- 1Department of Earth and Environmental Science, University of Pavia, Pavia, Italy
- 2Department of Sciences, University of Roma Tre, Rome, Italy
- 3Department of Life Sciences and Biotechnology, University of Ferrara, Ferrara, Italy
- 4Department of Chemistry, Life Sciences and Environmental Sustainability, University of Parma, Parma, Italy
- 5Faculty of Science and Technology, Free University of Bozen-Bolzano, Bolzano, Italy
Mountains and their biota are highly threatened by climate change. An important strategy that alpine plants use to escape this change consists in seed dispersal and the ability of seeds to germinate and establish in new sites at higher elevation. Little is known about the environmental factors that can affect the regeneration of plants above the elevational limit of growth. We present the first field evidence of recruitment success and plant performance in consequence of upward shift from the alpine to the nival life zone. Seeds of four alpine grassland species were sown at the current elevational limit of growth (site A) and 200 m upward, in a nival environment (site N) located in the Italian Alps. At site N part of the seeds were subjected to experimental manipulation of temperature (using an Open Top Chamber, OTC) or soil (using soil from site A). Recruitment success, soil surface temperature and water potential were monitored for five consecutive years. At the end of the experiment, vegetative growth and foliar traits were measured on individuals from all treatments. Mean annual soil surface temperature and length of the growing season at site A were ca. 2°C higher and ca. 44 days longer than at site N. Seedling emergence and seedling establishment generally were higher at N (with or without OTC) on local soil than at site A or at site N with soil originating from site A. Conversely, production was higher at site A and at site N with soil originating from site A. Recruitment success above the elevational leading edge was enhanced by coarser and nutrient-poor soil, which promoted seedling emergence and establishment but constrained plant growth. This trade-off between seedling recruitment and plant production underlines adaptive consequence and environmental filtering, both critical to forecast community assembly and responses of alpine species to climate warming.
Introduction
Mountains and their biota are highly threatened by climate change and are experiencing among the highest rates of warming (Pepin et al., 2015). Assessments of the impacts of climate change on global mountain systems foresee temperature warming between 1.8 and 4°C over the European Alps for the period 2051–2080 (Zimmermann et al., 2013). Such temperature increase is associated with a decrease in depth and duration of snow cover in mountain habitats (Marty, 2013; Frei et al., 2018), leading to longer growing seasons and higher frequency of frost events in winter (Gerdol et al., 2013). In response to these changes there are documented upward shifts of tree species within forests (Lenoir et al., 2008), tree-line ecotones (Du et al., 2018) and alpine species above tree line (Parolo and Rossi, 2008; Pauli et al., 2012), which results in plant community thermophilisation and changes in species richness (Gottfried et al., 2012). Recent observations revealed that this trend has accelerated, especially during the last 20–30 years (Steinbauer et al., 2018).
An important strategy that plants use to escape unsuitable environmental conditions driven by climate warming consists of seed dispersal and the ability of seeds to germinate and establish in new sites at higher elevations (Parolo and Rossi, 2008; Vittoz et al., 2009). Regeneration from seeds is also crucial for species persistence, because inherent genetic diversity and phenotypic plasticity of seeds may help species to adapt to future climate conditions (Walck et al., 2011; Bernareggi et al., 2016). Consequently, an increasing number of studies have addressed the effects of warming on seed germination and seedling establishment (see Briceño et al., 2015 for review). However, little is known about the regeneration capacity of plant species above the elevational leading edge of their distribution range, where new generations of species are expected to move.
The steep environmental gradients occurring in alpine habitats provide an ideal context for evaluating shifts of the species ecological niche (sensu Hutchinson, 1957) or just part of it, i.e., the recruitment niche (sensu Grubb, 1977; Young et al., 2005). Glacier foreland habitats are of additional interest because they represent highly attractive sites for studying primary dynamic succession (Matthews, 1992), thereby for understanding how the abiotic environment prevents establishment or persistence of plant species in the absence of (or at least in the presence of low) biotic interactions (Kraft et al., 2015). Glacier forelands experienced a substantial increase in species richness during the twentieth century (Holzinger et al., 2008; Fickert and Grüninger, 2018). Pioneer species inhabiting these habitats show high germination potential (Schwienbacher et al., 2012; Mondoni et al., 2015) and ability to grow relatively rapidly on nitrogen (N)—poor soils because of their inherently high nutrient uptake rates and photosynthetic efficiency (Chapin, 1993; Vittoz et al., 2009). Temperature warming per sé cannot represent the main driver of the upwards shift of species distribution because multiple environmental changes are needed to elicit vegetation dynamics in pioneer soils (Matthews, 1992). For example, seedlings are highly sensitive to desiccation (Leck et al., 2008) so that soil moisture may represent an important factor limiting seedling establishment, especially in glacier forelands where the coarse grain of the soil particles and the low humus content result in low water holding capacity of the soil (Körner, 2003). Furthermore, successful establishment of seedlings depends on availability of nutrients, mostly N and phosphorus (P), that limit plant growth in many alpine and arctic ecosystems (Van Wijk et al., 2004; Lett and Dorrepaal, 2018). Recruitment mechanisms in alpine plants are well known (e.g., Chapin et al., 1994; Jumpponen et al., 1999; Erschbamer et al., 2001). However, there is limited understanding of the environmental filters constraining species upward migration. Furthermore, little is known on how critical life stages, especially seedling recruitment and subsequent adult plant growth, respond to changing environment. Predicting the effects of global warming requires an understanding of how species are affected by the environment, which is most easily understood by investigating variation along gradients (McGill et al., 2006).
We present the first field evidence of seedling recruitment and plant performance in response to upward shift from the alpine life zone to the glacier foreland life zone, henceforth referred to as “nival zone” because of similarities among vegetation types (see paragraph “Study Area” for details). Seeds of four alpine grassland species were sown at the current elevational limit of growth and 200 m upward. At the high-elevation site part of the seeds were subjected to experimental manipulation of temperature or soil features. We hypothesized that alpine grassland species germinate less and are unable to establish in nival environment due to low temperature, dry and nutrient-poor soil, all constraining plant growth. We further hypothesized that weakly increased temperature and/or soil maturity enhance both seedling emergence and establishment of alpine species in nival environments.
Materials and Methods
Study Area
The study area (Figure 1) is located in the eastern Alps of Italy (Sondrio Province, Lombardy). The low-elevation site is located in the alpine vegetation belt (henceforth referred to as site A), at ca. 2,300 m (46°24′25.4″N, 10°12′24.7″ E), on stable slopes colonized by Carex curvula grasslands and small patches of Empetrum hermaphroditum—Loiseleuria procumbens dwarf heathlands. The high-elevation site is located in the nival vegetation belt (sensu Körner, 2003; henceforth referred to as site N), at ca. 2,500 m (46°24′12.6″N, 10°12′46.4″E), on the Dosdè glacier moraine. At the N site, vegetation consists of highly fragmented pioneer scree communities typical of the nival life zone (Reisigl and Keller, 1990), with Saxifraga aizoides, S. oppositifolia, Cerastium pedunculatum, Oxyria digyna, and Geum reptans as the most frequent species.
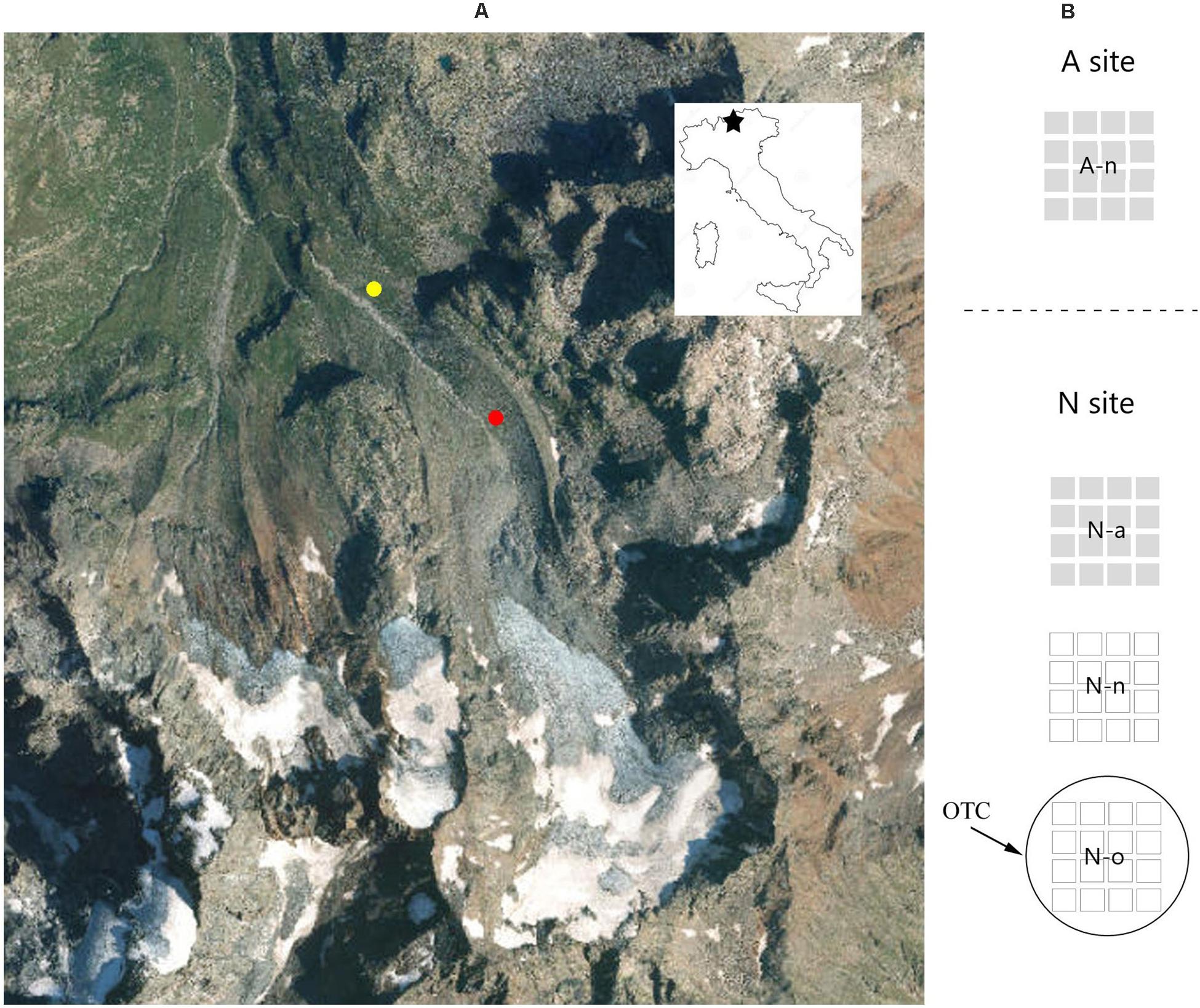
Figure 1. Study area and experimental design: (A) study area showing the location of the two experimental sites at the alpine vegetation belts (site A, 2,300 m a.s.l., yellow) and at the nival vegetation belts (site N, 2,500 m a.s.l., red); (B) schematic illustration of the 20 pots arranged at the A site on its natural soil (A-n) and the 60 pots at the N site, with one group on its natural soil (N-n), another with soil from A (N-a) and another heated by an OTC (N-o).
