- 1Animal Ecology, J. F. Blumenbach Institute of Zoology and Anthropology, University of Göttingen, Göttingen, Germany
- 2Soil Zoology and General Entomology, A. N. Severtsov Institute of Ecology and Evolution, Russian Academy of Sciences, Moscow, Russia
- 3Acarology, Tyumen State University, Tyumen, Russia
- 4Department of Soil Sciences and Land Resources, Faculty of Agriculture, Bogor Agricultural University (IPB), Bogor, Indonesia
- 5Department of Silviculture, Faculty of Forestry, Bogor Agricultural University (IPB), Bogor, Indonesia
- 6Centre of Biodiversity and Sustainable Land Use, Göttingen, Germany
Land-use change is threatening biodiversity worldwide and is predicted to increase in the next decades, especially in tropical regions. Most studies focused on the response of single or few species to land-use change, only few investigated the response of entire communities. In particular the response of belowground communities to changes in land use received little attention. Oribatid mites (Oribatida, Acari) are among the most abundant soil animals, involved in decomposition processes and nutrient cycling. Oribatid mite species span a wide range of trophic niches and are known to sensitively respond to changes in land use. Here, we investigated shifts in the community-level trophic niche of oribatid mites with the conversion of rainforest into rubber and oil palm plantations. Due to a wider range of resources in more natural ecosystems, we expected the community-level trophic niche to shrink with conversion of rainforest into plantations. As the conversion of rainforest into plantations is associated with reduced availability of litter resources, we expected the average trophic level (indicated by the 15N/14N ratio) to be higher and basal resources (indicated by the 13C/12C ratio) to shift toward living plant material in rubber and oil palm plantations. Our analysis showed that community-level trophic niches in rainforest and rubber agroforest (“jungle rubber”) were separated from those in monoculture plantation systems, indicating a trophic niche shift with land-use intensification. As hypothesized, oribatid mites shifted their diet toward predation and/or scavenging and toward the plant-based energy channel with transformation of rainforest into plantations. Exceptionally low minimum 13C/12C ratios in rubber plantations suggest that certain oribatid mite species in this land-use system use resources not available in the other studied ecosystems. We detected high isotopic uniqueness in oil palm plantations suggesting a low trophic redundancy and thus high vulnerability of trophic functioning in this system in comparison to rainforest. Overall, the results suggest that the conversion of rainforest into plantations is associated with pronounced shifts in community-level trophic niches of mesofauna detritivores with potential major consequences for the functioning of the decomposer system.
Introduction
The niche concept forms the basis of concepts explaining the coexistence of species (Hutchinson, 1959; Chase and Leibold, 2003). There are two different niche-related processes, i.e., environmental filtering, assuming that the assemblage of communities is based on the similarity of niches of the species coexisting locally, and niche differentiation resulting from competition among species (MacArthur and Levins, 1967; Vellend, 2010; Violle et al., 2011; Kraft et al., 2015). At stable environmental conditions long-term coexistence of species results in distinct niches of the coexisting species and in predictable community composition with pronounced niche differentiation among coexisting species (Giller, 1996). By contrast, in fragmented habitats or in systems with fluctuating environmental conditions, communities are likely to be assembled at least in part at random, and therefore niches of species may overlap (Giller, 1996; Chesson, 2000; Caruso et al., 2012). Trophic niches define the impact of one species on other species and ecosystem processes and therefore are closely related to the role of species in ecosystems (Korotkevich et al., 2018). The complement of trophic niches of coexisting species defines the community-level trophic niche, which is likely to differ between ecosystems due to species turnover and changes in trophic niches of individual species (Tilman, 2004; Salles et al., 2009). Species with broader trophic niches likely are able to better cope with disturbances, such as changes in land use or invading species, compared to species with narrower niches (Bommarco et al., 2010; Dammhahn et al., 2017). By contrast, species with narrower niches are more likely to go locally extinct if environmental conditions and the availability of food resources change (Gan et al., 2014). These changes in community-level trophic niches are likely to be associated with changes in the functionality of communities.
Mechanisms responsible for diversity—ecosystem functioning relationships are based on the niches of species and their functional space (Tilman, 2001; Adler and Bradford, 2002). In this approach, the community-level trophic niche can be described by using diversity indices. In particular, functional diversity, including functional richness, functional evenness and functional divergence, have been shown to be closely linked to ecosystem functioning (Hulot et al., 2000; Pearson, 2001; Bremner et al., 2003) and these indices can be applied to describe trophic niches (Cucherousset and Villéger, 2015). For example, if functional richness of trophic niches is low, part of the available resources remain unused by the local community, resulting in low productivity (Petchey, 2003). By contrast, increased ecosystem functioning is based on high functional richness, coupled with niche differentiation leading to efficient use of resources (Mason et al., 2005). This also applies to multitrophic communities, i.e., trophic complementarity across trophic levels in food webs is positively related to ecosystem functioning (Poisot et al., 2013). Along with other functional indices, trophic complexity of communities is expected to decline in disturbed ecosystems, as was shown for springtails in the temperate zone (Korotkevich et al., 2018). However, this pattern has not been tested in other soil taxa and other regions.
The global demand for food, fuel and fiber is associated with the transformation and degradation of ecosystems, and this is increasing rapidly (Sodhi et al., 2010; Wilcove et al., 2013; Meijide et al., 2018). Especially tropical regions are suffering from transformation and degradation (Sodhi et al., 2010; Wilcove et al., 2013; Meijide et al., 2018). Tropical forest ecosystems, characterized by the highest biodiversity and highest level of endemism worldwide, are shrinking and are transformed into intensively managed monoculture plantations at increasing rates (Myers et al., 2000; Sodhi et al., 2010; Drescher et al., 2016). Biodiversity in plantations typically is strongly reduced compared to rainforests due to reduced diversity of trees as well as more pronounced fluctuating environmental conditions (Wilcove and Koh, 2010). Reduced diversity in plantations may impair the functioning of these systems and this at least in part is likely to be due to changes in trophic interactions and community-level trophic niches (Potapov et al., 2019a).
Only little is known on the effect of land-use change in tropical regions on soil organisms responsible for major ecosystem functions such as decomposition processes and nutrient cycling, and this applies in particular to soil mesofauna such as oribatid mites (Maraun and Scheu, 2000; Bardgett, 2005). Oribatid mites span over about four trophic levels and include trophic guilds of lichen feeders, fungal feeders, primary and secondary decomposers as well as predators/scavengers (Schneider et al., 2004; Illig et al., 2005; Maraun et al., 2011). Due to their high diversity and wide range of trophic niches oribatid mites are an ideal model taxon for studying effects of land-use change on community functioning.
Natural variations in 15N/14N and 13C/12C ratios are increasingly used for characterizing trophic niches of soil animals and provide insight into trophic levels, basal resources as well as the trophic structure of entire communities (Tiunov, 2007; Potapov et al., 2019b). Animal tissue is enriched by about 3.4‰ per trophic level in 15N, allowing to study changes in the trophic position of species with environmental changes (DeNiro and Epstein, 1978; Post, 2002; Pollierer et al., 2009; Potapov et al., 2019b). In contrast to 15N, 13C is little enriched in consumers compared to their diet, allowing to trace basal food resources in food webs (DeNiro and Epstein, 1978; Spence and Rosenheim, 2005; Pollierer et al., 2009; Potapov et al., 2019b). Stable isotopes have been used to analyze trophic niches of a wide range of soil invertebrates (Scheu and Falca, 2000; Halaj et al., 2005; Maraun et al., 2007, 2011; Tiunov, 2007). However, they rarely have been used to investigate how changes in trophic niches of soil organisms are affected by changes in land use (but see Lagerlöf et al., 2017; Minor et al., 2017; Korotkevich et al., 2018). Additionally, previous studies based on stable isotopes did not account for abundance and/or biomass of the studied species, i.e., all species were assumed to have the same importance or impact (Layman et al., 2007; Jackson et al., 2011). Novel techniques allow accounting for these deficiencies but have not been applied to soil communities (Cucherousset and Villéger, 2015).
