- 1Department of Biological Sciences, Macquarie University, Sydney, NSW, Australia
- 2School of Biological Sciences, University of Aberdeen, Aberdeen, United Kingdom
- 3Department of Applied BioSciences, Macquarie University, Sydney, NSW, Australia
The environmental conditions experienced by parents influence next generations, with the parental nutritional status playing an important role in shaping offspring phenotypes. Our understanding of transgenerational effects of parental diet on offspring pathogen resistance is, however, poorly documented. We manipulated the quality of parental diet (i.e., mother, father, or both) and measured effects on offspring development and survival after an immune challenge by septic infection. We used Bactrocera tryoni as host model infected with the pathogenic bacterium, Serratia marcescens. Our results showed no significant effect of maternal, or paternal, diet on offspring resistance. Interestingly, when the diet of both parents was manipulated, sons from parents fed either carbohydrate- or protein-biased diets had higher survival upon pathogen infection than sons from parents fed balanced diets. The quality of the parental diet had no effect on offspring developmental traits with the exception of egg hatching percentage which decreased when mothers were fed a protein-biased diet. Our results emphasised the complexity of nutritional transgenerational effects on offspring pathogen resistance and development.
Introduction
Environmental conditions experienced by parents can have long-term effects (Burton and Metcalfe, 2014; Woestmann and Saastamoinen, 2016). In both vertebrates and invertebrates, parents in good physiological conditions (e.g., good nutritional condition or favourable temperature) tend to produce offspring with higher fitness relative to the offspring of parents with low physiological conditions (Mousseau and Fox, 1998; Qvarnström and Price, 2001; Bonduriansky and Head, 2007). The environmental conditions experienced by parents can also serve as a cue to predict conditions that offspring are likely to experience and offspring can gain benefits from acquiring information of their future environment (i.e., “transgenerational phenotypic plasticity”) (West-Eberhard, 2003; Pigliucci, 2001; Whitman and Agrawal, 2009). The outcome of transgenerational phenotypic plasticity depends, however, on whether environmental conditions experienced across generations are predictable or not (Burgess and Marshall, 2014) and, therefore, if the parental environment is a reliable predictor of the offspring environment (e.g., Gluckman and Hanson, 2004; Galloway and Etterson, 2007; Marshall and Uller, 2007; Bateson et al., 2014; Burgess and Marshall, 2014; Murren et al., 2015). A mismatch between the offspring’ and parents’ environments can potentially reduce offspring fitness (see e.g., Gluckman et al., 2007; Raubenheimer et al., 2012), although this is not true for all taxa (Uller et al., 2013).
Amongst the key environmental factors experienced by parents, nutrition is an essential factor that shapes offspring’s phenotype (Bonduriansky and Day, 2009). In insects, parental diet influences many offspring traits including body size and body weight (Vijendravarma et al., 2010; Triggs and Knell, 2012; Valtonen et al., 2012; Dew-Budd et al., 2016), developmental time (Vijendravarma et al., 2010; Valtonen et al., 2012; Matzkin et al., 2013), egg-to-adult survivorship (Prasad et al., 2003), egg hatching success (Bonduriansky et al., 2016), reproduction (Matzkin et al., 2013) and body composition (Buescher et al., 2013; Matzkin et al., 2013; Brookheart and Duncan, 2016; Dew-Budd et al., 2016). Parental diet can also affect the transcriptome of offspring through a decrease in the quantity of ribosomal RNA and changes in expression levels of metabolic and chromatin-coding genes, which might result in cancers and diabetes in the offspring (Buescher et al., 2013; Öst et al., 2014; Aldrich and Maggert, 2015).
Immunity and resistance to infections are also known to be influenced by the quality of the parental diet. In Drosophila melanogaster, expression level of immune genes is lower in sons of fathers fed low-protein diets compared to sons of fathers fed high-protein diets (Zajitschek et al., 2017). When offspring are immune-challenged, parental diet also influence their immune response level and impact their resistance to infections. In the Indian meal moth Plodia interpunctella, for instance, larvae from mothers reared in poor diets (i.e., diets with high cellulose content) are less resistant to viral and fungal infections than larvae from parents reared in rich diets (i.e., diets with low cellulose content) (Boots and Roberts, 2012). This effect might be explained by a reduced phenoloxidase activity and lower number of haemocytes (Kangassalo et al., 2015), (Boots and Roberts, 2012), (Triggs and Knell, 2012). In the mosquito Aedes aegypti, daughters from parents kept on a poor diet have higher viral load compared to daughters from parents in a rich diet, an effect that persists even when the offspring are fed a rich diet (Zirbel et al., 2018). Nonetheless, no difference in adult survival after bacterial infection has been detected between offspring from parents fed standard (balanced) or protein-deprived diets (Valtonen et al., 2012). In the Melitaea cinxia butterfly, maternal diet (ad libitum vs. starvation) does not have a significant effect on the total number of haemocytes, granular cells and oenocytoids of offspring infected with the parasitoid Cotesia melitaearum (Saastamoinen et al., 2013). Together, these findings highlight the complexity of the effects of parental diet on offspring immunity and pathogen resistance.
To date, however, investigations in this field are still partial because manipulations of parental diet have mainly focussed on the total energy of the food, overlooking the effect of relative ratio between diet components on offspring traits. In addition, studies have mainly manipulated the diet of mothers or both parents simultaneously, overlooking the effects of fathers’ diet. Yet, there is increasing evidence showing that the environment experienced by fathers can prime offspring basal immunity (Zanchi et al., 2011; Eggert et al., 2014) and increase offspring resistance to infection (Roth et al., 2010). As a result, we still lack a proper empirical investigation of the trans-generational effects of parental diet (both single-sex and both-sexes approaches) on immune state and resistance.
Here, we investigated transgenerational effects of nutrition by manipulating the protein-to-carbohydrate balance in the diet (hereafter referred to as “PC ratio”) of mothers, fathers, and both parents simultaneously. The fruit fly Bactrocera tryoni, one of the most damaging pest insect in Australia (Hancock et al., 2000; Sutherst et al., 2000; Clarke et al., 2011), was used as model system. Transgenerational effects of diet manipulation were measured on (i) development traits including egg hatching percentage, pupation percentage, emergence percentage, larval weight, pupal weight and larval body lipid reserves and (ii) resistance to infection of the offspring once adult by recording the survival of male and female offspring after a septic infection with the pathogenic bacterium Serratia marcescens. Knowledge gained from this study contributes insights into ecological questions investigating the effects of parental environmental conditions on offspring life history traits. Results are discussed in the context of transgenerational phenotypic plasticity and what type of information parental diet can pass to the next generations.
Materials and Methods
Fly Stock
Flies originated from a B. tryoni stock colony that was maintained on a gel-based larval diet (Moadeli et al., 2017) and raised as adult with ad libitum hydrolysed yeast (MP Biomedicals Cat. no 02103304) and sugar (CSR® White Sugar). The stock colony was maintained at 25°C and 65% humidity with a 12-h light/dark cycle for 25 generations.