Climatic features were derived from Worldclim datasets (Hijmans et al., 2005) at a spatial resolution of 30 arc s (i.e., 1 km), using the coordinates of the species growing site (site A). This area has mean annual rainfall of ca. 1,082 mm, mean annual temperature of 0.61°C, with average minimum of −6.3°C in the coldest months (January-February) and average maximum of 8.45°C in the warmest month (July) at 2,300 m. Based on climatic data measured at the closest weather station (Livigno La Vallaccia, 46°28′40.9″N 10°12′22.0″E; 2,660 m above the sea level, ca. 7 km apart), during the last 18 years there was a weak but not significant increase in annual and summer temperature of ca. 0.3 and 0.8°C, respectively (Supplementary Figure S1). Bedrock geology in the whole area consists of siliceous acidic rocks (mostly gneiss).
Study Species and Experimental Set-Up
Seeds of four species belonging to the Asteraceae family [Arnica montana L., Hieracium pilosella L., Homogyne alpina (L.) Cass., and Leontodon helveticus Mérat] were collected on 9 September 2013, at the time of natural dispersal (Hay and Smith, 2003). Seeds were collected at site A, lying at approximately the local leading edge of the elevational distribution of the four species. These species were selected based on two criteria. First, they are overall abundant in the alpine vegetation belt. Second, they may represent potentially good colonizers of higher-elevation sites because of diaspore traits facilitating long-distance dispersal (Parolo and Rossi, 2008; Vittoz et al., 2009).
Immediately after collection, the seeds were exposed to environmental conditions occurring at both sites (A and N). At each site, experiments involved sowing four replicates of 100 seeds of the four species, each on a 10 × 10 cm square pot filled with natural soil at both sites, henceforth referred to as A-n (at site A) and N-n (at site N), respectively; soil was sieved removing any visible plant material from resident vegetation. The pots were adapted making large openings on the sides and removing the bottom to allow water circulation in the root zone. To investigate the effect of soil and temperature on recruitment success, the same experimental design was repeated two more times at site N, either using soil originating from site A (henceforth referred to as N-a), or an Open Top Chamber (OTC; Marion et al., 1997) placed on the pots (henceforth referred to as N-o). The OTC followed the cone-design proposed by the International Tundra Experiment (ITEX), that is known to increase the mean daily air temperature by ca. 1.5°C, which approximately reflects the temperature difference between sites A and N (by assuming a vertical temperature lapse of ca. 0.6°C per 100 m elevation; Körner, 2003). Every year, the OTC was placed in the field immediately after snowmelt and removed at the end of the growing season. In each treatment, four more pots were left empty to serve as controls, so that a total of 80 pots were established, with 20 pots (4 species × 4 replicates × 1 treatment, plus 4 controls) at site A (i.e., treatment A-n) and 60 pots (4 species × 4 replicates × 3 treatments, plus 12 controls) at site N (i.e., treatments N-n, N-a, N-o).
Data loggers, recording soil temperature (Tiny Tag, Gemini, Chichester, United Kingdom) and soil water potential (SWP) at hourly intervals (MicroLog SP3, Environmental Measuring Systems, Brno, Czech Republic), were buried at a depth of 2 cm in each treatment (i.e., one data logger for measuring soil T and SWP in A-n, N-n, N-a, and N-o).
Periodic Measurements, Final Sampling, and Analyses
Prior to the start of the experiment, samples of soils were taken and subjected to granulometric analyses. The soils were sieved using five different meshes (10, 6, 3, 1, and 0.125 mm) and weighed. A 20 mg subsample of fine soil material (<0.125 mm) was used for analyzing total carbon (C) by a Shimadzu TOC-5000A (Shimadzu Corporation; Kyoto, Japan), connected with a solid sample module (Shimadzu SSM- 5000A). A 100 mg subsample of fine soil material was extracted in 3 ml of selenous H2SO4 at 420°C and analyzed for total N concentration by the salicylate method and total P concentration by the molybdenum blue method using a continuous flow autoanalyzer (FlowSys; Systea, Anagni, Italy). The whole soil material was used for setting up the square pots.
The experiment lasted 5 years (from September 2013 to July 2018). Seedling emergence (number of emerged seedlings/number of seeds sown) and seedling establishment (number of survived seedlings/number of seeds sown) were monitored at ca. 15-day intervals during the snow-free period for 2 years (from September 2013 to September 2015) and then at monthly intervals for the other 3 years (from September 2015 to July 2018).
At the end of the experiment (24 July 2018) all pots were harvested and the plants used for determining above ground production. The plant material was also used for measuring chlorophyll fluorescence (Fv/Fm), chlorophyll content, specific leaf area (SLA), leaf dry matter content (LDMC), foliar N and P concentrations and foliar N/P ratio, i.e., stomatal density (SD), stomatal length (SL) and C isotope discrimination (δ13C). Fv/Fm, chlorophyll content, SLA, LDMC, foliar N and P concentrations and foliar N/P ratio are functional traits controlling major plant functions, especially photosynthetic capacity (Pérez-Harguindeguy et al., 2013). On the other hand, SD and SL are related to drought stress (Sun et al., 2014) while δ13C can be regarded as a proxy of water use efficiency (Cernusak et al., 2013). All of these variables eventually affect plant production and can hence be used as indicators of plant growth potential (Tonin et al., 2019). Below we provide details on the methods for each of these measurements.
Chlorophyll a fluorescence was determined on three mature healthy leaves by a portable fluorimeter (OS1-FL, Opti-Sciences, Hudson, NH, United States). A small leaf-clip adapter was placed on the central portion of each leaf for 1 min to achieve dark pre-adaptation before determining Fo. Fm was obtained after a saturating pulse of actinic light. The ratio Fv/Fm [where Fv = (Fm – Fo)] was used to assess the photochemical efficiency of photosystem II. Non-destructive assessment of chlorophyll content based on apparent leaf transmittance using leaf-clip-type sensors (CCM-200, Opti-Sciences, Hudson, NH, United States) was carried out on three other mature healthy leaves from different individuals. The mean values of chlorophyll fluorescence and chlorophyll content calculated across the three measurements were used as single values for each pot. Five to fifteen healthy leaves (depending on leaf size) taken from different individuals were used for determining SLA and LDMC. The leaves were fresh-weighed and scanned using the Scion Image version Alpha 4.0.3.2 (Scion Corporation, Frederick, MD, United States). Then, the scanned leaves were oven-dried at 40°C for 48 h and weighed for determining dry leaf mass and then calculating SLA. The ratio between dry mass and fresh mass was used for determining LDMC. The remainder of the aboveground plant material was oven-dried at 40°C for 48 h and subsequently weighed. The dry weight of this material plus that of the leaves used for determining chlorophyll fluorescence, chlorophyll content, SLA and LDMC represented aboveground production of a given species in a given pot. Three subsamples of dry leaves were used for further analyses.
- Ca. 50 mg of dry leaf tissue were powdered, extracted and analyzed for total N and total P concentrations as for the soils.
- Five to ten leaves (depending on leaf size) were used for stomatal measurements. As all four species are amphistomatic, stomatal measurements were carried out on both the abaxial and the adaxial sides of the leaves by the clear nail polish method (Hilu and Randall, 1984). All measurements were performed using a light microscope (Leica DMLS, Leica Biosystems, Nussloch, Germany), and an attached digital camera (DeltaPix, Måløv, Denmark), at 400× magnification. To estimate stomatal density (SD) the number of stomata was counted in five different fields of view for each epidermal impression. Stomatal length (SL) was measured using the software DeltaPix, v.3.2.x.
- Ca. 2 mg of dry leaf tissue were powdered and used for determining stable C isotope discrimination (δ13C) with an elemental analyzer (EA 1110, Carlo Erba, Milan, Italy) coupled online with an isotope ratio mass spectrometer (delta Plus XP, ThermoFinnigan, Bremen, Germany). Carbon isotope discrimination and expressed as in formula (1):
where V-PDB is the primary international standard Belemnitella americana from the Pee Dee Formation.
Statistical Analyses
Statistical analyses of the data were carried out with the objective to limit the effect of spatial autocorrelation of samples within sites. For this purpose, we ran partial regression analyses for all target variables on binary site explanatory variables and used the regression residuals for assessing effects of species and/or treatment on all target variables (Legendre, 1993).
The data on soil granulometry (i.e., the percentage of weight in the six particle size classes: 10, 6, 3, 1, 0.125 and < 0.125 mm) were analysed by a multivariate ANOVA with treatment as fixed factor. The data on soil chemistry, i.e., soil C concentration (soil C), soil N concentration (soil N), soil P concentration (soil P) and soil N/P ratio (soil N/P) were analysed each by a univariate ANOVA with the soil chemical variable as the response variable and treatment as the fixed factor. Significance of differences of the means among treatments were assessed using the Fishers’ LSD post hoc test.
The data on total seedling emergence and establishment were analysed each by a generalized linear mixed model (GLMM) with binomial error and logit-link function. In the two GLMMs, seedling emergence and seedling establishment were the response variables, while species, treatment and their interaction were the fixed effects; replicates were considered as random effects. In order to avoid negative values in the GLMMs, the partial regression residuals were normalized according to formula (2):
where Xni is the normalized value of the i-th regression residual for the X-variable (i.e., seedling emergence or seedling establishment, respectively); Xoi is the original value of the i-th regression residual for the X-variable; Xmin is the minimum value of all regression residuals for the X-variable and Xmax is the maximum value of all regression residuals for the X-variable.
The relationship between seedling emergence and establishment was analysed by linear regressions.
