- 1Graduate Program in Ecology and Conservation, Universidade Federal do Paraná, Curitiba, Brazil
- 2Division of Ecology and Evolution, Australian National University, Canberra, ACT, Australia
- 3School of BioSciences, University of Melbourne, Parkville, VIC, Australia
Avian nests are critical for successful reproduction in birds. Nest microclimate can affect egg development, chick growth and fledgling success, suggesting that nest building behavior should be under strong selective pressure to nesting conditions. Given that the internal microclimate of the nest is critical for avian fitness, it is expected that nest morphology is shaped by the local environment. Here we review the relationship between nest morphology and climate across species’ distributions. We collate growing evidence that supports a link between environmental conditions and particular nest traits, within species and across species. We discuss the degree to which phenotypic plasticity in nesting behavior can contribute to observed variation in nest traits, the role of phylogenetic history in determining nest morphology, and which nest traits are likely to be influenced by climatic conditions. Finally, we identify gaps in our understanding of the evolution of nest morphology and suggest topics for future research. Overall, we argue that nests are part of the extended phenotype of a bird, they play a crucial role in their reproductive success, and may be an important factor in determining which species will be able to persist in the face of ongoing climate change.
In a Nutshell
• Birds build nests that are suitable for maintaining the ideal microclimate conditions for egg and chick development.
• We discuss the diversity of nest morphologies found among species and individuals.
• We show considerable evidence of building adaptations in nest size, lining and composition to local climate conditions.
• We suggest future research ideas to improve our understanding of how bird nest building behavior evolved.
Introduction
The role of environmental variation in the evolution of animal traits has been the subject of extensive study. There are hundreds of examples of selection on traits to various environmental conditions (Guidi et al., 2016; Meachen et al., 2016; Alhajeri and Steppan, 2018), such as beaks in Darwin’s finches and malagasy vangas that have diversified in different environments (Reddy et al., 2012; Lamichhaney et al., 2015). In the same way that selection acts on phenotypes generating adaptations, selection should exert pressure and influence the evolution of traits that arise from behaviors and are extrinsic to species’ phenotypes (extended phenotypes), such as birds’ nests (Mainwaring, 2015).
Nests are ephemeral structures but they are fundamental for species’ persistence (Hansell, 2000). They are built by parents to provide an optimal environment for their progeny, and several studies have shown that the conditions inside nests can dramatically affect offspring survival (Heenan, 2013; Mainwaring et al., 2014; DuRant et al., 2019). Therefore, nest morphology can be considered a phenotypic trait that should be subject to variation and driven by selection on nest building behavior of parents, like other traits that affect fitness.
There is a tight association between avian reproductive success and the climatic conditions inside the nest (microclimate) (Collias and Collias, 1984). An optimal nest microclimate influences the length of the incubation and nestling periods, which in turn affects parental condition and investment, hatching synchrony and brood size (Lombardo et al., 1995; Ardia et al., 2010; Griffith et al., 2016; Mueller et al., 2019). Nest microclimate can promote optimal embryonic development at the egg stage which enhances brood performance and chick survival (Durant et al., 2013a; Ospina et al., 2018). This is because temperature and humidity that are outside the species’ tolerance range can compromise chick growth (body mass and structural size), influence microbial activity and water loss from eggs, as well as affect innate immunity, thermoregulatory and motor performance, and even sex ratios (Lombardo et al., 1995; Ardia et al., 2010; Deeming, 2016; Rodríguez and Barba, 2016; Larson et al., 2018; Wada et al., 2018; Belnap et al., 2019; Merrill et al., 2019). When incubation and brooding conditions have such fitness consequences, selective pressures that guarantee a suitable nest microclimate are expected to be high (Hansell, 2000; Greno et al., 2008; Hepp and Kennamer, 2012).
Indeed, parents also alter incubation behavior in relation to time of day, time in the season, embryo age, ambient temperature and precipitation (Feldheim, 1997; Álvarez and Barba, 2014; McClintock et al., 2014; Walters et al., 2016; Carroll et al., 2018). Parental behavior is integral to mitigating the effects of weather at the nest, especially extreme weather events, such as heavy rain, flooding or abnormal temperatures (Burger, 1978; Clauser and McRae, 2017). In hot, arid environments, for instance, clutch overheating poses great threats to egg survival and parents modify the duration and frequency of incubation bouts to minimize absences at the nest (Mougeot et al., 2014) and shade eggs to prevent eggs from overheating (Clauser and McRae, 2017). Egg arrangement in the nest and the frequency of egg-turning can also alter cooling/warming rates (Šálek and Zárybnická, 2015). It has been shown that egg turning can be more frequent in unshaded nests and eggs on the edges that are more prone to temperature variation are moved more often (Šálek and Zárybnická, 2015; Kelsey et al., 2016). However, any form of nest structure per se (e.g. cup nest or mat of vegetation versus scrapes on the ground) has important influence on clutch microclimate. Deeming (2011) has shown that humidity within cup nests is more stable across species’ latitudes with distinct levels of humidity than in scrapes. Eggs on scrapes are particularly exposed to the environment, and parents must compensate for weather adversities (Collias and Collias, 1984). In fact, it has been suggested that it is less energetically costly for parents to invest in building a thermally suitable nest to promote optimal development, than to invest in behavioral compensation during incubation (Mainwaring and Hartley, 2013); a suitable nest with stable microclimate optimizes heat exchange in incubating parent/s and reduces heat loss or gain in eggs during parental absence, thereby reducing parental investment (Smith et al., 1974; Collias and Collias, 1984; Reid et al., 2000).