The current study formed part of the interdisciplinary project “Ecological and socioeconomic functions of tropical lowland rainforest transformation systems” (EFForTS), established in Jambi Province, southwest Sumatra, Indonesia (Drescher et al., 2016). In the framework of this project, it has been shown that plants as well as invertebrates above and below the ground strongly decline with the conversion of rainforest into rubber and oil palm plantations (Grass et al., 2020). In particular, the density of oribatid mites declined by ca. 20% and species richness by ca. 60% (D. Sandmann, unpubl. data). Here we focused on changes in the trophic structure of oribatid mite communities with conversion of rainforest into plantation systems by measuring natural variations in 15N/14N and 13C/12C ratios. Four land-use systems, widespread across Southeast Asia were investigated, i.e., rainforest, rubber agroforest (“jungle rubber”), rubber and oil palm monoculture plantations. We focused on the niche structure and overall niche position at the level of communities rather than on the changes in trophic niches of individual species, which were addressed in an earlier study (Krause et al., 2019). We hypothesized (1) that the community-level trophic niche of oribatid mites is larger in more natural ecosystems, such as rainforest and jungle rubber, than in intensively managed agricultural systems, such as rubber and oil palm plantations. Further, we hypothesized (2) that the community-level trophic niche of oribatid mites is shifted in intensively managed agricultural systems toward living plant-based resources due to lower availability of litter resources and more open canopy allowing more pronounced growth of understory plants and algae. In addition, we hypothesized (3) that functional redundancy in rainforest is higher compared to plantation systems due to more species rich oribatid mite communities.
Materials and Methods
Sampling Sites
Samples were taken in two landscapes of Jambi Province, Bukit Duabelas (2° 0′ 57″ S, 120° 45′ 12″ E) and Harapan (1° 55′ 40″ S, 103° 15′ 33″ E), located about 40 km from each other. In each landscape, four different land-use systems were investigated: rainforest, jungle rubber, rubber and oil palm plantations (Drescher et al., 2016). Rainforest sites were “primary degraded forests” (as classified by Margono et al., 2014) and had been selectively logged at least once. Jungle rubber sites were established by planting rubber trees (Hevea brasiliensis) into rainforest and contain rainforest tree species. Jungle rubber sites represent low intensive land-use systems, lacking fertilizer input as well as herbicide application. By contrast, rubber and oil palm (Elaeis guineensis) monocultures represent high land-use intensity plantation systems managed by the addition of fertilizers as well as herbicides. Oil palm plantations differ from those of rubber, among others, in that fronds of oil palms are cut regularly for fruit harvesting and piled up in rows, whereas in rubber leaves are shed annually at the end of the dry season and form a litter layer which is virtually lacking in oil palm plantations. More details on the study site are given in Drescher et al. (2016). For each of the four land-use systems four replicates were established in each of the two landscapes, resulting in a total of 32 plots. The two landscapes were spaced by ca. 80 km and the replicate plots per land-use system within landscapes by 2–40 km. Each plot was positioned within a larger plantation or forest patch, spanned 50 × 50 m and included three randomly placed subplots of 5 × 5 m. Subplots were spaced by a minimum of 5 m from the plot edges to avoid edge effects. More details on the location of the study sites and their characteristics are given in Drescher et al. (2016).
Sampling, Extraction, and Species Determination
In October 2013, randomly placed samples (16 × 16 cm; including the litter layer and 0–5 cm of the mineral soil) were taken from each of the subplots, i.e., a total of 96 samples. The samples were transported to the laboratory and extracted in a heat gradient (Kempson et al., 1963). Determination of oribatid mites was done to species/morphospecies level using Balogh and Balogh (2012). Species and morphospecies were documented by pictures, linked with morphological traits and species identification numbers (species ID), and included into the Ecotaxonomy database1. Until further analysis, species were stored in 70% ethanol. For calibration of animal stable isotope values mixed litter samples were taken from each subplot (Klarner et al., 2017).
Stable Isotope Analysis
For each land-use system in the two landscapes (Bukit Duabelas and Harapan) species for stable isotope analysis were selected based on their relative abundance; for each land-use system species representing 80% of total oribatid mite individuals were analyzed (Supplementary Table 1). These species were assumed to represent the functional “core” of the community of oribatid mites of the respective land-use system. Three individuals (if available) were pooled for one sample (sample weight 0.02–0.19 mg). In total, 367 samples of 117 species contributing to 80% of the individuals were analyzed (out of the total of 220 species occurring at the study sites; D. Sandmann, unpubl. data).
Prior to stable isotope analysis samples were dried at 60°C for 24 h. Litter samples were ground in a ball mill (Retsch Mixer Mill MM200, Haan, Germany). Samples were analyzed using a coupled system of an elemental analyzer (NA 1500, Carlo Erba, Milan, Italy) and a mass spectrometer (MAT 251, Finnigan, Bremen, Germany) adapted for the analysis of small sample sizes (Langel and Dyckmans, 2014). Stable isotope abundance (δX) was expressed using the δ notation with δX (‰) = (Rsample–Rstandard)/Rstandard × 1000, with X representing the target isotope (15N or 13C) and Rsample and Rstandard the respective target isotope ratio (15N/14N or 13C/12C). As standard for 13C Vienna PD Belemnite and for 15N atmospheric nitrogen were used. Acetanilid was used for internal calibration.
Means of δ13C and δ15N values of litter samples from all subplots were used for calibration of stable isotope values of oribatid mites of the respective plot following Klarner et al. (2014). Calibrated δ13C and δ15N values were calculated as difference between the plot-specific litter δ13C and δ15N values, and the δ13C and δ15N values of oribatid mite species, and given as Δ13C and Δ15N values, respectively. Calibrated values were used to ascribe oribatid mites to trophic levels including primary decomposers, secondary decomposers/fungal feeders and predators/scavengers based on Maraun et al. (2011) assuming an enrichment of 15N by 3.4‰ per trophic level except for primary decomposers, which were assumed to be only enriched in 15N by a maximum of 1.7‰ compared to litter (Vanderklift and Ponsard, 2003; Potapov et al., 2019b). Calibrated Δ13C and Δ15N values were used for statistical analyses. All data manipulations were done in R v 3.5.2 (R Core Team, 2018) with R studio interface (RStudio, Inc.).
Statistical Analysis
For characterizing the trophic structure of communities we calculated “isotopic metrics” (Layman et al., 2007; Cucherousset and Villéger, 2015). These metrics describe the position of species of a studied community within two-dimensional space, represented by δ15N and δ13C values. Cucherousset and Villéger (2015) further developed metrics based on the ones from Layman et al. (2007), by merging them with the functional diversity metrics suggested by Villéger et al. (2008) and Laliberté and Legendre (2010).
We used the R script provided by Cucherousset and Villéger (2015) to scale two stable isotope axes and calculate isotopic metrics for each community (Figure 1). Δ13C and Δ15N values were scaled between 0 and 1 based on maximum and minimum across communities to ensure equal contribution of two isotopes in the multidimensional metrics calculation (Cucherousset and Villéger, 2015). Before calculating community mean values and multidimensional metrics, species were weighted according to their contribution to the total community biomass per plot and therefore, those metrics were adjusted according to the functional relevance of the species (Cucherousset and Villéger, 2015). To calculate biomasses of species, individual body masses of all mite species were calculated from individual measurements of body length and width (D. Sandmann, unpubl. data) using the allometric regression log P = 1.53 × log L + 1.53 × log l− 6.67, with P body mass, L body length and l body width (Lebrun, 1971). Overall, thirteen isotopic metrics were calculated for each of 32 communities (i.e., sampling plots). First, we calculated single-dimensional metrics separately for Δ13C and Δ15N values—minimum, maximum, range and biomass-weighted mean. Maximum and minimum Δ13C and Δ15N values were represented by the species with the most extreme values within the community. The range represented the difference between minimum and maximum Δ13C and Δ15N values. Second, we calculated five multidimensional metrics including isotopic divergence, isotopic dispersion, isotopic evenness, isotopic uniqueness and isotopic richness, all weighted by biomass of species (Cucherousset and Villéger, 2015). Isotopic divergence represents the distance between all species and the center of the convex hull area. Isotopic divergence values close to 0 indicate that species with the most extreme stable isotope values, e.g., lichen-feeders and predators, are rare, whereas isotopic divergence values close to 1 indicate that species with the most extreme stable isotope values dominate. Isotopic dispersion combines the convex hull area with isotopic divergence values and can be interpreted as scaled multidimensional variance. Isotopic dispersion approaches 1 when species with contrasting stable isotope values have similar abundance (for instance, primary and high-level consumers are similarly abundant), whereas it approaches 0 when most biomass is positioned near the “center of gravity” of the community. Isotopic evenness, in contrast to the first two metrics, which do not consider the distance between species, quantifies the distribution of species in stable isotope space. Isotopic evenness values close to 1 indicate that the isotope values of the species of the studied community are evenly distributed. By contrast, isotopic evenness values close to 0 indicate that most stable isotope values of the studied species cluster together (for instance, all species are herbivores or predators, but no decomposers present). Isotopic uniqueness is defined as the inverse of the average isotopic redundancy, which reflects the closeness of stable isotope values of the studied species within the community. Isotopic uniqueness approaches 0 if stable isotope values of species within the community are similar indicating that most of the species have another isotopically similar species within this community. By contrast, isotopic uniqueness is close to 1 if stable isotope values of species differ within communities indicating that many species have no other isotopically similar species within this community. Finally, isotopic richness represents the isotopic space (convex hull area) filled by the community, i.e., the functional richness. Functional richness is scaled between 0 and 1 using the volume occupied by all studied species across sites as the maximum (Mason et al., 2005; Villéger et al., 2008).