The data on production and foliar traits, i.e., chlorophyll fluorescence (Fv/Fm), chlorophyll content, SLA, LDMC, foliar N concentration (leaf N), foliar P concentration (leaf P), foliar N/P ratio (leaf N/P), SD, SL and δ13C were analysed each by a univariate factorial ANOVA with production or foliar traits as the response variable and species, treatment and their interaction as fixed factors. Significance of differences of the means among treatments were assessed by Fishers’ LSD post hoc test. Relationships between production and foliar traits were analysed by Pearson’s correlation coefficients.
Relationships among seedling emergence, seedling establishment, production, soil T, SWP, soil granulometry and soil chemistry were analysed by a multivariate statistical method (principal component analysis; PCA). The analysis was based on the correlation matrix among eight variables: percentage seedling emergence, percentage seedling establishment, production, mean soil T during the growing seasons 2014–2017, SWP during the growing seasons 2014–2017, percentage weight of coarse soil particles (i.e., 10 mm + 6 mm), soil N and soil P. The PCA provided centred standardized scores for the seven variables, after scaling to unit variance all variable vectors. Production was calculated as mean values of production of the four species in each of the four treatments. As production differed strongly among species because of intrinsic species-specific features, the data of production were normalized to maximum values prior to calculating the means. Normalisation was performed according to formula:
where Xni is the normalized value of production for the X-species at the i-pot; Xoi is the original value of production for the X-species at the i-pot; Xmax is the maximum value of production for the X-species across all pots.
The statistical analyses were performed using the packages SPSS 21 (IBM SPSS Statistics, Armonk, New York, United States) and STATISTICA 6 (StatSoft Inc., Tulsa, OK, United States).
Results
Environment: Climate and Soil
Mean annual soil temperature at site A (treatment A-n) was ca. 2°C higher than at site N (treatments N-n and N-a); the OTC (treatment N-o) increased the mean annual soil temperature by ca. 0.5°C at site N (Table 1). The growing season (considered as the snow-free period) was on average ca. 1.7°C warmer and ca. 44 day longer at A-n (approx. mid May–October) than at N-n and N-a (July–October), mostly because of earlier snowmelt in spring (Supplementary Table S1). In the absence of OTC, the plants at site N accumulated 642 degree-days less than at site A (Supplementary Table S1); during the growing season, the OTC strongly buffered the differences in soil temperature and slightly increased the accumulated heat at site N. The SWP was similar in the four treatments. The SWP always was high, i.e., barely below zero, and ranged from ca. −0.04 MPa during the growing season to ca. −0.30 MPa in winter. The mean annual SWP was ca. −0.20 MPa in all treatments (Table 1 and Supplementary Table S1).
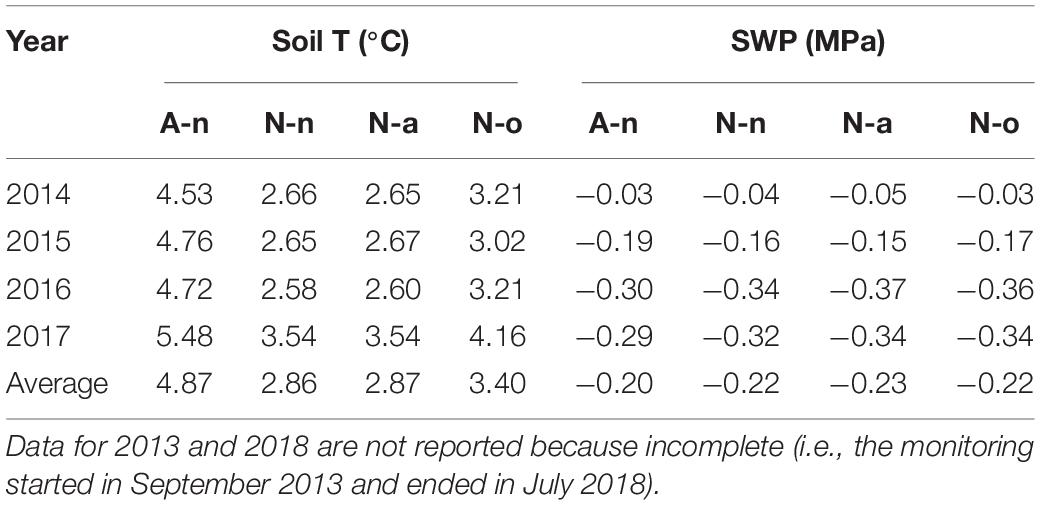
Table 1. Mean annual soil temperature and mean annual soil water potential (SWP) in the four years at the alpine vegetation belt (site A) on its natural soil (A-n) and at the nival vegetation belt (N site) on its natural soil (N-n), on soil originating from site A (N-a), and under the OTC (N-o).
Soil granulometry differed significantly among treatments [Wilks’Λ = 0.02, F(5, 15) = 4.06, P = 0.002]. The soils in A-n and N-a were poorer in coarse particles (size > 6 mm) compared to the soils in N-n and N-o (Table 2). The soils in A-n and N-a were richer in organic matter and hence presented higher C content [F(3, 11) = 85.40, P < 0.001] compared to the soils in N-n and N-o (Figure 2A). The soils in A-n and N-a also had higher N content [F(3, 11) = 38.04, P < 0.001; Figure 2B] whereas the soil P content [F(3, 11) = 0.53, P = 0.67] did not differ among treatments (Figure 2C). The soil N/P ratio mirrored the trend of soil C and soil N contents with significantly [F(3, 11) = 63.46, P < 0.001] higher values in A-n and N-a than in N-n and N-o (Figure 2D).
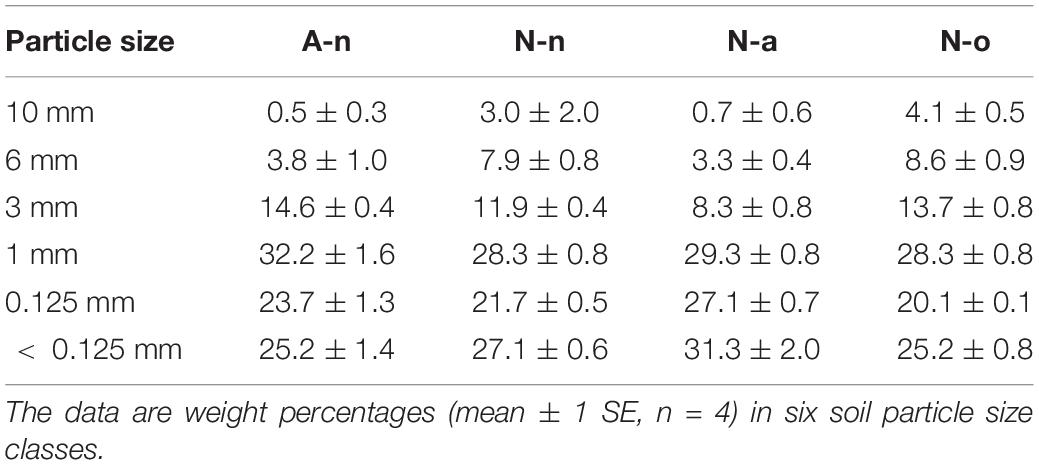
Table 2. Soil granulometry at the alpine vegetation belt (A site) on its natural soil (A-n) and at the nival vegetation belt (site N) on its natural soil (N-n), on soil originating from site A (N-a) and under the OTC (N-o).
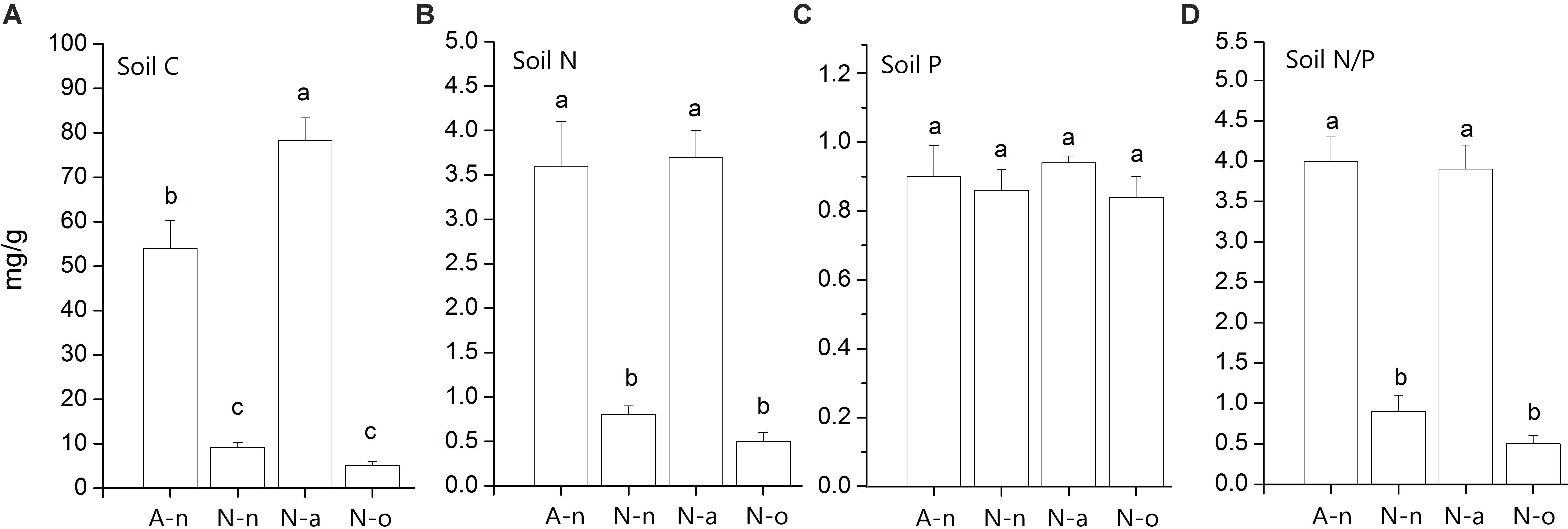
Figure 2. Mean (± 1 SE; n = 4) values of soil C content (A), soil N content (B), soil P content (C) and soil N/P ratio (D) in the four treatments. Within each panel different letters represent significant (P < 0.05) differences.
Seedling Recruitment
Seedling emergence was significantly different across species [F(3, 46) = 222.3; P < 0.001] and treatments [F(3, 46) = 126.0; P < 0.001] with significant species × treatment interaction [F(9, 46) = 29.6; P < 0.001]. The overall mean emergence was 56%, with the four species being ranked as follows: L. helveticus (76%) = H. alpina (71%) > A. montana (43%) = H. pilosella (35%). In general, seedling emergence was highest in N-n and lowest in N-a for all species except for H. alpina, that presented lowest germination in A-n, and for A. montana for which germination was similar across treatments except in N-a (Figures 3A–D). Seedling emergence occurred in the growing seasons 2014 and 2015 and no further germination was observed in the growing seasons 2016–2018 (Supplementary Figure S2). Germination mostly occurred during the first month after snowmelt, i.e., in June at site A and in July at site N. Afterwards, there was only minor emergence (but see A. montana and H. pilosella in N-n and N-o; Supplementary Figures S2A,C).
