- 1Department of Life Sciences, National Cheng Kung University, Tainan, Taiwan
- 2Faculty of Commerce, Otaru University of Commerce, Otaru, Japan
Mutualism is one of the major interaction types occurring in ecological communities, and has significant consequences for population dynamics and community structure. A major feature of mutualism is its context dependence; i.e., potentially mutualistic relationships may become parasitic or neutral depending on the abiotic conditions and presence of other species. Recently, stage structure has been recognized as an additional important factor underlying the context-dependence of mutualism, giving rise to the concept of stage-structured mutualism, which states that mutualistic partners at one life-history stage may become parasitic at other life-history stages. However, the ecological consequences of such a stage-structured interaction remain unknown. Here, we present a simplified one host–one partner model with a juvenile–adult stage structure of the host species, in which the partner species improves fecundity of the adult host but suppresses growth and maturation of the juvenile host. Numerical simulations identified two ecological conditions for stage-specific parasitism to counterintuitively increase host abundance: (i) the benefits derived by the adult host from the partner species are large and the costs imposed on the juvenile host by the partner species are small (the partner investment hypothesis), and (ii) host population growth is limited by adult reproduction instead of juvenile maturation (the ontogenetic asymmetry hypothesis). Although the partner investment hypothesis appears more intuitive, exhaustive parameter exploration revealed that the ontogenetic asymmetric hypothesis would be more likely to operate. This counterintuitive result implies that the dynamics of mutualistic interactions are more complex than previously thought. The present study provides novel insights into the ecological consequences of mutualism and illustrates the importance of the ontogenetic perspective of mutualism in community ecology. Further research is required in both empirical and theoretical approaches to better understand the mechanisms by which stage-structured mutualism mediates population dynamics and community structure in more complex systems.
Introduction
Mutualism, a reciprocally beneficial association between two species, is one of the major interaction types that shape and drive ecological communities (Bascompte and Jordano, 2014; Bronstein, 2015). Although it has been acknowledged that mutualism can critically affect population dynamics and community structure, the ecological consequences of mutualism remain relatively poorly understood in comparison with those of other interaction types such as predation and competition (Bascompte and Jordano, 2014; Bronstein, 2015). A major hindrance in our understanding of the ecological consequences of mutualism is its context-dependence, i.e., potentially mutualistic relationships between species may become parasitic or neutral depending on factors such as the abiotic environment and presence of other species (Chamberlain et al., 2014; Hoeksema and Bruna, 2015). Such a drastic change in the relationship between mutualistic species is referred to as the mutualism–parasitism continuum (Karst et al., 2008; Mandyam and Jumpponen, 2015). Detailed investigations of the context-dependent mutualism are required to better understand the mechanisms by which it mediates population dynamics and community structure (Bronstein, 2015).
Recently, stage structure has been recognized as an additional important factor underlying the context-dependence of mutualism, giving rise to the concept of stage-structured mutualism (reviewed by Nakazawa, 2020). Notably, ontogenetic growth is the most fundamental biological aspect of an organism (Wilbur, 1980; Werner and Gilliam, 1984), and the costs and benefits of mutualism may vary during the course of ontogenetic growth. Consequently, mutualistic relationships may change ontogenetically. More specifically, mutualistic partners at one life-history stage may become parasitic at other stages (hereafter referred to as stage-specific parasitism). For example, it is often observed that symbiotic microbes become mutualistic or parasitic, depending on the ontogenetic stage of the host plant (Merrild et al., 2013; Bachelot et al., 2018). Furthermore, some ant species protect myrmecophytic host plants against herbivores and competing plants but destroy their floral organs thereby impairing reproductive success of the host plant (Stanton et al., 1999; Palmer et al., 2010). Although the extent to which mutualism is likely to be parasitic during the course of ontogenetic development in nature remains unclear, stage-specific parasitism would be widespread in mutualistic interactions because mutualism is highly context-dependent and stage-structured (Nakazawa, 2020; see section “Results and Discussion” for empirical examples). At present, however, little is known about how stage-specific parasitism can mediate the population dynamics of host–partner interactions, because ecologists have long overlooked the importance of stage structure in community ecology (reviewed by Miller and Rudolf, 2011; de Roos and Persson, 2013; Nakazawa, 2015, 2017; de Roos, 2020b).
Here, we address the possibility that stage-specific parasitism may positively affect the long-term population dynamics of host species, in spite of its negative effects during a certain life-history stage. Traditionally, the parasitic aspect of mutualism has been considered to negatively affect the host species, hence the term “parasitism” is included in the mutualism–parasitism continuum (Karst et al., 2008; Mandyam and Jumpponen, 2015). Contrary to the traditional idea, “parasitism” (i.e., short-term over-exploitation by mutualistic partners) may be beneficial in stage-structured mutualism in the long run. An intuitive explanation for such a positive effect is that short-term exploitation by parasitic partners can be considered an investment for future fitness, by which hosts can increase partner abundance and then derive greater benefits at subsequent life-history stages. The feasibility of this notion remains untested in stage-structured systems, although a similar idea has been developed for non-structured mutualism (Bachelot and Lee, 2020). If stage-specific parasitic aspects of mutualism are long-term beneficial to host species, it implies the need to reconsider conventional views of context-dependent mutualism or the mutualism–parasitism continuum without stage structure.
In this study, we developed and analyzed a simple mathematical model for stage-structured host–partner dynamics, considering a juvenile–adult stage structure for the host species and a partner species that is parasitic and mutualistic to the juvenile and adult stages of the host species, respectively (Figure 1). Although the model was simple, it provided a good opportunity to reconsider the ecological consequences of mutualism in general. Our specific aim was to theoretically illustrate that juvenile-specific parasitism can increase host abundance in the long run. We further attempted to identify the ecological conditions for the counterintuitive outcome by exhaustively exploring the parameter space.
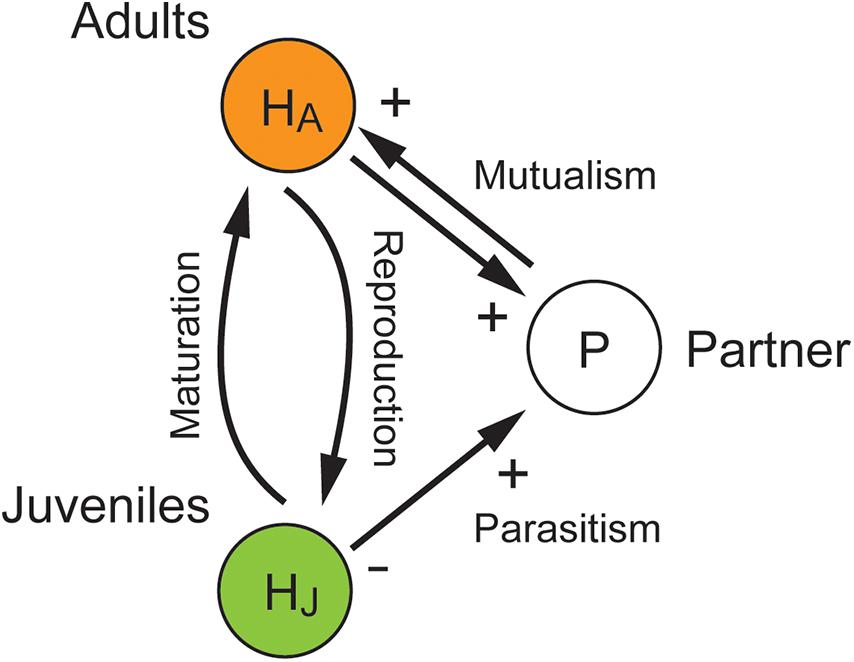
Figure 1. Conceptual illustration of the model investigated in the present study. The host species exhibits a juvenile–adult stage structure and the partner species is parasitic and mutualistic to the juvenile and adult stages of the host species, respectively.
Model
We considered a one host–one partner system with a juvenile–adult stage structure of the host species, in which the partner species improved the fecundity of the adult host (i.e., adult-specific mutualism), whereas it suppressed growth and maturation of the juvenile host without any benefits (i.e., juvenile-specific parasitism) (Figure 1). To model the adult-specific mutualism, we adopted the consumer-resource approach, which states that mutualistic benefits are obtained in return for rewarding the partners (Holland and DeAngelis, 2010). We thus assumed that the adult host and the partner species exploited each other to increase their own demographic performance. This system can be formulated as follows:
where Hi (i = J or A) is the juvenile or adult abundance of the host species and P is the abundance of the partner species. The adult host produces offspring with the basic reproduction rate rA and the additional term due to mutualism with the partner species, wherein aA and bA indicate the rate of partner exploitation and its conversion efficiency, respectively (Eq. 1a). The juvenile host matures to adulthood with the basic maturation rate rJ, which is reduced ascribable to exploitation by the partner species with the rate aPJ (Eqs. 1a and 1b). The max function in the maturation term ensures that the realized maturation rate is non-negative. The abundance of the partner species increases with the intrinsic growth rate rP and the additional terms due to the exploitation of both juvenile and adult hosts, wherein aPi and bPi indicate the rate of stage-specific host exploitation and its conversion efficiency, respectively (Eq. 1c). Note that adult-specific mutualism is facultative when rA or rP is positive, and obligate when it is zero. It is also assumed that bAaA-aPA > 0 and bPAaPA-aA > 0 for the short-term benefits of adult-specific mutualism to be net positive for both the adult host and partner species. All populations exhibit density-dependent death rate dij (i, j = J or A) or dP.