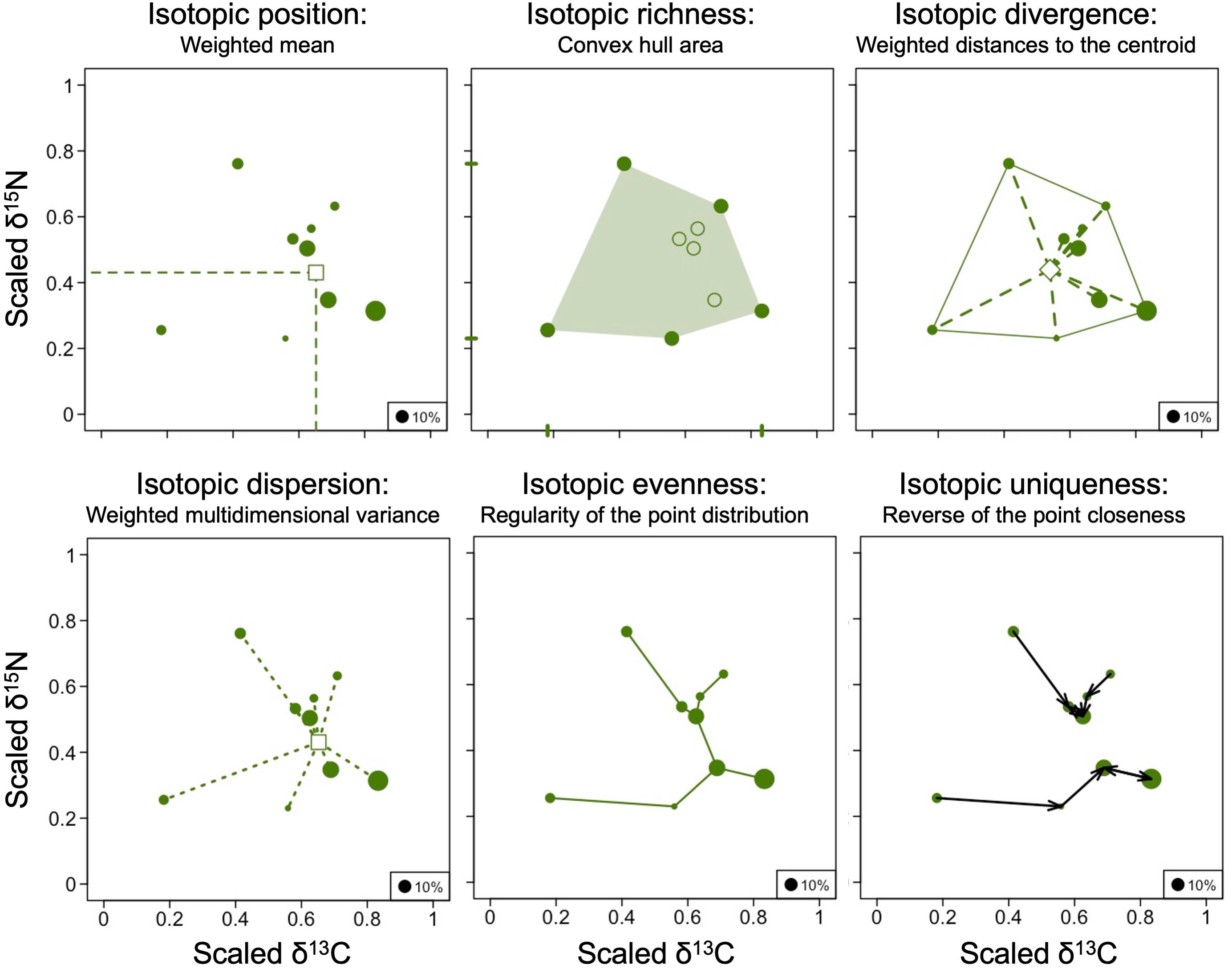
Figure 1. Calculated isotopic metrics. For the analysis, Δ13C and Δ15N values of species in each community were scaled between 0 and 1 and their distribution in isotopic space was analyzed with biomass-weighted isotopic metrics (see text for details). An example of metrics for one community (HR4) is given; eight such communities were analyzed for each of the four land-use systems.
Data were analyzed using the aov (analysis of variance) function with landscape and land-use system as factors followed by post hoc comparison of means using the HSD.test function to inspect differences between land-use systems. Results were displayed using the ggplot2 package (Wickham, 2016). The baseline was set to zero. Points on all figures in the main text represent communities, and not species or individuals.
Results
One-Dimensional Metrics
The weighted means of Δ13C and Δ15N values (isotope positions) across oribatid mite communities separated the two more natural land-use systems (rainforest and jungle rubber) from the two monoculture plantations (Figure 2). Communities of rainforest and jungle rubber overlapped widely in their isotopic positions; the same was true for oil palm and rubber plantations. The average community positions of both Δ13C and Δ15N values varied significantly between the land-use systems [F(3,27) = 7.09, p < 0.001 for Δ13C and F(3,27) = 7.93, p = < 0.001 for Δ15N; Figures 3A–E]. Average community positions of Δ13C were similar in rainforest and jungle rubber, but significantly lower in rubber and oil palm plantations, on average by 1.58‰ (Figure 3A and Supplementary Table 2). By contrast, average community positions of Δ15N in rubber and oil palm plantations in trend were higher than in jungle rubber, on average by 1.79‰, and significantly higher than in rainforest, on average by 3.03‰ (Figure 3E and Supplementary Table 2).
