- Department of Ecology, Evolution, and Marine Biology, University of California, Santa Barbara, Santa Barbara, CA, United States
Metabolic symbiosis is a form of symbiosis in which organisms exchange metabolites, typically for mutual benefit. For example, acquired phototrophs like Paramecium bursaria obtain photosynthate from endosymbiotic green algae called Chlorella. In addition to facilitating the persistence of P. bursaria by providing a carbon source that supplements P. bursaria’s heterotrophic digestion of bacteria, symbiotic Chlorella may impact competitive interactions between P. bursaria and other bacterivores, with cascading effects on community composition and overall diversity. Here, we tested the effects of metabolic symbiosis on coexistence by assessing the impacts of acquired phototrophy on priority effects, or the effect of species arrival order on species interactions, between P. bursaria and its competitor Colpidium. Our results suggest light-dependent priority effects. The acquired phototroph benefited from metabolic symbiosis during sequential arrival of each organism in competition, and led to increased growth of late-arriving Colpidium. These findings demonstrate that understanding the consequences of priority effects for species coexistence requires consideration of metabolic symbiosis.
Introduction
Metabolic symbiosis is a form of symbiosis in which organisms exchange metabolites, typically for mutual benefit. In some cases, metabolic partners are capable of integrating metabolic pathways across genomes to produce shared metabolites, such as amino acids and vitamins, for mutual survival (Husnik et al., 2013), and other organisms can directly use metabolites produced by a partner organism for energy or nutrient sources (Estrela et al., 2012). Symbiotic mycorrhizal fungi benefit plant hosts by increasing the efficiency of nutrient acquisition in exchange for carbohydrates (Marschner and Dell, 1994). Similarly, planktonic ciliates, such as Paramecium bursaria, host algae in exchange for photosynthates (Stoecker et al., 2009; Iwai et al., 2019). Symbiotic relationships, such as mutualisms that benefit the two organisms involved in the symbiosis, can also have important implications for community dynamics by promoting diversity, population stability (Pachepsky et al., 2002; Mougi and Kondoh, 2012), and even co-evolution (Guimarães et al., 2011). Metabolic symbiosis is context-dependent: environmental conditions are known to shift mutually beneficial relationships into host-parasitism when costs begin to outweigh benefits of symbiosis (Bronstein, 1994; Johnson et al., 1997; Lowe et al., 2016).
When organisms receive metabolic benefits from a symbiont, this symbiosis can increase competitive ability and facilitate coexistence between competitors. In plant and mycorrhizal symbiosis, fungal partners may increase competitive abilities of host plant species through differential benefits (Callaway et al., 2001), and may also mediate competition through common mycorrhizal networks within a plant population (Francis and Read, 1984; Grime et al., 1987). Acquired phototrophs, such as P. bursaria, can also become a better competitor by gaining access to a new resource via acquisition of photosynthetic machinery through their endosymbionts (Moeller et al., 2016). However, the extent of these benefits can vary. The growth rate of P. bursaria with endosymbiotic algae depends on temperature (Salsbery and DeLong, 2018) and light level (Pado, 1965; Weis, 1974). Here, we study the role of metabolic symbiosis on species coexistence under different community assemblage scenarios.
The effects of metabolic symbiosis on competitive outcomes may also be impacted by the particular timing and order in which species join a community, a phenomenon known as priority effects (Chase, 2003; Fukami, 2015). In other words, the competitive ability of a species can change based on its arrival order relative to its competitor. Priority effects have been studied across taxonomic domains between yeast and bacteria (Fukami, 2015), between functional groups in plants (Weidlich et al., 2018), and under various environmental conditions (Vannette and Fukami, 2014). The outcomes of priority effects are highly variable across species and ecosystems (Fukami, 2015). When priority effects are present, there are two possible outcomes: facilitation, in which the early arriving species positively influences the growth of the late-arriving species, or inhibition, where the opposite is true (Fukami, 2015). The impact of a metabolic symbiosis on the strength of priority effects has not been extensively investigated.
In this study, we used two bacterivorous ciliate competitors, Paramecium bursaria, and Colpidium sp., to test for priority effects. Colpidium is a strict heterotroph while P. bursaria engages in metabolic symbiosis with its algal endosymbiont, Chlorella. Paramecium bursaria almost always host Chlorella, yet is known to harbor endosymbionts that come from at least five different species, including Coccomyxa simplex and Micractinium conductrix (Hoshina and Imamura, 2008; Johnson, 2011; Pröschold et al., 2011). In the presence of light, endosymbiotic Chlorella undergoes photosynthesis and provides sugar metabolites for P. bursaria presumably in exchange for protection (Karakashian, 1963; Pado, 1965; Weis, 1974). Paramecium bursaria also provides essential nitrogen by prey cell digestion for Chlorella’s nutritional requirement, and P. bursaria cells with endosymbionts have increased bacterial ingestion (Albers and Wiessner, 1985; Johnson, 2011). To understand the role of metabolic symbiosis on species coexistence, we tested the strength of priority effects between P. bursaria and Colpidium. We manipulated light level to control the contribution of photosynthesis on P. bursaria’s growth. We hypothesized that as light level increased, Chlorella would also increase its photosynthetic capacity, thereby providing more metabolites for P. bursaria’s growth. In darkness, hosting photosynthetic endosymbionts becomes too costly and confers no energetic benefits, so Chlorella would likely be expelled and P. bursaria would rely entirely on heterotrophy (Lowe et al., 2016). In our study, we did not observe bleached P. bursaria, suggesting that expulsion, if it was occurring, proceeded at slower timescales than death of P. bursaria. The two species were inoculated sequentially to mimic differential species assemblage, and the strength of priority effects were measured by comparing species densities across treatments. Our results suggested light-dependent priority effects. The acquired phototrophs persisted in the presence of metabolic symbiosis with endosymbiotic algae when the competitors assembled sequentially, and even facilitated the growth of late-arriving Colpidium. Our findings demonstrated that understanding the consequences of priority effects for species coexistence and overall maintenance of biodiversity requires consideration of metabolic symbiosis.