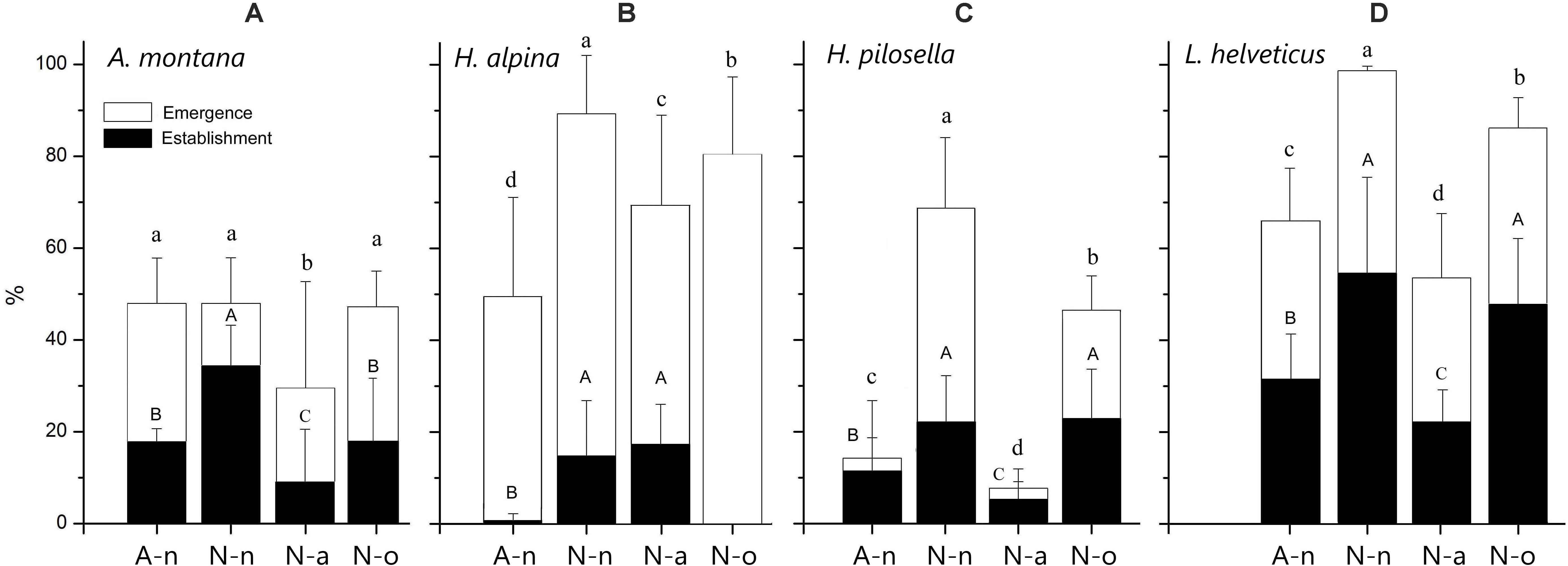
Figure 3. Final seedling emergence and establishment percentage (± 95% CI) for all species at the alpine vegetation belt (site A) on its natural soil (A-n) and at the nival vegetation belt (site N) on its natural soil (N-n), on soil originating from site A (N-a) and under the OTC (N-o). Within each panel different letters represent significant (P < 0.05) differences of emergence (lower-case) and establishment (upper-case).
Seedling establishment was significantly different across both species [F(3, 46) = 129.5; P < 0.001] and treatments [F(3, 46) = 56.8; P < 0.001], with strong species × treatment interaction [F(9, 46) = 18.9; P < 0.001; Figures 3A–D]. Seedling establishment was overall lower than seedling emergence (21%), with following ranking: L. helveticus (39%) > A. montana (19%) = H. pilosella (16%) > H. alpina (8%). Seedling establishment was highest in N-n and N-o for H. pilosella and L. helveticus (Figures 3C,D). Seedling establishment was strongly depressed in N-o for H. alpina and was highest only in N-n for A. montana (Figures 3A,B). Similar to seedling emergence, seedling establishment was lowest in N-a for A. montana, H. pilosella and A. helveticus (Figures 3A,C,D). In contrast, seedling establishment in A-n was extremely low for H. alpina (Figure 3B).
Production and Foliar Traits
Production differed significantly among species and treatments (Table 3). Production of A. montana, H. pilosella, and L. helveticus was highest in A-n, intermediate in N-a and lowest in both N-n and N-o (Figure 4A). Conversely, production of H. alpina was similar in A-n, N-a, and N-n and could not be recorded in N-o because no seedlings of H. alpina survived in this treatment (Figure 4A). Such different production patterns resulted in a significant species × treatment interaction (Table 3). Fv/Fm was unaffected by species and treatments (Table 3 and Figure 4B), whereas chlorophyll content did not differ among species but generally was highest in A-n and/or N-a (Table 3 and Figure 4C). All of the other foliar traits differed significantly across species, which reflected intrinsic differences among species (Tables 3, 4 and Figure 4). Most foliar traits were strongly affected by treatment, with the exceptions of leaf P, SL, and foliar δ13C (Tables 3, 4). The four species presented similar responses to the treatments in terms of SLA, LDMC, leaf N, and leaf N/P ratio, as shown by the lack of significant species × treatment interactions for these variables (Table 3). SLA generally was higher in A-n and N-a compared to N-n and N-o while the reverse was true for LDMC, although the differences were not always significant (Figures 4D,E). In A. montana, H. pilosella, and L. helveticus N concentrations always were ranked as follows A-n > N-a > N-n = N-o whereas the N/P ratio varied erratically across treatments. Few data on foliar nutrient concentrations were available for H. alpina because of scarcity of material for chemical analyses (Figures 4F–H). SD generally was higher at site N compared to site A but the response of SD to the treatments varied differently among species which resulted in a significant species × treatment interaction (Tables 3, 4). Finally, a total of 6 individuals of H. pilosella and 9 of L. helveticus reached sexual maturity by the end of the monitoring, flowering only at site A (data not shown).
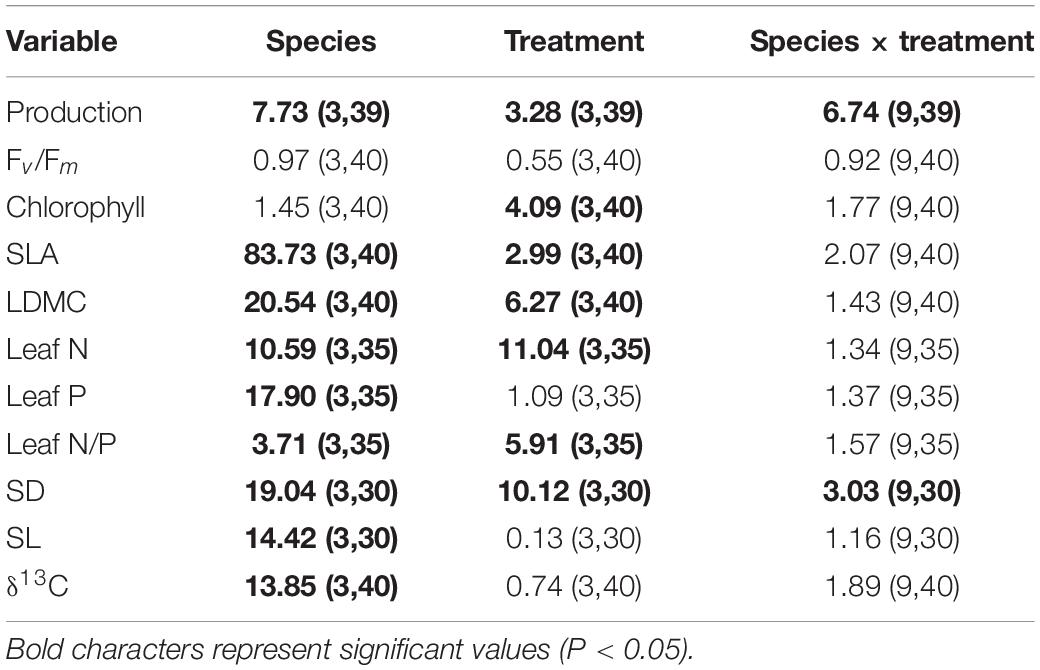
Table 3. Results from univariate factorial analyses of variance (ANOVAs; F-values and associated d.o.f. for numerator and denominator in parenthesis) used to assess the effects of species identity, treatment and their interaction on production and foliar traits.