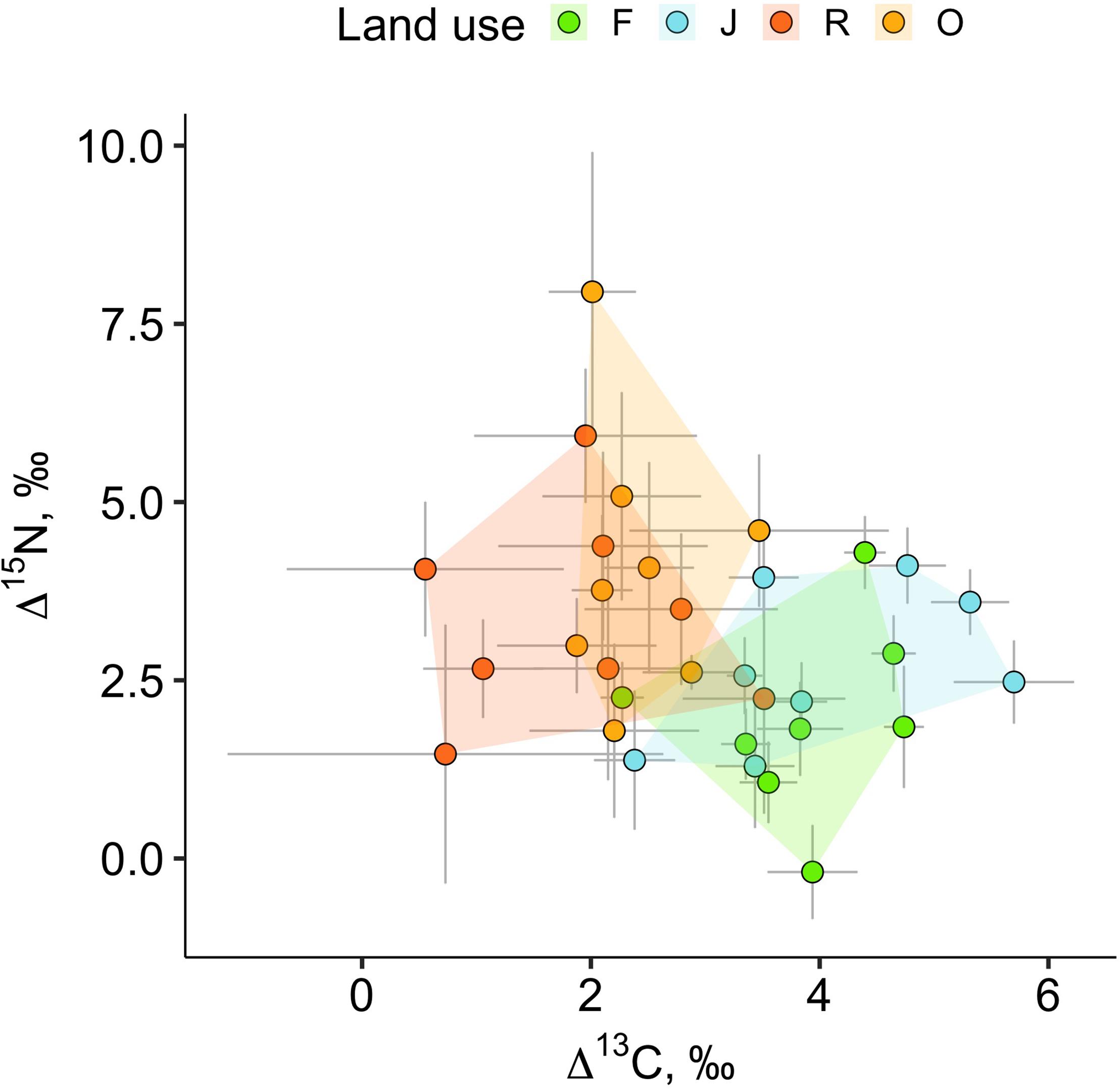
Figure 2. Weighted means of Δ13C and Δ15N values of oribatid mite communities in rainforest (green), jungle rubber (blue), rubber (red) and oil palm plantations (yellow) (eight replicates each). Each point represents a weighted mean (isotopic position) for one community, error bars represent standard errors; 32 communities were analyzed.
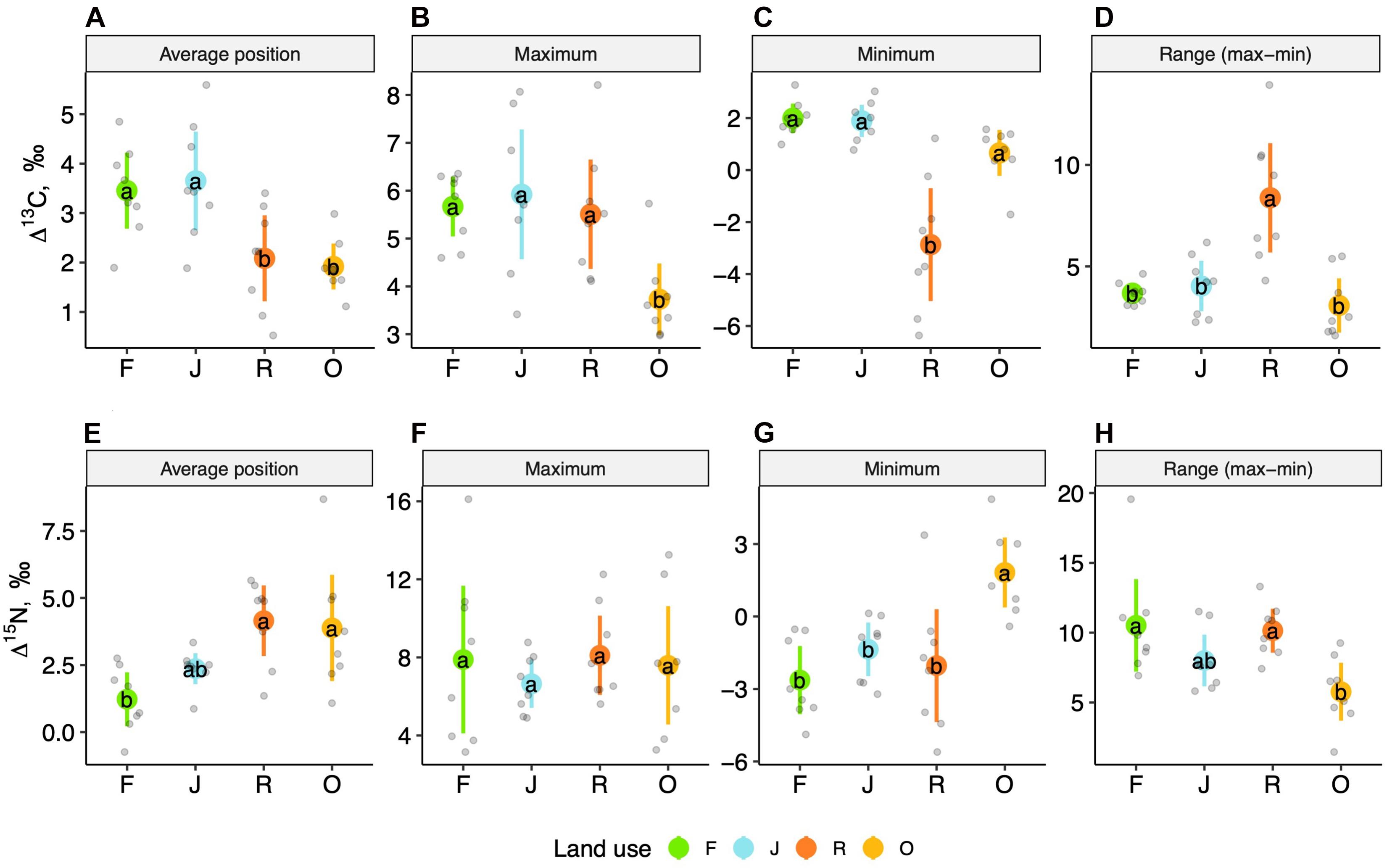
Figure 3. One-dimensional metrics for Δ13C (upper panel) and Δ15N values (lower panel) of oribatid mite communities in rainforest (F, green), jungle rubber (J, blue), rubber (R, red) and oil palm plantations (O, yellow). Average position (A,E), maximum (B,F), minimum (C,G), and range between minimum and maximum (D,H); means (circles) and confidence intervals. Each point represents one community. For the calculation of the metrics see section “Materials and Methods” and Supplementary Table 2. For the calculation of the average positions, species were weighted according to their contribution to the total community biomass per plot. Means, sharing the same letter are not significantly different (Tukey’s HSD test, P < 0.05).
Maximum Δ13C values, but not maximum Δ15N values, varied significantly with land-use system [F(3,27) = 7.22, p = 0.001 for Δ13C and F(3,27) = 0.41, p = 0.74 for Δ15N] (Figures 3B,F). Maximum Δ13C values were similar in rainforest, jungle rubber and rubber plantations, but significantly lower in oil palm plantations, on average by 2‰ (Figure 3B, Supplementary Table 2, and, Supplementary Figure 1D). Further, minimum Δ13C and Δ15N values varied significantly with land-use system [F(3,27) = 19.51, p < 0.001 for Δ13C and F(3,27) = 10.56, p ≤ 0.001 for Δ15N]. Minimum Δ13C were similar in rainforest, jungle rubber and oil palm plantations, but significantly lower in rubber plantations, on average by 4.46‰. Some oribatid mite species in rubber plantations were depleted in 13C relative to the litter up to 6‰ (Supplementary Figure 1C). By contrast, minimum Δ15N values were similar in rainforest, jungle rubber and rubber plantations, being significantly higher in oil palm plantations, on average by 4.15‰ (Figure 3G and Supplementary Table 2). Rainforest and rubber plantations harbored species with 15N values lower than those of litter (Supplementary Figures 1A,C).