Diet Preparation
We used four single liquid diets varying in the protein-to-carbohydrate ratio (PC 1:8, 1:5, 1:3, and 1:1) (Dinh et al., 2019). A choice diet was included as control where solutions of hydrolysed yeast and sugar were separately offered to the flies. Based on previous work, we considered PC 1:3 as a balanced diet for non-infected B. tryoni (see Fanson et al., 2009), PC 1:8 and 1:5 as carbohydrate-biased diets, and PC 1:1 as a protein-biased diet. We did not include any diets with a greater concentration of yeast because they would have had drastically affected fly mortality. Diets were prepared by mixing hydrolysed yeast (MP Biomedicals Cat. no 02103304) and/or sugar (CSR® White Sugar) in warm distilled water using a hot plate set at 80°C. All diets were made to a final concentration of 120 g/L. The hydrolysed yeast used in this study contains 62.1% protein and 1% carbohydrate.
Diet Manipulation in Parents
We performed three experiments where the diet of mothers, fathers, or both parents was manipulated, and the effects on offspring life-history traits were measured. Parental diet was manipulated only at adult stage. The experimental design is described in Figure 1. In the first experiment, the diet of mothers was manipulated by assigning groups of two-day old females to one of five diet treatments (PC 1:8, 1:5, 1:3, 1:1, or choice diet), whereas, fathers were given a choice diet. In contrast, in the second experiment, the diet of fathers was manipulated (same treatments as above) while mothers were fed a choice diet. In the third experiment, we manipulated the diet of both parents (i.e., both parents were fed the same diet). Food was provided to the flies via a cotton ball soaked in the liquid diets. Flies from all experiments were fed the experimental diets for 12 days and allowed to mate in groups of 45 females and 45 males, selected randomly (noted that we set up the experiment with 60 males/females in order to obtain a final sample size of 45 males and females). Three mating cages were prepared for each diet treatment. Eggs were collected in each mating cage for 24 h using an ovipositional device that consisted in a plastic bottle with numerous puncture holes and filled with 5 mL of water to maintain humidity. All eggs were pooled and used to generate offspring for subsequent experiments.
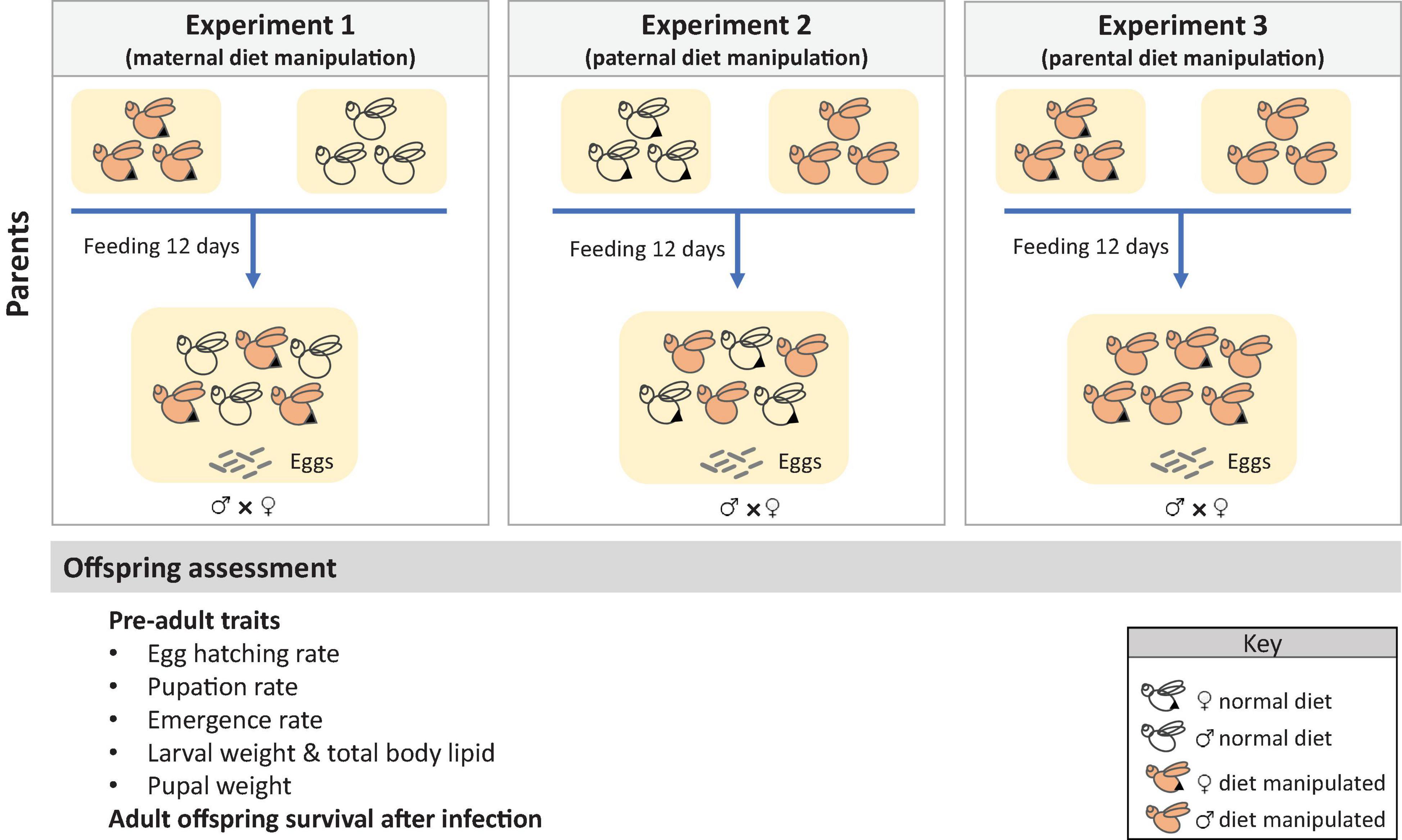
Figure 1. Experimental set up of the three experiments of this study. Effects of maternal, paternal and parental manipulation of the diet were measured on several pre-adult and adult traits of the offspring.
Offspring Generation
Collected eggs were used in two experimental set ups.
(i) Measuring the percentage of egg hatching, percentage of pupation and percentage of emergence.
Groups of 100 eggs were randomly selected and transferred to 100 mm Petri-dishes containing 15 mL of standard larval diet (Moadeli et al., 2017). We recorded the number of eggs that did not hatch after 4 days. Petri-dishes with hatched eggs were then placed in 1.75 mL containers with 40 mL vermiculite where larvae, once they reached fourth instar, were allowed to jump out of their larval environment and pupate. We recorded the number of pupae and newly emerged flies. Two sets of eggs were collected from each mating cage, yielding six sets of eggs in total (100 eggs/set) collected from each diet treatment.
(ii) Measuring larval weight, pupal weight, larval body lipid, and pathogen resistance of adult offspring.
For this purpose, 90 μL of an egg solution (approximately 1,000 eggs mixed with water) was collected from each mating cage. Eggs were transferred to diet trays containing 150 mL of standard larval diet yielding in three replicates for each diet treatment (Moadeli et al., 2017). Five days after seeding the eggs, the trays were placed in 12.5 L containers with 500 mL vermiculite and lids removed to allow the larvae to jump and pupate. Twenty larvae per tray (60 in total per diet) were collected within 24 h since they started jumping out of the diet (7 days after seeding eggs) to measure larval body weight and body lipid. The rest of the larvae were left to develop. Seven days later, 20 pupae per tray (60 in total per diet) were collected for measurement of pupal body weight. The rest of pupae were allowed to develop into adults for the infection experiment. Upon emergence, adult flies were fed standard adult diet [(ad libitum hydrolysed yeast (MP Biomedicals Cat. no 02103304) and sugar (CSR® White Sugar)].