In this review, we aim to highlight the potential role of climate in the evolution of nest building behavior and consequently, nest morphology. We introduce the topic by briefly examining the traits that we use to define nest morphology and discuss to what degree the phylogenetic history of these traits determines their potential to respond to climatic pressures. From there we move on to a discussion of the mechanisms that drive variation in nest construction with special focus on local climate and we suggest topics for future research on the evolution of nest morphology.
To gain insights into how ubiquitous the association between nest morphology and the local climate is, we used the Web of Science (1945–2020) database to search for papers in English on bird nest microclimate and structure, that contained the words: bird and nest temperature or nest structure. Of 1,290 records rendered by this search, 52 papers investigated explicitly the relationship between the climate and nest building adaptations. From these, 88% report significant associations (19.7% of those with humidity and temperature, 13% with humidity only and 56% with temperature only, the remaining showed nest morphology variation across seasons and distributions but did not infer the role of climate). Of the total, 65.4% of studies were of a single species, 34.6% included more than one species, and only 22.2% of those used phylogenetic comparative methods to address broad evolutionary questions on nest building behavior (Supplementary Table 1). As we examine the associations between nests and environment, we explore the extent to which nest building behavior responds to selective pressures and propose new research questions to gain further insights into the relationship between nest and environment. Moreover, although this review focuses on compiling evidence for the role of climate in nest morphology, we also discuss other selective forces that, in conjunction with climate, have the potential to shape nest morphology evolution.
Defining the Morphology of a Nest
Before we consider the role of climate in shaping nest morphology, it is important to clarify how we define nest morphology and the phylogenetic history of the traits involved. We use “nest morphology” to refer to the general nest structure, which is comprised of shape, size, lining, and composition. Like many other structures, it is hard to accurately describe a nest in a single dimension, given the diversity of nest morphologies and their component materials. This complexity reflects the general consensus that all aspects of nest morphology should be taken into account in order to understand function (Zyskowski and Prum, 1999; Hall et al., 2013; Medina, 2019).
Nest Shape
There is general agreement on the categories of classification for overall nest shape (open cup, dome, cavity; Figure 1), and these groupings have been widely used (Price and Griffith, 2017; Duursma et al., 2018; Fang et al., 2018). Broadly, nests can be classified as open cups and domes—those that have a roof. There are also cavity nests, which may or not contain an open cup or a dome within. Some nest shapes are hard to classify, like the pouches of some weavers, that are so deep that they are effectively more similar to a domed nest than an open nest of any kind. Similarly, the nests of some galliforms (like bush-turkey or malleefowl) are not conventional domed nests (with a roof and side entrance) but are, instead, large mounds on the ground where eggs are buried (Hansell, 2000).
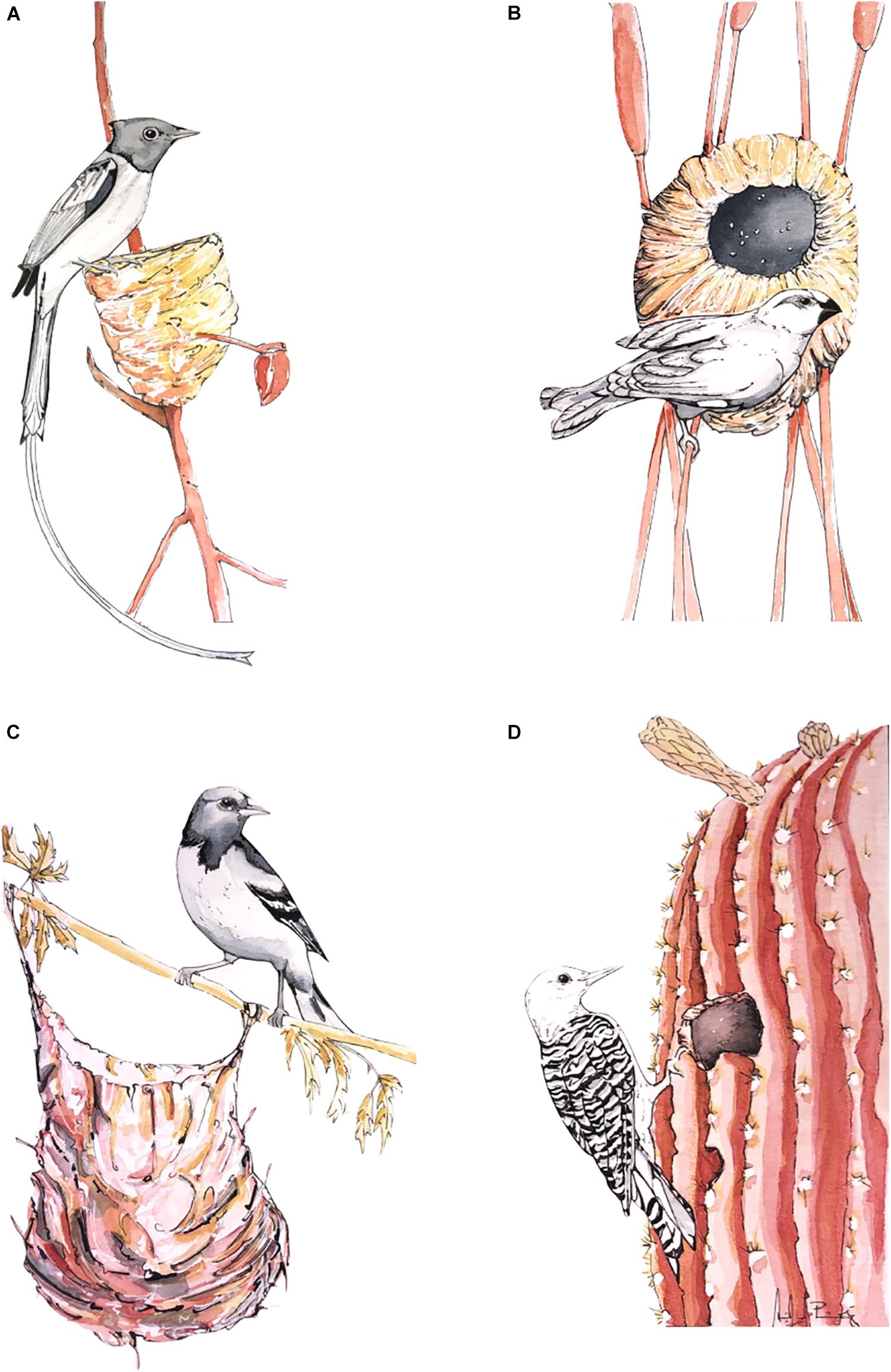
Figure 1. Bird nest shape classification (A) supported cup from Terpsiphone mutata; (B) dome from Amblyospiza albifrons; (C) suspended cup (pouch) from Icterus galbula; (D) cavity from Melanerpes uropygialis. Colors were chosen to contrast birds from nests and do not depict natural colors. Illustration by DMP.
