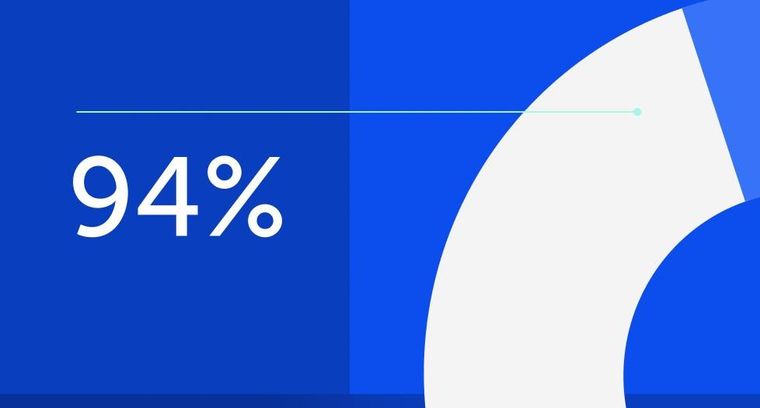
94% of researchers rate our articles as excellent or good
Learn more about the work of our research integrity team to safeguard the quality of each article we publish.
Find out more
ORIGINAL RESEARCH article
Front. Ecol. Evol., 01 March 2021
Sec. Behavioral and Evolutionary Ecology
Volume 9 - 2021 | https://doi.org/10.3389/fevo.2021.607802
This article is part of the Research TopicEcology, Evolution, and Behavior of Viviparous FishesView all 19 articles
Predation is known to have a significant effect on life history diversification in a variety of species. However, physical constraints of body shape and size can sometimes limit life history divergence. We test this idea in the Costa Rican livebearing fish Alfaro cultratus. Individuals in this species have a narrow body and keeled ventral surface, and females do not develop a distended abdomen when pregnant like other livebearing fishes. Here, we describe the life history of A. cultratus from 20 different populations across both high-predation and low-predation environments. We found significantly lower reproductive allotment in females from high-predation environments than in females from low-predation environments, but no significant difference in female or male size at maturity, number of offspring produced by females, or size of offspring. We found that A. cultratus exhibit isometric patterns of allocation for clutch dry mass in relation to female dry mass in high-predation and low-predation environments. Our results suggest that body shape constraints in this species limit the life history divergence we typically see between populations from high-predation and low-predation environments in other species.
A life history strategy defines how an organism utilizes and optimizes energy to survive and reproduce (Fisher, 1930; Williams, 1966; Stearns, 1977; Roff, 1992). The optimal strategy can be influenced by extrinsic factors such as mortality rate (Strauss, 1990; Jennions et al., 2006; Riesch et al., 2013; Mukherjee et al., 2014; Olinger et al., 2016), resource availability (Reznick et al., 1992; Riesch et al., 2013; Moore et al., 2016; Zandonà et al., 2017), population density (Bronikowski et al., 2002; Schrader and Travis, 2012), and environmental conditions (e.g., salinity, gradient, elevation, etc.) (Zúñiga-Vega et al., 2007; Jourdan et al., 2016; Rius et al., 2019). Predator environments have often been used to study the effects of mortality rate on life history strategies (Law, 1979; Reznick and Endler, 1982; Johnson and Belk, 1999; Gosline and Rodd, 2008) and have been found to affect a wide variety of taxa, including fish, anurans, and insects. Among other things, the presence of a predator can influence timing and size of maturation and changes among developmental stages (Chivers et al., 2001; Johnson, 2001; Hilton et al., 2002; Stoks et al., 2006; Peterson et al., 2019), growth rate (Lardner, 2000; Altwegg, 2002; Šupina et al., 2016; Brown et al., 2018; DeWitt et al., 2019), and investment in offspring (Johnson and Belk, 2001; Gorini-Pacheco et al., 2017). Previous work consistently finds divergent life history patterns in high-predation and low-predation environments. For example, in the family Poeciliidae (livebearing fishes) many studies have shown a divergent pattern of smaller size at maturity, higher fecundity, smaller offspring, and greater reproductive allotment in populations in high-predation environments relative to low-predation or no-predator environments (Reznick, 1990; Johnson, 2001; Jennions and Telford, 2002; Walsh and Reznick, 2009; Moore et al., 2016). Similarly, in anurans the timing and size of metamorphosis (Laurila et al., 1998; Lardner, 2000) and timing of hatching (Laurila et al., 2002; Capellán and Nicieza, 2007) change in response to the presence of a predator. Therefore, we expect to see patterns of life history divergence in response to predator environments in additional species.
There are limits, however, to divergent evolution in predator environments. Divergent evolution requires that populations are able to adapt to different selective pressures. That said, there are genetic, phylogenetic, morphological, and physiological constraints that can all limit adaptive evolution (Gould, 1980). Morphological constraints are particularly important in life history evolution as they can affect the internal body space available for reproduction. This has been frequently studied in turtles (Clark et al., 2001; Ryan and Lindeman, 2007; Rollinson and Brooks, 2008; Macip-Ríos et al., 2012). For example, the small African tortoise Homopus signatus, produces single-egg clutches. Although producing one large egg is best for the fitness and survival of the offspring, H. signatus is constrained by a small body size and pelvic canal limiting how large the egg can be (Hofmeyr et al., 2005). Similar patterns were found in other species of turtles where the pelvic girdle (also influenced by evolutionary pressures on locomotion) limited egg size, especially in small individuals (Congdon and Gibbons, 1987). Thus, morphology can constrain a life history trait due to internal space, size, and shape of an organism.