Similar to the previous metrics, the range between maximum and minimum Δ13C and Δ15N values varied significantly between land-use systems [F(3,27) = 16.31 p = 0.005 for Δ13C and F(3,27) = 5.18 p = 0.006 for Δ15N]. The range in Δ13C values was similar in rainforest, jungle rubber and oil palm plantations, but significantly higher in rubber plantations, on average by 4.87‰ (Figure 3D and Supplementary Table 2). The range in Δ15N values was similar in rainforest, jungle rubber and rubber plantations, but was significantly lower in oil palm plantations, on average by 4.94‰ (Figure 3H and Supplementary Table 2). As indicated by 15N values, rainforest, jungle rubber and rubber plantations harbored species spanning over three trophic levels including primary and secondary decomposers as well as predators (Supplementary Figures 1A–C). By contrast, oil palm plantations lacked primary decomposers and only comprised secondary decomposers and predators (Supplementary Figure 1D).
Multidimensional Metrics
Isotopic dispersion, isotopic divergence and isotopic evenness did not differ significantly between the four land-use systems (Figures 4A–C) [F(3,27) = 1.65, p = 0.201, F(3,27) = 0.46, p = 0.714, and F(3,27) = 1.53, p = 0.228, respectively]. By contrast, isotopic richness varied significantly between the land-use systems [F(3,27) = 8.1, p < 0.001]; it was similar in rainforest and jungle rubber, in trend lower in oil palm plantations and highest in rubber plantations, on average by 0.11 (scaled value; Figure 4D and Supplementary Table 3). Further, isotopic uniqueness increased slightly from rainforest to jungle rubber and rubber plantations, and was significantly higher in oil palm plantations than in rainforest, on average by 0.3 [F(3,27) = 5.33, p = 0.005; Figure 4E and Supplementary Table 3]. For detailed information on plot level metrics values see Supplementary Figures 2A–D.
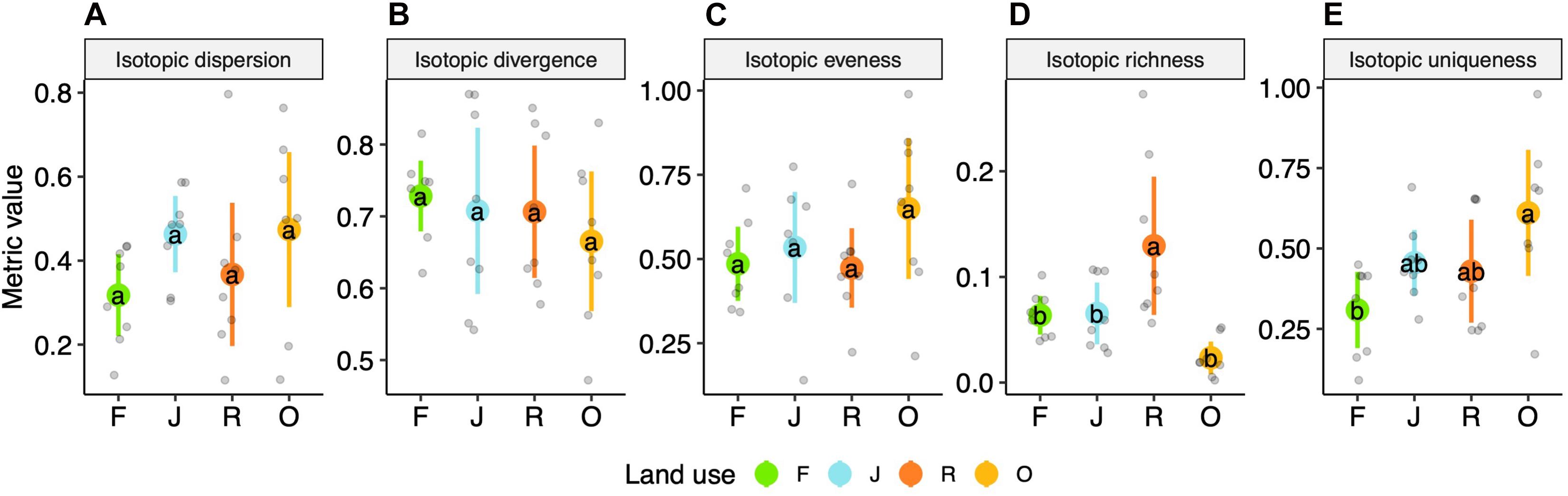
Figure 4. Multidimensional isotopic metrics of oribatid mite communities of four land-use systems, rainforest (F, green), jungle rubber (J, blue), rubber (R, red) and oil palm plantations (O, yellow)—(A) isotopic dispersion, (B) isotopic divergence, (C) isotopic evenness, (D) isotopic richness and (E) isotopic uniqueness; means (circles) and confidence intervals. Each point represents one community. For calculations see section “Materials and Methods” and Supplementary Table 3. For the calculation of the metrics, species were weighted according to their contribution to the total community biomass per plot. Means sharing the same letter are not significantly different (Tukey’s HSD test, P < 0.05).
Discussion
We investigated effects of land-use change on the trophic organization of oribatid mite communities as model taxon of soil invertebrates in the decomposer food web. The study adds to the few existing studies investigating variations in community trophic niches and energy fluxes in soil (Korotkevich et al., 2018; Kühn et al., 2018; Susanti et al., 2019). Our study represented species accounting for 80% of total oribatid mite individuals on each site and thus are likely to represent key functional changes of the entire community. The results are generally supporting our hypotheses and indicate that (i) the community niche of oribatid mites differs most between more natural systems (rainforest and jungle rubber) and monoculture plantation systems (rubber and oil palm plantations), and that communities in oil palm plantations have shorter trophic chains and lower trophic redundancy than in other systems. In addition, we found that in rubber monoculture plantations oribatid mites use basal resources not existing in any of the other studied land-use systems.
One-Dimensional Metrics
Generally, both Δ13C and Δ15N values were similar in rainforest and jungle rubber and distant from those in oil palm and rubber plantations, indicating that the trophic structure of soil food webs changes strongly with land-use change from natural systems or extensive agricultural systems to intensively managed agricultural systems. This supports findings of Krause et al. (2019) who analyzed six individual oribatid mite species and showed that trophic niches shifted most from rainforest to monoculture plantation systems, reflecting that oribatid mites are able to respond in a plastic way to changes in resource supply at the species level. Here, we showed changes in both the use of basal resources as well as trophic positions of the entire oribatid mite communities. Using fatty acids as trophic markers Susanti et al. (2019) found changes in the flux of energy through soil food webs with the conversion of rainforest into rubber and oil palm plantations. Changes in energy channels with land-use intensity suggest that soil fauna communities in general are able to respond in a flexible way to changes in the availability of resources and environmental conditions. In rainforest, energy in soil food webs is channeled mainly to higher trophic levels via the bacterial energy channel, whereas in oil palm plantations the plant-based channel is more important (Susanti et al., 2019). Furthermore, generalist predators such as centipedes (Chilopoda) have been shown to switch from decomposer prey to other prey, predominantly herbivore species, in monoculture plantations, presumably due to reduced or lacking litter layer in the latter two systems (Klarner et al., 2017). The amount of leaf litter in the litter layer in natural ecosystems typically is higher than in plantation systems (Hyodo et al., 2015), and this also applies to the ecosystems investigated in the present study (Krashevska et al., 2017). In our study the litter layer in oil palm plantations was lacking or poorly developed, but a dense herb layer of mostly introduced weeds was present (Drescher et al., 2016). Similar to the results of Klarner et al. (2017) and Susanti et al. (2019) the shift toward lower Δ13C values in oribatid mite communities in our study indicates that conversion of rainforest into plantation systems is associated with the strengthening of the plant-based energy channel in plantation systems (lower “detrital shift”; Pollierer et al., 2009; Potapov et al., 2019b). By contrast, high Δ13C values in rainforest and jungle rubber indicate that soil food webs in the more natural systems are predominantly based on microbial energy channels (Potapov et al., 2013, 2019b). Oribatid mite communities in oil palm plantations lack species with high Δ13C and low Δ15N values, reflecting that the primary decomposers among microarthropods are absent or strongly reduced. Earlier studies from Ecuadorian montane forests also showed that in some tropical ecosystems primary decomposers may be scarce or lacking (Illig et al., 2005). In these ecosystems, the trophic niche of oribatid mites likely shifted from feeding on detritus to scavenging or predation, e.g., by feeding on nematodes (Heidemann et al., 2011, 2014).