Methodology
Cultures of Colpidium sp., P. bursaria, and bacterial species (see below) were obtained from Carolina Biological Supply company. To allow Colpidium and P. bursaria to adjust to the light environments corresponding to the experimental conditions, the laboratory stock cultures were maintained at 50, 100, and 200 μmol quanta m–2 s–1 in an incubator at 24°C under controlled light conditions (12 h light: 12 h dark) for at least 2 weeks prior to experimentation.
Initially sterilized protozoan pellet media (Carolina Biological Supply Company) was inoculated with bacteria 2 days prior to the start of experimentation to control for bacterial prey availability in experimental microcosms. We inoculated treatments with three species of bacteria: (1) Serratia marcescens and the dominant bacterial species from purchased protist cultures of (2) P. bursaria and (3) Colpidium. The purchased protist cultures were plated onto Nutrient Agar (Research Products International), and we isolated the most abundant bacterial morphotype to identify the dominant bacterial morphospecies co-occurring with P. bursaria and Colpidium. These bacterial morphospecies were transferred from the agar plates to liquid media culture to be inoculated into treatment microcosms. Bacterizing experimental media with the same three bacterial morphospecies ensured that each treatment culture was primed with the same key bacterial prey community members.
To test how photosynthetic symbiosis impacted the strength of priority effects, we sequentially inoculated P. bursaria and Colpidium into experimental microcosms at four different light levels (0, 50, 100, 200 μmol quanta m–2 s–1) in triplicate. The two introduction treatments were: (i) “P. bursaria-first” sequential inoculations, in which P. bursaria was introduced first on Day 0, and Colpidium on Day 14; (ii) “Colpidium-first,” in which Colpidium was introduced first on Day 0, and P. bursaria on Day 7. The intrinsic growth rate and carrying capacity of both species were determined by conducting single-ciliate control treatments. In single-species treatments, Colpidium had a much higher intrinsic growth rate, and stopped growing exponentially by Day 7, while P. bursaria required 14 days for its populations to stabilize. Because, in our priority effects experiments, we wanted P. bursaria and Colpidium to grow to their maximum population sizes before the introduction of the second species, we decided to allow P. bursaria 14 days of growth before Colpidium inoculation for P. bursaria-first treatments, and 7 days for Colpidium in Colpidium-first treatments. Thus the total experiment contained 48 microcosms: 2 priority effect treatments (P. bursaria-first, Colpidium-first) × 4 light levels (0, 50, 100, 200 μmol quanta m–2 s–1) × 3 replicates, plus 2 single-species controls (P. bursaria only, Colpidium only) × 4 light levels × 3 replicates.
At the start of each experiment, we inoculated 50 ciliates/mL into the experimental microcosms with 40 mL of liquid media. For priority effects treatments, we subsequently inoculated 50 ciliates/mL of the second species on Day 7 (Colpidium-first) or Day 14 (P. bursaria-first) of the experiment. We quantified population densities of each ciliate three times per week over the duration of the experiment (40 days). During sampling, an aliquot containing at least 50 ciliate cells (50–1,000 μL) was removed from each microcosm, and the ciliates were individually counted using a dissecting microscope. We used a range of aliquot sample volumes because the population sizes throughout the duration of the experiment (40 days) often differed up to two orders of magnitude (Supplementary Figure S1). More specifically, during the first week, we removed 1 mL from each experimental flask for enumeration, during the second and third weeks we removed 200 μL, and during the subsequent weeks we removed 50 μL.
We quantified a priority effects metric (modified from Vannette and Fukami, 2014) by comparing the maximum population sizes achieved by each species when introduced first [D(i)ij] to maximum population when introduced last [D(i)ji]:
The order of the subscripts i and j indicate arrival order of the species. By taking the natural log of this ratio of population sizes, we achieve a metric for which positive values indicate positive priority effects (e.g., lower population sizes when introduced first, indicating that the early presence of the other species benefited the later arriving species) and negative values indicate negative priority effects (e.g., lower population sizes when introduced last, indicating that the early presence of the other species inhibited growth of the later arriving species).
To confirm that observed priority effects were not due to the presence of free-living Chlorella cells, we visually inspected stock cultures and experimental cultures using light microscopy for the presence of Chlorella colonies. We also performed chlorophyll-a extractions on unspent media (media that had never been inoculated with P. bursaria) as well as spent media from experimental cultures at all light levels. We obtained this spent media by passaging 1 mL of culture volume through an 8.0 mm transwell membrane filter (Corning Incorporated, Corning, NY, United States), and confirming using light microscopy that the filtrate did not contain any P. bursaria cells. We then collected this filtrate on a GF/F filter (Whatman, SigmaAldrich, St. Louis, MO, United States), placed the filter in 5 mL of a 90% acetone solution in water, and incubated overnight at -20°C. The following day, we measured chlorophyll-a content in relative fluorescence units using a Trilogy fluorometer with a 450 nm LED (Turner Designs, San Jose, CA).
We performed all data analyses and plotting using the open-source software package R (R Core Team, 2020). Our analysis script and data files can be found at https://doi.org/10.5281/zenodo.4342275.
Results
Paramecium bursaria was negatively affected by early arriving Colpidium, with P. bursaria populations visibly lower in all Colpidium-first treatments and nearly competitively excluded at the end of 40 days (Figures 1A–D). Conversely, Colpidium grew to higher population sizes when P. bursaria was introduced first, and density differences between Colpidium-first and P. bursaria-first treatments at each light level increased with increasing irradiance (Figures 1E–H). The calculated strength of priority effects using the metric defined by Eq. 1 supported these observations (Figure 2 and Supplementary Table S1). P. bursaria experienced negative priority effects, which indicates that early arriving Colpidium inhibited the growth of P. bursaria (Figure 2A). Figure 2B shows the strength of priority effects by light level, and at 0 μmol quanta m–2 s–1, P. bursaria populations died off independent of Colpidium (Figures 1, 2B). Yet increasing light level (from 50 to 200 μmol quanta m–2 s–1) did not enhance the negative affect of Colpidium on P. bursaria (Figure 2B). Conversely, P. bursaria had a positive priority effect on Colpidium (Figure 2A), and this positive priority effect strengthened with increasing light (Figure 2B). Colpidium populations at 100 and 200 μmol quanta m–2 s–1 both benefited from pre-established P. bursaria, with significantly higher positive priority effects at 200 μmol quanta m–2 s–1 (Figure 2B).