Nest shape has often been considered as an important taxonomic character that is ideal for mapping onto phylogenies for the purposes of ancestral reconstruction (Winkler and Sheldon, 1993; Zyskowski and Prum, 1999). This is because nest shape is considered to be invariable within families and genera, but variable at higher taxonomic levels (Price and Griffith, 2017; Fang et al., 2018; Medina, 2019). Hansell (2000) highlights, however, that some families have species with nests that are cup, domed and in cavities (e.g., Furnariidae, Thraupidae, Psittacidae). Parrots for example, are known for using cavities, but there are a handful of species that do not (e.g., Myopsitta monachus, domed nest).
Nest Size
Besides the general shape, another trait considered part of the morphology of a nest is its size. Many studies use length and width to describe nest size (Heenan and Seymour, 2011; Windsor et al., 2013; Lambrechts et al., 2017), although in the case of domed and cavity nests, width is harder to measure, and width is more often used as a measure for open nests (referred to as inner cup width). Other measures related to nest size that can be useful in describing the general structure of the nest are the cup depth, width of the walls and the thickness of the base.
Unlike general nest shape that is highly conserved across and within species, nest size is much more labile. There is considerable variation in nest size across species, and the main predictor of nest size is the body size of the builder (Slagsvold, 1989; Møller, 2005; Deeming, 2013). However, within individuals there is also significant variation in the nest size. African weavers (Ploceus spp.) build multiple nests during the breeding season and individuals can change the size of their nests over time, suggesting considerable plasticity in this trait, which in fact could be related to changing weather across the season (Walsh et al., 2010, 2011). It is important to consider that African weavers use nest building as part of courtship, so like other phenotypic traits, nest size may be driven by multiple selective pressures, including sexual selection (Soler et al., 1998; Møller, 2005; Moreno, 2012; Tomás et al., 2013). Although only affecting species where nests are sexually selected, if multiple selective forces shape the size of the nest, the potential to respond to climatic conditions might be constrained. Indeed, both sexual and natural selection can drive the evolution of nest size in the same direction (e.g., toward larger size), making it difficult to tease apart the contributing roles of drivers of trait variation. A similar example of concurring selection pressures involves female penduline tits (Remiz pendulinus), which face high energetic demands during incubation and, thus, select a mate based on the insulation capacity of the nest he constructs (Hoi et al., 1994).
Nest Lining and Composition
Another component that will be crucial in our discussion of the links between nests and climate is the lining of the nest. Many birds (but not all) collect feathers, plant down, and/or fur and use them to cover the inner cup, which is thought to be critical in providing insulation. Materials are classified as lining if they have no structural function and are placed within the nest (Hansell, 2000; Mainwaring et al., 2016). This trait is usually quantified by the amount (weight) of lining inside a nest (Mainwaring et al., 2012), but the type of materials used is also important as both are crucial in understanding the thermal efficiency of the nest as a whole. Materials used in nest building are highly variable and can range from mud and saliva in rufous hornero (Furnarius rufus) and swift (Aerodramus fuciphagus) nests, to paper money and bones in raptor nests (Hansell, 2000; Ellis et al., 2009). Thus, when analyzing other nest characteristics, such as mass, it is important to take component materials, and their physical density, into account.