Larval Weight, Pupal Weight, and Larval Body Lipid
Larval and pupal weights were measured using a microbalance (Sartorius, accuracy ± 0.001mg).
Larval body lipid was measured in 21 individual larvae (3 replicates per diet treatment, 7 larvae/replicate) using the protocol described in Dinh et al. (2019). Briefly, larvae were snap frozen at −20°C, bodies transferred into individual 6 mL glass tubes (Sigma-Aldrich) and dried in a drying oven (Binder) at 50°C for 48 h. Dry body weight was measured using a microbalance (Sartorius, accuracy ± 0.001 mg). Total body lipid was then extracted in three 24 h washes of chloroform (Sigma-Aldrich Cat. No 650498). At the end of the third chloroform wash, lipid-free bodies were re-dried and re-weighed to calculate lipid content. The percentage of body lipid was calculated by subtracting the lipid-free dry body weight to the initial dry body weight and dividing the difference by the initial body weight multiplied by 100.
Survival of Adult Flies After Septic Infection With Serratia marcescens
Serratia marcescens (ATCC 13880, Thermo Scientific) was cultured on Nutrition broth (Oxoid, CM0001) overnight (approximately 12 h) at 26°C, 200 rpm. The liquid culture was centrifuged at 10,000 × g for 2 min at 4°C to remove residues of the culture medium, and the pellet was washed twice using 1× Phosphate Buffered Saline (PBS, Sigma). The bacterial cells were resuspended in sterile PBS and the solution diluted to achieve an optical density (OD600) of 0.025. Injections were performed on two-day old flies that were fed choice diet prior to the bacterial challenge. A group of 15–20 flies were cold anesthetised at −20°C for 2 m and kept on dry bath (Product code: MK20) at −10°C during the injection. Bacterial cells were injected in the fly at the coxa of the third right leg using a MP4 microinjection system (World Precision Instruments). The injection volume was 0.2 μL dispensed at the rate of 50 nL/s, corresponding to approximately 2,000 bacterial cells/fly. PBS-injected flies were used as controls for injury. After injection, flies were kept in a 1.5 L plastic cage and fed a choice diet. Survival was recorded for six days post-infection (PI). We ran three replicates for each diet treatment (18–22 flies/replicate).
Statistical Analysis
Generalised Linear Models (GLM) with binomial distribution and quasi extension were fitted to test for effects of diet manipulation in mothers/fathers/both parents on egg hatching percentage, pupation percentage, emergence percentage, and percentage of larval body lipid reserves. A GLM with Gaussian distribution was used to assess for the effect of diet manipulation in mothers/fathers/both parents on pupal weight and larval weight. Effects of maternal or paternal or parental diet, offspring sex, offspring infection treatment and their interactions on the survival of adult offspring were tested using Cox regression analysis. All analyses were performed in R (R Development Core Team, 2011). Graphs and Kaplan–Meier survival curves were generated from BM SPSS Statistics 25.0.
Results
Effects of Maternal Diet on Offspring Development and Pathogen Resistance
Maternal diet significantly influenced egg hatching percentage (GLM, F4,26 = 9.401, P < 0.001) (Supplementary Table 1). Eggs from mothers fed the protein-biased diet (PC 1:1) had a lower hatching percentage compared to those from the other diet treatments (Figure 2). There was no significant effect of the maternal diet on pupation percentage (GLM, F4,24 = 1.964, P = 0.132) and emergence percentage (GLM, F4,24 = 2.570, P = 0.064) (Supplementary Table 1). Moreover, maternal diet had no effect on larval weight (GLM, F4,296 = 1.742, P = 0.141), pupal weight (GLM, F4,298 = 1.549, P = 0.188), and percentage of total body lipid in offspring larvae (GLM, F4,90 = 0.726, P = 0.576) (Supplementary Table 1).
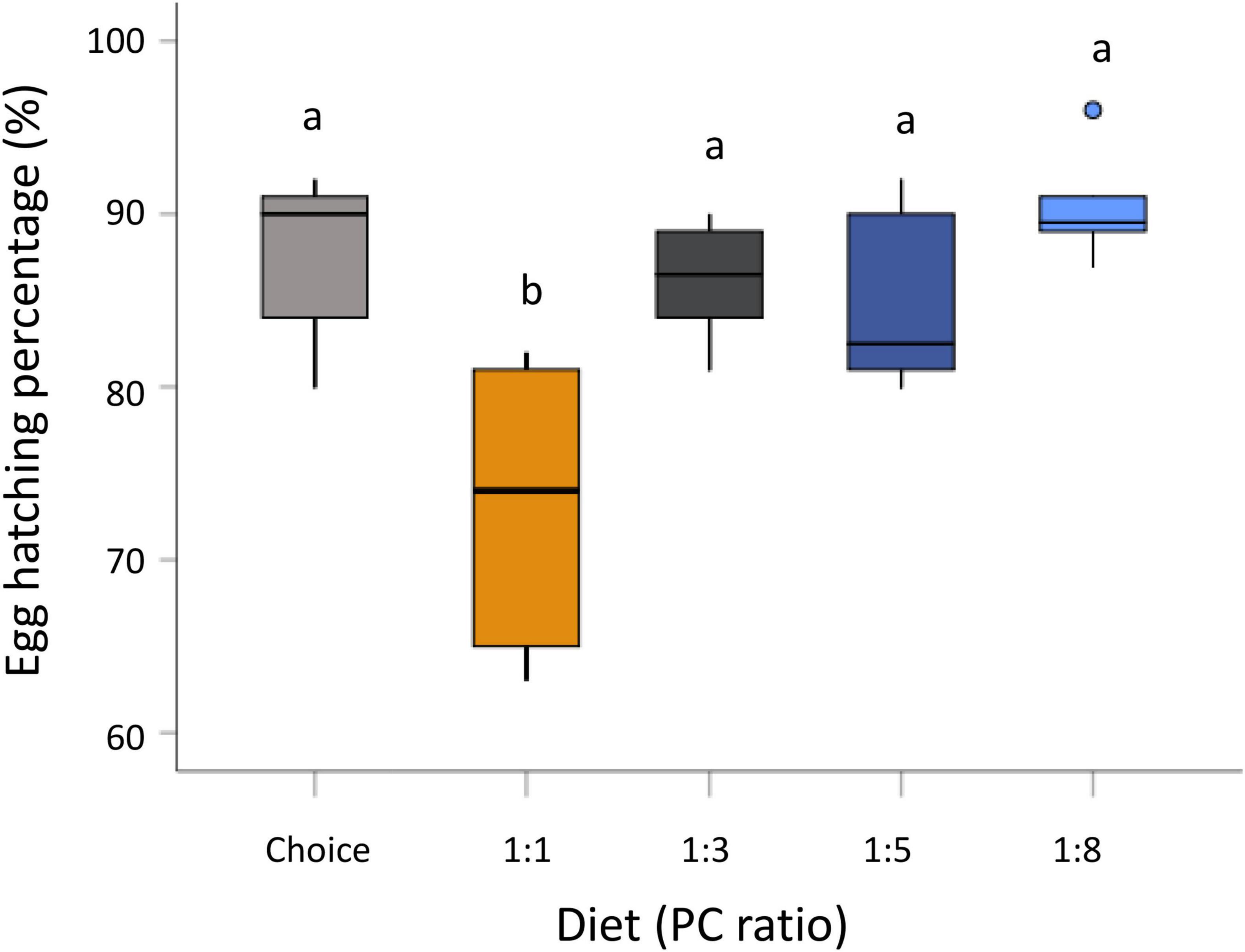
Figure 2. Effect of maternal diet on hatching percentage. Mothers were fed five diets varying in the protein-to-carbohydrate ratio (PC ratio) and egg hatching rate was measured. Bars indicate the percentage of egg hatching. Letters above bars indicate significant difference of egg hatching percentage between diet treatments. Significance was accessed by Student-Newman-Keuls (SNK) test and was considered at P < 0.05.