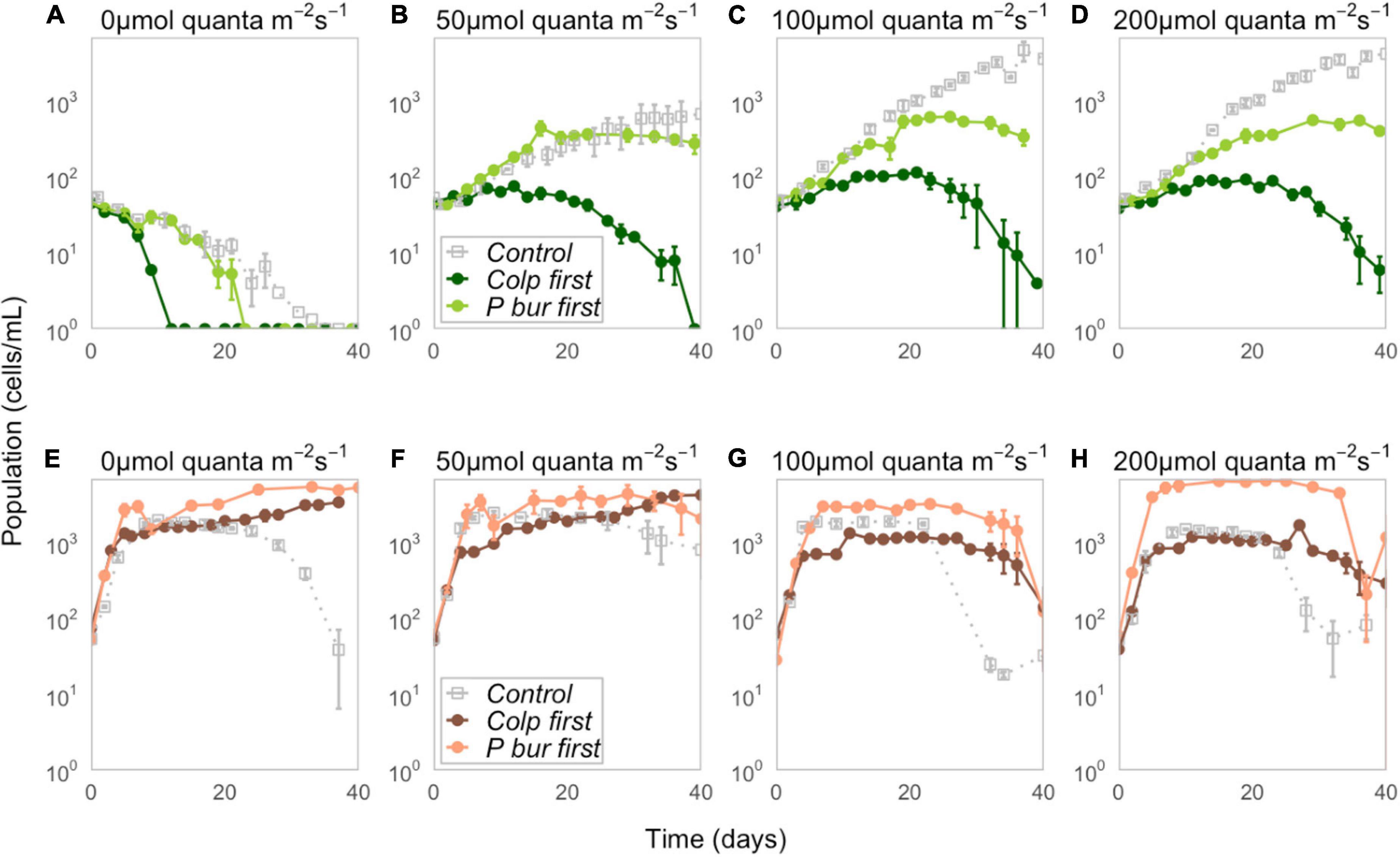
Figure 1. Top row (A–D) Population dynamics of P. bursaria for control (gray), Colpidium-first (dark green), and P. bursaria-first (light green) treatments at four different light levels (0, 50, 100, and 200 μmol quanta m–2 s–1). Time is measured in days since inoculation, rather than experimental day, so that t = 0 represents the date on which the focal (plotted) species was inoculated. (see Supplementary Figure S1 for population size as a function of experimental day). Bottom row (E–H) Population dynamics of Colpidium for control (gray), Colpidium-first (dark coral), and P. bursaria-first (light coral) treatments at four different light levels (0, 50, 100, and 200 μmol quanta m–2 s–1). Note that population densities were recorded on a logarithmic scale, and error bars represent ± one standard error.