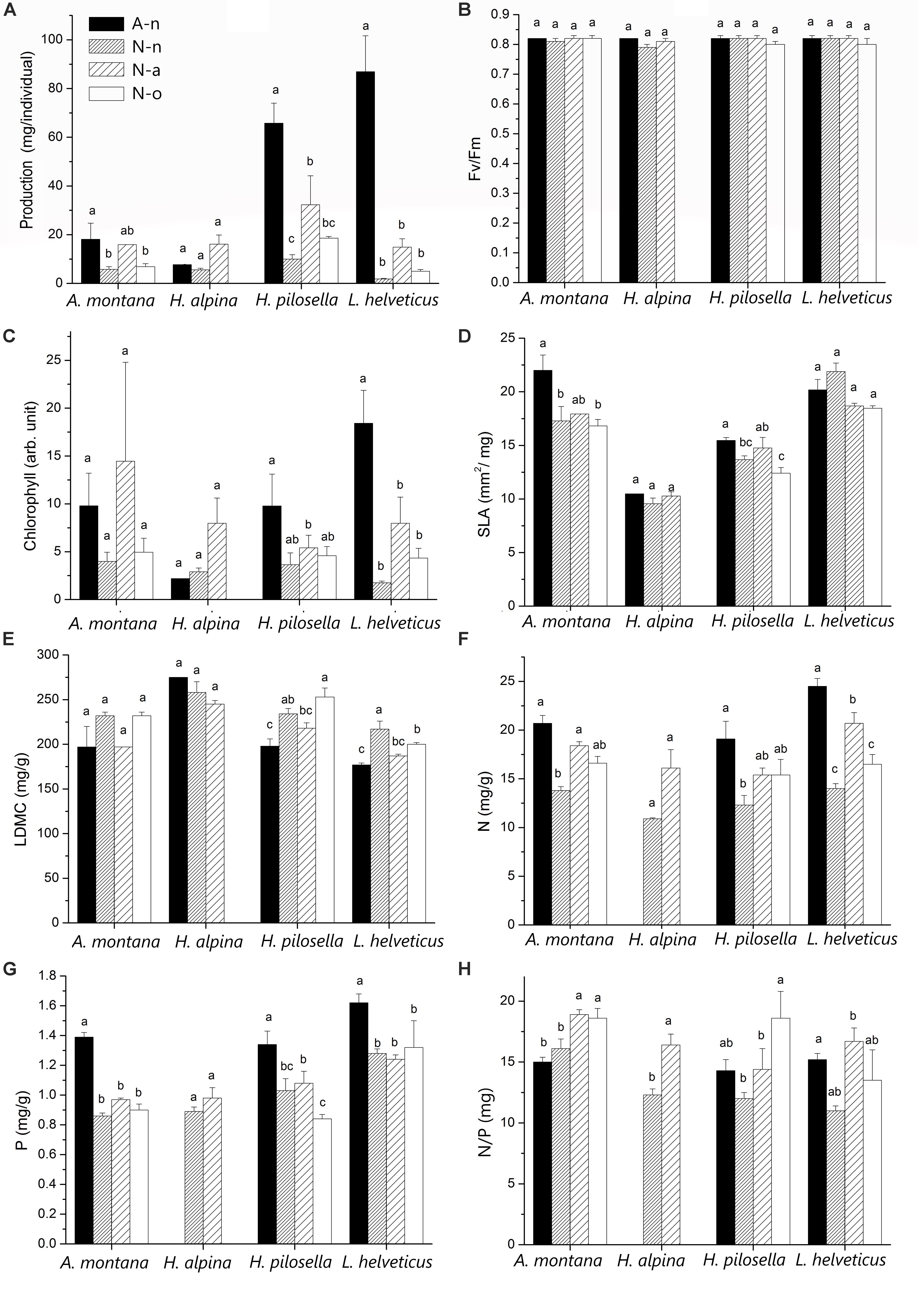
Figure 4. Mean (± 1 SE; n varies among species and treatments in relation to availability of leaf material) values of (A) production, (B) chlorophyll fluorescence (Fv/Fm), (C) chlorophyll content, (D) specific leaf area (SLA), (E) leaf dry matter content (LDMC), (F) foliar N concentration, (G) foliar P concentration and (H) foliar N/P ratio for each species at the alpine vegetation belt (site A) on its natural soil (A-n) and at the nival vegetation belt (site N) on its natural soil (N-n), on soil originating from site A (N-a) and under the OTC (N-o). Within each panel, different letters represent significant (P < 0.05) differences.
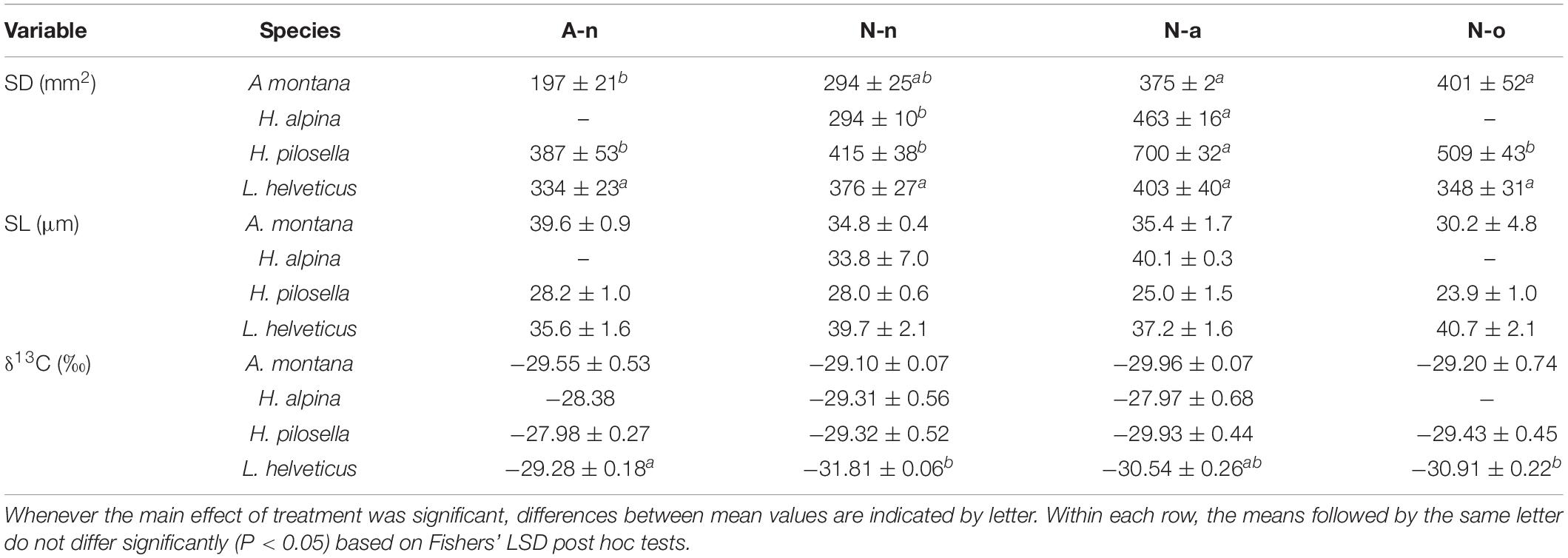
Table 4. Mean (± SE, n = 4) values of stomatal density (SD), stomatal length (SL) and foliar stable carbon isotope discrimination (δ13C) for the four species at the alpine vegetation belt (site A) on its natural soil (A-n) and at the nival vegetation belt (site N) on its natural soil (N-n), on soil originating from site A (N-a), and under the OTC (N-o).
Relationships Among Seedling Recruitment, Production, Foliar Traits, and Environmental Factors
Across all species and treatments, seedling establishment was positively correlated with seedling emergence [F(1, 60) = 15.567; P < 0.001]. Production was strongly positively correlated with chlorophyll content and leaf N (Table 5) and presented slightly lower positive correlations with leaf P and δ13C, and negative correlation with LDMC (Table 5). The results of PCA revealed a negative association between seedling recruitment and production as the seedling emergence and seedling establishment vectors were both located in the right-hand side of the diagram whereas the production vector had opposite orientation (Figure 5). The PCA diagram also revealed a close association between plant production and soil nutrient contents, i.e., soil N and to a lesser extent soil P. On the other hand, seedling emergence and seedling establishment were related to soil granulometry, i.e., the percentage of coarse size particles in the soil (Figure 5). Soil T and SWP were unrelated to both seedling recruitment and production because the vectors of soil T and SWP were oriented orthogonally to the vectors of seedling emergence, seedling establishment, and production (Figure 5).

Table 5. Pearson’s correlation coefficients between production and foliar traits calculated across all species in the four treatments (n = 42).
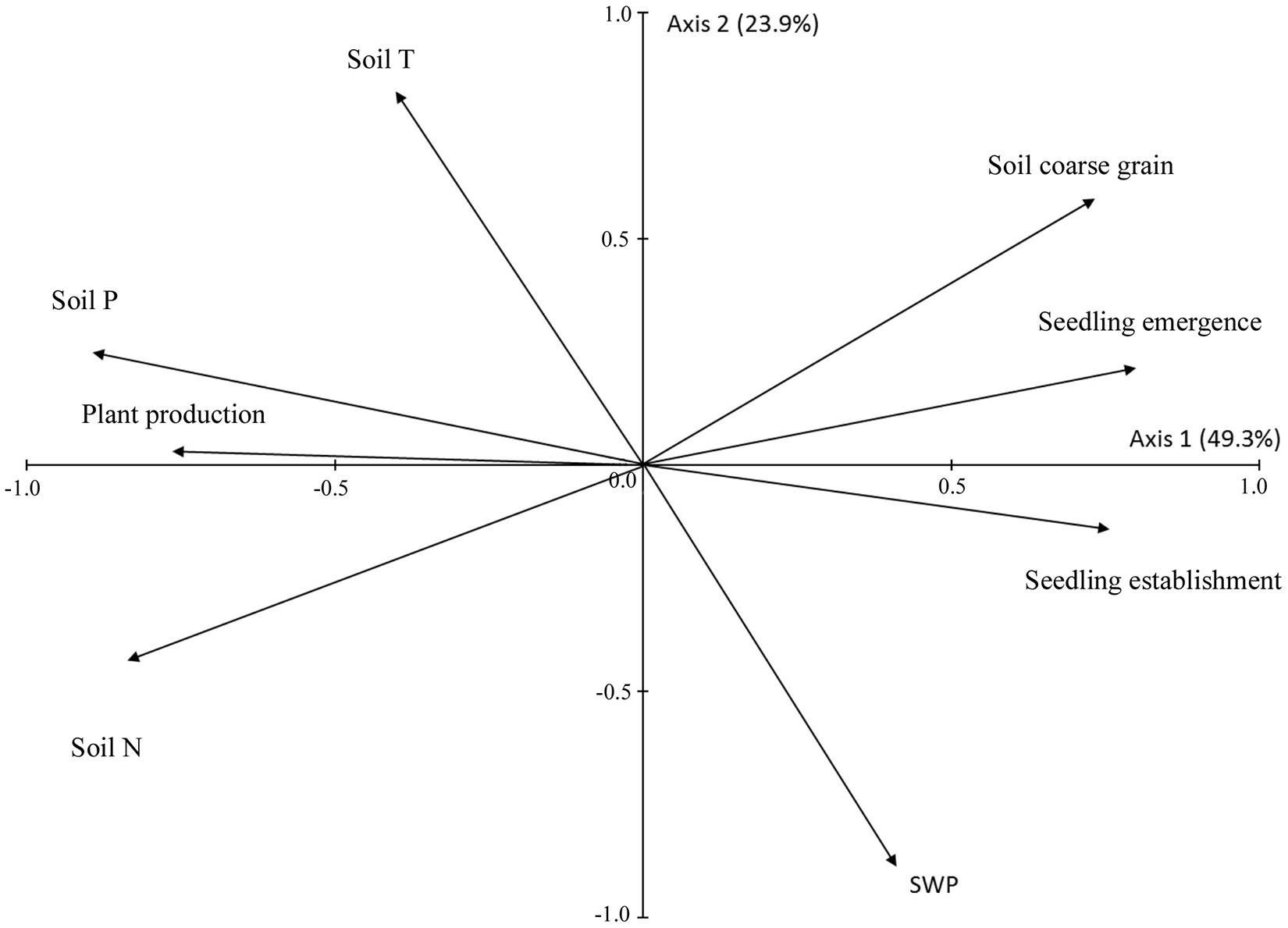
Figure 5. Scores of seedling emergence, seedling establishment, plant production, soil chemistry variables and soil coarse grains (>6 mm) on the first two principal component (PCA) axes. Plant production is the mean production of all four species based on normalized values. The percentage of variance accounted for by each axis is in parenthesis.