When survival was analysed during the six first days using a COX regression, we could not detect any statistically significance of the maternal diet treatment on the survival of infected offspring (Supplementary Table 2). However and as expected, non-infected offspring survived at a greater rate than infected ones (Supplementary Table 2). At day 4, there was in trend where the percentage of dead sons was greater on the two extreme diets (P:C = 1:8 & 1:1), while it was lower when mums where fed the choice diet (Figure 3A). We did not observe a similar trend for daughters (Figure 3B).
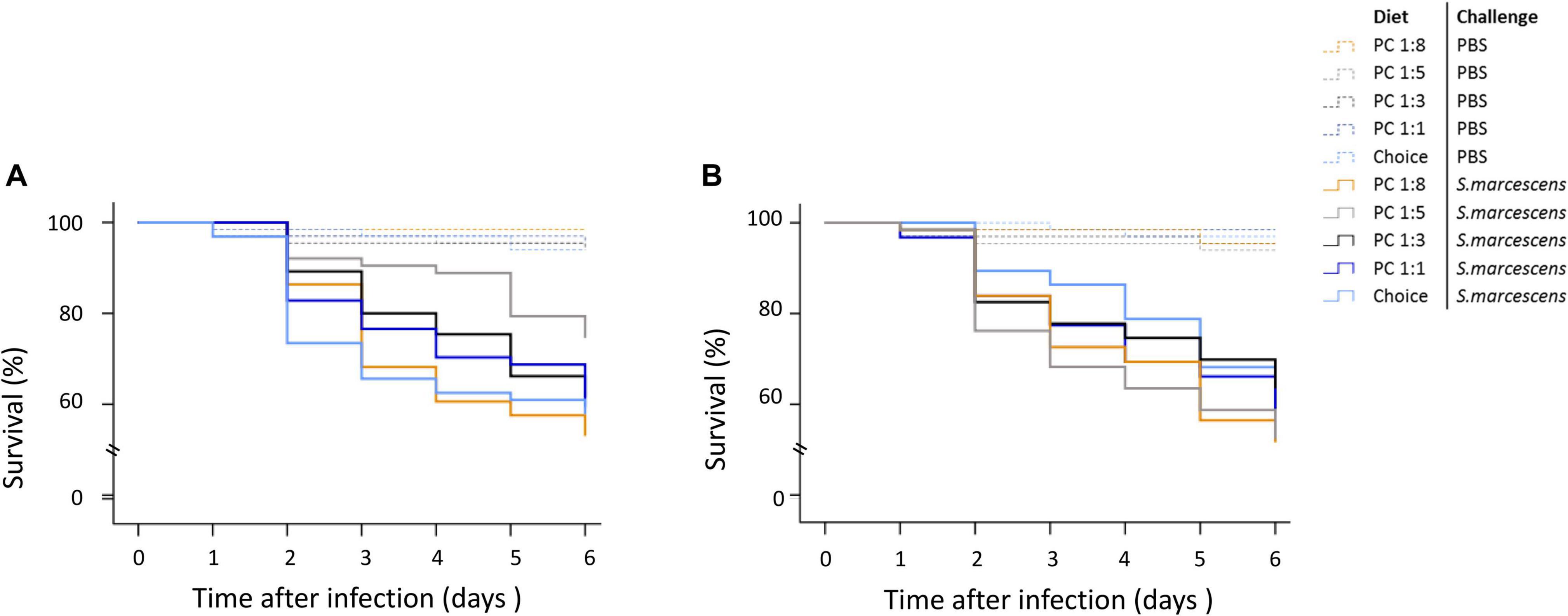
Figure 3. Effect of maternal diet on offspring survival during the first 6 days post septic infection. Mothers were fed one of five diets varying in the protein-to-carbohydrate ratio (PC ratio) whereas fathers were fed a choice diet. Their sons (A) and daughters (B) were injected with either PBS or Serratia marcescens at adult stage. Lines indicates Kaplan–Meier survival curves [dash lines (non-infected flies), continuous lines (infected flies)]. Colours indicate maternal diet treatments [orange (PC 1:1), light grey (choice diet), dark grey (PC 1:3), dark blue (PC 1:5) and light blue (PC 1:8)]. Significant differences between survival curves were determined by Cox regression analysis at P < 0.05.
Effects of Paternal Diet on Offspring Development and Pathogen Resistance
Paternal diet did not affect hatching percentage (GLM, F4,40 = 0.852, P = 0.500), pupation percentage (GLM, F4,40 = 0.328, P = 0.856) or emergence percentage (GLM, F4,40 = 0.329, P = 0.857) (Supplementary Table 3). We also did not observe any significant effects of paternal diet on larval weight (GLM, F4,297 = 1.575, P = 0.181), pupal weight (GLM, F4,294 = 2.091, P = 0.082), and percentage of total body lipid in offspring larvae (GLM, F4,86 = 0.999, P = 0.412) (Supplementary Table 3).
We did not detect any statistically significance of the paternal diet treatment on the survival trajectories of infected offspring (Supplementary Table 4 and Figure 4).