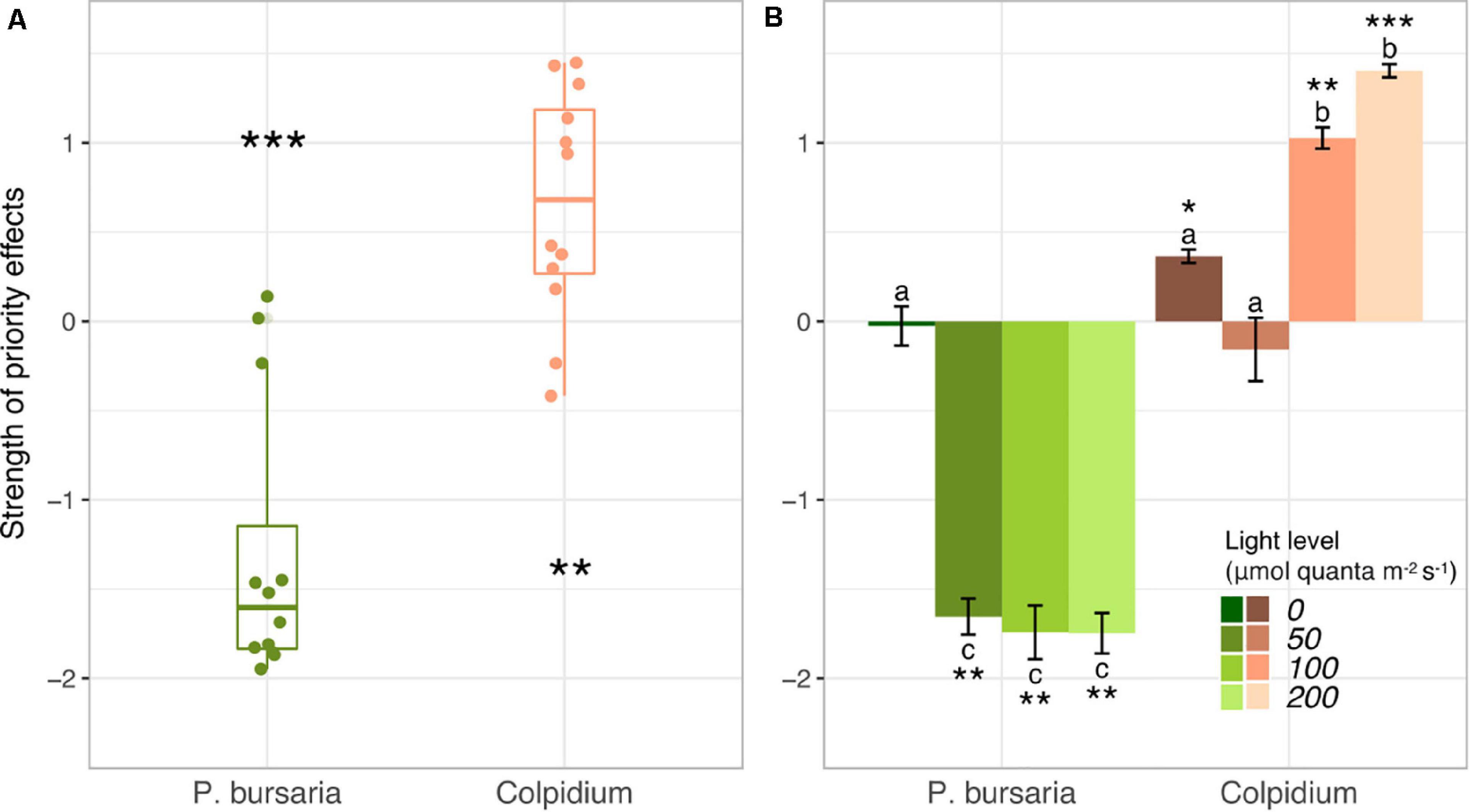
Figure 2. Priority effects metric (Eq. 1) between Colpidium and P. bursaria (Vannette and Fukami, 2014) generated from population dynamics shown in Figure 1. (A) Boxplots of priority effects metric demonstrate that Colpidium was positively affected by early arriving P. bursaria, and P. bursaria was negatively affected by early arriving Colpidium. (B) The strength of priority effects by light level. Different letters represent statistically significant differences at the p < 0.05 level (Tukey’s HSD, analysis of variance comparing all light × species interactions, see summary of initial ANOVA tests Supplementary Table S1). On all panels, error bars represent ± one standard error. Stars indicate significant differences from zero (T-test; *p < 0.05, **p < 0.01, ***p < 0.001).
We obtained maximum population sizes within the 40-day duration of the experiment (Figures 3A,B) and growth rates captured the initial exponential growth period before reaching carrying capacity, calculated from the first 10 days for Colpidium, and 20 days for P. bursaria (Figures 3C,D). Comparison of priority effect treatments with single-species controls demonstrated that presence of any competitor, regardless of arrival order, inhibited P. bursaria growth (Figure 1). In high light (100 and 200 μmol quanta m–2 s–1), P. bursaria maximum population abundances were significantly lower in the presence of Colpidium competitors (ANOVA F = 47.14, p < 0.01, Supplementary Table S2), and were visibly lower when Colpidium was introduced first (Figure 3A). Even when P. bursaria had pre-established populations before Colpidium was introduced in P. bursaria-first treatments, the later introduction of Colpidium resulted in lower maximum population sizes of P. bursaria (Figure 3A). The initial growth rates were not significantly different between the P. bursaria controls and P. bursaria-first treatments (Figure 3C), so lower populations sizes were not explained by initial growth rates. When P. bursaria was introduced second, however, the priority effect was strong enough to inhibit initial growth rates (Figure 3C) and ultimately, population sizes (Figure 3A).