Discussion
In this study, we investigated seedling recruitment and plant production in response to upward shift from the alpine to the nival life zone. We hypothesized that alpine grassland species are unable to establish in nival environment due to low temperature, dry and nutrient-poor soil, but that weakly increased temperature and/or soil maturity can enhance both recruitment from seeds and production of alpine plants in nival environment.
Seedling Recruitment
Emergence and establishment of seedlings can be affected by both abiotic and biotic factors (see Larson and Funk, 2016 for review). In recently deglaciated terrains seedling emergence and seedling establishment are principally controlled by abiotic filters (Jumpponen et al., 1999), especially water availability (Matthews, 1992) and temperature (Marcante et al., 2014). However, in our study the observed differences in recruitment success were generally unrelated either to SWP or temperature. Conversely, both recruitment traits for most species except H. alpina strongly differed between treatments experiencing similar temperature but different soil conditions. Contrary to our main hypothesis, seedling emergence and establishment were overall higher at site N, especially on coarse and nutrient-poor soil. This means that seed germination and seedling establishment were not hampered in cold, dry or nutrient-poor soils. Coarse soils are thought to facilitate seedling recruitment via multiple effects, such as enhancing moisture retention around seeds (Jumpponen et al., 1999), reducing the risk of predation (Enright and Lamont, 1989) and increasing the likelihood of radicle penetration (Sheldon, 1974). Soil C content and soil nutrient availability had minor effects on recruitment (Fraaije et al., 2015). Accordingly, recruitment traits showed strong relationships with soil granulometry and were unrelated to soil nutrient content.
Weaker recruitment success at site A compared to site N may either suggest that seeds do not germinate well in the field even under optimal conditions or that climate could be already too warm to elicit germination processes at the species growing site, but that it could have become warm enough for such process at higher elevation. Accordingly, during the last 18 years there was a subtle (but not significant) increase of temperature at the study area (Supplementary Figure S1). As a plant population migrates up a mountain in response to climate warming, the conditions for emergence and establishment at the leading edge become more favourable as the conditions at the trailing edge become less favourable (Mondoni et al., 2012). This threat might be strong especially for truly alpine species (i.e., those occurring only above the treeline), compared with those occurring also at lower elevations, which may show greater plasticity and, therefore, higher adaptability to both biotic and environmental changes. Warming has been found to decrease the emergence of several alpine species (Graae et al., 2008; Hoyle et al., 2013). Several mechanisms can play a role with this respect. For example, under a shorter winter season, seeds may remain partially dormant (Dong et al., 2010). In addition, advanced snowmelt (Frei et al., 2018) enhances frost penetration in the soil thereby hampering thermal protection of seedlings (Schaberg et al., 2008). Indeed, snow melted about 2 months earlier at site A although winter temperatures were on average similar across treatments. Another explanation of weaker recruitment is that temperature at site A exceeded the optimum for germination in some species (Cochrane et al., 2011). Contrary to our second hypothesis, warmer temperature did not improve seedling performance but even resulted in lower seedling emergence for three out of the four species. Lower emergence in N-o compared to N-n for H. pilosella, L. helveticus, and H. alpina indicates that warmer temperature could be responsible for weaker recruitment in site A. Importantly, our results can be generalized to emergence and establishment on bare ground, because our experimental set-up excluded possible indirect effects from resident vegetation.
Production and Foliar Traits
Plant growth in mountainous regions can be limited by temperature (Li et al., 2008), water availability (He et al., 2014) or nutrient availability (Boczulak et al., 2014). Temperature did affect plant growth because production was overall higher at site A. However, if plant growth at site N was primarily limited by cold temperature this would imply higher production in N-o compared to both N-n and N-a. This was not the case for any of the four species. Therefore, temperature did not appear to represent the major factor limiting plant growth across the four treatments. Plant growth in high-elevation habitats of the Alps generally does not experience drought limitation because precipitation increases and evapotranspiration decreases across elevational gradients (Van der Schrier et al., 2007). Nonetheless, we believe that water availability could be reduced by the coarse grain of the soil in N-n and N-o. In spite of marked differences in soil granulometry, SWP did not differ among treatments so that water availability, similar to temperature, was not responsible for the observed variation in plant production. Conversely, soil nutrient content was closely and positively related to production (Figure 5).
Relationships between production and environmental factors were consistently associated with variation patterns of foliar traits among treatments. Plants at the N site presented lower SLA, which supports the findings of previous studies demonstrating that plants in cold habitats generally exhibit low SLA (Midolo et al., 2019). If plant production was constrained by cold temperature, positive relationships would be expected between production and SLA because SLA is positively related to relative growth rate (Poorter and Remkes, 1990). Conversely, our study revealed no correlation between production and SLA (Table 5). Drought stress generally is associated with reduced size and density of stomata as a stomatal control of gas exchange. Low stomatal conductance improves water-use efficiency but meanwhile reduces net CO2 exchange with consequent lowering of growth rates. If plant growth was constrained by water availability, production would be negatively correlated with SD, SL, and foliar δ13C. Indeed, drought stress generally is accompanied by low size and/or density of stomata, implying higher water-use efficiency and lower growth rates. The observed relationships of plant production with foliar traits did not point to water availability as a factor affecting plant growth because production was unrelated to both SD and SL and even exhibited positive correlation with foliar δ13C for reasons that will be discussed below.
The positive correlations between production and leaf nutrient (especially N) content suggested that plant production was primarily controlled by foliar nutrient status, which partly supported our main hypothesis. In particular, high leaf N was associated with higher production in A-n and N-a. High foliar N content improves photosynthetic capacity (Hikosaka, 2004) because a large fraction of leaf N is allocated to the photosynthetic machinery and this fraction is largely constant (Evans, 1989). Consistently, production exhibited positive correlation with chlorophyll content as well as with foliar δ13C because C isotope discrimination is lower, i.e., the foliar δ13C is less negative, when photosynthetic capacity is higher (Cernusak et al., 2013). Plants growing in more fertile habitats reduce resource investment in leaf structural elements (Hulshof et al., 2013), which is supported by negative correlation between production and LDMC. Higher leaf N content in A-n and N-a closely mirrored the soil N content expressed in terms of total soil N concentration. Production also showed positive correlation with leaf P content but higher leaf P content in A-n and N-a were not associated with consistent patterns of soil P content. We do not have a mechanistic explanation of this finding. However, previous studies showed that total soil P need not represent an index of P availability where most of the total soil P consists of recalcitrant organic or inorganic P forms (Gerdol et al., 2017). We speculate that soils richer in fine particle with higher C content enhanced microbial P mineralisation that is the main source of inorganic P supply to the plants in alpine soils (Gerdol et al., 2019). Whatever the mechanism accounting for soil P availability, adequate levels of P supply were important for preventing stoichiometric imbalance between N and P content in the leaves and consequent lowering of the growth rates (Gerdol et al., 2017). Consistently, production was unrelated to the leaf N/P ratio.
Trait Trade-Off and Niche Shift
A trait-based approach has been widely used to explain the distribution of species along environmental gradients and to predict shifts in species distributions (Laughlin, 2014). Selecting traits that can act as drivers of particular ecological processes is crucial to predict the performance of species in a changing environment (Funk et al., 2017). Various plant traits have been considered to explain the mechanisms governing succession in alpine environments. In particular, seed traits are considered as reliable indicators of colonisation potential (Schwienbacher et al., 2012) because strong colonizers of alpine summits possess wind-dispersed seeds (Holzinger et al., 2008) with high germination potential (Stöcklin and Bäumler, 1996). In this study seedling emergence and seedling establishment were positively correlated, underlining the importance of the earliest regenerative phases in determining species distributions (Grubb, 1977; Fraaije et al., 2015). However, species’ responses to environmental conditions are better estimated considering different traits simultaneously (Kleyer and Minden, 2015). Indeed, our results showed that neither vegetative nor recruitment traits alone are as powerful as their combination to explain the processes by which species upward shift take place.
Upward shift of about 200 m from the range limit of alpine species had an opposite response in recruitment traits compared to vegetative traits, leading to a significant increase of recruitment success and a decrease of growth, respectively. This trade-off between recruitment traits and vegetative traits highlights that separate plant life stages differ in their ecological niche requirements. In particular, environmental conditions that allow a seed to germinate and establish (recruitment niche) differ from those needed for subsequent growth (adult niche). This is an example of shifts of the ontogenetic niche, i.e., the niche occupied by an organism during a specific life stage. Ontogenetic niche shifts have been often explored for animals but rarely for plants (Valdez et al., 2019 and references therein; but see Vitasse, 2013), for which it is assumed that life stages respond similarly to the environment. The ontogenetic niche shifts observed here indicate that alpine species have far greater potential to establish beyond rather than within their expressed niche, i.e., the niche where they are actually found, that is further limited by intra- and inter-specific competition not considered in this study. This may have important implications for community assembly and response to climate warming as such ontogenetic niche shifts enhance colonisation ability. With this respect, our results also show that alpine species did not reach the sexual maturity at the nival environment, indicating that their long-term persistence is not possible. Nevertheless, considering the exceptionally high rate of warming projected for alpine regions in this century (Hock et al., 2019), the possibility that alpine plants will establish in nival environments cannot be ruled out in a near future, at least for species with long-distance seed dispersal.
Conclusion
Our results show that adult niche represent a major bottleneck (filter) for alpine species to establish a viable population in nival environments. Hence, successional constraints in these habitats seem to be less related to colonisation abilities than to the ability to acquire (low) resources during subsequent growth. Furthermore, the trait trade-off highlighted here may differentiate ecological strategies for plant population maintenance and expansion. Seedling establishment was improved outside the adult niche, where there is low seed density and chance of growth and was constrained where environmental conditions support growth but there is higher risk of intraspecific competition.
Differential environmental filtering during regeneration and subsequent growth plays an important role in determining alpine plant population maintenance and expansion. In particular, soil granulometry and nutrients act as strong environmental filters improving recruitment in coarse, nutrient-poor soil and constraining it, but fostering growth on finer and nutrient-richer substrates. Thus, soil granulometry and nutrient form the major axes of an ecological framework for understanding species upward migration.