Species Persistence and Coexistence
Invasion analysis ensured that both the host and partner species could persist (i.e., not go extinct), and thus coexist in general. First, the persistence condition of the host species was derived as follows:
where the first term indicates the expected probability of a juvenile individual surviving to adulthood, and the second term indicates the expected number of juvenile individuals produced by an adult individual during the adult period. This represents the basic reproductive number. The host species can invade the host-free equilibrium and persist if the basic reproductive number is greater than one at H. Obviously, the inequality (2a) always holds true, regardless of the presence (P∗ > 0) or absence (P∗ = 0) of the partner species, as long as the maturation rate is positive (rJ-aPJp∗ > 0) and the adult-specific mutualism is net beneficial for the adult host (bAaA-aPA > 0). Similarly, the partner species can invade the partner-free equilibrium and persist if its per-capita population growth is positive at P∗= 0. The persistence condition of the partner species was derived as follows:
This condition also always holds true, regardless of the presence (Hi∗ > 0) or absence (Hi∗ = 0) of the host species, because the adult-specific mutualism is net beneficial for the partner species (bPAaPA-aA > 0). Overall, it was illustrated that the host and partner species can coexist for any initial condition if the maturation rate is positive at the steady state. Furthermore, as long as this condition is met, their coexistence is robust, regardless of whether the adult-specific mutualism is facultative (rA, rP > 0) or obligate (rA, rP = 0).
Numerical Simulations
We numerically investigated the steady state of host abundance along the gradient of the juvenile-specific parasitism rate aPJ. To assess the robustness of the results, we performed a sensitivity analysis by manipulating all parameters within a wide range of values. Simulations were run for 103 time steps, and the long-term average abundance of the host species was evaluated for the last 500 time steps to remove transient dynamics. The initial population abundances were chosen randomly between 0 and 1 for each simulation. As far as we observed, the dynamics always converged to a single equilibrium point.
Ecological Conditions for “Beneficial” Parasitism
In addition, we attempted to identify the ecological conditions under which juvenile-specific parasitism increased host abundance. We considered two hypothetical scenarios, one intuitive and the other counterintuitive. The intuitive scenario postulated juvenile-specific parasitism as an investment for future fitness, through which the host species could increase partner abundance and then derive greater benefits at the adult stage. A similar idea has been developed for non-structured mutualism (Bachelot and Lee, 2020). In this scenario, the benefits derived by the adult host from the partner species are large and the costs imposed on the juvenile host by the partner species (i.e., parasitic exploitation aPJ) are small. For this mechanism to work, the adult host is required to efficiently utilize the partner species (i.e., high exploitation rate aA and high conversion efficiency bA) as well as having weak parasitism at the juvenile stage. This mechanism is hereafter referred to as the partner investment hypothesis.
The other hypothetical scenario highlighted the limiting factor of population growth (Figure 2). The partner species suppresses juvenile maturation while improving adult reproduction (Eq. 1a). This would shift the host population toward a more juvenile-dominated state where the host population growth is likely to be limited by maturation rather than reproduction. In this scenario, if the host population is a priori dominated by adults and population growth is limited by adult reproduction, then juvenile-specific parasitism may increase host abundance by balancing the skewed stage distribution and relaxing the reproduction-limited population growth. For this mechanism to work, the host species is required to have low reproductive performance. This mechanism is hereafter referred to as the ontogenetic asymmetry hypothesis (sensu de Roos, 2020b).
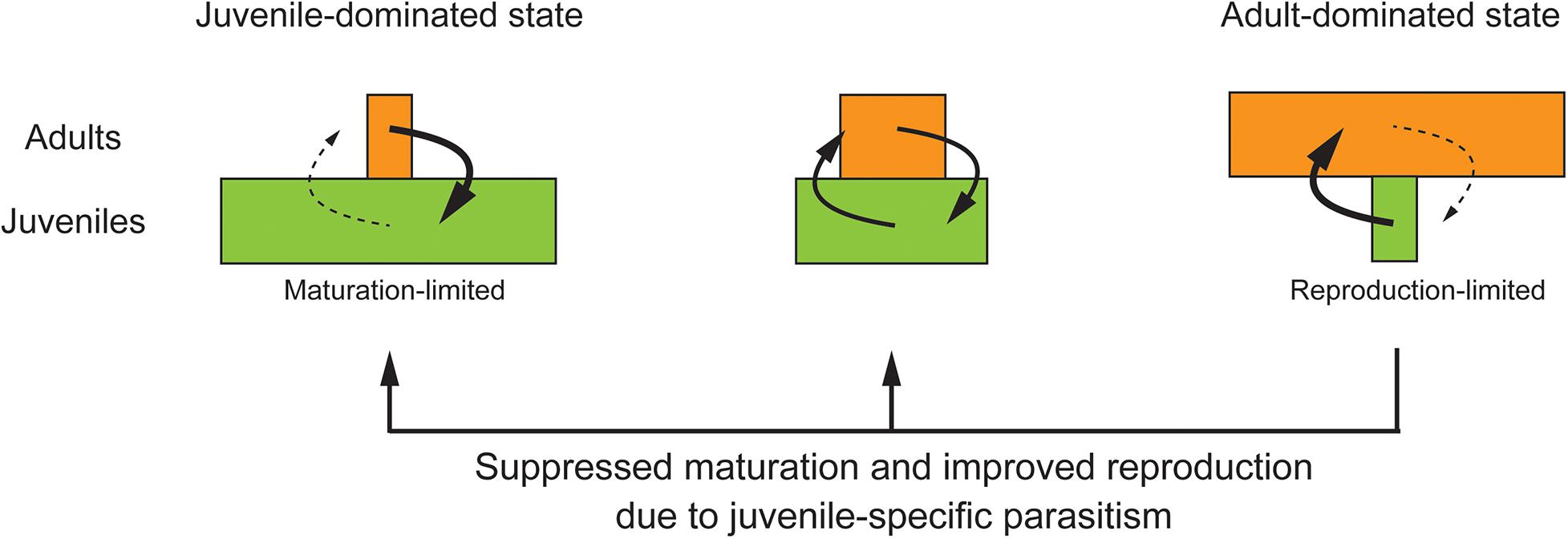
Figure 2. The ontogenetic asymmetry hypothesis based on changes in the limiting factors of population growth and stage distribution due to juvenile-specific parasitism. (Left) Maturation flow is considerably smaller than reproduction flow, leading to the juvenile-dominated state. (Right) Reproduction flow is considerably smaller than maturation flow, leading to the adult-dominated state. (Middle) Both maturation and reproduction limitations are relaxed, resulting in the highest population growth. Juvenile-specific parasitism suppresses juvenile maturation while increasing partner abundance and improving adult reproduction, thereby shifting the host population toward a more juvenile-dominated state. The ontogenetic asymmetry hypothesis predicts that if the host population is a priori dominated by the adult stage and the population growth is limited by adult reproduction, then juvenile-specific parasitism may increase host abundance by balancing the skewed stage distribution and relaxing the population growth limit.
The two scenarios described above are apparently contradictory because the partner investment hypothesis requires relatively high reproductive performance of the adult host, whereas the ontogenetic asymmetry hypothesis requires the opposite. To determine the mechanism that is more likely to operate, we tested for a correlation between the reproductive performance of the adult host and consequences of juvenile-specific parasitism for the steady-state host abundance. For the reproductive performance of the adult host, the long-term average of the expected reproductive success was calculated as follows (see Eq. 2a for details):
For the consequences of juvenile-specific parasitism for host abundance, a difference in the long-term average of the total host abundance HJ + HA in the presence and absence of weak parasitism (aPJ = 0.001) was calculated. Positive and negative values indicated an increase and decrease, respectively, in the host abundance at the steady state due to juvenile-specific parasitism. We used linear regression analysis to test the correlation between the expected reproductive success (Eq. 3) and a parasitism-induced change in the host abundance. A positive correlation suggests that the partner investment hypothesis is more likely to operate, whereas a negative correlation suggests that the ontogenetic asymmetry hypothesis is more likely to operate. 50,000 simulations were run with all parameters varied randomly up to four times the default values, of which we extracted approximately 75% of the simulations for the correlation analysis based on the prerequisite that the adult-specific mutualism was net beneficial for both the adult host and partner species (i.e., bAaA-aPA > 0 and bPAaPA-aA > 0). We did not perform a significance test to estimate the P-value because such a correlation analysis of numerical results is inappropriate (White et al., 2014); instead, we estimated the effect size, i.e., the correlation coefficient R in correlation analysis (Field, 2009).