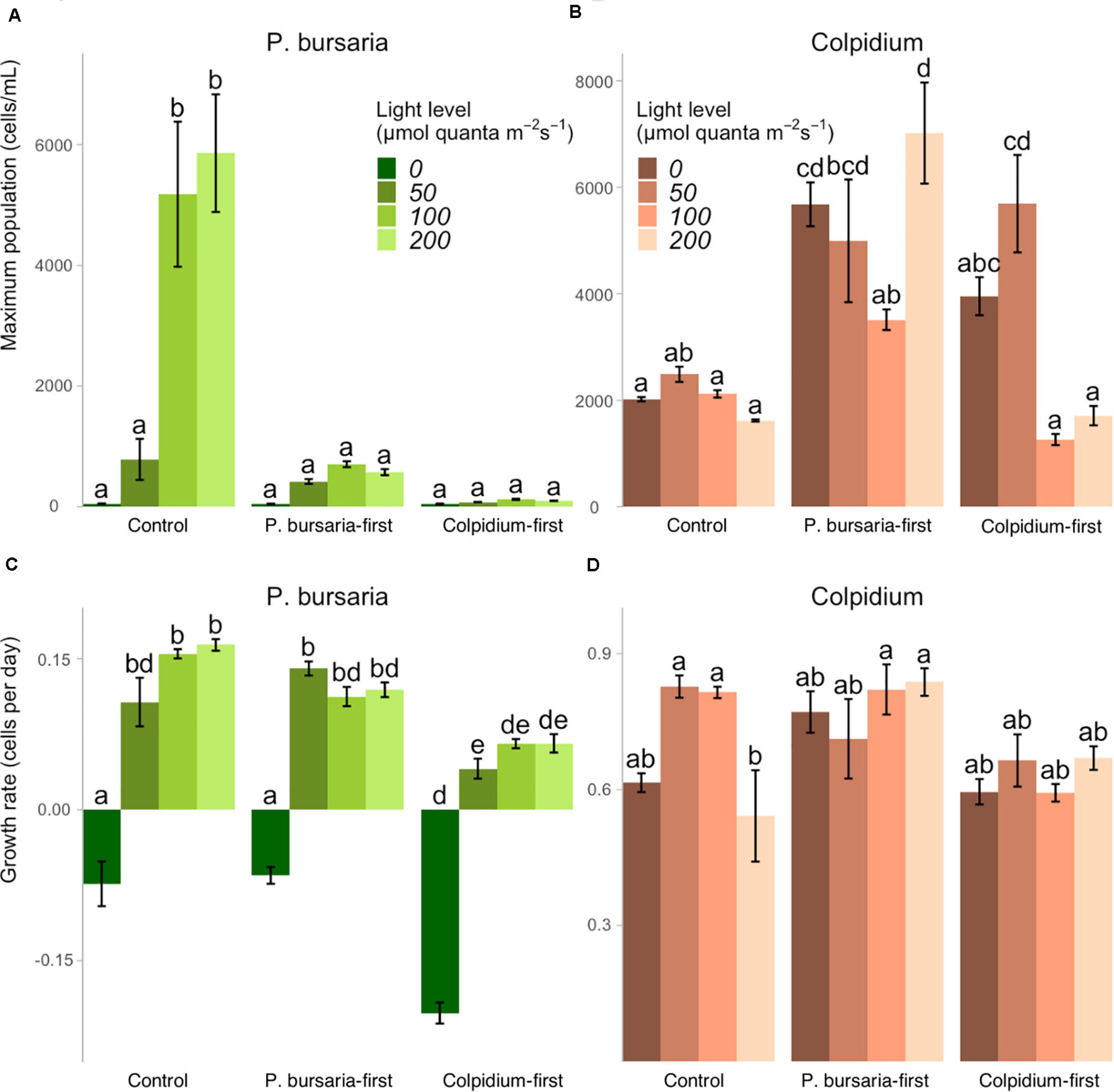
Figure 3. The effect of light levels on maximum population size and growth rates for single-celled controls, P. bursaria-first and Colpidium-first treatments, using the species’ observed light level response curves shown in Figure 1. Maximum population sizes for (A) P. bursaria demonstrates strong inhibition by early and late-arriving Colpidium, while maximum population sizes of (B) Colpidium generally benefited from presence of P. bursaria. The growth rates of (C) P. bursaria are significantly lower when Colpidium arrives first, while growth rates of (D) Colpidium are generally unaffected across treatments. Different letters represent statistically significant differences at the p < 0.05 level (Tukey’s HSD within each panel, analysis of variance comparing all treatments × light levels, see summary of initial ANOVA tests Supplementary Tables S2, S3), and error bars represent ± one standard error.
Colpidium population densities were generally greater in the presence of P. bursaria, especially when P. bursaria arrived first (Figure 3B). However, there was an exception in Colpidium-first treatments at 100 and 200 μmol quanta m–2 s–1, in which early arriving Colpidium did not benefit from the subsequent introduction of P. bursaria (Figure 3B). Effects on Colpidium were generally not mediated by initial growth rate, suggesting alleviation of later resource limitation was responsible for priority effects (Figure 3D). We found no evidence of free-living Chlorella cells in either microscope assays or through increases in chlorophyll-a fluorescence (Supplementary Figure S2).
Discussion
Our results highlight the importance of acquired metabolism to community assembly. In our study, P. bursaria, whose photosynthetic symbionts provide a secondary source of energy, facilitated the later arrival of the strict heterotroph, Colpidium (a positive priority effect). This facilitative priority effect of P. bursaria on Colpidium is absent in direct competition (Hsu et al., unpublished) and is unlikely due to free-living endosymbiotic algae Chlorella. Although Chlorella can escape host cells and grow independent of P. bursaria (Lowe et al., 2016), there was no significant increase in chlorophyll-a from free-living Chlorella across light levels (Supplementary Figure S2) perhaps due to the presence of Chlorella viruses in our cultures or lack of regulatory factors to survive on its own (Kato and Imamura, 2009; Johnson, 2011). Facilitative priority effects are often explained by niche modification, in which the early arriving species alters the type of niches available in the local environment, consequently shaping the species that are capable of colonization (Fukami, 2015). One mechanistic explanation could be that photosynthetic activity by early arriving P. bursaria increased available organic material in the system, increasing the bacterial resources and, ultimately, the maximum population size of Colpidium. Because Chlorella are endosymbiotic, such a release of organic matter would have to occur through either excretion of waste products by P. bursaria, or lysis of P. bursaria cells and subsequent remineralization of this organic matter by bacteria. Positive priority effects have also been observed when certain plant species increase nutrient availability and alter soil chemistry, facilitate the establishment of mutualistic mycorrhizal networks, or leave behind nutrient-rich litter (D’Antonio and Vitousek, 1992; van de Voorde et al., 2011). Species can also change the physical conditions of the environment to alter community succession. For example, grass invasions can promote seed germination of other alien grasses by changing soil temperature and moisture (D’Antonio and Vitousek, 1992).