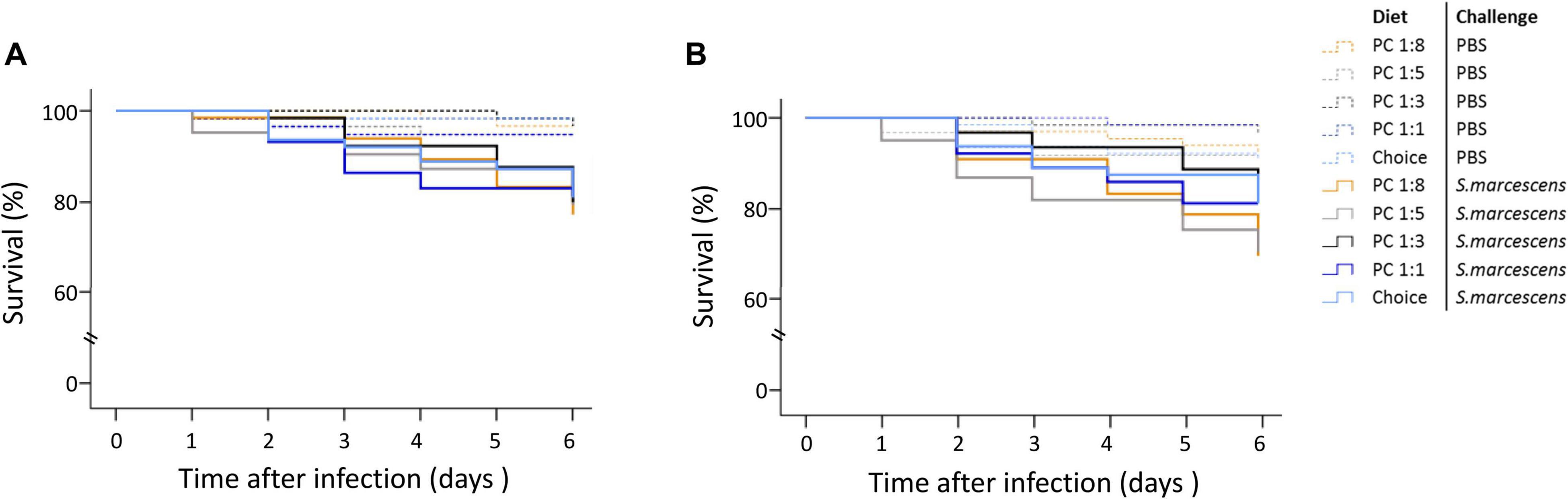
Figure 4. Effect of paternal diet on offspring survival during the first 6 days post septic infection. Fathers were fed one of five diets varying in the protein-to-carbohydrate ratio (PC ratio) whereas mothers were fed a choice diet. Their sons (A) and daughters (B) were injected with either PBS or Serratia marcescens at adult stage. Lines indicates Kaplan–Meier survival curves [dash lines (non-infected flies), continuous lines (infected flies). Colours indicate paternal diet treatments [orange (PC 1:1), light grey (choice diet), dark grey (PC 1:3), dark blue (PC 1:5) and light blue (PC 1:8)]. Significant differences between survival curves were determined by Cox regression analysis at P < 0.05.
Effects of Parental Diet on Offspring Development and Pathogen Resistance
Parental diet had no effect on hatching percentage (GLM, F4,34 = 0.749, P = 0.566), pupation percentage (GLM, F4,34 = 1.882, P = 0.136), and emergence percentage (GLM, F4,34 = 2.117, P = 0.100) (Supplementary Table 5). We also did not observe any significant effects of parental diet on larval weight (GLM, F4,294 = 0.964, P = 0.428), pupal weight (GLM, F4,294 = 2.091, P = 0.082), and percentage of total body lipid in offspring larvae (GLM, F4,99 = 0.706, P = 0.589) (Supplementary Table 5).
The interaction between parental diet, offspring sex and infection treatment significantly affected the survival of adult offspring (Supplementary Table 6). To better understand the result, we ran four models to test for the effect of parental diet on the survival rate of non-infected males, non-infected females, infected males, and infected females. We found that parental diet significantly affected the survival of infected sons but not infected daughters (Figure 5). Particularly, the survival of sons from parents fed PC 1:8, 1:5 and 1:1 were greater than sons from parents fed the PC 1:3 and choice diet (Figure 5A). Survival of non-infected sons and daughters was not significantly influenced by parental diet manipulation with, on average, 10% of non-infected offspring dying during the 6 first days post infection (Figure 5).
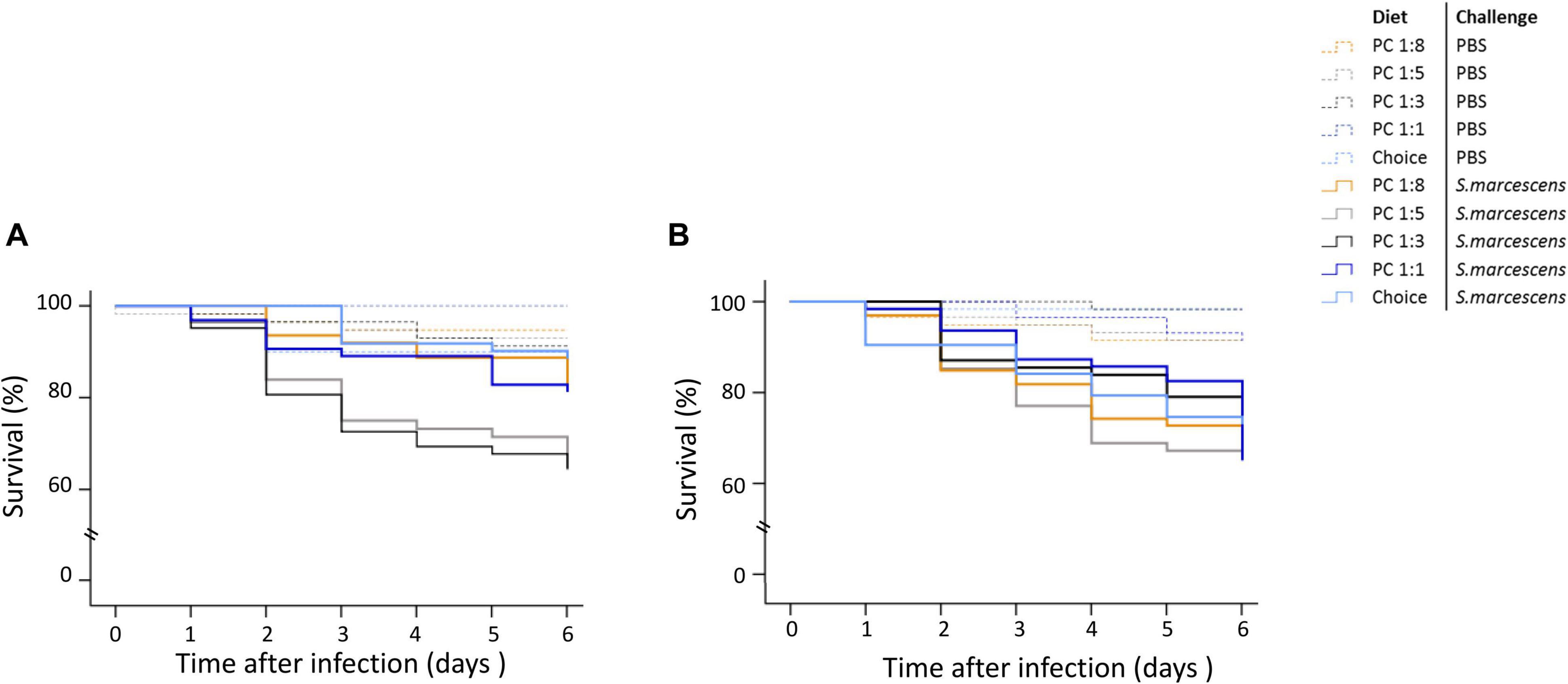
Figure 5. Effect of parental diet on offspring survival during the first 6 days post septic infection. Both mother and fathers were fed one of five diets varying in the protein-to-carbohydrate ratio (PC ratio). Their sons (A) and daughters (B) were injected with either PBS or Serratia marcescens at adult stage. Lines indicates Kaplan–Meier survival curves [dash lines (non-infected flies), continuous lines (infected flies). Colours indicate parental diet treatments [orange (PC 1:1), light grey (choice diet), dark grey (PC 1:3), dark blue (PC 1:5) and light blue (PC 1:8)]. Significant differences between survival curves were determined by Cox regression analysis and was determined at P < 0.05.