Morphology is also important in survival; tradeoffs between the optimal morphology for survival and the optimal morphology for reproduction may be present in some species. Size and shape can be very important in predator avoidance. For example, the humpback chub, Gila cypha, has a large dorsal cranial hump that increases the depth of their body and therefore protects against gape limited predators (Portz and Tyus, 2004). Tradeoffs occur because certain morphologies may be optimal to some selective pressures, but not to others. In the family Poeciliidae, fishes invest more in offspring when predators are present than when they are absent; however, this investment comes at a cost of decreased swimming performance (Ghalambor et al., 2004). Thus the optimal morphology for swimming performance and the optimal morphology for reproduction can be in conflict (Zúñiga-Vega et al., 2007; Wesner et al., 2011; Hassell et al., 2012; Ingley et al., 2016; Quicazan-Rubio et al., 2019). Selective pressures acting on morphology can limit the optimal adaptation in life history or vice versa in a given environment. However, we don’t know how morphological adaptations limit life history adaptations in predation environments.
An additional question is how predation and morphological constraints influence lifetime reproductive allocation. The terminal investment hypothesis predicts that organisms will invest more in reproduction as they age, as chances for future reproduction decrease (Williams, 1966). Specifically, in environments that experience high mortality (such as high-predation environments) individuals may allocate energy to current reproduction over future reproduction; however, in low mortality environments (such as low-predation environments) individuals may allocate more to future reproduction than current reproduction, consistent with the terminal investment hypothesis (Law, 1979; Michod, 1979; Billing et al., 2007; Belk et al., 2011; Billman et al., 2014; Nickley et al., 2016). Thus, reproductive allocation can change in response to mortality pressures presented in predation environments as high mortality limits the chance of survival and opportunities for future reproduction. Morphological constraints can also influence within-lifetime reproductive allocation. In Brachyrhaphis parismina (a poeciliid fish), populations showed isometric allocation of reproductive allotment to female body mass with age (Belk et al., 2011). This is possibly due to a narrow-bodied shape that might constrain reproductive allocation from being greater than proportionate to body size. Thus, mortality rates and morphological constraints can influence patterns of reproductive investment.
In this study, we test the ideas that: 1) divergence in life history traits among populations in different predator environments may be limited when there are strong morphological constraints; and 2) within lifetime reproductive allocation, consistent with the terminal investment hypothesis, may be limited within predation environments due to morphological constraints. If true, we expect to find isometric allocation rather than hyper-allometric allocation in morphologically constrained species. To test these ideas, we used the fish Alfaro cultratus (Regan, 1908) from the family Poeciliidae. Poeciliids provide an optimal study system as they are livebearers, have a short generation time, and are found in many different selective environments (Reznick and Endler, 1982). Alfaro cultratus is an ideal species for our study as it is an extremely narrow-bodied poeciliid with a keeled ventral surface (Figure 1). Additionally, A. cultratus do not develop a distended abdomen during pregnancy. The body morphology of this fish is likely a constraint for reproduction as it does not allow additional space via abdominal expansion during pregnancy as exemplified in other poeciliids.
Figure 1. Photos of the lateral and dorsal view of a female Alfaro cultratus. Dorsal view shows laterally compressed body shape.
We collected fish from eight different sites in Costa Rica during February and May 2006, and May 2007. Additionally, we collected A. cultratus from 12 different sites in northeast Costa Rica during April 2019 (Figure 2). We collected fish under Brigham Young University IACUC committee approval (Protocol #15-0404). All fish were collected with permission and corresponding permits from the Sistema Nacional De Áreas De Conservación in Costa Rica (011-2006-SINAC, 015-2007-SINAC, R-SINAC-PNI-ACAHN-011-2019). We collected samples with a handheld seine (1.3 × 5 m; 8 mm mesh size). We tried to collect approximately 100 females (Table 1) from each site to ensure that we had enough mature and immature individuals for analysis without taking more than a fraction of the local population. We euthanized all fish in the field with an overdose of 3- amenobenzoic acid ethyl ester (MS-222), preserved samples in the field in 95% ethanol, and then transported them to the laboratory for analysis where they were stored in 70% ethanol.
Figure 2. Map of Costa Rica collection locations. Localities are numbered according to the site number found in Table 1. High-predation sites are triangles; low-predation sites are circles.
Table 1. Descriptive statistics for life history characteristics of Alfaro cultratus for 20 populations.
We identified high-predation sites as locations where the piscivorous species Parachromis dovii (Johnson and Belk, 2001) and/or Parachromis managuensis were found during seining. At each location we made multiple seine hauls (10 or more). Low-predation sites were identified as locations where A. cultratus was found alone or only with non-piscivorous fishes. Here, we analyze 11 high-predation sites (one from 2006, three from 2007, and seven from 2019) and nine low-predation sites (two from 2006, two from 2007, and five from 2019). We term these sites as “high predation environments” or “low-predation environments,” respectively. High-predation and low-predation environments are expected to vary in predation risk but also may be confounded with other environmental factors such as resource availability, temperature, elevation, flow, and density (Johnson, 2002; Jourdan et al., 2016; Olinger et al., 2016). Thus, predation environments are characterized by the presence or absence of a predator, but they are called “environments” to encompass the many different factors that may be causally or incidentally correlated with the presence or absence of a predator (see Johnson, 2002). In addition to piscivorous predators, other factors can contribute to morality rates, including avian and invertebrate predators. Previous work on the fish Brachyrhaphis rhabdophora suggests that categorizing locations this way into high and low-predation environments does accurately predict mortality rates and divergent life history traits (Johnson and Belk, 2001; Johnson, 2002; Johnson and Zúñiga-Vega, 2009). Therefore, although we have not measured mortality rates in this system, we use the presence/absence of a predator as a predictor of mortality (Johnson, 2001; Johnson and Belk, 2001; Belk et al., 2011; Wesner et al., 2011).