Minimum Δ13C values of oribatid mite communities were significantly lower (reflecting one species with the most extreme values from the community) in rubber plantations (up to 6‰) than in the other three land-use systems. In fact, four species in rubber plantations, i.e., cf. Trachyoribates cf. florens, cf. Rostrocetes cf. shibai, Cyrthermannia tuberculata and Eremulus densus, had δ13C values <−32‰, indicating that oribatid mites in rubber plantations use some basal resources with exceptionally low δ13C values not available in the other land-use systems. Such low δ13C values are unique and have never been observed before in soil invertebrates (Potapov et al., 2019b). The pattern in part might be explained by the use of carbon from understory plants and algae, which assimilated 13C-depleted CO2 originating from decomposing organic matter (canopy effect; Van der Merwe and Medina, 1991). However, this is unlikely to explain the extreme difference in minimum Δ13C values of oribatid mite communities between rubber plantations and the other land-use systems studied as neither the abundance of algae nor of understory plants is higher in rubber monoculture plantations than in the other land-use systems studied (Rembold et al., 2017; Schulz et al., 2019; Susanti et al., 2019). Another possibility might be that low Δ13C values of oribatid mite communities reflect the use of plant compounds depleted in 13C such as lignin (Bowling et al., 2008; Pollierer et al., 2009). However, the use of lignin as carbon source is also unlikely since—with very few exceptions—animals are unable to digest lignin (Berg and Ryszard, 2005). Future studies are needed to clarify the low Δ13C values of oribatid mites in rubber plantations. Due to the very low minimum Δ13C values in oribatid mites of rubber plantations the range of Δ13C values and isotopic richness were significantly higher compared to the other three land-use systems. Very high 13C values in jungle rubber and rubber such as in Plonaphacarus kugohi presumably were due to the incorporation of calcium carbonate in their exoskeleton (Norton and Behan-Pelletier, 1991; Maraun et al., 2011; Pachl et al., 2012).
Multidimensional Metrics
Isotopic dispersion, isotopic divergence and isotopic evenness did not differ significantly between the four land-use systems indicating that the oribatid mite communities in the studied land-use systems are rather balanced and the niche differentiation, on average, differed little. Presumably, at least in part this reflects the ability of oribatid mite species to adapt to local resources (Krause et al., 2019). In contrast to the three metrics above, isotopic richness was significantly higher in rubber plantations than in the three other land-use systems studied, confirming the results of isotopic ranges due to the use of resources depleted in 13C (see above).
Isotopic uniqueness in oil palm plantations exceeded that in the other three land-use systems, with jungle rubber and rubber plantations being intermediate between oil palm plantations and rainforest. High isotopic uniqueness indicates that all abundant species in the community have very different stable isotope values, e.g., species are “unique” in their trophic niche (Cucherousset and Villéger, 2015). High values in oil palm plantations and low values in rainforest therefore suggest low functional redundancy in the former and high functional redundancy in the latter. This indicates that further loss of species is more likely to be associated with a loss of functions in oil palm plantations than in rainforest.
Conclusion
Our results document that oribatid mites in tropical ecosystems occupy a wide range of trophic niches allowing insight into changes in the structure and functioning of decomposer systems with changes in land use. Changes in community-level trophic niches with conversion of rainforest into plantation systems were due to both changes in trophic position (Δ15N values) as well as changes in the use of basal resources (Δ13C values). Notably, changes in the use of basal resources were more prominent and consistent than those in trophic positions, indicating that land use mainly affects the use of basal resources of soil microarthropods. Oribatid mite communities in both rubber and oil palm monocultures rely more heavily on plant-based resources and lack primary decomposers, whereas communities in rainforest and jungle rubber are based predominantly on microorganisms and litter-based resources. Oribatid mite communities in rubber plantations are using carbon resources not existing in the other land-use systems studied, but the identity of these resources remains unknown. High isotopic uniqueness in oil palm plantations suggests that in particular these plantations are susceptible to losses of function with the loss of species. Overall, the results document that conversion of rainforest into plantation systems is associated with pronounced shifts in community-level trophic niches of soil microarthropods, which are likely associated with changes in the functioning of the decomposer system and the channeling of energy through the decomposer food web.
Data Availability Statement
The datasets presented in this study can be found in Supplementary Material. Morphospecies descriptions are available from ecotaxonomy.org.
Author Contributions
AK and SS led the manuscript writing. AP, RW, NH, DS, and MM contributed to the manuscript writing. AP designed the data analysis. AK and AP analyzed the data. DS determined the oribatid mite species. SE validated the species determination. SS and MM designed the experiment and were the scientific supervisors of the experiment. All authors contributed to the article and approved the submitted version.
Funding
This study was funded by the Deutsche Forschungsgemeinschaft (DFG, German Research Foundation)—project no. 192626868—SFB 990 in the framework of the collaborative German—Indonesian research project CRC990. Work of AP was supported by the Russian Science Foundation (project 19-74-00154). The study was conducted based on the research permits no. 332/SIP/FRP/SM/IX/2012, 389/SIP/FRP/SM/X/2013 and 145/SIP/FRP/SM/V/2013 issued by the State Ministry of Research and Technology of the Republic of Indonesia (RISTEK), and the collection permit no. S.07/KKH-2/2013 issued by the Ministry of Forestry of the Republic of Indonesia. Sample export for analysis in Germany was based on permit no. 125/KKH-5/TRP/2014 issued by the Ministry of Forestry of the Republic of Indonesia.
Conflict of Interest
The authors declare that the research was conducted in the absence of any commercial or financial relationships that could be construed as a potential conflict of interest.
Acknowledgments
We thank Bernhard Klarner for collecting the samples as well as Svenja Meyer, Kristina Richter, Anneliese Beckendorff, and Melissa Jüds for help in determining oribatid mites. Furthermore, we thank the staff of the Kompetenzzentrum Stabile Isotope Göttingen (KOSI) for measurement of stable isotopes.
Supplementary Material
The Supplementary Material for this article can be found online at: https://www.frontiersin.org/articles/10.3389/fevo.2021.592149/full#supplementary-material
Footnotes
References
Adler, P. B., and Bradford, J. B. (2002). Compensation: an alternative method for analyzing diversity-productivity experiments. Oikos 96, 411–420. doi: 10.1034/j.1600-0706.2002.960303.x
Balogh, P., and Balogh, J. (2012). The Soil Mites of the World: Vol. 3: Oribatid Mites of the Neotropical Region II, 3rd Edn. Amsterdam: Elsevier.
Bardgett, R. (2005). The Biology of Soil: A Community and Ecosystem Approach. Oxford: Oxford University Press.
Berg, B., and Ryszard, L. (2005). Decomposers: soil microorganisms and animals. Adv. Ecol. Res. 38, 73–100. doi: 10.1016/s0065-2504(05)38003-2
Bommarco, R., Biesmeijer, J. C., Meyer, B., Potts, S. G., and Pöyry, J. (2010). Dispersal capacity and diet breadth modify the response of wild bees to habitat loss. Proc. R. Soc. B: Biol. Sci. 277, 2075–2082. doi: 10.1098/rspb.2009.2221
Bowling, D. R., Pataki, D. E., and Randerson, J. T. (2008). Carbon isotopes in terrestrial ecosystem pools and CO2 fluxes. New Phytol. 178, 24–40. doi: 10.1111/j.1469-8137.2007.02342.x
Bremner, J., Rogers, S. I., and Frid, C. L. J. (2003). Assessing functional diversity in marine benthic ecosystems: a comparison of approaches. Mar. Ecol. Prog. Ser. 254, 11–25. doi: 10.3354/meps254011
Caruso, T., Taormina, M., and Migliorini, M. (2012). Relative role of deterministic and stochastic determinants of soil animal community: a spatially explicit analysis of oribatid mites. J. Animal Ecol. 81, 214–221. doi: 10.1111/j.1365-2656.2011.01886.x
Chase, J. M., and Leibold, M. A. (2003). Ecological Niches: Linking Classical and Contemporary Approaches. Chicago, IL: University of Chicago Press.