Discussion
In this study, we investigated how parental diet (i.e., diet of mothers, fathers, or both) influences offspring developmental traits and adult survival after septic infection with the pathogenic bacterium S. marcescens. Surprisingly, when the diet composition was manipulated for both parents, we found that infected sons from parents fed unbalanced diets survived at a greater rate after infection compared to those from parents fed balanced diets. Parental diet manipulation hardly affected offspring developmental traits, with only a lower hatching percentage observed when mothers were fed a protein-biased diet. Paternal and maternal diets did not affect the survival of infected offspring.
A recent study in Drosophila has shown that maternal environmental condition other than infection (i.e., cohabitation with a parasitic wasp) results in offspring that are more capable of resisting an infection (Bozler et al., 2020). Our results showed a sex-dependent effects of both parents’, not only mothers’, diet on offspring pathogen resistance. Previous studies have shown that the parental diet can affect differently the age and size at maturity of male and female offspring (Zizzari et al., 2016). Considering the relationship between hosts’ age and body size with resistance to infection (Garbutt and Little, 2017; Soumya et al., 2017), a sex-dependent effect of parental diet on offspring disease resistance is therefore possible. While our knowledge of the mechanisms involved in sex−specific effects are still limited, this might involve sex-specific transfer of genetic material. This is supported by a study in Drosophila showing specific transfer of ribosomal DNA from fathers to daughters but not to sons when fathers were fed a protein-rich diet (Aldrich and Maggert, 2015). More investigations of the molecular mechanisms will give us a better understanding of how parental diet can have sex-specific effects.
Transgenerational effects can be adaptive with offspring having greater fitness in environments similar to those experienced by their parents (see for instance, Gluckman and Hanson, 2004; Galloway and Etterson, 2007; Gluckman et al., 2007; Marshall and Uller, 2007; Raubenheimer et al., 2012; Bateson et al., 2014; Burgess and Marshall, 2014; Murren et al., 2015). Our results showed, however, that offspring from parents fed unbalanced diets had a greater survival despite the mismatch between the nutritional conditions of parents and offspring (i.e., parents were fed unbalanced diets and offspring were fed a balanced diet). Despite this, it remains to be tested whether the same effect is observed when parents are fed a balanced diet and offspring unbalanced diets. We can speculate, in this case, that parents in poor nutritional conditions might have primed their offspring to better survive the infection (see also (Mitchell and Read, 2005; Ben-Ami et al., 2009; Boots and Roberts, 2012). The differences between maternal and paternal environments might be another important factor when explaining transgenerational plasticity. If the environmental conditions experienced by mothers and fathers are different, this may cause a conflict in the parental strategies to maximise their offspring performance. On the other hand, when both parents experienced the same condition, they may transfer the same environmental information to their offspring. In this study, we found sex-specific transgenerational effects when diets of both parents were manipulated but no effects were detected when only the paternal or maternal diet was manipulated. This reflexes the complexity of the effects of parental diet on offspring pathogen resistance that cannot be predicted by measuring the single effects of paternal and maternal diet (see also Valtonen et al., 2012). Further, this study was focused on better understanding the potential eco-evolutionary effects of parental diet, and therefore mating experiments were conducted in large groups rather than in single pairs, which precludes us to infer the contribution of individual variation. The extent to which individual variation vs population level effects contribute to the findings presented here is beyond the scope of this paper and remains an important avenue for future research.
Our study, together with previous work in Galleria mellonella moth and D. melanogaster, showed no effect of paternal diet on the survival of offspring during infection (Valtonen et al., 2012; Kangassalo et al., 2015). Although effects of poor paternal diet on offspring phenoloxidase activity and expression level of immune genes have been observed in P. interpunctella moths (Triggs and Knell, 2012) and D. melanogaster (Zajitschek et al., 2017), changes in immune components does not necessarily lead to changes in survival after infection (Adamo, 2004). Measuring different immune traits in offspring in addition to their survival rate would help to gain a more comphrehensive view on how parental diet affects immunity and resistance in offspring. Furthermore, successful host defence involves resistance (i.e., clear pathogen) and/or tolerance (i.e., reduce the damage of the infection on its health) mechanisms (Ayres and Schneider, 2009; Kutzer and Armitage, 2016; Miller and Cotter, 2018); and effects of an infection on can be pathogen-specific. For instance, in D. melanogaster, while Salmonella typhimurium infection involves resistance mechanism, Listeria monocytogenes infection involves tolerance mechanism (Ayres and Schneider, 2009). Given that parental diet can influence both tolerance and resistance in offspring, measuring the bacterial load and fitness traits (e.g., fecundity and growth) of infected offspring would provide helpful information of the mechanisms underlying the effect of parental diet on offspring’s survival after infection.
Lastly, we found that parental diet hardly affected offspring developmental traits. These findings are consistent with previous observations in a neriid flies, Telostylinus angusticollis showing that only the egg hatching success was affected by maternal diet (Bonduriansky et al., 2016). The weak effects of parental diet on developmental traits might be due to the abundant resources that were available to the offspring at larval stage. Indeed, parental effects on offspring performance have been suggested to be more pronounced when juveniles encounter poor environmental conditions (Marshall et al., 2006; Bonduriansky and Head, 2007; Donelson et al., 2009; Vijendravarma et al., 2010). Interestingly, in D. melanogaster, parental diet has weak effects on offspring’s phenotype, but was found to significantly influence grand-offspring (Deas et al., 2019). Future investigations measuring the effects of parental diet on offspring traits under different larval dietary conditions and throughout several generations might improve our understanding of the transgenerational effects of nutrition on offspring life-history traits.
Data Availability Statement
The original contributions presented in the study are included in the article/Supplementary Materials, further inquiries can be directed to the corresponding author/s.
Author Contributions
HD and FP designed the experiment. HD and BN collected the data. HD, JM, and FP analysed the data. All authors contributed to the writing and revision of the manuscript, and approved the final version submitted to the journal.
Funding
This research was conducted as part of the SITplus collaborative fruit fly program. Project Raising Q-fly Sterile Insect Technique to World Standard (HG14033) is funded by the Hort Frontiers Fruit Fly Fund, part of the Hort Frontiers strategic partnership initiative developed by Hort Innovation, with co-investment from Macquarie University and contributions from the Australian Government. HD was supported by Macquarie University Research Excellence Scholarship. BN was funded by the international Research Training Program (iRTP) scholarship from Macquarie University (NSW, Australia).
Conflict of Interest
The authors declare that the research was conducted in the absence of any commercial or financial relationships that could be construed as a potential conflict of interest.