Birds are selective in choosing materials for their nests, which suggests adaptive value in this behavior (Bailey et al., 2014; Muth and Healy, 2014; Briggs and Mainwaring, 2019). The choice of material can be relatively consistent within species (Biddle et al., 2018a). Pied flycatchers (Ficedula hypoleuca) prefer deer fur over other materials (Briggs and Mainwaring, 2019), and zebra finches (Taeniopygia guttata) choose materials based on size and structural properties (Muth and Healy, 2014). Considerable variation across closely related species is also observed. The magpie lark (Grallina cyanoleuca) and its sister species the torrent-lark (Grallina bruijnii) build a cup nest made of mud, but most members of the family (Monarchidae) weave their nests from plant material (Del Hoyo et al., 2017). Indeed, birds can show flexibility in selection of materials observed within species and across very small spatial scales (up to 4 km) (pied flycatchers, Briggs and Deeming, 2016). Parents actively adjust nest building decisions to match the availability of local materials, such that urban populations of multiple species have been shown to incorporate man-made materials (Wang et al., 2009; Suárez-Rodríguez et al., 2013). Thus, unlike other traits like nest shape, the choice (and amount) of nest materials seems to have great evolutionary potential to respond to selective pressures.
The thermal performance and structural properties of the materials used for nest construction are of primary importance, and the way materials are laid down is critical in changing air flow and water absorption thereby influencing insulation capacity (Skowron and Kern, 1980; Rohwer and Law, 2010; Crossman et al., 2011; Deeming and Biddle, 2015; Biddle et al., 2018b,c). However, the choice of nest materials is also associated with factors other than thermal properties that, in combination, enhance breeding success. Males often select nest materials to increase nest appearance and thus his attractiveness, or can also use materials that have anti-parasite benefits (Veiga et al., 2006; Mennerat et al., 2009). The spotless starling uses unpigmented feathers and aromatic plants to enhance antimicrobial protection and nestling survival (Ruiz-Castellano et al., 2018). The European starling adds aromatic herbs to their nests that improve nestling condition through stimulating higher parent attendance and longer incubation bouts (Gwinner et al., 2018).
Nest Morphology and Climate
Nest Shape
Among species that build a nest structure, the presence or absence of a roof, or whether or not, it is placed in a cavity, can have large effects on the thermal performance (Griffith et al., 2016), leading to the expectation of intraspecific variation in nest shape in species with wide geographic ranges. It is puzzling that within-species, the potential to dramatically alter nest shape (e.g., from cup to domed or vice versa) appears to be rare (Cardoni et al., 2017). It is possible that general nest shape is already well adapted to environmental conditions in the species’ current distribution, such that variation at this level is unnecessary at smaller taxonomic scales. Alternatively, changing nest shape could require high levels of flexibility in building behaviors, which might be difficult to develop. Although very rare, there are examples of variation in nest shape within species. One example is the golden-headed cisticola (Cisticola exilis), which is described as having open cup nests in some parts of its distribution in NE India and domed nests in other parts (Del Hoyo et al., 2017), but the causes for these differences are unknown. Another interesting case is that of the bay-capped wren-spinetail (Spartonoica maluroides), which builds two different nest types in the same region in Argentina, but the causes for such variation are not completely clear either (Cardoni et al., 2017). These systems might be ideal to investigate the role of climate in driving intraspecific nest variation at broader scales. Current information, however, is not enough to draw any conclusions, and climate is not the sole selective pressure acting on nest shape. For example, weavers, which have domed nests, are heavily parasitized by cuckoos in Africa and their domed nests have been suggested to decrease parasitism rates (Krüger and Davies, 2004; Medina et al., 2020).
Nest shape can play an important role in extreme environments where suboptimal nest design can be lethal to the offspring. The zebra finch, for example, faces high temperatures during the breeding season in Australia, and there is some evidence that by constructing domed nests parents can protect the eggs from direct sunlight which may reduce the risk of eggs reaching lethal temperatures (Griffith et al., 2016). Indeed, looking at the interspecific level some recent studies have suggested that domed nests might be a type of specialization in arid environments. Duursma et al. (2018) found that in Australia, arid places have a higher frequency of domed nest species compared to other non-arid regions. Conversely, Medina (2019) did not find associations between nest shape and particular environments in Australian species, but evidence that species with domed nests have smaller distributions and suggested that dome nests were lost as birds expanded to other environments and the range of climatic conditions. Nevertheless, these findings remain to be rigorously tested worldwide, through broad comparative studies that take into account phylogenetic, and spatial correlations.
Overall, explanations for the evolution of nest shape in passerine birds have focused on two main hypotheses: predation pressure versus microclimate variation (Martin et al., 2017). According to a long-standing assumption, domed nests are more common in tropical and southern hemisphere regions because the shape of the dome reduces predation risk (Oniki, 1979). However, studies have recently revealed that predation rates are similar for different nest types (cup and dome) and across latitudes (Martin et al., 2017; Mouton and Martin, 2019), and birds seem to respond to predation risk by changing nest placement rather than nest shape (Forstmeier and Weiss, 2004; Peluc et al., 2008). In fact, domed nests are associated with smaller absolute body size in passerines, supporting thermoregulatory explanations for their evolution (Martin et al., 2017). Smaller individuals have higher rates of heat exchange due to their disproportionately large surface area to volume ratios compared with larger-bodied individuals, with consequences for energy and water budgets at both ends of the temperature scale (hot and cold conditions) (Boyles et al., 2011). Domed nests that confer a stable microclimate, with protection from temperature extremes, rainfall and sun exposure, may reduce the thermoregulatory costs of attending parents and provide thermal benefits for embryos and nestlings. Such benefits may lead to a reduction in parental effort, increased offspring growth rates and reductions in the lengths of the incubation and nestling periods, thereby reducing predation risk, with disproportionate effects for smaller species (Martin et al., 2017; Matysiokov and Remes, 2018).