Data Availability Statement
The raw data supporting the conclusions of this article will be made available by the authors, without undue reservation.
Author Contributions
AM designed the study. AM, SO, TA, and GR carried out the periodic field work. AM, TA, GR, LB, and RG performed the final sampling. LB, AC, PI, RT, RM, and RG carried out the laboratory work. AM and RG analysed the data and wrote the first draft of the manuscript. All authors contributed to the final version of the manuscript.
Conflict of Interest
The authors declare that the research was conducted in the absence of any commercial or financial relationships that could be construed as a potential conflict of interest.
Acknowledgments
We thank Davide Sai, Paolo Cauzzi, Giulietta Bernareggi, and Silvano Lodetti for assistance during the field work and laboratory work, Caterina Bellosta for designing icons of the graphical abstract and Rifugio CAI Federico in Dosdè for logistic support. The Grant of Excellence Departments, MIUR-Italy (ARTICOLO 1, COMMI 314 – 337 LEGGE 232/2016) is gratefully acknowledged for the support to one of the authors (TA).
Supplementary Material
The Supplementary Material for this article can be found online at: https://www.frontiersin.org/articles/10.3389/fevo.2020.572878/full#supplementary-material
References
Bernareggi, G., Carbognani, M., Mondoni, A., and Petraglia, A. (2016). Seed dormancy and germination changes of snowbed species under climate warming: the role of pre- and post- dispersal temperatures. Ann. Bot. 118, 529–539. doi: 10.1093/aob/mcw125
Boczulak, S. A., Hawkins, B. J., and Roy, R. (2014). Temperature effects on nitrogen form uptake by seedling roots of three contrasting conifers. Tree Physiol. 34, 513–523. doi: 10.1093/treephys/tpu028
Briceño, V. F., Hoyle, G. L., and Nicotra, A. B. (2015). Seeds at risk: how will a changing alpine climate affect regeneration from seeds in alpine areas? Alpine Bot. 125, 59–68. doi: 10.1007/s00035-015-0155-1
Cernusak, L. A., Ubierna, N., Winter, K., Holtum, J. A. M., Marshall, J. D., and Farquhar, G. D. (2013). Environmental and physiological determinants of carbon isotope discrimination in terrestrial plants. New Phytol. 200, 950–965. doi: 10.1111/nph.12423
Chapin, F. S. III (1993). “Physiological controls over plant establishment in primary succession,” in Primary Succession on Land, eds J. Miles and D. W. H. Walton (Oxford: Blackwell Scientific Publications), 161–178.
Chapin, F. S. III, Walker, L. R., Fastie, C. L., and Sharman, L. C. (1994). Mechanisms of primary succession following deglaciation at Glacier Bay, Alaska. Ecol. Monographs 64, 149–175. doi: 10.2307/2937039
Cochrane, A., Daws, M. I., and Hay, F. R. (2011). Seed-based approach for identifying flora at risk from climate warming. Austral Ecol. 36, 923–935. doi: 10.1111/j.1442-9993.2010.02211.x
Dong, W., Jiang, Y., and Yang, S. (2010). Response of the starting dates and the lengths of seasons in Mainland China to global warming. Clim. Change 99, 81–91. doi: 10.1007/s10584-009-9669-0
Du, H., Liu, J., Li, M. H., Büntgen, U., Yang, Y., Wang, L., et al. (2018). Warming−induced upward migration of the alpine treeline in the Changbai Mountains, northeast China. Glob. Change Biol. 24, 1256–1266. doi: 10.1111/gcb.13963
Enright, N. J., and Lamont, B. B. (1989). Seed banks, fire season, safe sites and seedling recruitment in five co-occurring Banksia species. J. Ecol. 77, 1111–1122. doi: 10.2307/2260826
Erschbamer, B., Kneringer, E., and Niederfriniger Schlag, R. (2001). Seed rain, soil seed bank, seedling recruitment, and survival of seedlings on a glacier foreland in the Central Alps. Flora 196, 304–312. doi: 10.1016/S0367-2530(17)30059-2
Evans, J. R. (1989). Photosynthesis and nitrogen relationships in leaves of C3 plants. Oecologia 78, 9–19. doi: 10.1007/BF00377192
Fickert, T., and Grüninger, F. (2018). High−speed colonization of bare ground - Permanent plot studies on primary succession in recently deglaciated glacier forelands. Land Degrad. Dev. 29, 2668–2680. doi: 10.1002/ldr.3063
Fraaije, R. G. A., Ter Braak, C. J. F., Verduyn, B., Breeman, L. B. S., Verhoeven, J. T. A., and Soons, M. B. (2015). Early plant recruitment stages set the template for the development of vegetation patterns along a hydrological gradient. Funct. Ecol. 29, 971–980. doi: 10.1111/1365-2435.12441
Frei, P., Kotlarski, S., Liniger, M. A., and Schär, C. (2018). Future snowfall in the Alps: projections based on the EURO-CORDEX regional climate models. Cryosphere 12, 1–24. doi: 10.5194/tc-12-1-2018
Funk, J. L., Larson, J. E., Ames, G. M., Butterfield, B. J., Cavender-Bares, J., Firn, J., et al. (2017). Revisiting the Holy Grail: using plant functional traits to understand ecological processes. Biol. Rev. Camb. Philos. Soc. 92, 1156–1173. doi: 10.1111/brv.12275
Gerdol, R., Iacumin, P., and Brancaleoni, L. (2019). Differential effects of soil chemistry on the foliar resorption of nitrogen and phosphorus across altitudinal gradients. Funct. Ecol. 33, 1351–1361. doi: 10.1111/1365-2435.13327
Gerdol, R., Marchesini, R., and Iacumin, P. (2017). Bedrock geology interacts with altitude in affecting leaf growth and foliar nutrient status of mountain vascular plants. J. Plant Ecol. 10, 839–850. doi: 10.1093/jpe/rtw092
Gerdol, R., Siffi, C., Iacumin, P., Gualmini, M., and Tomaselli, M. (2013). Advanced snowmelt affects vegetative growth and sexual reproduction of Vaccinium myrtillus in a sub-alpine heath. J. Veg. Sci. 24, 569–579. doi: 10.1111/j.1654-1103.2012.01472.x
Gottfried, M., Pauli, H., Futschik, A., Akhalkatsi, M., Baranèok, P., Alonso, J. L. B., et al. (2012). Continent-wide response of mountain vegetation to climate change. Nat. Clim. Change 2, 111–115. doi: 10.1038/nclimate1329
Graae, B. J., Alsos, I. G., and Ejrnaes, R. (2008). The impact of temperature regimes on development, dormancy breaking and germination of dwarf shrub seeds from arctic, alpine and boreal sites. Plant Ecol. 198, 275–284. doi: 10.1007/s11258-008-9403-4
Grubb, P. J. (1977). The maintenance of species-richness in plant communities: the importance of the regeneration niche. Biol. Rev. 52, 107–145. doi: 10.1111/j.1469185X.1977.tb01347.x
Hay, F. R., and Smith, R. D. (2003). “Seed maturity: when to collect seeds from wild plants,” in Seed Conservation: Turning Science Into Practice, eds R. D. Smith, J. B. Dickie, S. H. Linnington, and H. W. Pritchard (London: Royal Botanic Gardens), 97–133.
He, Y., Kueffer, C., Shi, P., Zhang, X., Du, M., Yan, W., et al. (2014). Variation of biomass and morphology of the cushion plant Androsace tapete along an elevational gradient in the Tibetan Plateau. Plant Spec. Biol. 29, E64–E71. doi: 10.1111/1442-1984.12031
Hijmans, R. J., Cameron, S. E., Parra, J. L., Jone, P. G., and Jarvis, A. (2005). Very high resolution interpolated climate surfaces for global land areas. Int. J. Climatol. 25, 1965–1978. doi: 10.1002/joc.1276
Hikosaka, K. (2004). Interspecific difference in the photosynthesis-nitrogen relationship: patterns, physiological causes, and ecological importance. J. Plant Res. 117, 481–494. doi: 10.1007/s10265-004-0174-2
Hilu, K. W., and Randall, J. L. (1984). Convenient method for studying grass leaf epidermis. Taxon 33, 413–415. doi: 10.2307/1220980
Hock, R., Rasul, G., Adler, C., Cáceres, B., Gruber, S., Hirabayashi, Y., et al. (2019). “High mountain areas,” in IPCC Special Report on the Ocean and Cryosphere in a Changing Climate, eds H.-O. Pörtner, D. C. Roberts, V. Masson-Delmotte, P. Zhai, M. Tignor, E. Poloczanska, et al. (Geneva: The Intergovernmental Panel on Climate Change).
Holzinger, B., Hülber, K., Camenisch, M., and Grabherr, G. (2008). Changes in plant species richness over the last century in the eastern Swiss Alps: elevational gradient, bedrock effects and migration rates. Plant Ecol. 195, 179–196. doi: 10.1007/s11258-007-9314-9
Hoyle, G. L., Venn, S. E., Steadman, K. J., Good, R. B., McAuliffe, E. J., Williams, E. R., et al. (2013). Soil warming increases plant species richness but decreases germination from the alpine soil seed bank. Glob. Change Biol. 19, 1549–1561. doi: 10.1111/gcb.12135
Hulshof, C. M., Violle, C., Spasojevic, M. J., McGill, B., Damschen, E., Harrison, S., et al. (2013). Intra-specific and inter-specific variation in specific leaf area reveal the importance of abiotic and biotic drivers of species diversity across elevation and latitude. J. Veg. Sci. 24, 921–931. doi: 10.1111/jvs.12041
Hutchinson, G. (1957). Concluding remarks. Cold SH. Q. B. 22, 415–427. doi: 10.1101/SQB.1957.022.01.039
Jumpponen, A., Väre, H., Mattson, K. J., Ohtonen, R., and Trappe, J. M. (1999). Characterization of ‘safe sites’ for pioneers in primary succession on recently deglaciated terrain. J. Ecol. 87, 98–105. doi: 10.1046/j.1365-2745.1999.00328.x
Kleyer, M., and Minden, V. (2015). Why functional ecology should consider all plant organs: an allocation-based perspective. Basic Appl. Ecol. 16, 1–9. doi: 10.1016/j.baae.2014.11.002
Körner, C. (2003). Alpine Plant Life: Functional Plant Ecology of High Mountain Ecosystems, 2 Edn. Berlin: Springer.
Kraft, N. J. B., Adler, P. B., Godoy, O., James, E. C., Fuller, S., and Levine, J. M. (2015). Community assembly, coexistence and the environmental filtering metaphor. Funct. Ecol. 29, 592–599.