We measured five life history traits: 1) male size at maturity; 2) female size at maturity; 3) number of offspring; 4) size of offspring; and 5) reproductive allotment. All traits were measured from alcohol-preserved specimens. We recognize that this preservation technique results in the extraction of fats from the specimens, an approach that has been applied widely across life history studies, including in our previous work (Johnson and Belk, 2001; Belk et al., 2011; Brown et al., 2018; Molina-Moctezuma et al., 2020), thus allowing us to compare findings here with previous work. We collected life history data using methods described in Johnson and Belk (2001). In brief, we did this as follows. We first measured the length of each adult female fish. We then dissected each specimen on the left lateral side where we removed stomachs and embryos. We counted and staged each embryo. To score female size at maturity for each population, we first divided females into 2 mm size classes. We identified size at maturity as the size class where at least half of the females were mature with developing embryos. Developing embryos were classified using Haynes (1995) classification method (stages 1–11). Stage 1 and 2 are immature and unfertilized eggs, and stage 3 and above are developing embryos. Stage 3 is a fully yolked and fertilized egg, and stage 11 is a mature embryo with the yolk sac entirely, or almost entirely, absorbed (Haynes, 1995). In cases where population samples of mature females were small, the actual value may be slightly smaller or larger than reported because we lacked adequate sampling. We counted number of offspring as the number of developing embryos contained in each mature female. We determined size of offspring as the dry mass of the brood divided by the number of offspring in each brood. We measured reproductive allotment as the dry mass of the brood. Female dry mass (digestive tract removed) and brood dry mass were measured after they were separated and dried for 24 h in a 55°C desiccating oven. We determined male size at maturity as the mean standard length of all mature males (male poeciliids grow little, if at all, after maturation) (Turner, 1942; Johnson and Belk, 2001; Belk et al., 2011). We identified mature males by the presence of a fully developed modified anal fin (gonopodium).
We built two models of reproductive allotment as the relationship between the natural log of clutch dry mass and the natural log of female dry mass in both high-predation and low-predation environments. We used the slopes of these models as allometric coefficients (Table 2). We included developmental stage of offspring as a covariate and collection location as a random effect in the models. We determined patterns of allometry using ordinary least squares regression (Kilmer and Rodríguez, 2017). When the slope was equal to one, this indicated isometry and not terminal investment. A slope greater than one is consistent with terminal investment (Billman et al., 2014), where the mass of the clutch is proportionately larger than predicted by body size. Females exhibit indeterminate growth; thus, we used size of females as a surrogate of age. All analyses were done using R version 3.5.2 (R Project for Statistical Computing, RRID:SCR_001905).
Table 2. Allometric coefficients for Alfaro cultratus in high-predation and low-predation environments.
We ran general linear models for each life history trait to assess the effect of predation. We included covariates for the life history models as described in Johnson and Belk (2001). In brief, when analyzing number of offspring, we included female dry mass as a covariate. When analyzing offspring size and reproductive allotment, we used female dry mass and developmental stage of embryos as covariates. We did not include any covariates for male or female size at maturity. Brood dry mass was our measure of reproductive allotment. We log transformed reproductive allotment and number of offspring in the analysis to satisfy assumptions of the linear model. All output data for reproductive allotment and number of offspring were back-transformed to the original scale before being included in graphs or tables. We included location in each model as a random effect. We calculated population least squares means for reproductive allotment, number of offspring, and size of offspring for comparable estimates (Table 1). Additionally, we ran the analysis for reproductive allotment with and without the population from Quebrada Serena (a possible outlier) to determine the significance of this population. We found that with the removal of this site, predation no longer significantly affected reproductive allotment. All analyses were done using R version 3.5.2 (R Project for Statistical Computing, RRID:SCR_001905).
Life history traits in A. cultratus did not differ significantly between high-predation versus low-predation environments except reproductive allotment (Table 3). Females from high-predation environments had significantly lower values of reproductive allotment than those from low-predation environments (ANCOVA, F = 5.7, df = 1, P = 0.017, slope = −0.15, R2 = 0.46). The statistical significance of this relationship is entirely due to one population with high brood dry mass in the low-predation category (Quebrada Serena) (see S1, Supplementary Material). Size of offspring, number of offspring, and size at maturity for males and females did not differ significantly in high-predation versus low-predation environments (Table 3 and Figures 3, 4).
Figure 3. Plot of population least squares means for reproductive allotment (brood dry mass), number of offspring, and size of offspring in high-predation and low-predation environments. Population means (dots) are jittered for better visualization.
Figure 4. Plot of size at maturity for males and females in high-predation and low-predation environments. Female size at maturity is the size where at least 1/2 females in that size category contain developing embryos. For males this is the mean size of mature males. Population points (dots) are jittered for better visualization.
Similarly, the allometric coefficients for reproductive allotment did not differ between high-predation and low-predation environments. Individuals in both environments displayed isometric reproductive allocation with age, inconsistent with the terminal investment hypothesis (Table 2 and Figure 5).
Figure 5. Graph of allometric coefficients for high-predation and low-predation environments. Low-predation sites are open circles and high-predation sites are closed circles. The low-predation allometric coefficient (1.052) is the dashed line and the high-predation allometric coefficient (1.083) is the solid line.
There was no divergence in four life history traits or allometric coefficients for reproductive allotment in A. cultratus from different predation environments. All life history traits showed no significant difference between high-predation and low-predation environments, except for reproductive allotment, which did differ significantly. However, it differed in a direction opposite to what theory predicts (Reznick, 1990) – we found lower allotment in high-predation environments than in low-predation environments. This significant result and allotment pattern are driven by our collection from Quebrada Serena (site 3). With the removal of this site, the difference in reproductive allotment is no longer significant. This site appears to be unique in that all mature females had a large number of offspring (greater than 8). However, it does not appear to be unique in any other way. Thus, it is possible that the life history phenotype observed at this site is shaped by other selective pressures. One possible explanation is resource availability. High resource availability has been found to influence a high fecundity (Reznick and Yang, 1993) and with the high fecundity found at this site this may be a likely explanation. The allometric coefficient for reproductive allotment also did not differ among predation environments but instead showed an isometric pattern of allocation in both environments. This isometric pattern of investment is not consistent with the terminal investment hypothesis. Thus terminal investment is not evident in this species.