In contrast, early arrival by Colpidium accelerated competitive exclusion of P. bursaria (a negative priority effect). In a previous study, Müller et al. (2012) cultured P. bursaria in Colpidium conditioned media, and found that allelochemicals produced by Colpidium did not inhibit P. buraria growth. Thus, the negative priority effects of Colpidium on P. bursaria may have been driven by competition for common resources, such as bacterial prey, though we did not collect data on bacterial abundance as part of our study. Paramecium bursaria needs to maintain bacterial ingestion to provide important nutrients, such as nitrogen, essential for both P. bursaria and its endosymbiont, Chlorella (Albers and Wiessner, 1985; Johnson, 2011). This competition may be enhanced when Colpidium populations are pre-established. Indeed, niche preemption, in which the early arriving species reduces the resources available (Fukami, 2015), is a widely known phenomenon observed in other microbial systems (Peay et al., 2012; Ng et al., 2013; Vannette and Fukami, 2014), as well as between competing plants (Grman and Suding, 2010; Kardol et al., 2013) and animals (Geange and Stier, 2009).
The availability of benefits from metabolic symbiosis shapes the strength and direction of priority effects. In our study, the photosynthetic contribution of Chlorella was limited by light availability: In light environments (>0 μmol quanta m–2 s–1) when symbiotic benefits were greatest, P. bursaria persisted for longer than in dark environments (0 μmol quanta m–2 s–1). In other systems, the presence or absence of symbiotic partners has a similar effect. For example, in grassland systems where pioneer species preempt light and space niches from subsequent community members, the inclusion of soil mutualists (such as mycorrhizal fungi) reduced the strength of priority effects and allowed for the later establishment of native grass (Burkle and Belote, 2015).
In our study system, the metabolic value of symbiosis (acquired phototrophy) should scale with light availability. We found that the strength of positive priority effects (facilitation of Colpidium) increased with increasing light, which supports our proposed mechanism: that Colpidium is facilitated by increased bacterial growth supported by P. bursaria symbiont photosynthetic activity. Because photosynthetic rates (and population sizes of P. bursaria in control environments, Figure 3A) increase with increasing light levels, the supply of organic matter should also increase. However, the negative effect of Colpidium on P. bursaria did not scale with light. We found that increasing light (and, presumably, increasing photosynthetic benefits from acquired metabolism) did not alleviate competitive effects of Colpdium on P. bursaria, perhaps because P. bursaria initial growth (Figure 3C) and establishment were inhibited by the presence of established Colpidium populations, which are not light-dependent. This phenomenon may be explained by niche preemption, a mechanism of priority effects in which the early arriving species limits the resources available for successive species (Fukami, 2015). Pre-established Colpidium could have reduced bacterial densities, which limited P. bursaria population sizes across all light levels due to limited access to essential nitrogen. In this situation, additional photosynthate benefits of Chlorella were apparently insufficient to compensate for limited bacterial supply, especially since bacterial ingestion by P. bursaria is also a primary source of nitrogen for Chlorella (Lowe et al., 2016).
Although symbioses, such as metabolic symbiosis of P. bursaria, provide access to new resources and increased competitive ability, there are tradeoffs that constrain the survival in certain environments. Paramecium bursaria does not dominate Colpidium in low light environments, perhaps because it experiences tradeoffs between heterotrophy and photosynthesis (e.g., spatial constraints within its cytoplasm, where space is shared between digestive vacuoles and vacuoles containing Chlorella photosymbionts) that prevent P. bursaria from persisting on heterotrophy alone (Salsbery and DeLong, 2018; Flynn et al., 2019). A previous model found that mixotrophs are most successful by being mostly photosynthetic and supplementing nutritional needs with heterotrophy (Crane and Grover, 2010), which could explain why the P. bursaria in our experiments were light-dependent. In darkness, endosymbiotic Chlorella cells may also become parasitic because they no longer provide photosynthates, and these costs may contribute to the accelerated declines in P. bursaria populations (Lowe et al., 2016). In other systems in which hosts rely on acquired photosynthesis, hosts have become obligately reliant on photosynthesis. For example, the acquired phototroph Mesodinium rubrum requires light for growth (Moeller et al., 2011), and zooxanthellate corals (which depend on symbiotic dinoflagellates for photosynthates) have decreased growth and survival without the symbiont (Brown, 1997; Douglas, 2003). Similarly, insects can have thermally sensitive bacterial partners that constrain the insects adaptation to elevated temperatures (Wernegreen, 2012). Metabolic symbiosis allows species to access new resources that were previously unavailable. However, these benefits require energetic trade-offs that would become disadvantageous when environmental conditions change.
Because metabolic symbiosis alters competitive abilities and priority effects, it has cascading effects on the maintenance of diversity in ecosystems. In our system, acquired photosynthesis can facilitate the subsequent invasion by a second, heterotrophic species, and facilitate long-term coexistence of P. bursaria and Colpidium (Hsu et al., unpublished). By using a stylized model system—protist microcosms whose light levels mimic a range of natural freshwater pond settings yet which contain a constrained subset of species—we are able to more clearly identify the direction and strength of species interactions, including the facilitative effects of symbiosis. Previous work in grassland systems has shown that microbial symbionts (e.g., mycorrhizae) can increase grassland biodiversity and ecosystem functioning via facilitative priority effects (Burkle and Belote, 2015). Long term studies show that wood-decaying fungi, metabolic symbionts of some insects, can mediate positive priority effects of rare, wood-living beetles, therefore maintaining biodiversity of beetle species in boreal forests in Sweden (Weslien et al., 2011). Thus, accounting for metabolic symbiosis allows us to better predict the outcomes of competition, strength of priority effects, and success of restoration projects. The results of our study demonstrate the importance of metabolic symbiosis on competition outcomes under different community assemblage scenarios, and should encourage future work on priority effects and symbiosis.
Data Availability Statement
All datasets and code used in this study can be accessed at https://doi.org/10.5281/zenodo.4342275. Further inquiries can be directed to the corresponding authors.
Author Contributions
VH and HM conceived the ideas, designed methodology, analyzed the data, drafted the manuscript, contributed to subsequent revisions, and gave final approval for publication. VH collected the experimental data. Both authors contributed to the article and approved the submitted version.