Results and Discussion
The results illustrated that juvenile-specific parasitism (i.e., positive values of aPJ) can potentially increase host abundance in the long run (Figure 3). Specifically, the total host abundance can increase and vary unimodally with small values of aPJ for some parameter sets, or decrease monotonically with the parameter. Below, we describe the consequences of varying different parameters for the host–partner dynamics and the mechanisms underlying the positive effect of juvenile-specific parasitism on the host species.
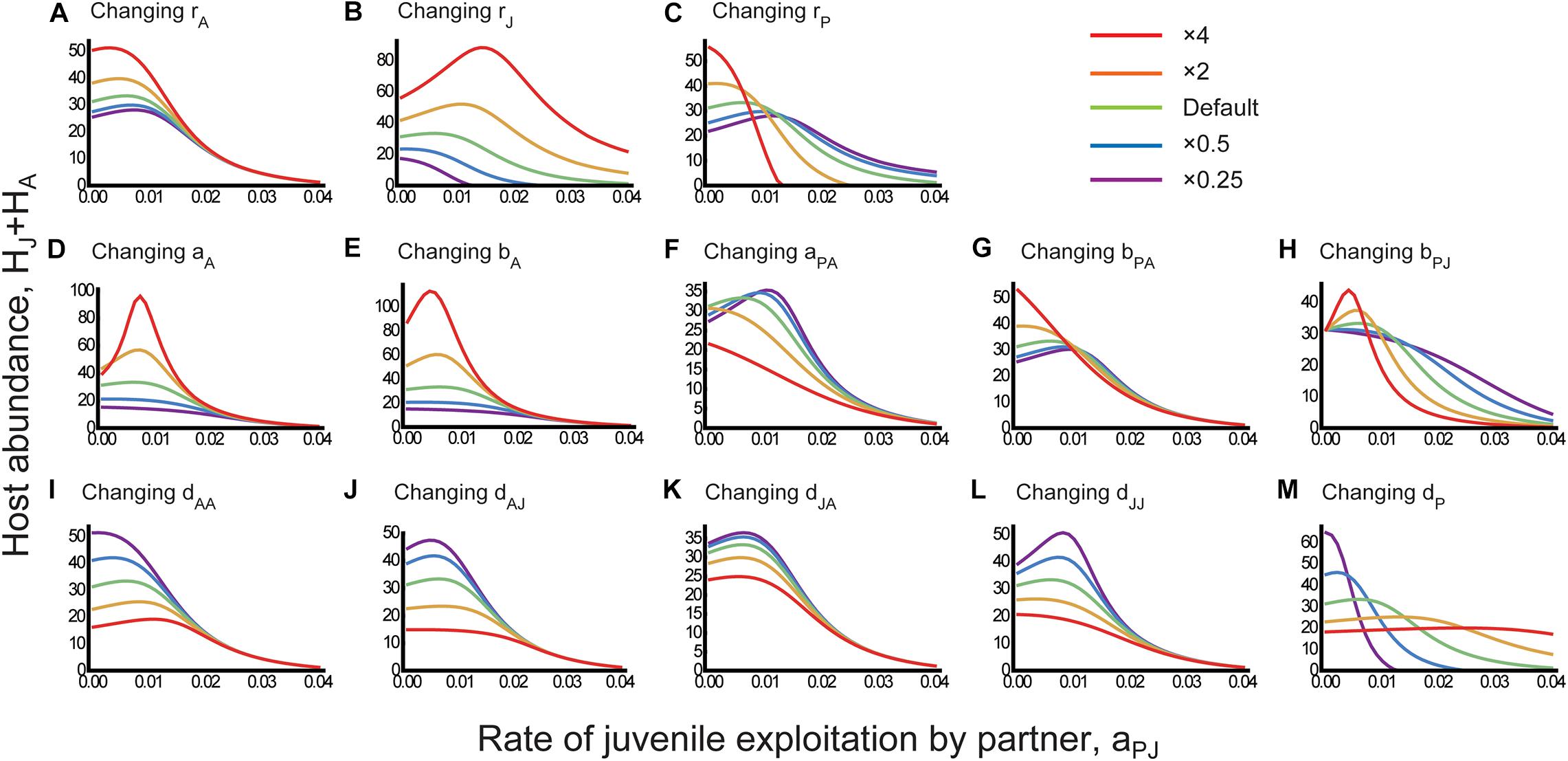
Figure 3. Parameter effects on the consequences of juvenile-specific parasitism for host abundance. The total host abundance HJ + HA is plotted against the rate of juvenile exploitation by the partner species aPJ for different parameter sets. One parameter is manipulated in each panel: (A) rA, (B) rJ, (C) rP, (D) aA, (E) bA, (F) aPA, (G) bPA, (H) bPJ, (I) dAA, (J) dAJ, (K) dJA, (L) dJJ, and (M) dP. Different line colors indicate the magnitude of parameter manipulation in comparison with the default value, as indicated in the top right-hand corner. The default parameters were ri = rP = 1, aA = 0.02, aPA = 0.01, bA = bPi = 5, dii = dP = 0.05, dJA = 0.01, and dAJ = 0.1.
Partner Investment Versus Ontogenetic Asymmetry
The results supported the more intuitive expectation (i.e., the partner investment hypothesis) that if the benefits derived by the adult host from the partner species are large and the costs imposed on the juvenile host by the partner species are small, juvenile-specific parasitism would increase host abundance in the long run. More specifically, the model predicted that the host abundance could increase with small values of the parameter aPJ when the adult host effectively utilized the partner species (i.e., high aA and bA) (Figures 3D,E) and adult-specific mutualism was not too costly for the adult host (i.e., low aPA) (Figure 3F). These predictions suggest that the partner investment hypothesis can explain the outcome that juvenile-specific parasitism can increase host abundance when the adult host has high reproductive performance.
At the same time, our results supported the ontogenetic asymmetry hypothesis as well (Figure 2). Specifically, the expected reproductive success (Eq. 3) in the absence of juvenile-specific parasitism was low when the basic reproduction rate rA was low (Figure 4A), the basic maturation rate rJ was high (Figure 4B), or the intrinsic growth rate rP of the partner species was low (Figure 4C). Under these parameter conditions, the host abundance could increase with aPJ (see Figures 3A–C). In conjunction, it is suggested that juvenile-specific parasitism can increase host abundance when the host population is reproduction-limited compare corresponding panels in Figures 3, 4. Exceptional patterns were also found where the expected reproductive success was high for parameter conditions under which the host abundance could increase with aPJ. However, such exceptions were observed when the partner investment hypothesis was supported where the adult host effectively utilized the partner species (i.e., high aA and bA) and mutualism was not too costly for the adult host (i.e., low aPA) (compare Figures 3D–F, 4D–F).
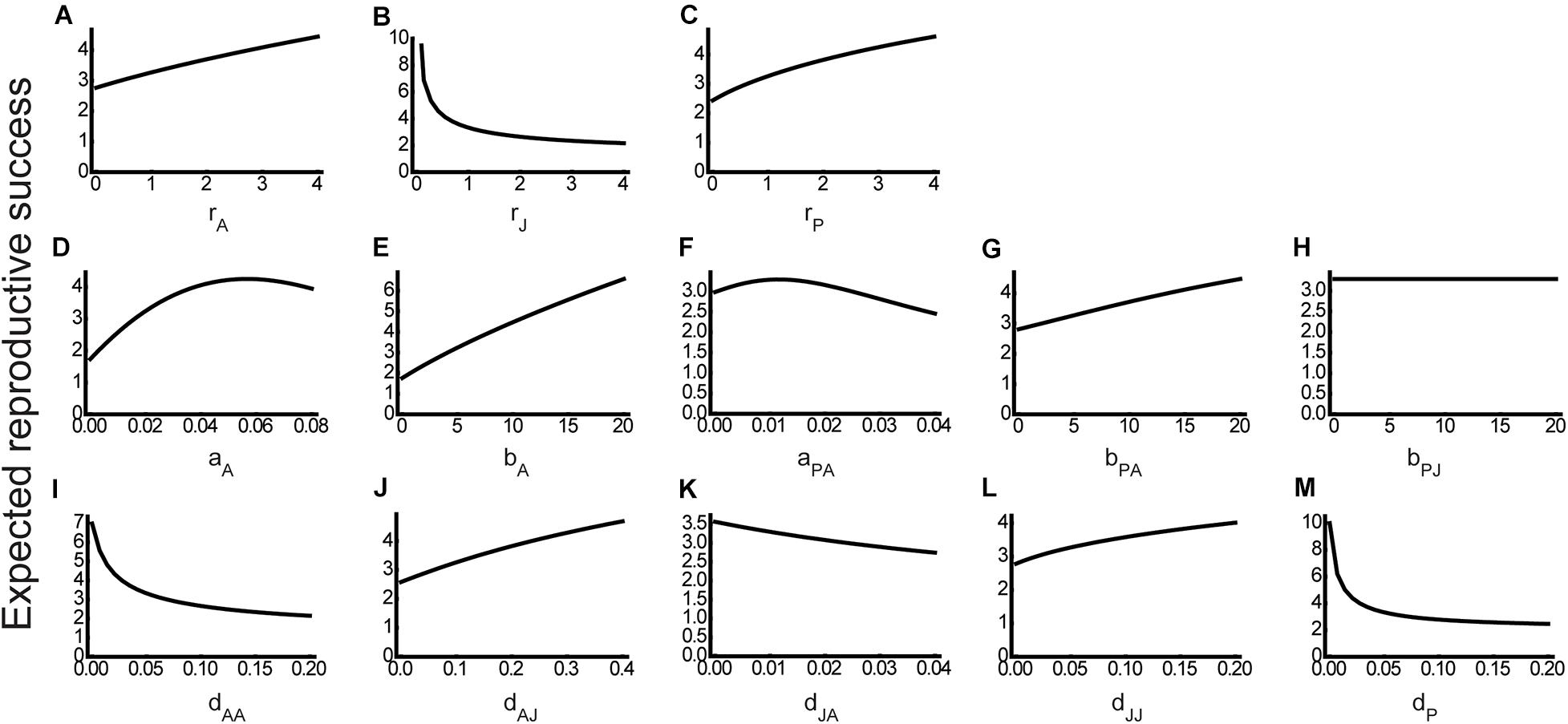
Figure 4. Parameter effects on the expected reproductive success in the absence of juvenile-specific parasitism (aPJ = 0). In each panel, the expected reproductive success at the steady state is calculated along a different parameter: (A) rA, (B) rJ, (C) rP, (D) aA, (E) bA, (F) aPA, (G) bPA, (H) bPJ, (I) dAA, (J) dAJ, (K) dJA, (L) dJJ, and (M) dP. Note that in panel (H), the expected reproductive success is constant against the conversion efficiency of the juvenile-specific parasitism bPJ because this parameter is meaningless in the absence of juvenile-specific parasitism. Other parameter values are the same as in Figure 3.