In summary, studies have pointed to considerably low intraspecific variability in nest shape, and species with such variation are still poorly investigated. Under the broad interspecific level, however, nest shape may represent an important adaptation to the selective pressures of the environment with dome nests commonly occurring in small ranges and arid places (Duursma et al., 2018; Medina, 2019). Studies have also demonstrated associations between dome nests and body size highlighting the thermal function of these structures, although further research is still required to fully dissociate climate-related hypotheses from predation risk (Martin et al., 2017; Medina, 2019). Future research testing for associations between nest shape (e.g., domed versus cup), passerine body size and the nature and extent of weather extremes, both hot and cold, may improve our understanding of the direct effects of weather on nest microclimate versus body size-related thermoregulatory costs.
Nest Size
Traditionally, large nests are thought to be adaptive in cooler regions where they can confer a more stable microclimate than smaller nests (Collias and Collias, 1984) and, thus, climate-driven selection pressures on nest size are likely to be pronounced. Variation in nest size is more extensively investigated at the intraspecific level across large geographic ranges with distinct environmental conditions or in relation to the length of breeding seasons where large shifts in weather are observed across months. For instance, the ring ouzel (Turdus torquatus) is widely distributed in Eurasia, including across elevations, such as in the montane regions of West Carpathians, Slovakia (Janinga and Višòovská, 2004). Variation in nest width and depth in this species is correlated with differences in the climate (wider and deeper nests are found in cooler climates), a result of either latitude or elevation gradients (Janinga and Višòovská, 2004). The yellow warbler (Dendroica petechia) shows even more pronounced building modifications, whose distribution extends to subarctic climate where they build larger, thicker, taller cup nests that are also less porous and more insulated than nests from warmer regions (Rohwer and Law, 2010).
Although there is a good deal of correlational evidence for a link between nest size and local climate, studies need to apply scrutiny in assigning causal mechanisms and consider additional factors that are potentially important in the interplay between climate and nest size (or morphology in general). For example, in the black-throated blue warblers (Setophaga caerulescens), nest wall thickness increases with elevation where temperatures are generally lower. Yet, there is no relationship between wall thickness and ambient temperature during the breeding season (Smith et al., 2018) and further studies are needed to understand the real extent of temperature in driving differences in nest size across elevation in the species. Likewise, the bearded reedling (Panurus biarmicus) builds larger and thicker cup nests when facing lower daily minimum temperatures, but nest height and the shape of the base are influenced mostly by the density of reeds, as these birds adapt their nests to the structure of the supporting vegetation (Malzer and Hansell, 2017). The cavity nester, thorn-tailed rayadito (Aphrastura spinicauda) builds smaller nests in warmer months as a consequence of using less insulating material (Botero-Delgadillo et al., 2017). However, a smaller nest size is not necessarily the result of selection for cooler nests in warmer temperatures, as time constraints on breeding later in the season might cause parents to accelerate the nest building process, or nest material might simply become scarce, thereby affecting nest morphology (Botero-Delgadillo et al., 2017). In a similar example, the wood thrush (Hylocichla musteline), builds shorter, shallower and thinner open cup nests as the season progresses and temperatures increase (Powell and Rangen, 2000). However, the increasing energetic constraints parents face as the season advances could also cause a reduction in building effort over time. Additionally, bigger nests might be more exposed to predators later in the season when leaf-out occurs, suggesting variation in nest construction may be driven by changing predation pressure (Powell and Rangen, 2000).
Indeed, selection for larger nests could be constrained by predation risk, since many predators are diurnal and use vision to locate nests, and it is known that bigger nests, relative to body size, are more susceptible to predation (Møller, 1990; Martin and Li, 1992; Biancucci and Martin, 2010; Mouton and Martin, 2019). In fact, the pressure to construct small and inconspicuous nests may outweigh the pressure to produce a suitable nest microclimate (Møller, 1990; Crossman et al., 2011, but see Akresh et al., 2017; Kubelka et al., 2019). These competing selection pressures can be particularly important for exposed, open cup nesters (Matysiokov and Remes, 2018). If competing pressures constrain the evolution of thermally adapted structures, parents might compensate for the thermal deficiencies of smaller nests by spending more time incubating or covering the clutch with leaves to regulate the temperature and relative humidity, although the latter strategy can also be employed to camouflage the clutch (Collias and Collias, 1984; Kreisinger and Albrecht, 2008; Prokop and Trnka, 2011). However, supposing that parents instead build thicker walls and base, the resulting reduction in the area of the inner cup could limit clutch or egg size (Suárez et al., 2005), and parents would face a trade-off between predation avoidance and offspring production. Although it is also possible that smaller nests have denser walls or that nest size (cup space) is not linked to clutch size (Antonov, 2004; Biancucci and Martin, 2010; Akresh et al., 2017; Malzer and Hansell, 2017). Collating data on nest dimensions and density for a wide range of species from distinct climatic regions and predator regimes would be key to further our understanding on this system by testing two inter-related questions: could predation and thermal selection pressures on open nests lead to lower offspring production?; and would a decrease in size be compensated by material choice and wall density?