Chesson, P. (2000). General theory of competitive coexistence in spatially-varying environments. Theor. Popul. Biol. 58, 211–237. doi: 10.1006/tpbi.2000.1486
Cucherousset, J., and Villéger, S. (2015). Quantifying the multiple facets of isotopic diversity: new metrics for stable isotope ecology. Ecol. Indicators 56, 152–160. doi: 10.1016/j.ecolind.2015.03.032
Dammhahn, M., Randriamoria, T. M., and Goodman, S. M. (2017). Broad and flexible stable isotope niches in invasive non-native rattus spp. in anthropogenic and natural habitats of central Eastern Madagascar. BMC Ecology 17:16. doi: 10.1186/s12898-017-0125-0
DeNiro, M. J., and Epstein, S. (1978). Influence of diet on the distribution of nitrogen isotopes in animals. Geochim. Cosmochim. Acta 45, 341–351. doi: 10.1016/0016-7037(81)90244
Drescher, J., Rembold, K., Allen, K., Beckschäfer, P., Buchori, D., Clough, Y., et al. (2016). Ecological and socio-economic functions across tropical land use systems after rainforest conversion. Philosophical Trans. R. Soc. Lond. Series B Biol. Sci. 231, 1–7. doi: 10.1098/rstb.2015.0275
Gan, H., Zak, D. R., and Hunter, M. D. (2014). Trophic stability of soil oribatid mites in the face of environmental change. Soil Biol. Biochem. 68, 71–77. doi: 10.1016/j.soilbio.2013.09.019
Giller, P. S. (1996). The diversity of soil communities, the ‘poor man’s tropical rainforest’. Biodiversity Conserv. 5, 135–168. doi: 10.1007/bf00055827
Grass, I., Kubitza, C., Krishna, V. V., Corre, M. D., Mußhoff, O., Pütz, P., et al. (2020). Trade-offs between multifunctionality and profit in tropical smallholder landscapes. Nat. Commun. 11:1186. doi: 10.1038/s41467-020-15013-5
Halaj, J., Peck, R. W., and Niwa, C. G. (2005). Trophic structure of a macroarthropod litter food web in managed coniferous forest stands: a stable isotope analysis with δ15N and δ13C. Pedobiologia 49, 109–118. doi: 10.1016/j.pedobi.2004.09.002
Heidemann, K., Hennies, A., Schakowske, J., Blumenberg, L., and Ruess, L. (2014). Free-living nematodes as prey for higher trophic levels of forest soil food webs. Oikos 123, 1199–1211. doi: 10.1111/j.1600-0706.2013.00872.x
Heidemann, K., Scheu, S., Ruess, L., and Maraun, M. (2011). Molecular detection of nematode predation and scavenging in oribatid mites: laboratory and field experiments. Soil Biol. Biochem. 43, 2229–2236. doi: 10.1016/j.soilbio.2011.07.015
Hulot, F. D., Lacroix, G., Lescher-Moutoue, F., and Loreau, M. (2000). Functional diversity governs ecosystem response to nutrient enrichment. Nature 405, 340–344. doi: 10.1038/35012591
Hutchinson, G. E. (1959). Homage to santa rosalia or why are there so many kinds of animals. Am. Natrualist 93, 145–159. doi: 10.1086/282070
Hyodo, F., Matsumoto, T., Takematsu, Y., and Itioka, T. (2015). Dependence of diverse consumers on detritus in a tropical rain forest food web as revealed by radiocarbon analysis. Funct. Ecol. 29, 423–429. doi: 10.1111/1365-2435.12357
Illig, J., Langel, R., Norton, R. A., Scheu, S., and Maraun, M. (2005). Where are the decomposers? Uncovering the soil food web of a tropical montane rain forest in southern ecuador using stable isotopes (15N). J. Tropical Ecol. 21, 589–593. doi: 10.1017/s0266467405002646
Jackson, A. L., Inger, R., Parnell, A. C., and Bearhop, S. (2011). Comparing isotopic niche widths among and within communities: siber - stable isotope bayesian ellipses in R. J. Animal Ecol. 80, 595–602. doi: 10.1111/j.1365-2656.2011.01806.x
Kempson, D., Lloyd, M., and Ghelardi, R. (1963). A new extractor for woodland litter. Pedobiologia 3, 1–21.
Klarner, B., Ehnes, R. B., Erdmann, G., Eitzinger, B., Pollierer, M. M., Maraun, M., et al. (2014). Trophic shift of soil animal species with forest type as indicated by stable isotope analysis. Oikos 123, 1173–1181. doi: 10.1111/j.1600-0706.2013.00939.x
Klarner, B., Winkelmann, H., Krashevska, V., Maraun, M., Widyastuti, R., and Scheu, S. (2017). Trophic niches, diversity and community composition of invertebrate top predators (Chilopoda) as affected by conversion of tropical lowland rainforest in sumatra (Indonesia). PLoS One 1:e180915. doi: 10.1371/journal.pone.0180915
Korotkevich, A. Y., Potapov, A. M., Tiunov, A. V., and Kuznetsova, N. A. (2018). Collapse of trophic-niche structure in belowground communities under anthropogenic disturbance. Ecosphere 9:e02528. doi: 10.1002/ecs2.2528
Kraft, N. J. B., Adler, P. B., Godoy, O., James, E. C., Fuller, S., and Levine, J. M. (2015). Community assembly, coexistence and the environmental filtering metaphor. Functional Ecol. 29, 592–599. doi: 10.1111/1365-2435.12345
Krashevska, V., Sandmann, D., Marian, F., Maraun, M., and Scheu, S. (2017). Leaf litter chemistry drives the structure and composition of soil testate amoeba communities in a tropical montane rainforest of the ecuadorian andes. Microb. Ecol. 74, 681–690. doi: 10.1007/s00248-017-0980-4
Krause, A., Sandmann, D., Bluhm, S. L., Ermilov, S., Widyastuti, R., Haneda, N. F., et al. (2019). Shift in trophic niches of soil microarthropods with conversion of tropical rainforest into plantations as indicated by stable isotopes (15N, 13C). PLoS One 14:e0224520. doi: 10.1371/journal.pone.0224520
Kühn, J., Richter, A., Kahl, T., Bauhus, J., Schöning, I., and Ruess, L. (2018). Community level lipid profiling of consumers as a tool for soil food web diagnostics. Methods Ecol. Evol. 9, 1265–1275. doi: 10.1111/2041-210X.12966
Lagerlöf, J., Maribie, C., and John, J. M. (2017). Trophic interactions among soil arthropods in contrasting land-use systems in Kenya, studied with stable isotopes. Eur. J. Soil Biol. 79, 31–39. doi: 10.1016/j.ejsobi.2017.01.002
Laliberté, E., and Legendre, P. (2010). A distance-based framework for measuring functional diversity from multiple traits. Ecology 91, 299–305. doi: 10.1890/08-2244.1
Langel, R., and Dyckmans, J. (2014). Combined 13C and 15N isotope analysis on small samples using a near-conventional elemental analyzer/isotope ratio mass spectrometer setup. Rapid Commun. Mass Spectro. 28, 1019–1022. doi: 10.1002/rcm.6878
Layman, C. A., Arrington, A. D., Montaña, C. G., and Post, D. M. (2007). Can stable isotope ratios provide for community - wide measures of trophic structure. Ecol. Soc. Am. 88, 42–48.
Lebrun, P. (1971). Écologie et Biocénotique de Quelques Peuplements D’arthropodes Édaphiques. Bruxelles: Institut royal des sciences naturelles de Belgique.
MacArthur, R., and Levins, R. (1967). The limiting similarity hypothesis. Am. Naturalist 101, 377–385.
Maraun, M., Erdmann, G., Fischer, B. M., Pollierer, M. M., Norton, R. A., Schneider, K., et al. (2011). Stable isotopes revisited: their use and limits for oribatid mite trophic ecology. Soil Biol. Biochem. 43, 877–882. doi: 10.1016/j.soilbio.2011.01.003
Maraun, M., Schatz, H., and Scheu, S. (2007). Awesome or ordinary? Global diversity patterns of oribatid mites. Ecography 30, 209–216. doi: 10.1111/j.0906-7590.2007.04994.x
Maraun, M., and Scheu, S. (2000). The structure of oribatid mite communities (Acari, Oribatida): patterns, mechanisms and implications for future research. Source: Ecogr. 23, 374–383. doi: 10.1111/j.1600-0587.2000.tb00294.x
Margono, B. A., Potapov, P. V., Turubanova, S., Stolle, F., and Hansen, M. C. (2014). Primary forest cover loss in Indonesia over 2000-2012. Nat. Climate Change 4, 730–735.