Overall, our results identified both the partner investment hypothesis and ontogenetic asymmetry hypothesis as feasible mechanisms underlying the positive consequences of stage-specific parasitism for host abundance. To determine the mechanism that was more likely to operate, we tested for a correlation between the expected reproductive success and a weak parasitism-induced change in host abundance (corresponding to the slope of curves at aPJ = 0 in Figure 3) by a more exhaustive exploration of the parameter space. The result showed that a parasitism-induced change in host abundance was more likely to be positive when the expected reproductive success was low (Figure 5), implying that juvenile-specific parasitism is likely to increase host abundance if the host population growth is limited by adult reproduction. The correlation coefficient was R = 0.15 (i.e., small effect size) regardless of whether outliers were included or excluded. Although a significance test is inappropriate for such simulation results (White et al., 2014), the observed negative, but not positive, correlation suggests that the ontogenetic asymmetry hypothesis is more likely to operate than the partner investment hypothesis. Although apparently counterintuitive, this conclusion is not unreasonable in terms of the limiting factor for population growth. Note that juvenile-specific parasitism suppresses juvenile maturation while increasing the partner species and improving adult reproduction (Eq. 1a). If the host population growth is maturation-limited, suppressed maturation due to juvenile-specific parasitism can further decrease the population growth, whereas improved reproduction due to adult-specific mutualism would have little effect. In contrast, if the host population growth is reproduction-limited, suppressed maturation due to juvenile-specific parasitism would have little effect on population growth, whereas improved reproduction due to adult-specific mutualism could increase the population growth.
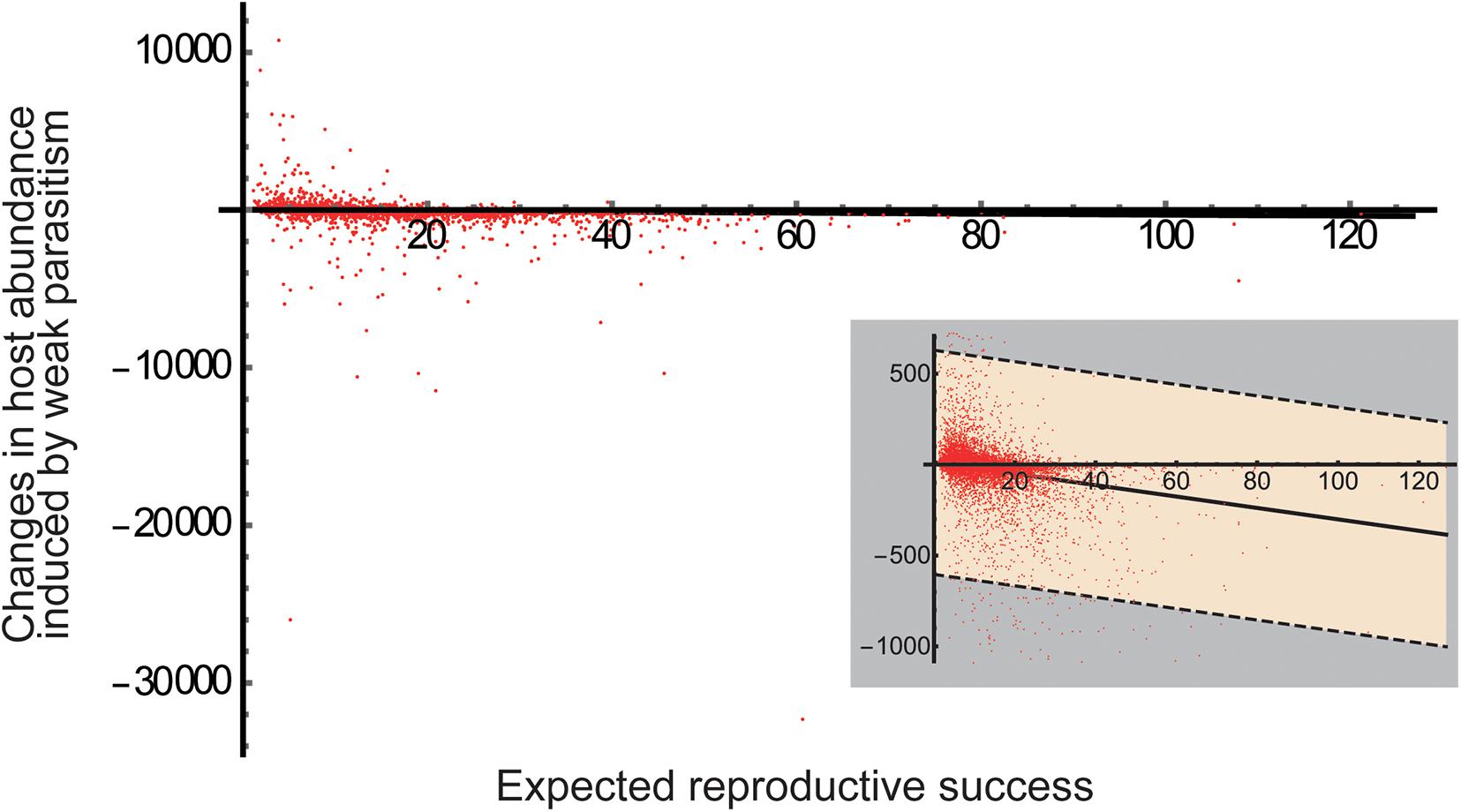
Figure 5. Linear correlation between weak parasitism-induced changes in host abundance and expected reproductive success. The horizontal axis represents the expected reproductive success at the steady state in the absence of juvenile-specific parasitism (aPJ = 0). The vertical axis represents a difference in the total host abundance in the presence and absence of weak parasitism (aPJ = 0.001) at the steady state. The red dots indicate the results of ca. 75% of 50,000 simulations that met the assumption that adult-specific mutualism was net beneficial for both the adult host and partner species (i.e., bAaA-aPA > 0 and bPAaPA-aA > 0). The small panel provides an enlarged view of the results. The solid and dashed lines indicate the predicted regressions and 95% confidence intervals, respectively. Other parameter values are the same as in Figure 3.
It must be noted that the above theoretical arguments regarding juvenile-specific parasitism presented can apply to adult-specific parasitism (Supplementary Material). Suppose that the partner species improves maturation of the juvenile host (i.e., juvenile-specific mutualism), whereas it suppresses reproduction of the adult host. It is intuitively expected that adult-specific parasitism can increase the host abundance when the benefits derived by the juvenile host from the partner species are relatively large and the costs imposed on the adult host by the partner species are small (i.e., partner investment hypothesis). While this mechanism can work (Supplementary Figure S1), the results supported that ontogenetic asymmetry hypothesis is more likely to operate (Supplementary Figure S2). In other words, adult-specific parasitism can increase the host abundance when the host population growth is limited by juvenile maturation instead of adult reproduction.