Larson, J. E., and Funk, J. L. (2016). Regeneration: an overlooked aspect of trait-based plant community assembly models. J. Ecol. 104, 1284–1298. doi: 10.1111/1365-2745.12613
Laughlin, D. C. (2014). The intrinsic dimensionality of plant traits and its relevance to community assembly. J. Ecol. 102, 186–193. doi: 10.1111/1365-2745.12187
Leck, M. A., Parker, V. T., and Simpson, R. L. (2008). Seedling Ecology and Evolution. Cambridge: Cambridge University Press.
Legendre, P. (1993). Spatial autocorrelation: trouble or new paradigm? Ecology 74, 1659–1673. doi: 10.2307/1939924
Lenoir, J., Gégout, J. C., Marquet, P. A., de Ruffray, P., and Brisse, H. (2008). A significant upward shift in plant species optimum elevation during the 20th century. Science 320, 1768–1771. doi: 10.1126/science.1156831
Lett, S., and Dorrepaal, E. (2018). Global drivers of tree seedling establishment at alpine treelines in a changing climate. Funct. Ecol. 32, 1666–1680. doi: 10.1111/1365-2435.13137
Li, M. H., Xiao, W. F., Wang, S. G., Cheng, G. W., Cherubini, P., Cai, X. H., et al. (2008). Mobile carbohydrates in Himalayan treeline trees I. Evidence for carbon gain limitation but not for growth limitation. Tree Physiol. 28, 1287–1296. doi: 10.1093/treephys/28.8.1287
Marcante, S., Erschbamer, B., Buchner, O., and Neuner, G. (2014). Heat tolerance of early developmental stages of glacier foreland species in the growth chamber and in the field. Plant Ecol. 215, 747–758. doi: 10.1007/s11258-014-0361-8
Marion, G. M., Henry, G. H. R., Freckman, D. W., Johnstone, J., Jones, G., Jones, M. H., et al. (1997). Open-top design for manipulating field temperature in high-latitude ecosystems. Glob. Change Biol. 3, 20–32. doi: 10.1111/j.1365-2486.1997.gcb136.x
Marty, C. (2013). “Climate change and snow cover in the European Alps,” in The Impacts of Skiing and Related Winter Recreational Activities on Mountain Environments, eds C. Rixen, A. Rolando, and U. A. E. Sharjah (Sharjah: Bentham Science Publisher), 30–44.
Matthews, J. A. (1992). The Ecology of Recently Deglaciated Terrain. A Geoecological Approach to Glacier Forelands and Primary Succession. Cambridge: Cambridge University Press.
McGill, B. J., Enquist, B. J., Weiher, E., and Westoby, M. (2006). Rebuilding community ecology from functional traits. Trends Ecol. Evolut. 21, 178–185.
Midolo, G., De Frenne, P., Hölzel, N., and Wellstein, C. (2019). Global patterns of intraspecific leaf trait response to elevation. Glob. Change Biol. 25, 2485–2498. doi: 10.1111/gcb.14646
Mondoni, A., Pedrini, S., Bernareggi, G., Rossi, G., Abeli, T., Probert, R. J., et al. (2015). Climate warming could increase recruitment success in glacier foreland plants. Ann. Bot. 116, 907–916. doi: 10.1093/aob/mcv101
Mondoni, A., Rossi, G., Orsenigo, S., and Probert, R. J. (2012). Climate warming could shift the timing of seed germination in alpine plants. Ann. Bot. 110, 155–164. doi: 10.1093/aob/mcs097
Parolo, G., and Rossi, G. (2008). Upward migration of vascular plants following a climate warming trend in the Alps. Basic Appl. Ecol. 9, 100–107. doi: 10.1016/j.baae.2007.01.005
Pauli, H., Gottfried, M., Dullinger, S., Abdaladze, O., Akhalkatsi, M., Alonso, J. L. B., et al. (2012). Recent plant diversity changes on Europe’s mountain summits. Science 336, 353–355. doi: 10.1126/science.1219033
Pepin, N., Bradley, R. S., Diaz, H. F., Baraer, M., Cáceres, E. B., Forsythe, N., et al. (2015). Elevation−dependent warming in mountain regions of the world. Nat. Clim. Change 5, 424–430. doi: 10.1038/nclimate2563
Pérez-Harguindeguy, N., Díaz, S., Garnier, E., Lavorel, S., Poorte, H., Jaureguiberry, P., et al. (2013). New handbook for standardised measurement of plant functional traits worldwide. Aust. J. Bot. 61, 167–234. doi: 10.1071/BT12225
Poorter, H., and Remkes, C. (1990). Leaf area ratio and net assimilation rate of 24 wild species differing in relative growth rate. Oecologia 83, 553–559. doi: 10.1007/BF00317209
Reisigl, H., and Keller, R. (1990). Alpenpflanzen im Lebensraum: Alpine Rasen-, Schutt- und Felsvetation. Stuttgart, New York: Gustav Fisher Verlag.
Schaberg, P. G., Hennon, P. E., D’Amore, D. V., and Hawley, G. J. (2008). Influence of simulated snow cover on the cold tolerance and freezing injury of yellowcedar seedlings. Glob. Change Biol. 14, 1282–1293. doi: 10.1111/j.1365-2486.2008.01577.x
Schwienbacher, E., Navarro-Cano, J. A., Neuner, G., and Erschbamer, B. (2012). Correspondence of seed traits with niche position in glacier foreland succession. Plant Ecol. 213, 371–382. doi: 10.1007/s11258-011-9981-4
Sheldon, J. C. (1974). The behaviour of seeds in soil: III. The influence of seed morphology and the behaviour of seedlings on the establishment of plants from surface lying seeds. J. Ecol. 62, 47–66. doi: 10.2307/2258879
Steinbauer, M. J., Grytnes, J. A., Jurasinsk, G., Kulonen, A., Lenoir, J., Pauli, H., et al. (2018). Accelerated increase in plant species richness on mountain summits is linked to warming. Nature 556, 231–234. doi: 10.1038/s41586-018-0005-6
Stöcklin, J., and Bäumler, E. (1996). Seed rain, seedling establishment and clonal growth strategies on a glacier foreland. J. Veg. Sci. 7, 45–56. doi: 10.2307/3236415
Sun, Y., Yan, F., Cui, X., and Liu, F. (2014). Plasticity in stomatal size and density of potato leaves under different irrigation and phosphorus regimes. J. Plant Physiol. 171, 1248–1255. doi: 10.1016/j.jplph.2014.06.002
Tonin, R., Gerdol, R., Tomaselli, R., Petraglia, A., Carbognani, M., and Wellstein, C. (2019). Intraspecific functional trait response to advanced snowmelt suggests increase of growth potential but decrease of seed production in snowbed plant species. Front. Plant Sci. 10:289. doi: 10.3389/fpls.2019.00289
Valdez, J. W., Hartig, F., Fennel, S., and Poschlod, P. (2019). The recruitment niche predicts plant community assembly across a hydrological gradient along plowed and undisturbed transects in a former agricultural wetland. Front. Plant Sci. 10:88. doi: 10.3389/fpls.2019.00088
Van der Schrier, G., Efthymiadis, D., Briffa, K. R., and Jones, P. D. (2007). European Alpine moisture variability for 1800-2003. Int. J. Climatol. 27, 415–427. doi: 10.1002/joc.1411
Van Wijk, M. T., Clemmensen, K. E., Shaver, G. R., Williams, M., Callaghan, T. V., Chapin, F. S. I. I. I., et al. (2004). Long-term ecosystem level experiments at Toolik Lake, Alaska and at Abisko, Northern Sweden: Generalizations and differences in ecosystem and plant type responses to global change. Glob. Change Biol. 10, 105–123. doi: 10.1111/j.1365-2486.2003.00719.x
Vitasse, Y. (2013). Ontogenic changes rather than difference in temperature cause understory trees to leaf out earlier. New Phytol. 198, 149–155. doi: 10.1111/nph.12130
Vittoz, P., Dussex, N., Wassef, J., and Guisan, A. (2009). Diaspore traits discriminate good from weak colonisers on high-elevation summits. Basic Appl. Ecol. 10, 508–515. doi: 10.1016/J.BAAE.2009.02.001
Walck, J. L., Hidayati, S. N., Dixon, K. W., Thompson, K., and Poschlod, P. (2011). Climate change and plant regeneration from seed. Glob. Change Biol. 17, 2145–2161. doi: 10.1111/j.1365-2486.2010.02368.x
Young, T. P., Petersen, D. A., and Clary, J. J. (2005). The ecology of restoration: historical links, emerging issues and unexplored realms. Ecol. Lett. 8, 662–673. doi: 10.1111/j.1461-0248.2005.00764.x
Zimmermann, N. E., Gebetsroither, E., Zuger, J., Schmatz, D., and Psomas, A. (2013). “Future climate of the European Alps,” in Management Strategies to Adapt Alpine Space Forests to Climate Change Risks, eds G. Cerbu, M. Hanewinkel, G. Gerosa, and R. Jandl (London: InTech), 27–36. doi: 10.5772/56278
Keywords: alpine plants, ecological niche, foliar traits, germination, global warming, soil
Citation: Mondoni A, Orsenigo S, Abeli T, Rossi G, Brancaleoni L, Corli A, Iacumin P, Tonin R, Marchesini R and Gerdol R (2020) Plant Regeneration Above the Species Elevational Leading Edge: Trade-Off Between Seedling Recruitment and Plant Production. Front. Ecol. Evol. 8:572878. doi: 10.3389/fevo.2020.572878
Received: 15 June 2020; Accepted: 12 October 2020;
Published: 12 November 2020.
Edited by:
Angela Sierra-Almeida, University of Concepcion, ChileReviewed by:
Susanna Venn, Deakin University, AustraliaDavid Draper, University of Lisbon, Portugal
Fermin J. Rada, University of the Andes, Venezuela
Copyright © 2020 Mondoni, Orsenigo, Abeli, Rossi, Brancaleoni, Corli, Iacumin, Tonin, Marchesini and Gerdol. This is an open-access article distributed under the terms of the Creative Commons Attribution License (CC BY). The use, distribution or reproduction in other forums is permitted, provided the original author(s) and the copyright owner(s) are credited and that the original publication in this journal is cited, in accordance with accepted academic practice. No use, distribution or reproduction is permitted which does not comply with these terms.
*Correspondence: Andrea Mondoni, YW5kcmVhLm1vbmRvbmlAdW5pcHYuaXQ=