Lack of intraspecific life history variation is not unique to Alfaro cultratus. Absence of significant life history differences between populations is also seen in the species Brachyrhaphis parismina (Belk et al., 2011). However, differing predation pressures often evoke a divergent pattern of life history variation as is seen in Brachyrhaphis rhabdophora, Brachyrhaphis episcopi, and Poecilia reticulata (Reznick and Endler, 1982; Johnson and Belk, 2001; Jennions and Telford, 2002). The almost complete lack of intraspecific life history divergence across predation environments in Alfaro cultratus is unexpected and requires further exploration.
There are several possible explanations for the lack of divergence in life history in A. cultratus. It is possible that there may not be differences in environmental selective pressures among the sites. In environments where multiple factors are highly correlated, using one factor such as predator presence, is sufficient in representing a suite of putative selective agents at sites (Johnson, 2002). If environmental factors are not highly correlated, then using one factor such as predation may not adequately represent variation among selective environments. It is also possible that our predation environment as categorized here does not accurately predict mortality rates. Variation in actual mortality rates among localities could prove problematic to our simple placement of populations into either high or low mortality groups. This said, such categories have proved effective at predicting mortality rates in other systems (Johnson and Belk, 2001; Johnson and Zúñiga-Vega, 2009; Ingley et al., 2014; Belk et al., 2020). Lack of phenotypic divergence might also be attributed to gene flow between populations that can limit the ability of populations to adapt to selective pressures in their environment and therefore decrease differences between populations (Storfer, 1999). Unfortunately, we currently have no estimates of gene flow for this species – this said, the geographic distribution of high-predation and low-predation populations suggest that this explanation is not likely (Figure 2). Another alternative is that there is limited additive genetic variation. Again, we have no direct measure of additive genetic variation for A. cultratus. None of these explanations were examined in full in this study, but they may be a good direction for future research.
The most obvious explanation for lack of variation in this narrow-bodied species is that morphology acts as a constraint on reproductive traits. Body morphology influences swimming performance and predator avoidance (McPeek et al., 1996; Kolar and Wahl, 1998; Langerhans et al., 2004; Langerhans, 2009; Araújo et al., 2017). Pregnancy, in many species of poeciliids, can drastically change swimming performance causing predator avoidance to decline as pregnancy progresses (Ghalambor et al., 2004; Belk and Tuckfield, 2010). This may be caused by a morphological convergence across species during pregnancy which limits burst swimming near the end of pregnancy as the abdomen becomes distended and reproductive investment is favored over predator escape speed (Ghalambor et al., 2003; Wesner et al., 2011; Ingley et al., 2014). Some species are able to moderate the distension of the abdomen during pregnancy by superfetation, the simultaneous carrying of multiple broods, and thus maintain a more streamlined body morphology during pregnancy (Zúñiga-Vega et al., 2007; Fleuren et al., 2019). Alfaro cultratus does not exhibit superfetation, instead, their narrow body appears to limit abdominal distension during pregnancy. We suggest that the narrow body and distinctive ventral keel are important for swimming ability and that swimming ability may be favored in all environments in this species. Thus, limiting the space available at the end of pregnancy for a distended abdomen and contributing to the lack of difference seen among populations and individuals in life history characteristics.
For Alfaro cultratus, both the ventral keel and the narrow, streamlined body shape likely contribute to stabilized swimming ability. Morphological adaptations in fish are critical to increasing thrust and decreasing drag despite swimming style (e.g., stead or unsteady) (Webb, 1984). Small differences in morphology can have a large effect on locomotion (Webb, 1982), with body shape and fins both playing important roles in swimming performance (Blake, 2004; Langerhans and Reznick, 2010). First, a keeled ventral surface has been shown to be important in swimming performance (George and Westneat, 2019). In scombroid fishes, the presence of a keel on both sides of a caudal peduncle decreases drag and is more efficient than a cylinder or vertically elliptical peduncle (Walters, 1962). A keeled surface increases the surface area that is used for thrust (Graham and Lowell, 1987). Specifically, a ventral keel creates a negative pressure that increases stabilization and resistance to rolling (Van Wassenbergh et al., 2015). The ventral keeled surface of Alfaro cultratus may contribute to swimming performance by increasing stability for steady swimming. Second, a narrow-bodied morphology can likewise be beneficial for steady swimming. The body morphology of a fish influences energy demands by favoring either steady or unsteady swimming (Ohlberger et al., 2006). A streamlined body shape reduces turbulence and energetic costs (Araújo et al., 2017). Thus, the thin, streamlined body shape of Alfaro cultratus likely contributes to steady swimming, allowing for cruising at low energy costs (Figure 1). Both the ventral keel and the streamlined morphology of A. cultratus appear to be adaptations for steady swimming.
Typically, we would not expect to see stabilized swimming or the same morphology in all predation environments. Previous studies have found that in high-predation environments, unsteady swimming is favored but in low-predation environments steady swimming is favored (Langerhans, 2009; Langerhans and Reznick, 2010). Maintaining the same morphology in both high-predation and low-predation environments is thought to be costly as morphological divergence across predator regimes is commonly found in prey fish (Langerhans et al., 2004). The optimal morphology in a high-predation environment must be suboptimal in a low-predation environment or else we would expect to see the same morphology in both (Langerhans et al., 2004). No measure of morphological divergence in predation environments has been assessed in this species. Therefore, divergence may occur across predator regimes despite the persistence of a streamlined morphology. The narrow-bodied morphology may be influenced by other factors such as selective pressures like stream flow and resource acquisition as well as behaviors like habitat preference and foraging habits. For example, little is known about the habitat preference and foraging habits of A. cultratus. However, if foraging occurs in high flows, this may influence a steady swimming morphology despite suboptimal escape maneuvers that are limited in direction because of high flows (Anwar et al., 2016). It is important to acknowledge that body shape and life history may be unassociated. Fish with the same body shape may have varying patterns of life history if the gonads of the fish simply displace other internal organs (Zúñiga-Vega et al., 2011). Further work is needed to directly assess body shape, swimming performance, and selective pressures in this species.