Complex Dynamics of Stage-Structured Mutualism
We observed that the dynamics of stage-structured mutualistic interactions were complex. For example, the host abundance could increase with aPJ when the adult host had a low basic reproduction rate rA (Figure 3A) and the partner species had a low intrinsic growth rate rP (Figure 3C). These predictions suggest that juvenile-specific parasitism is likely to increase (or decrease) the host abundance if adult-specific mutualism is obligate (or facultative). Moreover, the positive effect of juvenile-specific parasitism was greater when the juvenile host had a high basic maturation rate rJ (Figure 3B). Furthermore, host abundance could increase with aPJ when the partner species efficiently utilized the juvenile host (i.e., high bPJ) but inefficiently utilized the adult host (i.e., low bPA) (Figures 3G,H). These predictions are complicated, and it is difficult to ecologically interpret why obligate mutualism at the adult stage increased the benefits of juvenile-specific parasitism or why stage-specific relevant parameters (e.g., rA versus rJ and bPA versus bPJ) differentially influenced the consequences of juvenile-specific parasitism. Similarly, complex patterns were observed for the effects of density-dependent death rates dij (Figures 3I–M). We attribute such complex dynamics to the fact that the juvenile and adult hosts affect each other not only directly through the life cycle, but also indirectly through association with the partner species. For example, the adult host directly increases the juvenile host via reproduction, but indirectly harm the juvenile host by increasing parasitic exploitation. The feedback processes between life-history stages may explain the complex consequences of juvenile-specific parasitism in certain circumstances.
Stage-structured populations often exhibit complex and counterintuitive dynamics (Miller and Rudolf, 2011; de Roos and Persson, 2013; Nakazawa, 2015; de Roos, 2020b). For example, an increase in stage-specific mortality can increase the abundance of stage-structured populations, which is known as the hydra effect (Abrams, 2009). Multiple predator species competing for a shared prey species may coexist without competitive exclusion and rather assist each other to persist if they selectively feed on different life-history stages of the prey species, which is called emergent facilitation (de Roos et al., 2008). In addition, exploitation of different prey species at different life-history stages by a predator species (i.e., ontogenetic diet shift in apparent competition) can lead to alternative stable states where the community dynamics converge to different steady states depending on the initial condition (Schreiber and Rudolf, 2008; Guill, 2009; Nakazawa, 2011a,b). Recently, de Roos (2020b) described a phenomenon called the “double-handicapped loser” in a one resource–two consumers–one predator system, where an intermediate consumer can oust the opponent even when it is less efficient at utilizing the basal resource and more vulnerable to the top predator, if both consumers are stage-structured. Notably, ontogenetic asymmetry (i.e., differences in demographic performances between individuals of different life-history stages or body sizes) has been identified as the major mechanism underlying all these counterintuitive dynamics (see references cited above). In stage-structured populations, ontogenetic asymmetry can create a life-history bottleneck in population growth. Given the density dependence of stage-specific demographic performance (e.g., density-dependent reproduction and food-dependent maturation), a reduction in the population abundance at a certain life-history stage (e.g., due to increased mortality and predation) can increase the biomass flux (e.g., reproduction and maturation) within a population even if it diminishes per-capita demographic performances. Indeed, this mechanism can readily explain the thinning effect commonly observed in agriculture, aquaculture, and forestry, where the removal of certain individuals can increase the total productivity. In the present system, the partner species may not only directly increase the host abundance via adult-specific mutualism but also indirectly improve the reproduction-limited population growth by suppressing maturation to adulthood and relaxing competition (density-dependent mortality) at the adult stage.
We also note that juvenile-specific parasitism by a mutualistic partner theoretically resemble cannibalism (i.e., intraspecific predation) in that it benefits the adult stage while imposing negative effects on early life-history stages. There are many theoretical studies on how cannibalism can mediate population dynamics (reviewed by Claessen et al., 2004). While cannibalism has negative effects on a population (i.e., additional mortality of victims), it can also have positive effects, which can be divided into direct and indirect ones. The direct positive effect is the energy gain through consuming conspecifics. For example, if juveniles can utilize external resources inaccessible to adults, cannibalism may benefit a cannibalistic population through exploiting a broader ecological niche (van den Bosch et al., 1988; Diekmann et al., 2003). The indirect positive effect is reduced competition among victims, which in turn benefits the potential victims that survive. If density-dependent demographic performance of juveniles is non-linear, a reduction of their abundance may substantially increase their maturation rate thereby increasing adult abundance (Botsford, 1981; Fisher, 1987). We consider that these ideas of the direct and indirect positive effects are apparently similar to those of partner investment and ontogenetic asymmetry, respectively, in the present study (see above). In the context of cannibalism, however, the previous studies have focused mainly on investigating whether a cannibalistic population can persist or not and whether cannibalism will stabilize or destabilize the population dynamics (Claessen et al., 2004). So far, relatively few studies have explicitly addressed whether or when cannibalism can increase the population size. We therefore emphasize that the present study is significant not in terms of a comparison with the previous studies on cannibalism but in terms of providing a novel perspective on stage-structured mutualism.
Empirical Relevance
Here, we present some empirical examples of stage-specific parasitism by potentially mutualistic partners to which the present model (Eq. 1) applies or can be easily adapted.
- Nutritional mutualism between terrestrial plants and soil microbes (e.g., arbuscular mycorrhizae and rhizobia) is a representative example of context-dependent mutualism (Karst et al., 2008; Mandyam and Jumpponen, 2015). Symbiotic microbes have often been observed to become parasitic, depending on the ontogenetic stage of the host plant. Many studies have reported highly variably microbial effects, particularly during the early developmental stages, with both positive and negative effects being observed (Merrild et al., 2013; Bachelot et al., 2018). This is probably because the microbes do not provide sufficient nutrients to small seedlings, which cannot provide photosynthetic products as efficiently as adult plants. This represents a good example of juvenile-specific parasitism.
- Myrmecophytes, represented by plants such as Acacia and Macaranga, develop a defensive mutualistic relationship with ants, where they provide rewards (e.g., extrafloral nectaries, food bodies, and domatia) to symbiotic ants in return for protection against herbivores and competing plants (Heil and McKey, 2003; Heil, 2015). Some ants become detrimental to host plants, while being aggressive toward herbivores, at certain life-history stages. For example, Crematogaster nigriceps destroy floral organs of Acacia drepanolobium to reallocate plant resource toward vegetative growth, thereby impairing reproductive success of the host plant (Stanton et al., 1999; Palmer et al., 2010). Such castration behavior is observed in other ant species (Yu and Pierce, 1998; Izzo and Vasconcelos, 2002; Gaume et al., 2005). This is an example of adult-specific parasitism.
- Some ant species protect not only plants but also insects, such as Coccoidea and Lycaenidae. The Aphididae represent the most extensively studied group of ant-attended insects. They provide honeydew (sugar and water) to ants in return for protection against predators and parasitoids (Stadler and Dixon, 2005; Yao, 2014). In this system, as the aphid colony grows, ants sacrifice them for food because the cost of defending the increasing aphids exceeds the quantity and quality of the available per-capita honeydew (Breton and Addicott, 1992). Considering the asexual colony growth of aphids as ontogenetic growth of a superorganism (Hölldobler and Wilson, 1990), this example represents adult-specific parasitism.
- Pollination is the most representative example of mutualism. Plants require pollinators only at the reproductive stage, while they are often attacked by herbivores during their vegetative stage. Notably, major pollinators of many flowering plants are holometabolous insects that undergo metamorphosis, such as Hymenoptera (e.g., bees) and Lepidoptera (e.g., butterflies). Their larvae are generally antagonistic to plants and do not contribute to pollination (Wäckers et al., 2007). In particular, Lepidoptera larvae are crucial herbivores of plants (Hahn and Brühl, 2016; Rader et al., 2016). From the viewpoint of plants, such insects act as parasitic partners at the vegetative stage. In an extreme case, the hawkmoth Manduca sexta is a specialist herbivore of the jimsonweed Datura wrightii at the larval stage whereas it is a specialist pollinator of the host plant at the adult stage (Bronstein et al., 2009). This example represents a variant version of juvenile-specific parasitism as the partner (i.e., pollinating insects) is also stage-structured and often switches between different host plants following metamorphosis.
Interestingly, some of the above examples presented obligate mutualism where the host and/or partner species cannot survive in the absence of the other (e.g., arbuscular mycorrhizae, myrmecophytes, and hawkmoth M. sexta). In these examples, the parasitic aspects of mutualistic partners may provide long-term benefits to the host species, as shown by the present results that juvenile-specific parasitism can increase host abundance when adult-specific mutualism is less facultative (Figures 3A,C). Nakazawa (2020) introduced other potential examples of stage-specific parasitic aspects in stage-structured mutualism (e.g., seed dispersal and cleaning mutualism; see references therein).
An important implication of the present study for empirical research is that short-term observations may yield misleading conclusions about the long-term ecological consequences of mutualism, firstly because apparently mutualistic interactions may include parasitic stages, and secondly because short-term stage-specific parasitism may be beneficial in the long run. The ecological consequences of stage-structured mutualism remain largely understudied (Ke and Nakazawa, 2018; Nakazawa, 2020). Further research is required in both empirical and theoretical approaches to better understand how stage-structured mutualism can mediate population dynamics and community structure.