The evidence presents a tight link between nest size and thermal properties of the nest, but structural support might be a very important pressure as well. An investigation with cup nest from 36 Australian species revealed that as species body size increases, nest surface area increases isometrically, but nest wall thickness increases on a higher scale than would be expected isometrically or if nests were built to prevent heat loss (Heenan and Seymour, 2011). This result led the authors to conclude that structural support was the most fundamental selective pressure driving the evolution in nest size among cup nesters (Heenan and Seymour, 2011). However, this study did not look into the relationships between nest morphology and the environment, and a later investigation using the same species revealed that nest insulation is in fact highly correlated with local climate (Heenan et al., 2015). Thicker nest base and better insulating materials, such as wool and feathers, are used by different species in cool climates and thinner nest base and poorly insulating materials, such as sticks and grasses, in warm climates. This trend is more pronounced when accounting for humidity, where species from warm climates facing high precipitation build nests that are poorly insulated and consequently less absorptive (better draining). Authors concluded that nest material, more than nest size, is the central element varying with climate across Australian cup nesting species (Heenan et al., 2015).
Unlike nest shape, nest size shows considerably high variation both within and between species. The variety of nest sizes seems to match the constraints of local and temporal climate conditions, where larger structures are built in cooler environments. From a macroevolutionary perspective, size seems to correlate with climate where nests with denser walls and thicker base are found in cooler and drier regions (Heenan et al., 2015). However, a clear trend is not always detected as many interplaying factors seem to drive nest shape (Kern et al., 1993; Biancucci and Martin, 2010) and further studies including phylogenetic comparisons a global sample of species are needed to reach a thorough understanding of these findings.
Nest Lining and Composition
Nest lining can comprise a large part of the total nest mass and is among the most flexible of nest traits (McGowan et al., 2004). In particular, species that face changing weather conditions throughout the months (e.g., those with long breeding seasons in temperate regions), show large variation in the composition and amount of nest lining (Mainwaring and Hartley, 2008; Akresh et al., 2017). For example, in cavity nesters, the amount of animal hair in collared flycatcher nests and the diversity of animal hair in great tit and blue tit nests decreases as the breeding season advances. This suggests that the insulating properties of fur are no longer necessary as ambient temperatures increase (Harnièárová and Adamík, 2016).
Seasonal change in the composition and amount of lining, however, can be a by-product of material availability. The preference for animal hair early in the season may reflect low availability of plant and other nest materials (Harnièárová and Adamík, 2016). In the blue tit, the mass of nest lining also declines with the season as ambient temperatures increase. Authors suggest that the decrease in lining is adaptative, rather than a reflection of availability, because nest base mass remains constant over the season (Mainwaring and Hartley, 2008). Similarly, in long-tailed tits, a dome nesting species, seasonal decline in nest lining mass could be attributed to changes in resource availability. However, by supplying feathers to breeding pairs, McGowan et al. (2004) confirmed that parents incorporate lining material in a manner that matched insulating properties with local temperature.
Besides variation in nest lining and materials within individuals through the season, there is also regional variation in the types of materials used in nest construction. Populations from the same species that inhabit different regions choose materials depending on regional availability (Clark, 1991; Suárez-Rodríguez et al., 2013). Nest composition is the central factor for nest microclimate in the mound nest of the Australian bush-turkey. Males build mounds of leaf litter on the ground and the balance between the amount of material, water and sufficient mixing provides the appropriate temperature through microbial heat generation. Mounds can self-generate heat for up to several weeks and it is known that in higher latitudes the rate of decomposition is lower than in lower latitudes. Curiously, this is directly due to material composition, and male bush-turkeys have to compensate for the low rate of decomposition of plant species in higher latitudes by using more material (Seymour and Bradford, 1992). The common amakihi (Hemignathus virens virens) is distributed across a range of elevations in the islands of Hawaii with distinct vegetation communities and climatic conditions. The environmental breadth across this species range led to high variation in nest morphology, which was associated with successful breeding under varying environmental conditions. The common amakihi adjusts insulation by changing composition in elevated areas with cold, dry conditions compared with low wetland areas with extremely hot, wet conditions (van Riper, 1980; Kern and Van Riper, 1984). In addition, nests in warmer areas are built higher in the canopy and closer to the tree’s trunk, while in cooler areas, nests are placed near the edge of the canopy, possibly to optimize exposure to sunlight (Kern and Van Riper, 1984). Furthermore, this example provides evidence that nest material as well as placement have a combined role in conferring a suitable microclimate (Horvath, 1964; Kern and Van Riper, 1984). The tight link between habitat and nest building shows the importance of habitat conservation and the availability of nest materials and nesting locations. Such impacts should test a species’ ability to adaptively respond by changing its nest morphology to conform to the newly imposed conditions (Martin, 2014).
In summary, similarly to nest size, there is considerable variation in nest composition within and between species. In support to the climate adaptation hypothesis, less insulating material is used in lower latitudes and altitudes, but a stronger pattern is observed with the advancement of breeding season and a consequent increase in temperature (e.g., Mainwaring and Hartley, 2008; Deeming et al., 2012). Yet, we still observe high building variability specially across locations (Supplementary Table 2) evidencing that alternative selective pressures, such the availability of nest materials, represent constraints to nest lining and composition. In particular, the level of habitat conservation has a direct impact on material availability and broad scale studies testing species’ ability to adaptively respond to environmental degradation by changing nest construction should reveal interesting patterns on how species conform to unfamiliar and unexpected conditions and if that hampers building adaptation to climate conditions (Martin, 2014).