Supplementary Material
The Supplementary Material for this article can be found online at: https://www.frontiersin.org/articles/10.3389/fevo.2021.606993/full#supplementary-material
References
Adamo, S. A. (2004). How should behavioural ecologists interpret measurements of immunity? Anim. Behav. 68, 1443–1449. doi: 10.1016/j.anbehav.2004.05.005
Aldrich, J. C., and Maggert, K. A. (2015). Transgenerational inheritance of diet-induced genome rearrangements in Drosophila. PLoS Genet. 11:e1005148. doi: 10.1371/journal.pgen.1005148
Ayres, J. S., and Schneider, D. S. (2009). The role of anorexia in resistance and tolerance to infections in Drosophila. PLoS Biol. 7:e1000150. doi: 10.1371/journal.pbio.1000150
Bateson, P., Gluckman, P., and Hanson, M. (2014). The biology of developmental plasticity and the Predictive Adaptive Response hypothesis. J. Physiol. 592, 2357–2368. doi: 10.1113/jphysiol.2014.271460
Ben-Ami, F., Ebert, D., and Regoes, R. R. (2009). Pathogen dose infectivity curves as a method to analyze the distribution of host susceptibility: A quantitative assessment of maternal effects after food stress and pathogen exposure. Am. Nat. 175, 106–115. doi: 10.1086/648672
Bonduriansky, R., and Day, T. (2009). Nongenetic inheritance and its evolutionary implications. Annu. Rev. Ecol. Evol. Syst. 40, 103–125. doi: 10.1146/annurev.ecolsys.39.110707.173441
Bonduriansky, R., and Head, M. (2007). Maternal and paternal condition effects on offspring phenotype in Telostylinus angusticollis (Diptera: Neriidae). J. Evol. Biol. 20, 2379–2388. doi: 10.1111/j.1420-9101.2007.01419.x
Bonduriansky, R., Runagall-McNaull, A., and Crean, A. J. (2016). The nutritional geometry of parental effects: maternal and paternal macronutrient consumption and offspring phenotype in a neriid fly. Funct. Ecol. 30, 1675–1686. doi: 10.1111/1365-2435.12643
Boots, M., and Roberts, K. E. (2012). Maternal effects in disease resistance: Poor maternal environment increases offspring resistance to an insect virus. Proc. R. Soc. B Biol. Sci. 279, 4009–4014. doi: 10.1098/rspb.2012.1073
Bozler, J., Kacsoh, B. Z., and Bosco, G. (2020). Maternal priming of offspring immune system in Drosophila. G3 Genes Genomes Genetics 10, 165–175. doi: 10.1534/g3.119.400852
Brookheart, R. T., and Duncan, J. G. (2016). Drosophila melanogaster: An emerging model of transgenerational effects of maternal obesity. Mol. Cell. Endocrinol. 435, 20–28. doi: 10.1016/j.mce.2015.12.003
Buescher, J. L., Musselman, L. P., Wilson, C. A., Lang, T., Keleher, M., Baranski, T. J., et al. (2013). Evidence for transgenerational metabolic programming in Drosophila. DMM Dis. Model. Mech. 6, 1123–1132. doi: 10.1242/dmm.011924
Burgess, S. C., and Marshall, D. J. (2014). Adaptive parental effects: The importance of estimating environmental predictability and offspring fitness appropriately. Oikos 123, 769–776. doi: 10.1111/oik.01235
Burton, T., and Metcalfe, N. B. (2014). Can environmental conditions experienced in early life influence future generations? Proc. R. Soc. B Biol. Sci. 2014:311. doi: 10.1098/rspb.2014.0311
Clarke, A. R., Powell, K. S., Weldon, C. W., and Taylor, P. W. (2011). The ecology of Bactrocera tryoni (Diptera: Tephritidae): what do we know to assist pest management? Ann. Appl. Biol. 158, 26–54. doi: 10.1111/j.1744-7348.2010.00448.x
Deas, J. B., Blondel, L., and Extavour, C. G. (2019). Ancestral and offspring nutrition interact to affect life-history traits in Drosophila melanogaster. Proc. R. Soc. B Biol. Sci. 286:488809. doi: 10.1098/rspb.2018.2778
Dew-Budd, K., Jarnigan, J., and Reed, L. K. (2016). Genetic and sex-specific transgenerational effects of a high fat diet in drosophila melanogaster. PLoS One 11:e0160857. doi: 10.1371/journal.pone.0160857
Dinh, H., Mendez, V., Tabrizi, S. T., and Ponton, F. (2019). Macronutrients and infection in fruit flies. Insect Biochem. Mol. Biol. 110, 98–104. doi: 10.1016/j.ibmb.2019.05.002
Donelson, J. M., Munday, P. L., and McCormick, M. I. (2009). Parental effects on offspring life histories: when are they important? Biol. Lett. 5, 262–265. doi: 10.1098/rsbl.2008.0642
Eggert, H., Kurtz, J., and Diddens-de Buhr, M. F. (2014). Different effects of paternal transgenerational immune priming on survival and immunity in step and genetic offspring. Proc. R. Soc. B Biol. Sci. 281:2089. doi: 10.1098/rspb.2014.2089
Fanson, B. G., Weldon, Christopher, WTaylor, P. W., Pérez-Staples, D., and Simpson, S. J. (2009). Nutrients, not caloric restriction, extend lifespan in Queensland fruit flies (Bactrocera tryoni). Aging Cell 8, 514–523. doi: 10.1111/j.1474-9726.2009.00497.x
Galloway, L. F., and Etterson, J. R. (2007). Transgenerational plasticity is adaptive in the wild. Science 318, 1134–1136. doi: 10.1126/science.1148766
Garbutt, J. S., and Little, T. J. (2017). Bigger is better: changes in body size explain a maternal effect of food on offspring disease resistance. Ecol. Evol. 7, 1403–1409. doi: 10.1002/ece3.2709
Gluckman, P. D., and Hanson, M. A. (2004). Living with the past: Evolution, development, and patterns of disease. Science 2014:1095292. doi: 10.1126/science.1095292
Gluckman, P. D., Hanson, M. A., and Beedle, A. S. (2007). Early life events and their consequences for later disease: A life history and evolutionary perspective. Am. J. Hum. Biol. 19, 1–19. doi: 10.1002/ajhb.20590
Hancock, D. L., Hamacek, E. L., Lloyd, A. C., and Elson-Harris, M.M. (2000). The Distribution and Host Plants of Fruit Flies (Diptera: Tephritidae) in Australia. Brisbane: DPI Publications.