Evolutionary Perspectives
Although the present study investigated if and when juvenile-specific parasitism can increase host abundance, it should be cautioned that here we cannot discuss its evolutionary consequences because a larger population size does not necessarily indicate greater individual fitness. As a preliminary test, here we use an evolutionary invasion analysis to assess how the host species would evolve its relationship with the juvenile-specific partner in the present system. For this, we introduced few mutant individuals, which differed from the residents only in the rate of juvenile-specific parasitism aPJ, into the host population at the steady state. Numerical simulations of the resident–mutant competition revealed that the mutant could replace the resident only when its aPJ value was lower than that of the resident, implying that the host species would evolve the parameter aPJ toward zero (Figure 6). This situation resembles the tragedy of the commons in game theory (Hardin, 1968), i.e., at the individual level, it is more profitable to cut off juvenile-specific parasitic partners, even though they would contribute to an increase in host abundance in the long run, and eventually, less selective individuals with higher aPJ will be removed from the population. Indeed, plants have often been observed to ontogenetically change mutualistic partners in nutritional (Baudoin et al., 2002; Husband et al., 2002; Mougel et al., 2006; Houlden et al., 2008; Micallef et al., 2009; Chaparro et al., 2014; Sugiyama et al., 2014; Oono et al., 2015) and defensive mutualism (Fonseca and Benson, 2003; Dejean et al., 2008; Palmer et al., 2010; Miller and Flynn, 2014; Koch et al., 2016). In these examples, host plants may select out parasitic partners. From an evolutionary perspective, it would be interesting to determine if and how stage-specific parasitism would persist under such conditions.
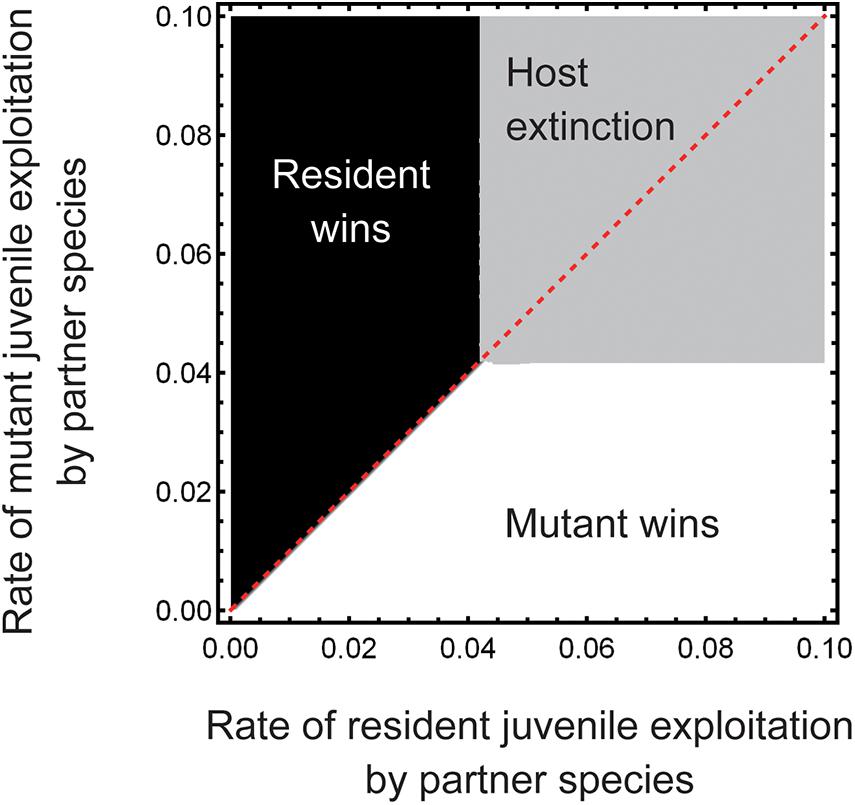
Figure 6. Pairwise invasibility plots. The horizontal and vertical axes represent the rates of resident and mutant juvenile exploitation by the partner species, respectively. The white region indicates mutant dominance, whereas the black region indicates expulsion of the mutant by the resident. The gray region indicates extinction of the host species. The red dashed line indicates a one-to-one correspondence. The origin of the graph indicates the evolutionarily stable strategy where any mutants cannot invade the population, and thus, evolution does not occur. Other parameter values are the same as in Figure 3.
We propose three mechanisms to explain the maintenance of potentially beneficial but evolutionarily maladaptive stage-specific parasitism. First, the partner species may also evolve to overcome the anti-parasitism strategy (i.e., ontogenetic partner shift) of the host species. Second, the anti-parasitism strategy may impose some fitness costs on the host species. For example, selective host individuals with lower aPJ can avoid juvenile-specific parasitism, but may present slower growth than the less selective individuals. Third, the spatial structure may allow the coexistence of selective and less selective host populations. Specifically, local populations of less selective host individuals with higher aPJ would grow faster due to beneficial stage-specific parasitism and disperse to other habitats more frequently than selective host populations, which is known as the competition–colonization trade-off (Levins and Culver, 1971; Calcagno et al., 2006). The importance of spatial structure has been acknowledged in evolutionary game theory (Roca et al., 2009; Nowak et al., 2010). To theoretically test these ideas, the present model (Eq. 1) should be extended to include coevolutionary processes, energetic constraints, and spatial structure as well as ontogenetic partner shifts between multiple partners in future work (Nakazawa, in preparation).
Model Limitations and Future Directions
Here, we briefly discuss the limitations of the present model (Eq. 1) and suggest future directions for model improvement to better understand the ecological consequences of stage-structured mutualism (see above for evolutionary perspectives). First, we note that the present model assumes that the partner species is always parasitic to the juvenile host. Although this was assumed to explicitly describe stage-specific parasitism, the type of the juvenile-specific interaction may vary along the mutualism-parasitism continuum, similarly to that of the adult-specific interaction. This implies that the juvenile host may also exploit the partner species. In this case, if partner exploitation by the juvenile host is intense and net parasitic to the partner species, it can have indirect negative indirect effects on the adult host due to the reduced mutualistic benefits. This may lead to another counterintuitive outcome where partner exploitation by the juvenile host may counterintuitively decreases the host abundance.
It is also crucial to study multispecies systems beyond a pairwise host–partner interaction. As mentioned above, the host species may not only cut off the parasitic partner, but also obtain other more profitable partners during the course of ontogenetic growth (references cited above). In a previous study, Nakazawa (2020) modeled a stage-structured one host–two partner system in which the host was associated with different partner species at the juvenile and adult stages. The model a priori assumed that the partners were stage-specific specialists with fixed interaction strengths. It would be interesting to consider a situation where ontogenetic partner shifts occur more dynamically, based on the optimal partner choice and inter-partner competition for the rewards provided by the host (Nakazawa, in preparation).
Community network models are particularly important for describing multiple host–partner interactions. However, to the best of our knowledge, the stage structure has not been included in any existing mutualistic network model (e.g., Okuyama and Holland, 2008; Valdovinos et al., 2013; Bascompte and Jordano, 2014). This is also the case for hybrid community networks, which integrate the different interaction types, such as mutualism and antagonism (parasitism) (e.g., Melián et al., 2009; Allesina and Tang, 2012; Mougi and Kondoh, 2012; Sauve et al., 2014; Suweis et al., 2014; Lurgi et al., 2016). Although the importance of stage structure in community ecology has been recognized in recent years (Miller and Rudolf, 2011; de Roos and Persson, 2013; Nakazawa, 2015, 2017; de Roos, 2020b), stage-structured network models remain limited to food web systems consisting only of purely antagonistic interactions (Mougi, 2017; de Roos, 2020a). Future theoretical work should incorporate the ontogenetic perspective (age, stage, and body mass) into mutualistic and hybrid community networks.
Conclusion
Stage-structured (size-structured) prey–predator interactions have been extensively studied for better describing food-web dynamics (Miller and Rudolf, 2011; de Roos and Persson, 2013; Nakazawa, 2015, 2017; de Roos, 2020b). In contrast, little is known about the ecological consequences of stage-structured mutualism. In this study, we numerically investigated if and under which conditions juvenile-specific parasitism by a mutualistic partner species can increase host abundance in a pairwise stage-structured mutualism. The results identified the following two ecological conditions:
(i) The benefits derived by the adult host from the partner species are relatively large and the costs imposed on the juvenile host by the partner species are small (partner investment hypothesis).
(ii) The host population growth is limited by adult reproduction instead of juvenile maturation (ontogenetic asymmetry hypothesis).
Although both these hypotheses were found to be feasible, extensive numerical simulations revealed that the ontogenetic asymmetry hypothesis was more likely to operate than the partner investment hypothesis. These results suggest that we will need to more carefully interpret the ecological consequences of mutualism. This is one of the few studies to examine the ecological consequences of stage-structured mutualism (Ke and Nakazawa, 2018; Nakazawa, 2020). Further research is required in both empirical and theoretical approaches to better understand the mechanism by which stage-structured mutualism mediates population dynamics and community structure in more complex systems. Furthermore, it is also important to study the evolutionary dynamics of stage-structured mutualism and in particular, the maintenance of stage-specific parasitism in response to anti-parasitism strategies. We believe that our work will contribute to the establishment of ontogenetic perspectives for the ecology of mutualism and provide a basis for future investigations and discussions on broader ecological aspects, including biodiversity conservation and ecosystem management.