In conclusion, divergent evolution in different predation environments was not seen in Alfaro cultratus. It appears that the ability for divergent evolution to occur in A. cultratus in response to predation pressures may be constrained by a narrow-bodied morphology adapted to stabilized swimming performance. Clearly, additional research focused on the cause of the lack of divergence in life history traits in this species will yield promising results.
The raw data supporting the conclusions of this article will be made available by the authors, without undue reservation.
Ethical review and approval was not required for the animal study because this study was done on preserved specimens.
All authors formulated the idea for the study and conducted fieldwork. JJ and KG oversaw collection of life history data. KG collected 2019 female life history data, performed the analyses, and wrote the first draft of the manuscript. All authors reviewed and edited the manuscript, and approved the final version.
This work was funded by Brigham Young University and was completed in partial fulfillment of a master’s thesis to KG.
The authors declare that the research was conducted in the absence of any commercial or financial relationships that could be construed as a potential conflict of interest.
This work was supported by the Department of Biology and Graduate Studies at Brigham Young University. This was originally published in partial fulfillment of a master’s thesis that can be located through the BYU Scholars Archive (Golden, 2020). We are grateful for the help of Javier Guevara Siquier, Sandra Díaz Alvarado, and others at the Sistema Nacional de Áreas de Conservación (SINAC) who assisted us with collection permits. Tom Quinn and his lab at University of Washington provided lab space for data collection. Kelsie Bonnett and Kaeli Mueller helped collect data on male specimens. Kelsey Beard and Megan Pew helped prepare specimens to be accessioned into the Monte L. Bean Life Science Museum at Brigham Young University. Justin Bagley helped collect samples in 2006 and 2007, and oversaw the collection of life history data from these samples. Blaine Griffen provided feedback on several early versions of the manuscript.
The Supplementary Material for this article can be found online at: https://www.frontiersin.org/articles/10.3389/fevo.2021.607802/full#supplementary-material
Altwegg, R. (2002). Predator-induced life-history plasticity under time constraints in pool frogs. Ecology 83, 2542–2551.
Anwar, S. B., Cathcart, K., Darakananda, K., Gaing, A. N., Shin, S. Y., Vronay, X., et al. (2016). The effects of steady swimming on fish escape performance. J. Comp. Physiol. Neuroethol. Sensory Neural Behav. Physiol. 202, 425–433. doi: 10.1007/s00359-016-1090-1093
Araújo, M. S., Layman, C. A., and Brian Langerhans, R. (2017). Body streamlining is related to higher growth in Bahamian mosquitofish. Evol. Ecol. Res. 18, 383–391.
Belk, M. C., Ingley, S. J., and Johnson, J. B. (2020). Life history divergence in livebearing fishes in response to predation: is there a microevolution to macroevolution barrier? Diversity 12:179. doi: 10.3390/D12050179
Belk, M. C., Nance, E. E., and Johnson, J. B. (2011). Life history of brachyrhaphis parismina: variation within and among populations. Copeia 2011, 372–378. doi: 10.1643/ce-10-116
Belk, M. C., and Tuckfield, R. C. (2010). Changing costs of reproduction: age-based differences in reproductive allocation and escape performance in a livebearing fish. Oikos 119, 163–169.
Billing, A. M., Rosenqvist, G., and Berglund, A. (2007). No terminal investment in pipefish males: only young males exhibit risk-prone courtship behavior. Behav. Ecol. 18, 535–540. doi: 10.1093/beheco/arm007
Billman, E. J., Rasmussen, J. E., Creighton, J. C., Johnson, J. B., and Belk, M. C. (2014). A multivariate approach to the analysis of within lifetime variation in life history. Methods Ecol. Evol. 5, 797–805. doi: 10.1111/2041-210X.12211
Blake, R. W. (2004). Fish functional design and swimming performance. J. Fish Biol. 65, 1193–1222. doi: 10.1111/j.1095-8649.2004.00568.x
Bronikowski, A. M., Clark, M. E., Rodd, F. H., and Reznick, D. N. (2002). Population-dynamic consequences of predator-induced life history variation in the guppy (Poecilia reticulata). Ecology 83, 2194–2204.
Brown, H. N., Gale, B. H., Johnson, J. B., and Belk, M. C. (2018). Testes mass in the livebearing fish Brachyrhaphis rhabdophora (Poeciliidae) varies hypoallometrically with body size but not between predation environments. Ecol. Evol. 8, 11656–11662. doi: 10.1002/ece3.4618
Capellán, E., and Nicieza, A. G. (2007). Trade-offs across life stages: does predator-induced hatching plasticity reduce anuran post-metamorphic performance? Evol. Ecol. 21, 445–458. doi: 10.1007/s10682-006-9133-9139
Chivers, D. P., Kiesecker, J. M., Marco, A., Devito, J., Anderson, M. T., and Blaustein, A. R. (2001). Predator-induced life history changes in amphibians: egg predation induces hatching. Oikos 92, 135–142. doi: 10.1034/j.1600-0706.2001.920116.x
Clark, P. J., Ewert, M. A., and Nelson, C. E. (2001). Physical apertures as constraints on egg size and shape in the common musk turtle. Sternotherus odoratus. Br. Ecol. Soc. 15, 70–77.
Congdon, J. D., and Gibbons, J. W. (1987). Morphological constraint on egg size: a challenge to optimal egg size theory? Proc. Natl. Acad. Sci. U S A. 84, 4145–4147.
DeWitt, P. D., Visscher, D. R., Schuler, M. S., and Thiel, R. P. (2019). Predation risks suppress lifetime fitness in a wild mammal. Oikos 128, 790–797. doi: 10.1111/oik.05935
Fisher, R. A. (1930). The Genetical Theory of Natural Selection. New York, NY: Oxford University Press.