Mason, N. W. H., Mouillot, D., Lee, W. G., and Wilson, B. J. (2005). Functional richness, functional evenness and functional divergence: the primary components of functional diversity. Oikos 111, 112–118. doi: 10.1111/j.0030-1299.2005.13886.x
Meijide, A., Badu, C. S., Moyano, F., Tiralla, N., Gunawan, D., and Knohl, A. (2018). Impact of forest conversion to oil palm and rubber plantations on microclimate and the role of the 2015 ENSO Event. Agric. For. Meteorol. 252, 208–219. doi: 10.1016/j.agrformet.2018.01.013
Minor, M. A., Ermilov, S. G., and Tiunov, A. V. (2017). Taxonomic resolution and functional traits in the analysis of tropical oribatid mite assemblages. Exp. Appl. Acarol. 73, 365–381. doi: 10.1007/s10493-017-0190-2
Myers, N., Mittermeier, R. A., Mittermeier, C. G., da Fonseca, G. A., and Kent, J. (2000). Biodiversity hotspots for conservation priorities. Nature 403, 853–858.
Norton, R. A., and Behan-Pelletier, V. M. (1991). Calcium carbonate and calcium oxalate as cuticular hardening agents in oribatid mites (Acari: oribatida). Can. J. Zool. 69, 1504–1511. doi: 10.1139/z91-210
Pachl, P., Domes, K., Schulz, G., Norton, R. A., Scheu, S., Schaefer, I., et al. (2012). Convergent evolution of defense mechanisms in oribatid mites (acari, oribatida) shows no ‘ghosts of predation past’. Mol. Phylogenet. Evol. 65, 412–420. doi: 10.1016/j.ympev.2012.06.030
Pearson, T. H. (2001). Functional group ecology in soft-sediment marine benthos: the role of bioturbation. Oceanogr. Mar. Biol. 39, 233–267.
Petchey, O. L. (2003). Integrating methods that investigate how complementarity influences ecosystem functioning. Oikos 101, 323–330. doi: 10.1034/j.1600-0706.2003.11828.x
Poisot, T., Nicolas, M., and Gravel, D. (2013). Trophic complementarity drives the biodiversity–ecosystem functioning relationship in food webs. Ecol. Lett. 16, 853–861. doi: 10.1111/ele.12118
Pollierer, M. M., Langel, R., Scheu, S., and Maraun, M. (2009). Compartmentalization of the soil animal food web as indicated by dual analysis of stable isotope ratios (15N/14N and 13C/12C). Soil Biol. Biochem. 41, 1221–1226. doi: 10.1016/j.soilbio.2009.03.002
Post, D. M. (2002). Using stable isotopes to estimate trophic position: models, methos, and assumptions. Ecology 83, 703–718.
Potapov, A. M., Klarner, B., Sandmann, D., Widyastuti, R., and Scheu, S. (2019a). Linking size spectrum, energy flux and trophic multifunctionality in soil food webs of tropical land-use systems. J. Animal Ecol. 88, 1845–1859. doi: 10.1111/1365-2656.13027
Potapov, A. M., Semenina, E. E., Kurakov, A. V., and Tiunov, A. V. (2013). Large 13C/12C and small 15N/14N isotope fractionation in an experimental detrital foodweb (litter-fungi-collembolans). Ecol. Res. 28, 1069–1079. doi: 10.1007/s11284-013-1088-z
Potapov, A. M., Tiunov, A. V., and Scheu, S. (2019b). Uncovering trophic positions and food resources of soil animlas using bulk natural stable isotope composition. Biol. Rev. 94, 37–59. doi: 10.1111/brv.12434
R Core Team. (2018). R: A Language and Environment for Statistical Computing. R Foundation for Statistical Computing. Vienna: R Core Team.
Rembold, K., Mangopo, H., Tjitrosoedirdjo, S. S., and Kreft, H. (2017). Plant diversity, forest dependency, and alien plant invasions in tropical agricultural landscapes. Biol. Conserv. 213, 234–242. doi: 10.1016/j.biocon.2017.07.020
Salles, J. F., Poly, F., Schmid, B., and Roux, X. L. (2009). Community niche predicts the functioning of denitrifying bacterial assemblages. Ecology 90, 3324–3332. doi: 10.1890/09-0188.1
Scheu, S., and Falca, M. (2000). The soil food web of two beech forests (Fagus Sylvatica) of contrasting humus type: stable isotope analysis of a macro- and a mesofauna-dominated community. Oecologia 123, 285–296.
Schneider, K., Migge, S., Norton, R. A., Scheu, S., Langel, R., Reineking, A., et al. (2004). Trophic niche differentiation in soil microarthropods (Oribatida, Acari): evidence from stable isotope ratios (15N/14N). Soil Biol. Biochem. 36, 1769–1774. doi: 10.1016/j.soilbio.2004.04.033
Schulz, G., Schneider, D., Brinkmann, N., Edy, N., Daniel, R., Polle, A., et al. (2019). Changes in trophic groups of protists with conversion of rainforest into rubber and oil palm plantations. Front. Microbiol. 10:240. doi: 10.3389/fmicb.2019.00240
Sodhi, N. S., Posa, M. R. C., Lee, T. M., Bickford, D., Koh, L. P., and Brook, B. W. (2010). The state and conservation of southeast asian biodiversity. Biodiversity Conserv. 19, 317–328.
Spence, K. O., and Rosenheim, J. A. (2005). Isotopic enrichment in herbivorous insects: a comparative field-based study of variation. Oecologia 146, 89–97.
Susanti, W. I., Pollierer, M. M., Widyastuti, R., Scheu, S., and Potapov, A. (2019). Conversion of rainforest to oil palm and rubber plantations alters energy channels in soil food webs. Ecol. Evol. 9, 9027–9039. doi: 10.1002/ece3.5449
Tilman, D. (2004). Niche tradeoffs, neutrality, and community structure: a stochastic theory of resource competition, invasion, and community assembly. Proc. Natl. Acad. Sci. U.S.A. 101, 10854–10861. doi: 10.1073/pnas.0403458101
Tiunov, A. V. (2007). Stable isotopes of carbon and nitrogen in soil ecological studies. Biol. Bull. 34, 395–407.
Van der Merwe, N. J., and Medina, E. (1991). The canopy effect, carbon isotope ratios and foodwebs in amazonia. J. Archaeol. Sci. 18, 249–259. doi: 10.1016/0305-4403(91)90064-V
Vanderklift, M. A., and Ponsard, S. (2003). Sources of variation in consumer-diet δ15N enrichment: a meta-analysis. Oecologia 136, 169–182. doi: 10.1007/s00442-003-1270-z
Villéger, S., Mason, N. W. H., and Mouillot, D. (2008). New multidimensional functional diversity indices for a multifaceted framework in functional ecology. Ecology 89, 2290–2301. doi: 10.1890/07-1206.1
Violle, C., Nemergut, D. R., Pu, Z., and Jiang, L. (2011). Phylogenetic limiting similarity and competitive exclusion. Ecol. Lett. 14, 782–787. doi: 10.1111/j.1461-0248.2011.01644.x
Wickham, H. (2016). ggplot2: Elegant Graphics for Data Analysis. New York, NY: Springer-Verlag New York.
Wilcove, D. S., Giam, X., Edwards, D. P., Fisher, B., and Koh, L. P. (2013). Navjot’s nightmare revisited: logging, agriculture, and biodiversity in Southeast Asia. Trends Ecol. Evol. 28, 531–540.
Keywords: niche differentiation, community structure, mesofauna, Acari, Oribatida, land-use change, Sumatra, isotopic metrics
Citation: Krause A, Sandmann D, Potapov A, Ermilov S, Widyastuti R, Haneda NF, Scheu S and Maraun M (2021) Variation in Community-Level Trophic Niches of Soil Microarthropods With Conversion of Tropical Rainforest Into Plantation Systems as Indicated by Stable Isotopes (15N, 13C). Front. Ecol. Evol. 9:592149. doi: 10.3389/fevo.2021.592149
Received: 06 August 2020; Accepted: 12 April 2021;
Published: 13 May 2021.
Edited by:
Hideyuki Doi, University of Hyogo, JapanReviewed by:
Felicity Victoria Crotty, Royal Agricultural University, United KingdomKyle Wickings, Cornell University, United States
Copyright © 2021 Krause, Sandmann, Potapov, Ermilov, Widyastuti, Haneda, Scheu and Maraun. This is an open-access article distributed under the terms of the Creative Commons Attribution License (CC BY). The use, distribution or reproduction in other forums is permitted, provided the original author(s) and the copyright owner(s) are credited and that the original publication in this journal is cited, in accordance with accepted academic practice. No use, distribution or reproduction is permitted which does not comply with these terms.
*Correspondence: Anton Potapov, potapov.msu@gmail.com