Future Directions
We highlight that many of the examples identified in this review are correlative and, so, causal relationships have only been inferred. Thus, it is important to exert caution when drawing conclusions about factors underlying nest building flexibility, as multiple factors can influence nest building behavior and morphology. We have identified several of these, including predation pressure, anti-parasite benefits, sexual selection, other parental strategies, availability of nest material and time constraints. In addition, a paucity of phylogenetic breadth in investigations of nest building behavior also points to a wide gap in our knowledge of the evolution of nest morphology. In particular, although also correlative, broad phylogenetic scales can provide an evolutionary perspective about the broad and global correlates of climate and nest morphology. Here we point to research directions that, to date, have received insufficient or no attention, and if explored with experiments or on a macroevolutionary scale, will be crucial in further elucidating a comprehensive understanding of nest building behavior evolution.
As we show during this review, humidity seems to strongly influence nest construction behavior (Heenan et al., 2015), although, most studies of nest microclimate primarily consider the effect of temperature (73%, Supplementary Table 1). Recent advances in our understanding of thermal physiology suggest that humidity may have consequences for chick growth and parental body condition via increasing the costs of thermoregulation; high humidity reduces the gradient driving evaporation thereby reducing the rate of heat loss via evaporative cooling, with potential to compromise energy and water budgets (Gerson et al., 2014). This may be particularly important in the tropics where high humidity coincides with high temperatures, and evaporative cooling via panting is the primary means by which passerines dissipate excess body heat. Comparing the morphology and microclimates of nests of tropical species with those from other climate zones may provide greater insights into how weather as a whole, rather than simply temperature, shapes the evolution of nest morphology.
Another important factor that deserves careful consideration when investigating climate-related selection pressures on nest morphology is the embryo physiology. Altricial and precocial species have different thermoregulatory capacities early in life (DuRant et al., 2013b), which may pose distinct nest microclimate demands. Precocial species possess an early capacity to maintain body temperatures, as they are quite mature at hatching when they may not depend on nest microclimate anymore. Much of the energy in precocial chicks is, therefore, allocated to thermoregulation and, as a consequence, these birds grow at slower rates. Conversely, altricial nestlings grow at higher rates, but depend on nest microclimate and parental care to maintain optimal body temperatures (Tortosa and Villafuerte, 1999). In that way, nest insulation could be highly crucial for altricial species until they reach an age where they present effective endothermy. These two types of embryo development are likely to have been important in driving the evolution of nest morphology and positioning, because maintaining an adequate environment for nestlings could lead to stronger pressures in altricial species (DuRant et al., 2013b). This idea has not been tested before, but many Paleognaths like emus and other precocial species have minimal nests or lack any type of nest structure. Alternatively, given the fast embryonic development in precocial species, it is also possible that nest microclimate is equally or more important to these species, or that they possess high levels of embryonic resistance (Fu et al., 2017). Phylogenetic comparative studies on nest morphology are needed, including non-passerine species—which are mostly precocial—because they will have the potential to reveal which nest traits are essential for chick development. Comparing the structure and microclimates of nests of species with different developmental modes may provide insights into which traits of nest morphology are important for incubation and which others are important for chick development and protection.
Conclusion
We have shown that variation in climate conditions across space (latitudinal and altitudinal gradients) and over timescales (years and breeding season) are important drivers of nest building behavior. We have provided multiple examples of how variation in nest morphology can be explained as an adaptive response to climatic variation, although many studies are correlative. Nest shape, and to a lesser extent, nest size, lining and composition are components of nest morphology that are heritable and phylogenetically conserved (Møller, 2005; Heenan et al., 2015; Price and Griffith, 2017; Fang et al., 2018; Medina, 2019). This conservatism would primarily indicate relative resistance to selective pressures. However, high phylogenetic signal may generally reflect that building behavior interacts with evolutionary pressures of species local climate under a large macroevolutionary scale (Heenan et al., 2015; Price and Griffith, 2017; Medina, 2019). In particular, nest composition has been pointed as the central component responding to climatic selective drivers, as insulation is higher in low ambient temperature and humidity (Skowron and Kern, 1980; Heenan and Seymour, 2011; Heenan et al., 2015). Parallelly, at the intraspecific level, nest shape is markedly less labile than the remaining features of nest morphology revealing that it is likely less subject to selection. As a consequence, we observe a considerably higher number of studies investigating adaptations in size, lining and composition within species, all traits that seem correlated (92%, Supplementary Table 1). We have observed that species generally tend to converge on similar solutions for nest design under similar environmental conditions, although a clear trend cannot be conclusively drawn (Supplementary Tables 1, 2). Nests tend to be bigger and contain more material in higher latitudes and elevations (61% of studies) but mostly so early in breeding seasons (76% of studies; Supplementary Tables 1, 2). These adaptations are most commonly driven by low temperatures where bigger diameters, thicker walls, deeper inner cups and more insulating materials confer protection against heat loss, although the local ambient humidity is also crucial in determining if nests will be thicker and more insulated (Supplementary Tables 1, 2).