Fleuren, M., Van Leeuwen, J. L., and Pollux, B. J. A. (2019). Superfetation reduces the negative effects of pregnancy on the fast-start escape performance in live-bearing fish. Proc. R. Soc. B Biol. Sci. 286:20192245. doi: 10.1098/rspb.2019.2245
George, A. B., and Westneat, M. W. (2019). Functional morphology of endurance swimming performance and gait transition strategies in balistoid fishes. J. Exp. Biol. 222:jeb194704. doi: 10.1242/jeb.194704
Ghalambor, C. K., Reznick, D. N., and Walker, J. A. (2004). Constraints on adaptive evolution: the functional trade-off between reproduction and fast-start swimming performance in the trinidadian guppy (Poecilia reticulata). Am. Nat. 164, 38–50.
Ghalambor, C. K., Walker, J. A., and Reznick, D. N. (2003). Multi-trait selection. adaptation, and constraints on the evolution of burst swimming performance. Integr. Comp. Biol. 43, 431–438. doi: 10.1093/icb/43.3.431
Golden, K. B. (2020). Predation Environment Does Not Predict Life History in Morphologically-Constrained Fish Alfaro cultratus (Cyprinodontiformes: Poeciliidae). master’s thesis, Brigham Young University: Provo (UT).
Gorini-Pacheco, B., Zandonà, E., and Mazzoni, R. (2017). Predation effects on matrotrophy, superfetation and other life history traits in Phalloceros harpagos. Ecol. Freshw. Fish. 27, 442–452. doi: 10.1111/eff.12359
Gosline, A. K., and Rodd, F. H. (2008). Predator-induced plasticity in guppy (Poecilia reticulata) life history traits. Aquat. Ecol. 42, 693–699. doi: 10.1007/s10452-007-9138-9137
Graham, B. Y. J. B., and Lowell, W. R. (1987). Surface and subsurface swimming of the sea snake pelamis platurus. J. Exp. Biol. 127, 27–44.
Hassell, E. M. A., Meyers, P. J., Billman, E. J., Rasmussen, J. E., and Belk, M. C. (2012). Ontogeny and sex alter the effect of predation on body shape in a livebearing fish: sexual dimorphism, parallelism, and costs of reproduction. Ecol. Evol. 2, 1738–1746. doi: 10.1002/ece3.278
Haynes, J. L. (1995). Standardized classification of poeciliid development for life-history studies. Copeia 1, 147–154.
Hilton, C., Walde, S. J., and Leonard, M. L. (2002). Intense episodic predation by shorebirds may influence life history strategy of an intertidal amphipod. Oikos 99, 368–376.
Hofmeyr, M. D., Henen, B. T., and Loehr, V. J. T. (2005). Overcoming environmental and morphological constraints: egg size and pelvic kinesis in the smallest tortoise. Homopus signatus. Can. J. Zool. 83, 1343–1352. doi: 10.1139/z05-132
Ingley, S. J., Billman, E. J., Belk, M. C., and Johnson, J. B. (2014). Morphological divergence driven by predation environment within and between species of brachyrhaphis fishes. PLoS One 9:e90274. doi: 10.1371/journal.pone.0090274
Ingley, S. J., Camarillo, H., Willis, H., and Johnson, J. B. (2016). Repeated evolution of local adaptation in swimming performance: population-level trade-offs between burst and endurance swimming in Brachyrhaphis freshwater fish. Biol. J. Linn. Soc. 119, 1011–1026. doi: 10.1111/bij.12852
Jennions, M. D., and Telford, S. (2002). Life-history phenotypes in populations of Brachyrhaphis episcopi (Poeciliidae) with different predator communities. Oecologia 132, 44–50. doi: 10.1007/s00442-002-0942-944
Jennions, M. D., Wong, B. B. M., Cowling, A., and Donnelly, C. (2006). Life-history phenotypes in a live-bearing fish Brachyrhaphis episcopi living under different predator regimes: seasonal effects? Environ. Biol. Fishes 76, 211–219. doi: 10.1007/s10641-006-9022-9027
Johnson, J. B. (2001). Adaptive life-history evolution in the livebearing fish Brachyrhaphis rhabdophora: genetic basis for parallel divergence in age and size at maturity and a test of predator-induced plasticity. Evolution (N. Y) 55, 1486–1491.
Johnson, J. B. (2002). Divergent life histories among populations of the fish brachyrhaphis rhabdophora: detecting putative agents of selection by candidate model analysis. Oikos 96, 82–91.
Johnson, J. B., and Belk, M. C. (1999). Effects of predation on life-history evolution in utah chub (Gila atraria). Copeia 1999, 948–957.
Johnson, J. B., and Belk, M. C. (2001). Predation environment predicts divergent life-history phenotypes among populations of the livebearing fish Brachyrhaphis rhabdophora. Oecologia 126, 142–149. doi: 10.1007/s004420000504
Johnson, J. B., and Zúñiga-Vega, J. J. (2009). Differential mortality drives life-history evolution and population dynamics in the fish Brachyrhaphis rhabdophora. Ecology 90, 2243–2252. doi: 10.1890/07-1672.1
Jourdan, J., Krause, S. T., Lazar, V. M., Zimmer, C., Sommer-Trembo, C., Arias-Rodriguez, L., et al. (2016). Shared and unique patterns of phenotypic diversification along a stream gradient in two congeneric species. Sci. Rep. 6:38971. doi: 10.1038/srep38971
Kilmer, J. T., and Rodríguez, R. L. (2017). Ordinary least squares regression is indicated for studies of allometry. J. Evol. Biol. 30, 4–12. doi: 10.1111/jeb.12986
Kolar, C. S., and Wahl, D. H. (1998). Daphnid morphology deters fish predators. Oecologia 116, 556–564. doi: 10.1007/s004420050621
Langerhans, R. B. (2009). Trade-off between steady and unsteady swimming underlies predator-driven divergence in Gambusia affinis. J. Evol. Biol. 22, 1057–1075. doi: 10.1111/j.1420-9101.2009.01716.x
Langerhans, R. B., Layman, C. A., Shokrollahi, A. M., and Dewitt, T. J. (2004). Predator-Driven phenotypic diversification in Gambusia affinis. Evolution (N. Y) 58, 2305–2318. doi: 10.1111/j.0014-3820.2004.tb01605.x
Langerhans, R. B., and Reznick, D. N. (2010). “Ecology and evolution of swimming performance in fishes: predicting evolution with biomechanics,” in Fish Locomotion: an Ecoethological Perspective, eds P. Domenici and B. G. Kapoor (Hauppauge, NY: Science Publishers), 220–248.