Data Availability Statement
The original contributions presented in the study are included in the article/Supplementary Material, further inquiries can be directed to the corresponding authors.
Author Contributions
TN conceived the idea, carried out the model analysis, and wrote the draft. NK carried out the statistical analysis and helped finalizing the manuscript. Both authors contributed to the article and approved the submitted version.
Funding
This work was supported by the Ministry of Science and Technology, Taiwan (MOST108-2628-B-006-007).
Conflict of Interest
The authors declare that the research was conducted in the absence of any commercial or financial relationships that could be construed as a potential conflict of interest.
Acknowledgments
We thank the editor and the reviewers for their helpful comments on the manuscript.
Supplementary Material
The Supplementary Material for this article can be found online at: https://www.frontiersin.org/articles/10.3389/fevo.2020.602675/full#supplementary-material
References
Abrams, P. A. (2009). When does greater mortality increase population size? The long history and diverse mechanisms underlying the hydra effect. Ecol. Lett. 12, 462–474. doi: 10.1111/j.1461-0248.2009.01282.x
Allesina, S., and Tang, S. (2012). Stability criteria for complex ecosystems. Nature 483, 205–208. doi: 10.1038/nature10832
Bachelot, B., and Lee, C. T. (2020). Disturbances can promote and hinder coexistence of competitors in ongoing partner choice mutualisms. Am. Nat. 195, 445–462. doi: 10.1086/707258
Bachelot, B., Uriarte, M., Muscarella, R., Forero-Montaña, J., Thompson, J., McGuire, K., et al. (2018). Associations among arbuscular mycorrhizal fungi and seedlings are predicted to change with tree successional status. Ecology 99, 607–620. doi: 10.1002/ecy.2122
Bascompte, J., and Jordano, P. (2014). Mutualistic Networks. Princeton, NJ: Princeton University Press.
Baudoin, E., Benizri, E., and Guckert, A. (2002). Impact of growth stage on the bacterial community structure along maize roots, as determined by metabolic and genetic fingerprinting. Appl. Soil Ecol. 19, 135–145. doi: 10.1016/S0929-1393(01)00185-8
Botsford, L. W. (1981). The effects of increased individual growth rates on depressed population size. Am. Nat. 117, 38–63. doi: 10.1086/283685
Breton, L. M., and Addicott, J. F. (1992). Density-dependent mutualism in an aphid–ant interaction. Ecology 73, 2175–2180. doi: 10.2307/1941465
Bronstein, J. L., Huxman, T., Horvath, B., Farabee, M., and Davidowitz, G. (2009). Reproductive biology of Datura wrightii: the benefits of a herbivorous pollinator. Ann. Bot. 103, 1435–1443. doi: 10.1093/aob/mcp053
Calcagno, V., Mouquet, N., Jarne, P., and David, P. (2006). Coexistence in a metacommunity: the competition–colonization trade-off is not dead. Ecol. Lett. 9, 897–907. doi: 10.1111/j.1461-0248.2006.00930.x
Chamberlain, S. A., Didham, R. K., Bascompte, J., and Wardle, D. A. (2014). How context dependent are species interactions? Ecol. Lett. 17, 881–890. doi: 10.1111/j.1461-0248.2008.01250.x
Chaparro, J. M., Badri, D. V., and Vivanco, J. M. (2014). Rhizosphere microbiome assemblage is affected by plant development. ISME J. 8, 790–803. doi: 10.1038/ismej.2013.196
Claessen, D., De Roos, A. M., and Persson, L. (2004). Population dynamic theory of size–dependent cannibalism. Proc. R. Soc. Lond. B Biol. Sci. 271, 333–340. doi: 10.1098/rspb.2003.2555
de Roos, A. M. (2020a). Dynamic population stage-structure stabilizes complex ecological communities. bioRxiv [Preprint] doi: 10.1101/2020.06.27.174755
de Roos, A. M. (2020b). Effects of life history and individual development on community dynamics: a review of counterintuitive consequences. Ecol. Res. (in press). doi: 10.1111/1440-1703.12174
de Roos, A. M., and Persson, L. (2013). Population and Community Ecology of Ontogenetic Development. Princeton, NJ: Princeton University Press.
de Roos, A. M., Schellekens, T., Van Kooten, T., and Persson, L. (2008). Stage-specific predator species help each other to persist while competing for a single prey. Proc. Natl. Acad. Sci. U.S.A. 105, 13930–13935. doi: 10.1073/pnas.0803834105
Dejean, A., Djiéto-Lordon, C., Céréghino, R., and Leponce, M. (2008). Ontogenetic succession and the ant mosaic: an empirical approach using pioneer trees. Basic Appl. Ecol. 9, 316–323. doi: 10.1016/j.baae.2007.03.001
Diekmann, O., Gyllenberg, M., and Metz, J. A. J. (2003). Steady-state analysis of structured population models. Theor. Popul. Biol. 63, 309–338. doi: 10.1016/S0040-5809(02)00058-8
Fisher, M. E. (1987). An age-structured fish population model with coupled size and population density. Math. Biosci. 86, 15–34. doi: 10.1016/0025-5564(87)90061-7
Fonseca, C. R., and Benson, W. W. (2003). Ontogenetic succession in Amazonian ant trees. Oikos 102, 407–412. doi: 10.1034/j.1600-0579.2003.12021.x
Gaume, L., Zacharias, M., and Borges, R. M. (2005). Ant–plant conflicts and a novel case of castration parasitism in a myrmecophyte. Evol. Ecol. Res. 7, 435–452.
Guill, C. (2009). Alternative dynamical states in stage-structured consumer populations. Theor. Popul. Biol. 76, 168–178. doi: 10.1016/j.tpb.2009.06.002
Hahn, M., and Brühl, C. A. (2016). The secret pollinators: an overview of moth pollination with a focus on Europe and North America. Arthropod Plant Interact. 10, 21–28. doi: 10.1007/s11829-016-9414-3
Hardin, G. (1968). The tragedy of the commons. Science 162, 1243–1248. doi: 10.1126/science.162.3859.1243
Heil, M. (2015). Extrafloral nectar at the plant–insect interface: a spotlight on chemical ecology, phenotypic plasticity and food webs. Annu. Rev. Entomol. 60, 213–232. doi: 10.1146/annurev-ento-010814-020753
Heil, M., and McKey, D. (2003). Protective ant–plant interactions as model systems in ecological and evolutionary research. Annu. Rev. Ecol. Evol. Syst. 34, 425–453. doi: 10.1146/annurev.ecolsys.34.011802.132410
Hoeksema, J. D., and Bruna, E. M. (2015). “Context-dependent outcomes of mutualistic interactions,” in Mutualisms, ed. J. Bronstein (Oxford: Oxford University Press), 181–202. doi: 10.1093/acprof:oso/9780199675654.003.0010
Holland, J. N., and DeAngelis, D. L. (2010). A consumer–resource approach to the density-dependent population dynamics of mutualism. Ecology 91, 1286–1295. doi: 10.1890/09-1163.1
Houlden, A., Timms-Wilson, T. M., Day, M. J., and Bailey, M. J. (2008). Influence of plant developmental stage on microbial community structure and activity in the rhizosphere of three field crops. FEMS Microbiol. Ecol. 65, 193–201. doi: 10.1111/j.1574-6941.2008.00535.x
Husband, R., Herre, E. A., Turner, S. L., Gallery, R., and Young, J. P. W. (2002). Molecular diversity of arbuscular mycorrhizal fungi and patterns of host association over time and space in a tropical forest. Mol. Ecol. 11, 2669–2678. doi: 10.1046/j.1365-294X.2002.01647.x
Izzo, T. J., and Vasconcelos, H. L. (2002). Cheating the cheater: domatia loss minimizes the effects of ant castration in an Amazonian ant-plant. Oecologia 133, 200–205. doi: 10.1007/s00442-002-1027-0
Karst, J., Marczak, L., Jones, M. D., and Turkington, R. (2008). The mutualism–parasitism continuum in ectomycorrhizas: a quantitative assessment using meta-analysis. Ecology 89, 1032–1042. doi: 10.1890/07-0823.1
Ke, P. J., and Nakazawa, T. (2018). Ontogenetic antagonism–mutualism coupling: perspectives on resilience of stage-structured communities. Oikos 127, 353–363. doi: 10.1111/oik.04702
Koch, E. B., Camarota, F., and Vasconcelos, H. L. (2016). Plant ontogeny as a conditionality factor in the protective effect of ants on a Neotropical tree. Biotropica 48, 198–205. doi: 10.1111/btp.12264
Levins, R., and Culver, D. (1971). Regional coexistence of species and competition between rare species. Proc. Natl. Acad. Sci. U.S.A. 6, 1246–1248. doi: 10.1073/pnas.68.6.1246
