, J. A. (2007). Ecological processes and spatial patterns before, during and after simulated deforestation. Ecol. Model 202, 397–409. doi: 10.1016/j.ecolmodel.2006.11.012
Malavasi, U. C., and Malavasi, M. M. (2001). Leaf characteristics and chlorophyll concentration of schyzolobium parahybum and hymenaea stilbocarpa seedlings grown in different light regimes. Tree Physiol. 21, 701–703. doi: 10.1093/treephys/21.10.701
Matesanz, S., Gianoli, E., and Valladares, F. (2010). Global change and the evolution of phenotypic plasticity in plants. Ann. N. Y. Acad. Sci. 1206, 35–55. doi: 10.1111/j.1749-6632.2010.05704.x
Montgomery, R. (2004). Relative importance of photosynthetic physiology and biomass allocation for tree seedling growth across a broad light gradient. Tree Physiol. 24, 155–167. doi: 10.1093/treephys/24.2.155
Niinemets, U. (2006). The controversy over traits conferring shade-tolerance in trees: ontogenetic changes revisited. J. Ecol. 94, 464–470. doi: 10.1111/j.1365-2745.2006.01093.x
Oguchi, R., Hikosaka, K., Hiura, T., and Hirose, T. (2008). Costs and benefits of photosynthetic light acclimation by tree seedlings in response to gap formation. Oecologia 155, 665–675. doi: 10.1007/s00442-007-0951-4
Oliver, T., Roy, D. B., Hill, J. K., Brereton, T., and Thomas, C. D. (2010). Heterogeneous landscapes promote population stability. Ecol. Lett. 13, 473–484. doi: 10.1111/j.1461-0248.2010.01441.x
Osada, N., Takeda, H., Kitajima, K., and Pearcy, R. W. (2003). Functional correlates of leaf demographic response to gap release in saplings of a shade-tolerant tree, Elateriospermum tapos. Oecologia 137, 181–187. doi: 10.1007/s00442-003-1335-z
Patty, L., Halloy, S. R. P., Hiltbrunner, E., and Korner, C. (2010). Biomass allocation in herbaceous plants under grazing impact in the high semi-arid Andes. Flora 205, 695–703. doi: 10.1016/j.flora.2009.12.039
Portsmuth, A., and Niinemets, U. (2007). Structural and physiological plasticity in response to light and nutrients in five temperate deciduous woody species of contrasting shade tolerance. Funct. Ecol. 21, 61–77. doi: 10.1111/j.1365-2435.2006.01208.x
Ramankutty, N., and Foley, J. A. (1999). Estimating historical changes in global land cover: croplands from 1700 to 1992. Global Biogeochem. Cycle 13, 997–1027. doi: 10.1029/1999GB900046
Reich, P. B., Ellsworth, D. S., Walters, M. B., Vose, J. M., Gresham, C., Volin, J. C., et al. (1999). Generality of leaf trait relationships: a test across six biomes. Ecology 80, 1955–1969. doi: 10.1890/0012-9658(1999)080[1955:GOLTRA]2.0.CO;2
Reich, P. B., Uhl, C., Walters, M. B., and Ellsworth, D. S. (1991). Leaf life-span as a determinant of leaf structure and function among 23 Amazonian tree species. Oecologia 86, 16–24. doi: 10.1007/BF00317383
Reich, P. B., Walters, M. B., and Ellsworth, D. S. (1992). Leaf life-span in relation to leaf, plant, and stand characteristics among diverse ecosystems. Ecol. Monogr. 62, 365–392. doi: 10.2307/2937116
Roa-Fuentes, L. L., Campo, J., and Parra-Tabla, V. (2012). Plant biomass allocation across a precipitation gradient: an approach to seasonally dry tropical forest at Yucatan, mexico. Ecosystems 15, 1234–1244. doi: 10.1007/s10021-012-9578-3
Rozendaal, D. M. A., Hurtado, V. H., and Poorter, L. (2006). Plasticity in leaf traits of 38 tropical tree species in response to light; relationships with light demand and adult stature. Funct. Ecol. 20, 207–216. doi: 10.1111/j.1365-2435.2006.01105.x
Rw, S., and Jj, E. (2002). Ecological Stoichiometry: The Biology of Elements from Molecules to the Biosphere. Princeton, NJ: Princeton University Press.
Sih, A. (2004). “A behavioral ecological view of phenotypic plasticity,” in Phenotypic Plasticity: Functional and Conceptual Approaches, eds T. J. DeWitt and S. M. Scheiner (Oxford: Oxford University Press), 112–125.