Kangassalo, K., Valtonen, T. M., Roff, D., Pölkki, M., Dubovskiy, I. M., Sorvari, J., et al. (2015). Intra- and trans-generational effects of larval diet on susceptibility to an entomopathogenic fungus, Beauveria bassiana, in the greater wax moth, Galleria mellonella. J. Evol. Biol. 28, 1453–1464. doi: 10.1111/jeb.12666
Kutzer, M. A. M., and Armitage, S. A. O. (2016). Maximising fitness in the face of parasites: a review of host tolerance. Zoology 119, 281–289. doi: 10.1016/j.zool.2016.05.011
Marshall, D. J., Cook, C. N., and Emlet, R. B. (2006). Offspring size effects mediate competitive interactions in a colonial marine invertebrate. Ecology 87, 214–225. doi: 10.1890/05-0350
Marshall, D. J., and Uller, T. (2007). When is a maternal effect adaptive? Oikos 116, 1957–1963. doi: 10.1111/j.2007.0030-1299.16203.x
Matzkin, L. M., Johnson, S., Paight, C., and Markow, T. A. (2013). Preadult Parental diet affects offspring development and metabolism in Drosophila melanogaster. PLoS One 8:e59530. doi: 10.1371/journal.pone.0059530
Miller, C. V. L., and Cotter, S. C. (2018). Resistance and tolerance: The role of nutrients on pathogen dynamics and infection outcomes in an insect host. J. Anim. Ecol. 87, 500–510. doi: 10.1111/1365-2656.12763
Mitchell, S. E., and Read, A. F. (2005). Poor maternal environment enhances offspring disease resistance in an invertebrate. Proc. R. Soc. B Biol. Sci. 272, 2601–2607. doi: 10.1098/rspb.2005.3253
Moadeli, T., Taylor, P. W., and Ponton, F. (2017). High productivity gel diets for rearing of Queensland fruit fly, Bactrocera tryoni. J. Pest Sci. 90, 507–520. doi: 10.1007/s10340-016-0813-0
Mousseau, T. A., and Fox, C. W. (1998). The adaptive significance of maternal effects. Trends Ecol. Evol. 13, 403–407. doi: 10.1016/S0169-5347(98)01472-4
Murren, C. J., Auld, J. R., Callahan, H., Ghalambor, C. K., Handelsman, C. A., Heskel, M. A., et al. (2015). Constraints on the evolution of phenotypic plasticity: Limits and costs of phenotype and plasticity. Heredity 115, 293–301. doi: 10.1038/hdy.2015.8
Öst, A., Lempradl, A., Casas, E., Weigert, M., Tiko, T., Deniz, M., et al. (2014). Paternal diet defines offspring chromatin state and intergenerational obesity. Cell 159, 1352–1364. doi: 10.1016/j.cell.2014.11.005
Pigliucci, M. (2001). Phenotypic Plasticity: Beyond nature and nurture: Syntheses in ecology and evolution. Baltimore: BaltimoreJohns Hopkins University Press.
Prasad, N. G., Shakarad, M., Rajamani, M., and Joshi, A. (2003). Interaction between the effects of maternal and larval levels of nutrition on pre-adult survival in Drosophila melanogaster. Evol. Ecol. Res. 5, 903–911.
Qvarnström, A., and Price, T. D. (2001). Maternal effects, paternal effects and sexual selection. Trends Ecol. Evol. 16, 95–100. doi: 10.1016/S0169-5347(00)02063-2
R Development Core Team (2011). R: A language and environment for statistical computing. Vienna: R Foundation for Statistical Computing.
Raubenheimer, D., Simpson, S. J., and Tait, A. H. (2012). Match and mismatch: Conservation physiology, nutritional ecology and the timescales of biological adaptation. Philos. Trans. R. Soc. B Biol. Sci. 367, 1628–1646. doi: 10.1098/rstb.2012.0007
Roth, O., Joop, G., Eggert, H., Hilbert, J., Daniel, J., Schmid-Hempel, P., et al. (2010). Paternally derived immune priming for offspring in the red flour beetle, Tribolium castaneum. J. Anim. Ecol. 79, 403–413. doi: 10.1111/j.1365-2656.2009.01617.x
Saastamoinen, M., Hirai, N., and van Nouhuys, S. (2013). Direct and trans-generational responses to food deprivation during development in the Glanville fritillary butterfly. Oecologia 171, 93–104. doi: 10.1007/s00442-012-2412-y
Soumya, K., Ganga Visalakshy, P. N., Krishnamoorthy, A., Kavitha, S. J., and Gopalakrishna Pillai, K. (2017). Host-age-dependent parasitism and reproductive success of Apanteles stantoni (Hymenoptera: Braconidae), a larval parasitoid of Diaphania indica (Lepidoptera: Pyralidae). Bio. Sci. Tech. 27, 70–80. doi: 10.1080/09583157.2016.1253829
Sutherst, R. W., Collyer, B. S., and Yonow, T. (2000). The vulnerability of australian horticulture to the queensland fruit fly, Bactrocera (Dacus) tryoni, under climate change. Aust. J. Agric. Res. 51, 467–480. doi: 10.1071/AR98203
Triggs, A. M., and Knell, R. J. (2012). Parental diet has strong transgenerational effects on offspring immunity. Funct. Ecol. 26, 1409–1417. doi: 10.1111/j.1365-2435.2012.02051.x
Uller, T., Nakagawa, S., and English, S. (2013). Weak evidence for anticipatory parental effects in plants and animals. J. Evol. Biol. 26, 2161–2170. doi: 10.1111/jeb.12212
Valtonen, T. M., Kangassalo, K., Pölkki, M., and Rantala, M. J. (2012). Transgenerational effects of parental larval diet on offspring development time, adult body size and pathogen resistance in Drosophila melanogaster. PLoS One 7:0031611. doi: 10.1371/journal.pone.0031611
Vijendravarma, R. K., Narasimha, S., and Kawecki, T. J. (2010). Effects of parental larval diet on egg size and offspring traits in Drosophila. Biol. Lett. 6, 238–241. doi: 10.1098/rsbl.2009.0754
West-Eberhard, M. J. (2003). Developmental Plasticity and Evolution. New York, NY: Oxford University Press.
Whitman, D., and Agrawal, A. (2009). What is phenotypic plasticity and why is it important?. New York, NY: Science Publishers, doi: 10.1201/b10201-2
Woestmann, L., and Saastamoinen, M. (2016). The importance of trans-generational effects in lepidoptera. Curr. Zool. 62, 489–499. doi: 10.1093/cz/zow029
Zajitschek, F., Zajitschek, S., and Manier, M. (2017). High-protein paternal diet confers an advantage to sons in sperm competition. Biol. Lett. 13:20160914. doi: 10.1098/rsbl.2016.0914
Zanchi, C., Troussard, J. P., Martinaud, G., Moreau, J., and Moret, Y. (2011). Differential expression and costs between maternally and paternally derived immune priming for offspring in an insect. J. Anim. Ecol. 80, 1174–1183. doi: 10.1111/j.1365-2656.2011.01872.x
Zirbel, K., Eastmond, B., and Alto, B. W. (2018). Parental and offspring larval diets interact to influence life-history traits and infection with dengue virus in Aedes aegypti. R. Soc. Open Sci. 5:180539. doi: 10.1098/rsos.180539
Keywords: transgenerational effects, parental diet, Serratia marcescens, offspring, development, disease resistance
Citation: Dinh H, Nguyen B, Morimoto J, Lundback I, Kumar SS and Ponton F (2021) Transgenerational Effects of Parental Diet on Offspring Development and Disease Resistance in Flies. Front. Ecol. Evol. 9:606993. doi: 10.3389/fevo.2021.606993
Received: 16 September 2020; Accepted: 03 June 2021;
Published: 28 June 2021.
Edited by:
Dalial Freitak, University of Graz, AustriaReviewed by:
Nora Kristin Elisa Schulz, Vanderbilt University, United StatesJoël Meunier, UMR 7261 Institut de recherche sur la biologie de l’insecte (IRBI), France
Copyright © 2021 Dinh, Nguyen, Morimoto, Lundback, Kumar and Ponton. This is an open-access article distributed under the terms of the Creative Commons Attribution License (CC BY). The use, distribution or reproduction in other forums is permitted, provided the original author(s) and the copyright owner(s) are credited and that the original publication in this journal is cited, in accordance with accepted academic practice. No use, distribution or reproduction is permitted which does not comply with these terms.
*Correspondence: Fleur Ponton, ZmxldXIucG9udG9uQG1xLmVkdS5hdQ==