Lurgi, M., Montoya, D., and Montoya, J. M. (2016). The effects of space and diversity of interaction types on the stability of complex ecological networks. Theor. Ecol. 9, 3–13. doi: 10.1007/s12080-015-0264-x
Mandyam, K. G., and Jumpponen, A. (2015). Mutualism–parasitism paradigm synthesized from results of root-endophyte models. Front. Microbiol. 5:776. doi: 10.3389/fmicb.2014.00776
Melián, C. J., Bascompte, J., Jordano, P., and Krivan, V. (2009). Diversity in a complex ecological network with two interaction types. Oikos 118, 122–130. doi: 10.1111/j.1600-0706.2008.16751.x
Merrild, M. P., Ambus, P., Rosendahl, S., and Jakobsen, I. (2013). Common arbuscular mycorrhizal networks amplify competition for phosphorus between seedlings and established plants. New Phytol. 200, 229–240. doi: 10.1111/nph.12351
Micallef, S. A., Channer, S., Shiaris, M. P., and Colón-Carmona, A. (2009). Plant age and genotype impact the progression of bacterial community succession in the Arabidopsis rhizosphere. Plant Signal. Behav. 4, 777–780. doi: 10.4161/psb.4.8.9229
Miller, T. E. X., and Flynn, D. (2014). Plant size and reproductive state affect the quantity and quality of rewards to animal mutualists. J. Ecol. 102, 496–507. doi: 10.1111/1365-2745.12210
Miller, T. E. X., and Rudolf, V. H. W. (2011). Thinking inside the box: community-level consequences of stage-structured populations. Trends Ecol. Evol. 26, 457–466. doi: 10.1016/j.tree.2011.05.005
Mougel, C., Offre, P., Ranjard, L., Corberand, T., Gamalero, E., Robin, C., et al. (2006). Dynamic of the genetic structure of bacterial and fungal communities at different developmental stages of Medicago truncatula Gaertn. cv. Jemalong line J5. New Phytol. 170, 165–175. doi: 10.1111/j.1469-8137.2006.01650.x
Mougi, A. (2017). Persistence of a stage-structured food-web. Sci. Rep. 7:11055. doi: 10.1038/s41598-017-11686-z
Mougi, A., and Kondoh, M. (2012). Diversity of interaction types and ecological community stability. Science 337, 349–351. doi: 10.1126/science.1220529
Nakazawa, T. (2011a). Alternative stable states generated by ontogenetic niche shift in the presence of multiple resource use. PLoS One 6:e14667. doi: 10.1371/journal.pone.0014667
Nakazawa, T. (2011b). Ontogenetic niche shift, food-web coupling, and alternative stable states. Theor. Ecol. 4, 479–494. doi: 10.1007/s12080-010-0090-0
Nakazawa, T. (2015). Ontogenetic niche shifts matter in community ecology: a review and future perspectives. Popul. Ecol. 57, 347–354. doi: 10.1007/s10144-014-0448-z
Nakazawa, T. (2017). Individual interaction data are required in community ecology: a conceptual review of the predator–prey mass ratio and more. Ecol. Res. 32, 5–12. doi: 10.1007/s11284-016-1408-1
Nakazawa, T. (2020). A perspective on stage-structured mutualism and its community consequences. Oikos 129, 297–310. doi: 10.1111/oik.06653
Nowak, M. A., Tarnita, C. E., and Antal, T. (2010). Evolutionary dynamics in structured populations. Philos. Trans. R. Soc. Lond. B Biol. Sci. 365, 19–30. doi: 10.1098/rstb.2009.0215
Okuyama, T., and Holland, J. N. (2008). Network structural properties mediate the stability of mutualistic communities. Ecol. Lett. 11, 208–216. doi: 10.1111/j.1461-0248.2007.01137.x
Oono, R., Lefèvre, E., Simha, A., and Lutzoni, F. (2015). A comparison of the community diversity of foliar fungal endophytes between seedling and adult loblolly pines (Pinus taeda). Fungal Biol. 119, 917–928. doi: 10.1016/j.funbio.2015.07.003
Palmer, T. M., Doak, D. F., Stanton, M. L., Bronstein, J. L., Kiers, E. T., Young, T. P., et al. (2010). Synergy of multiple partners, including freeloaders, increases host fitness in a multispecies mutualism. Proc. Natl. Acad. Sci. U.S.A. 107, 17234–17239. doi: 10.1073/pnas.1006872107
Rader, A. R., Bartomeus, I., Garibaldi, L. A., Garratt, M. P., Howlett, B. G., Winfree, R., et al. (2016). Non-bee insects are important contributors to global crop pollination. Proc. Natl. Acad. Sci. U.S.A. 113, 146–151. doi: 10.1073/pnas.1517092112
Roca, C. P., Cuesta, J. A., and Sánchez, A. (2009). Evolutionary game theory: temporal and spatial effects beyond replicator dynamics. Phys. Life Rev. 6, 208–249. doi: 10.1016/j.plrev.2009.08.001
Sauve, A. M. C., Fontaine, C., and Thébault, E. (2014). Structure-stability relationships in networks combining mutualistic and antagonistic interactions. Oikos 123, 378–384. doi: 10.1111/j.1600-0706.2013.00743.x
Schreiber, S., and Rudolf, V. H. W. (2008). Crossing habitat boundaries: coupling dynamics of ecosystems through complex life cycles. Ecol. Lett. 11, 576–587. doi: 10.1111/j.1461-0248.2008.01171.x
Stadler, B., and Dixon, A. F. G. (2005). Ecology and evolution of aphid–ant interactions. Annu. Rev. Ecol. Evol. Syst. 36, 345–372. doi: 10.1146/annurev.ecolsys.36.091704.175531
Stanton, M. L., Palmer, T. M., Young, T. P., Evans, A., and Turner, M. L. (1999). Sterilization and canopy modification of a swollen thorn acacia tree by a plant-ant. Nature 401, 578–581. doi: 10.1038/44119
Sugiyama, A., Ueda, Y., Zushi, T., Takase, H., and Yazaki, K. (2014). Changes in the bacterial community of soybean rhizospheres during growth in the field. PLoS ONE 9:e100709. doi: 10.1371/journal.pone.0100709
Suweis, S., Grilli, J., and Maritan, A. (2014). Disentangling the effect of hybrid interactions and of the constant effort hypothesis on ecological community stability. Oikos 123, 525–532. doi: 10.1111/j.1600-0706.2013.00822.x
Valdovinos, F. S., Moisset, de Espanés, P., Flores, J. D., and Ramos-Jiliberto, R. (2013). Adaptive foraging allows the maintenance of biodiversity of pollination networks. Oikos 122, 907–917. doi: 10.1111/j.1600-0706.2012.20830.x
van den Bosch, F., de Roos, A. M., and Gabriel, W. (1988). Cannibalism as a life boat mechanism. J. Math. Biol. 26, 619–633. doi: 10.1007/BF00276144
Wäckers, F. L., Romeis, J., and van Rijn, P. (2007). Nectar and pollen feeding by insect herbivores and implications for multitrophic interactions. Annu. Rev. Entomol. 52, 301–323. doi: 10.1146/annurev.ento.52.110405.091352
Werner, E. E., and Gilliam, J. F. (1984). The ontogenetic niche and species interactions in size-structured populations. Annu. Rev. Ecol. Syst. 15, 393–425. doi: 10.1146/annurev.es.15.110184.002141
White, J. W., Rassweiler, A., Samhouri, J. F., Stier, A. C., and White, C. (2014). Ecologists should not use statistical significance tests to interpret simulation model results. Oikos 123, 385–388. doi: 10.1111/j.1600-0706.2013.01073.x
Wilbur, H. M. (1980). Complex life cycles. Annu. Rev. Ecol. Syst. 11, 67–93. doi: 10.1146/annurev.es.11.110180.000435
Yao, I. (2014). Costs and constraints in aphid–ant mutualism. Ecol. Res. 29, 383–391. doi: 10.1007/s11284-014-1151-4
Keywords: context dependent mutualism, hybrid community dynamics, intraspecific variation, life history, mutualism-parasitism continuum, ontogenetic asymmetry, ontogenetic niche shift, stage-structured mutualism
Citation: Nakazawa T and Katayama N (2020) Stage-Specific Parasitism by a Mutualistic Partner Can Increase the Host Abundance. Front. Ecol. Evol. 8:602675. doi: 10.3389/fevo.2020.602675
Received: 04 September 2020; Accepted: 26 October 2020;
Published: 12 November 2020.
Edited by:
Pavel Kindlmann, Charles University, CzechiaReviewed by:
Donald Lee DeAngelis, United States Geological Survey (USGS), United StatesBenedicte Bachelot, Oklahoma State University, United States
Copyright © 2020 Nakazawa and Katayama. This is an open-access article distributed under the terms of the Creative Commons Attribution License (CC BY). The use, distribution or reproduction in other forums is permitted, provided the original author(s) and the copyright owner(s) are credited and that the original publication in this journal is cited, in accordance with accepted academic practice. No use, distribution or reproduction is permitted which does not comply with these terms.
*Correspondence: Takefumi Nakazawa, dGFrZS5ua3p3QGdtYWlsLmNvbQ==