Lardner, B. (2000). Morphological and life history responses to predators in larvae of seven anurans. Oikos 88, 169–180. doi: 10.1034/j.1600-0706.2000.880119.x
Laurila, A., Kujasalo, J., and Ranta, E. (1998). Predator-Induced changes in life history in two anuran tadpoles: effects of predator diet. Oikos 83, 307–317.
Laurila, A., Pakkasmaa, S., Crochet, P. A., and Merilä, J. (2002). Predator-induced plasticity in early life history and morphology in two anuran amphibians. Oecologia 132, 524–530. doi: 10.1007/s00442-002-0984-987
Macip-Ríos, R., Brauer-Robleda, P., Casas-Andreu, G., Arias-Cisneros, M., de, L., and Sustaita-Rodríguez, V. H. (2012). Evidence for the morphological constraint hypothesis and optimal offspring size theory in the mexican mud turtle (Kinosternon integrum). Zoolog. Sci. 29, 60–65. doi: 10.2108/zsj.29.60
McPeek, M. A., Schrot, A. K., and Brown, J. M. (1996). Adaptation to predators in a new community: swimming performance and predator avoidance in damselflies. Ecology 77, 617–629.
Michod, R. E. (1979). Evolution of life histories in response to age-specific mortality factors. Am. Nat. 113, 531–550.
Molina-Moctezuma, A., Hernández-Rosas, A. L., and Zúñiga-Vega, J. J. (2020). Resource availability and its effects on mother to embryo nutrient transfer in two viviparous fish species. J. Exp. Zool. Part A Ecol. Integr. Physiol. 333, 181–193. doi: 10.1002/jez.2342
Moore, M. P., Riesch, R., and Martin, R. A. (2016). The predictability and magnitude of life-history divergence to ecological agents of selection: a meta-analysis in livebearing fishes. Ecol. Lett. 19, 435–442. doi: 10.1111/ele.12576
Mukherjee, S., Heithaus, M. R., Trexler, J. C., Ray-Mukherjee, J., and Vaudo, J. (2014). Perceived risk of predation affects reproductive life-history traits in Gambusia holbrooki, but not in Heterandria formosa. PLoS One 9:e88832. doi: 10.1371/journal.pone.0088832
Nickley, B., Saintignon, D., and Roberts, J. A. (2016). Influence of predator cues on terminal investment in courtship by male Schizocosa ocreata (Hentz, 1844) wolf spiders (Araneae: Lycosidae). J. Arachnol. 44, 176–181. doi: 10.1636/j15-64
Ohlberger, J., Staaks, G., and Hölker, F. (2006). Swimming efficiency and the influence of morphology on swimming costs in fishes. J. Comp. Physiol. B Biochem. Syst. Environ. Physiol. 176, 17–25. doi: 10.1007/s00360-005-0024-20
Olinger, C. T., Peoples, B. K., and Frimpong, E. A. (2016). Reproductive life history of Heterandria bimaculata (Heckel, 1848) (Poeciliinae: Poeciliidae) in the honduran interior highlands: trait variation along an elevational gradient. Neotrop. Ichthyol. 14:e150050. doi: 10.1590/1982-0224-20150050
Peterson, S. H., Ackerman, J. T., Herzog, M. P., Hartman, C. A., Croston, R., Feldheim, C. L., et al. (2019). Sitting ducklings: timing of hatch, nest departure, and predation risk for dabbling duck broods. Ecol. Evol. 9, 5490–5500. doi: 10.1002/ece3.5146
Portz, D. E., and Tyus, H. M. (2004). Fish humps in two colorado river fishes: a morphological response to cyprinid predation? Environ. Biol. Fishes 71, 233–245. doi: 10.1007/s10641-004-0300-y
Quicazan-Rubio, E. M., Van Leeuwen, J. L., Van Manen, K., Fleuren, M., Pollux, B. J. A., and Stamhuis, E. J. (2019). Coasting in live-bearing fish: the drag penalty of being pregnant. J. R. Soc. Interface 16:20180714. doi: 10.1098/rsif.2018.0714
Reznick, D. N. (1990). Experimentally induced life-history evolution in a natural population. Nature 346, 357–359.
Reznick, D. N., and Endler, J. A. (1982). The impact of predation on life history evolution in trinidadian guppies (Poecilia reticulata). Evolution (N. Y) 36, 160–177. doi: 10.1111/j.1558-5646.1982.tb05493.x
Reznick, D. N., Miles, D. B., and Winslow, S. (1992). Life history of Poecilia picta (Poeciliidae) from the Island of trinidad. Copeia 1992, 782–790.
Reznick, D. N., and Yang, A. P. (1993). The influence of fluctuating resources on life history: patterns of allocation and plasticity in female guppies. Ecology 74, 2011–2019.