Funding
This research was supported by a UC Santa Barbara Undergraduate Research and Creative Activities grant to VH. Research was also sponsored by the U.S. Army Research Office and was accomplished under Cooperative Agreement No. W911NF-19-2-0026 for the Institute for Collaborative Biotechnologies. The views and conclusions contained in this document are those of the authors and should not be interpreted as representing the official policies, either expressed or implied, of the U.S. Government. The U.S. Government is authorized to reproduce and distribute reprints for Government purposes notwithstanding any copyright notation herein.
Conflict of Interest
The authors declare that the research was conducted in the absence of any commercial or financial relationships that could be construed as a potential conflict of interest.
Acknowledgments
We are grateful for laboratory assistance provided by M. Lepori-Bui. T. Fukami and C. Rodgers provided helpful discussion of experimental design and tools to quantify priority effects.
Supplementary Material
The Supplementary Material for this article can be found online at: https://www.frontiersin.org/articles/10.3389/fevo.2020.614367/full#supplementary-material
References
Albers, D., and Wiessner, W. (1985). Nitrogen nutrition of endosymbiotic Chlorella spec. Endocytob. Cell Res. 2, 55–64.
Bronstein, J. L. (1994). Conditional outcomes in mutualistic interactions. Trends Ecol. Evol. 9, 214–217. doi: 10.1016/0169-5347(94)90246-1
Brown, B. E. (1997). Coral bleaching: causes and consequences. Coral Reefs 16, S129–S138. doi: 10.1007/s003380050249
Burkle, L. A., and Belote, R. T. (2015). Soil mutualists modify priority effects on plant productivity, diversity, and composition. Appl. Veg. Sci. 18, 332–342. doi: 10.1111/avsc.12149
Callaway, R., Newingham, B., Zabinski, C. A., and Mahall, B. E. (2001). Compensatory growth and competitive ability of an invasive weed are enhanced by soil fungi and native neighbours. Ecol. Lett. 4, 429–433. doi: 10.1046/j.1461-0248.2001.00251.x
Chase, J. M. (2003). Community assembly: when should history matter? Oecologia 136, 489–498. doi: 10.1007/s00442-003-1311-7
Crane, K. W., and Grover, J. P. (2010). Coexistence of mixotrophs, autotrophs, and heterotrophs in planktonic microbial communities. J. Theor. Biol. 262, 517–527. doi: 10.1016/j.jtbi.2009.10.027
D’Antonio, C. M., and Vitousek, P. M. (1992). Biological invasions by exotic grasses, the grass/fire cycle, and global change. Annu. Rev. Ecol. Syst. 23, 63–87. doi: 10.1146/annurev.es.23.110192.000431
Douglas, A. E. (2003). Coral bleaching—-how and why? Mar. Pollut. Bull. 46, 385–392. doi: 10.1016/S0025-326X(03)00037-7
Estrela, S., Trisos, C. H., and Brown, S. P. (2012). From metabolism to ecology: cross-feeding interactions shape the balance between polymicrobial conflict and mutualism. Am. Nat. 180, 566–576. doi: 10.1086/667887
Flynn, K. J., Mitra, A., Anestis, K., Anschütz, A. A., Calbet, A., Ferreira, G. D., et al. (2019). Mixotrophic protists and a new paradigm for marine ecology: where does plankton research go now? J. Plankton Res. 41, 375–391. doi: 10.1093/plankt/fbz026
Francis, R., and Read, D. J. (1984). Direct transfer of carbon between plants connected by vesicular–arbuscular mycorrhizal mycelium. Nature 307, 53–56. doi: 10.1038/307053a0
Fukami, T. (2015). Historical contingency in community assembly: integrating niches, species pools, and priority effects. Annu. Rev. Ecol. Evol. Syst. 46, 1–23. doi: 10.1146/annurev-ecolsys-110411-160340
Geange, S. W., and Stier, A. C. (2009). Order of arrival affects competition in two reef fishes. Ecology 90, 2868–2878. doi: 10.1890/08-0630.1
Grime, J. P., Mackey, J. M. L., Hillier, S. H., and Read, D. J. (1987). Floristic diversity in a model system using experimental microcosms. Nature 328, 420–422. doi: 10.1038/328420a0
Grman, E., and Suding, K. N. (2010). Within-year soil legacies contribute to strong priority effects of exotics on native california grassland communities. Restoration Ecol. 18, 664–670. doi: 10.1111/j.1526-100X.2008.00497.x
Guimarães, P. R. Jr., Jordano, P., and Thompson, J. N. (2011). Evolution and coevolution in mutualistic networks. Ecol. Lett. 14, 877–885. doi: 10.1111/j.1461-0248.2011.01649.x
Hoshina, R., and Imamura, N. (2008). Multiple origins of the symbioses in Paramecium bursaria. Protist 159, 53–63. doi: 10.1016/j.protis.2007.08.002
Husnik, F., Nikoh, N., Koga, R., Ross, L., Duncan, R. P., Fujie, M., et al. (2013). Horizontal gene transfer from diverse bacteria to an insect genome enables a tripartite nested mealybug symbiosis. Cell 153, 1567–1578. doi: 10.1016/j.cell.2013.05.040
Iwai, S., Fujita, K., Takanishi, Y., and Fukushi, K. (2019). Photosynthetic endosymbionts benefit from host’s phagotrophy, including predation on potential competitors. Curr. Biol. 29, 3114.e3–3119.e3. doi: 10.1016/j.cub.2019.07.074
Johnson, M. D. (2011). The acquisition of phototrophy: adaptive strategies of hosting endosymbionts and organelles. Photosynth. Res. 107, 117–132. doi: 10.1007/s11120-010-9546-8
Johnson, N. C., Graham, J. H., and Smith, F. A. (1997). Functioning of mycorrhizal associations along the mutualism–parasitism continuum. New Phytol. 135, 575–585. doi: 10.1046/j.1469-8137.1997.00729.x