Stewart, A. J. A., John, E. A., and Hutchings, M. J. (2000). “The ecological consequences of environmental heterogeneity.the 40th symposium of the british ecological society the University of Sussex,” in The World is Heterogeneous: Ecological Consequences of Living in a Patchy Environment, eds M. J. Hutchings, E. A. John, and A. J. A. Stewart (Hoboken, NJ: Blackwell).
Tilman, D. (1982). Resource Competition and Community Structure. Princeton, NJ: Princeton University Press. doi: 10.1515/9780691209654
Townsend, A. R., Cleveland, C. C., Asner, G. P., and Bustamante, M. M. C. (2007). Controls over foliar N: P ratios in tropical rain forests. Ecology 88, 107–118. doi: 10.1890/0012-9658(2007)88[107:COFNRI]2.0.CO;2
Valladares, F., and Niinemets, U. (2008). Shade tolerance, a key plant feature of complex nature and consequences. Ann. Rev. Ecol. Evol. Systemat. 39, 237–257. doi: 10.1146/annurev.ecolsys.39.110707.173506
Valladares, F., Wright, S. J., Lasso, E., Kitajima, K., and Pearcy, R. W. (2000). Plastic phenotypic response to light of 16 congeneric shrubs from a Panamanian rainforest. Ecology 81, 1925–1936. doi: 10.1890/0012-9658(2000)081[1925:PPRTLO]2.0.CO;2
van Doorn, A. M., and Bakker, M. M. (2007). The destination of arable land in a marginal agricultural landscape in south Portugal: an exploration of land use change determinants. Landscape Ecol. 22, 1073–1087. doi: 10.1007/s10980-007-9093-7
Vile, D., Garnier, E., Shipley, B., Laurent, G., Navas, M. L., Roumet, C., et al. (2005). Specific leaf area and dry matter content estimate thickness in laminar leaves. Ann. Bot. 96, 1129–1136. doi: 10.1093/aob/mci264
Walther, G. R., Post, E., Convey, P., Menzel, A., Parmesan, C., Beebee, T. J. C., et al. (2002). Ecological responses to recent climate change. Nature 416, 389–395. doi: 10.1038/416389a
Weiner, J. (2004). Allocation, plasticity and allometry in plants. Perspect. Plant Ecol. Evol. Syst. 6, 207–215. doi: 10.1078/1433-8319-00083
Wilson, J. B. (1988). A review of evidence on the control of shoot-root ratio, in relation to models. Ann. Bot. 61, 433–449. doi: 10.1093/oxfordjournals.aob.a087575
Yang, Y. H., Fang, J. Y., Ji, C. J., and Han, W. X. (2009). Above- and belowground biomass allocation in Tibetan grasslands. J. Veg. Sci. 20, 177–184. doi: 10.1111/j.1654-1103.2009.05566.x
Yang, Y. H., Fang, J. Y., Ma, W. H., Guo, D. L., and Mohammat, A. (2010). Large-scale pattern of biomass partitioning across china’s grasslands. Global Ecol. Biogeogr. 19, 268–277. doi: 10.1111/j.1466-8238.2009.00502.x
Keywords: leaf traits, ecological stoichiometry, biomass partitioning, phenotypic plasticity, heterogeneous habits, life forms
Citation: Wang J, Gao J, Wu Y, Xu B, Shi F, Zhou H, Bisht N and Wu N (2021) Effects of Heterogeneous Environment After Deforestation on Plant Phenotypic Plasticity of Three Shrubs Based on Leaf Traits and Biomass Allocation. Front. Ecol. Evol. 9:608663. doi: 10.3389/fevo.2021.608663
Received: 21 September 2020; Accepted: 16 March 2021;
Published: 27 April 2021.
Edited by:
Bayartungalag Batsaikhan, Mongolian Academy of Sciences (MAS), MongoliaReviewed by:
Zhanhuan Shang, Lanzhou University, ChinaLin Zhang, Institute of Tibetan Plateau Research (CAS), China
Copyright © 2021 Wang, Gao, Wu, Xu, Shi, Zhou, Bisht and Wu. This is an open-access article distributed under the terms of the Creative Commons Attribution License (CC BY). The use, distribution or reproduction in other forums is permitted, provided the original author(s) and the copyright owner(s) are credited and that the original publication in this journal is cited, in accordance with accepted academic practice. No use, distribution or reproduction is permitted which does not comply with these terms.
*Correspondence: Yan Wu, d3V5YW5AY2liLmFjLmNu; Ning Wu, d3VuaW5nQGNpYi5hYy5jbg==