Riesch, R., Martin, R. A., and Langerhans, R. B. (2013). Predation’s role in life-history evolution of a livebearing fish and a test of the trexler-DeAngelis model of maternal provisioning. Am. Nat. 181, 78–93. doi: 10.1086/668597
Rius, B. F., Petry, A. C., Langerhans, R. B., Figueiredo-Barros, M. P., Bozelli, R. L., Honda, L. K., et al. (2019). Correlates of life-history variation in the livebearing fish Poecilia vivipara (Cyprinodontiformes: Poeciliidae) inhabiting an environmental gradient. Biol. J. Linn. Soc. 126, 436–446. doi: 10.1093/biolinnean/bly208
Rollinson, N., and Brooks, R. J. (2008). Optimal offspring provisioning when egg size is “constrained”: a case study with the painted turtle Chrysemys picta. Oikos 117, 144–151. doi: 10.1111/j.2007.0030-1299.16088.x
Ryan, K. M., and Lindeman, P. V. (2007). Reproductive allometry in the common map turtle. graptemys geographica. Am. Midl. Nat. 158, 49–59.
Schrader, M., and Travis, J. (2012). Assessing the roles of population density and predation risk in the evolution of offspring size in populations of a placental fish. Ecol. Evol. 2, 1480–1490. doi: 10.1002/ece3.255
Stearns, S. C. (1977). The evolution of life history traits: a critique of the theory and a review of the data. Annu. Rev. Ecol. Syst. 8, 145–171. doi: 10.1146/annurev.es.08.110177.001045
Stoks, R., De Block, M., Slos, S., Van Doorslaer, W., and Rolff, J. (2006). Time constraints mediate predator-induced plasticity in immune function, condition, and life history. Ecology 87, 809–815.
Storfer, A. (1999). Gene flow and local adaptation in a sunfish-salamander system. Behav. Ecol. Sociobiol. 46, 273–279.
Strauss, R. E. (1990). Predation and life-history variation in Poecilia reliculata (Cyprinodontiformes: Poeciliidae). Environ. Biol. Fishes 27, 121–130. doi: 10.1007/BF00001941
Šupina, J., Bojková, J., and Boukal, D. (2016). Influence of food availability and predation risk on growth and maturation of Cloeon dipterum (Ephemeroptera: Baetidae). Zoosymposia 11, 53–64.
Turner, C. L. (1942). Morphogenesis of the gonopodial suspensorium in gambusia affinis and the induction of male suspensorial characters in the female by androgenic hormones. J. Exp. Biol. 91, 167–193.
Van Wassenbergh, S., Van Manen, K., Marcroft, T. A., Alfaro, M. E., and Stamhuis, E. J. (2015). Boxfish swimming paradox resolved: forces by the flow of water around the body promote manoeuvrability. J. R. Soc. Interface 12:20141146. doi: 10.1098/rsif.2014.1146
Walsh, M. R., and Reznick, D. N. (2009). Phenotypic diversification across an environmental gradient: a role for predators and resource availability on the evolution of life histories. Evolution (N. Y) 63, 3201–3213. doi: 10.1111/j.1558-5646.2009.00785.x
Walters, V. (1962). Body form and swimming performance in the scombroid fishes. Am. Zool. 2, 143–149.
Webb, P. W. (1982). Locomotor patterns in the evolution of actinopterygian fishes. Am. Zool. 22, 329–342.
Webb, P. W. (1984). Body form, locomotion and foraging in aquatic vertebrates. Am. Zool. 24, 107–120. doi: 10.1093/icb/24.1.107
Wesner, J. S., Billman, E. J., Meier, A., and Belk, M. C. (2011). Morphological convergence during pregnancy among predator and nonpredator populations of the livebearing fish Brachyrhaphis rhabdophora (Teleostei: Poeciliidae). Biol. J. Linn. Soc. 104, 386–392.
Williams, G. C. (1966). Natural selection, the costs of reproduction, and a refinement of lack’s principle. Am. Nat. 100, 687–690.
Zandonà, E., Dalton, C. M., El-Sabaawi, R. W., Howard, J. L., Marshall, M. C., Kilham, S. S., et al. (2017). Population variation in the trophic niche of the Trinidadian guppy from different predation regimes. Sci. Rep. 7:5770. doi: 10.1038/s41598-017-06163-6166
Zúñiga-Vega, J. J., Reznick, D. N., and Johnson, J. B. (2007). Habitat predicts reproductive superfetation and body shape in the livebearing fish Poeciliopsis turrubarensis. Oikos 116, 995–1005. doi: 10.1111/j.0030-1299.2007.15763.x
Keywords: Poeciliidae, life history, Alfaro cultratus, allometry, constraints, predation, terminal investment hypothesis
Citation: Golden KB, Belk MC and Johnson JB (2021) Predator Environment Does Not Predict Life History in the Morphologically Constrained Fish Alfaro cultratus (Cyprinodontiformes: Poeciliidae). Front. Ecol. Evol. 9:607802. doi: 10.3389/fevo.2021.607802
Received: 18 September 2020; Accepted: 05 February 2021;
Published: 01 March 2021.
Edited by:
Shannon J. McCauley, University of Toronto Mississauga, CanadaReviewed by:
Matthew Wund, The College of New Jersey, United StatesCopyright © 2021 Golden, Belk and Johnson. This is an open-access article distributed under the terms of the Creative Commons Attribution License (CC BY). The use, distribution or reproduction in other forums is permitted, provided the original author(s) and the copyright owner(s) are credited and that the original publication in this journal is cited, in accordance with accepted academic practice. No use, distribution or reproduction is permitted which does not comply with these terms.
*Correspondence: Kaitlyn B. Golden, a2FpdGx5bmJnb2xkZW5AZ21haWwuY29t
Disclaimer: All claims expressed in this article are solely those of the authors and do not necessarily represent those of their affiliated organizations, or those of the publisher, the editors and the reviewers. Any product that may be evaluated in this article or claim that may be made by its manufacturer is not guaranteed or endorsed by the publisher.
Research integrity at Frontiers
Learn more about the work of our research integrity team to safeguard the quality of each article we publish.