Karakashian, S. J. (1963). Growth of paramecium bursaria as influenced by the presence of algal symbionts. Physiol. Zool. 36, 52–68. doi: 10.1086/physzool.36.1.30152738
Kardol, P., Souza, L., and Classen, A. T. (2013). Resource availability mediates the importance of priority effects in plant community assembly and ecosystem function. Oikos 122, 84–94. doi: 10.1111/j.1600-0706.2012.20546.x
Kato, Y., and Imamura, N. (2009). Amino acid transport systems of JapaneseParamecium symbiont F36-ZK. Symbiosis 47, 99–107. doi: 10.1007/BF03182293
Lowe, C. D., Minter, E. J., Cameron, D. D., and Brockhurst, M. A. (2016). Shining a light on exploitative host control in a photosynthetic endosymbiosis. Curr. Biol. 26, 207–211. doi: 10.1016/j.cub.2015.11.052
Marschner, H., and Dell, B. (1994). Nutrient uptake in mycorrhizal symbiosis. Plant Soil 159, 89–102. doi: 10.1007/BF00000098
Moeller, H. V., Johnson, M. D., and Falkowski, P. G. (2011). Photoacclimation in the phototrophic marine ciliate mesodinium rubrum (ciliophora)1. J. Phycol. 47, 324–332. doi: 10.1111/j.1529-8817.2010.00954.x
Moeller, H. V., Peltomaa, E., Johnson, M. D., and Neubert, M. G. (2016). Acquired phototrophy stabilises coexistence and shapes intrinsic dynamics of an intraguild predator and its prey. Ecol. Lett. 19, 393–402. doi: 10.1111/ele.12572
Mougi, A., and Kondoh, M. (2012). Diversity of interaction types and ecological community stability. Science 337, 349–351. doi: 10.1126/science.1220529
Müller, J. P., Hauzy, C., and Hulot, F. D. (2012). Ingredients for protist coexistence: competition, endosymbiosis and a pinch of biochemical interactions. J. Anim. Ecol. 81, 222–232. doi: 10.1111/j.1365-2656.2011.01894.x
Ng, K. M., Ferreyra, J. A., Higginbottom, S. K., Lynch, J. B., Kashyap, P. C., Gopinath, S., et al. (2013). Microbiota-liberated host sugars facilitate post-antibiotic expansion of enteric pathogens. Nature 502, 96–99. doi: 10.1038/nature12503
Pachepsky, E., Taylor, T., and Jones, S. (2002). Mutualism promotes diversity and stability in a simple artificial ecosystem. Artif. Life 8, 5–24. doi: 10.1162/106454602753694747
Pado, R. (1965). Mutual relation of protozoans and symbiotic algae in Paramaecium bursaria. I. The influence of light on the growth of symbionts. Folia Biol. 13, 173–182.
Peay, K. G., Belisle, M., and Fukami, T. (2012). Phylogenetic relatedness predicts priority effects in nectar yeast communities. Proc. R. Soc. B Biol. Sci. 279, 749–758. doi: 10.1098/rspb.2011.1230
Pröschold, T., Darienko, T., Silva, P. C., Reisser, W., and Krienitz, L. (2011). The systematics of Zoochlorella revisited employing an integrative approach. Environ. Microbiol. 13, 350–364. doi: 10.1111/j.1462-2920.2010.02333.x
R Core Team (2020). R: A Language and Environment for Statistical Computing. Vienna: R Foundation for Statistical Computing.
Salsbery, M. E., and DeLong, J. P. (2018). The benefit of algae endosymbionts in Paramecium bursaria is temperature dependent. Evol. Ecol. Res. 19, 669–678.
Stoecker, D. K., Johnson, M. D., de Vargas, C., and Not, F. (2009). Acquired phototrophy in aquatic protists. Aquat. Microb. Ecol. 57, 279–310. doi: 10.3354/ame01340
van de Voorde, T. F. J., van der Putten, W. H., and Bezemer, T. M. (2011). Intra- and interspecific plant–soil interactions, soil legacies and priority effects during old-field succession. J. Ecol. 99, 945–953. doi: 10.1111/j.1365-2745.2011.01815.x
Vannette, R. L., and Fukami, T. (2014). Historical contingency in species interactions: towards niche-based predictions. Ecol. Lett. 17, 115–124. doi: 10.1111/ele.12204
Weidlich, E. W. A., von Gillhaussen, P., Max, J. F. J., Delory, B. M., Jablonowski, N. D., Rascher, U., et al. (2018). Priority effects caused by plant order of arrival affect below-ground productivity. J. Ecol. 106, 774–780. doi: 10.1111/1365-2745.12829
Weis, D. S. (1974). Sparing effect of light on bacterial consumption of Paramecium bursaria. Trans. Am. Microsc. Soc. 93, 135–140. doi: 10.2307/3225232
Wernegreen, J. J. (2012). Mutualism meltdown in insects: bacteria constrain thermal adaptation. Curr. Opin. Microbiol. 15, 255–262. doi: 10.1016/j.mib.2012.02.001
Keywords: acquired photosynthesis, ciliates, Colpidium, Paramecium bursaria, protist microcosm
Citation: Hsu V and Moeller HV (2021) Metabolic Symbiosis Facilitates Species Coexistence and Generates Light-Dependent Priority Effects. Front. Ecol. Evol. 8:614367. doi: 10.3389/fevo.2020.614367
Received: 05 October 2020; Accepted: 09 December 2020;
Published: 08 January 2021.
Edited by:
Guillaume Chomicki, Durham University, United KingdomCopyright © 2021 Hsu and Moeller. This is an open-access article distributed under the terms of the Creative Commons Attribution License (CC BY). The use, distribution or reproduction in other forums is permitted, provided the original author(s) and the copyright owner(s) are credited and that the original publication in this journal is cited, in accordance with accepted academic practice. No use, distribution or reproduction is permitted which does not comply with these terms.
*Correspondence: Veronica Hsu, dmVyb25pY2Foc3VAdWNzYi5lZHU=; Holly V. Moeller, aG9sbHkubW9lbGxlckBsaWZlc2NpLnVjc2IuZWR1