More studies of nest morphology, with broad global representation and larger temporal scales, will provide greater understanding of how, and to what extent, climatic variation shapes nest morphology and provide insight into whether birds can adaptively respond to rapid changes in contemporary climate (Mainwaring, 2015). Our review provides strong evidence for links between nest morphology and climatic variation, suggesting that nest building behavior can respond to temporal changes in contemporary climate. Indeed, a recent study showed that Goshawks in Denmark have been responding to rising spring temperatures over the past several decades (1977–2014) by increasing nest size (Møller and Nielsen, 2015). However, whether such shifts will be sufficient to track changing climate remains in question and requires greater understanding of whether adaptation will require evolutionary, genetic change in nest construction behavior or can be achieved via plasticity in existing behavior (Møller, 2005). Our review details high levels of plasticity in nest building behavior associated with particular nest traits (e.g., nest size and composition are more flexible than nest shape) but more research is needed on the genetic basis of these behaviors, if we are to improve our capacity to predict species’ responses to ongoing climate change.
Author Contributions
DMP and IM designed the study. DMP, JLG, and IM performed the bibliographic research and prepared the manuscript. All authors contributed to the article and approved the submitted version.
Funding
This study was financed by the Coordenação de Aperfeiçoamento de Pessoal de Nível Superior – Brazil (CAPES) – Finance code 001 to DMP, the Australian Research Council Future Fellowship (FT150100139) to JLG, and a McKenzie Fellowship to IM.
Conflict of Interest
The authors declare that the research was conducted in the absence of any commercial or financial relationships that could be construed as a potential conflict of interest.
Supplementary Material
The Supplementary Material for this article can be found online at: https://www.frontiersin.org/articles/10.3389/fevo.2020.566018/full#supplementary-material
References
Akresh, M. E., Ardia, D. R., and King, D. I. (2017). Effect of nest characteristics on thermal properties, clutch size, and reproductive performance for an open-cup nesting songbird. Avian Biol. Res. 10, 107–118. doi: 10.3184/175815617x14878495604724
Alhajeri, B. H., and Steppan, S. J. (2018). A phylogenetic test of adaptation to deserts and aridity in skull and dental morphology across rodents. J. Mammal. 99, 1197–1216. doi: 10.1093/jmammal/gyy099
Álvarez, E., and Barba, E. (2014). Behavioural responses of great tits to experimental manipulation of nest temperature during incubation. Ornis Fennica 91, 220–230.
Antonov, A. (2004). Smaller eastern olivaceous warbler Hippolais pallida elaeica nests suffer less predation than larger ones. Acta Ornithol. 39, 87–92. doi: 10.3161/068.039.0205
Ardia, D. R., Pérez, J. H., and Clotfelter, E. D. (2010). Experimental cooling during incubation leads to reduced innate immunity and body condition in nestling tree swallows. Proc. R. Soc. B Biol. Sci. 277, 1881–1888. doi: 10.1098/rspb.2009.2138
Bailey, I. E., Morgan, K. V., Bertin, M., Meddle, S. L., and Healy, S. D. (2014). Physical cognition: birds learn the structural efficacy of nest material. Proc. R. Soc. B Biol. Sci. 281:20133225. doi: 10.1098/rspb.2013.3225
Belnap, S. C., Currea, J. P., and Lickliter, R. (2019). Prenatal incubation temperature affects neonatal precocial birds’ locomotor behavior. Physioll Behav. 206, 51–58. doi: 10.1016/j.physbeh.2019.03.002
Biancucci, L., and Martin, T. E. (2010). Can selection on nest size from nest predation explain the latitudinal gradient in clutch size? J. Anim. Ecol. 79, 1086–1092. doi: 10.1111/j.1365-2656.2010.01720.x
Biddle, L. E., Broughton, R. E., Goodman, A. M., and Deeming, D. C. (2018a). Composition of bird nests is a species-specific characteristic. Avian Biol. Res. 11, 132–153. doi: 10.3184/175815618x15222318755467
Biddle, L. E., Deeming, D. C., and Goodman, A. M. (2018b). Birds use structural properties when selecting materials for different parts of their nests. J. Ornithol. 159, 999–1008. doi: 10.1007/s10336-018-1571-y
Biddle, L. E., Goodman, A. M., and Deeming, D. C. (2018c). Infrared thermography provides insight into the thermal properties of bird nests. J. Therm. Biol. 76, 95–100. doi: 10.1016/j.jtherbio.2018.07.008
Botero-Delgadillo, E., Serrano, D., Orellana, N., Poblete, Y., and Vásquez, R. A. (2017). Effects of temperature and time constraints on the seasonal variation in nest morphology of the Thorn-tailed Rayadito (Aphrastura spinicauda). Emu Austr. Ornithol. 117, 181–187. doi: 10.1080/01584197.2017.1298400
Boyles, J. G., Seebacher, F., Smit, B., and McKechnie, A. E. (2011). Adaptive thermoregulation in endotherms may alter responses to climate change. Integr. Compar. Biol. 51, 676–690. doi: 10.1093/icb/icr053
Briggs, K. B., and Deeming, D. C. (2016). Use of materials in nest construction by Pied Flycatchers Ficedula hypoleuca reflects localized habitat and geographical location. Bird Study 63, 516–524. doi: 10.1080/00063657.2016.1238867
Briggs, K. B., and Mainwaring, M. C. (2019). Experimental evidence of non-random nest material selection in pied flycatchers. Behav. Proces. 164, 59–64. doi: 10.1016/j.beproc.2019.04.008
Burger, J. (1978). Determinants of nest repair in laughing gulls. Anim. Behav. 26, 856–861. doi: 10.1016/0003-3472(78)90152-5
Cardoni, D. A., Isacch, J. P., and Baladrón, A. (2017). Causes and consequences of nest mass and structure variation in the Bay-capped Wren-spinetail Spartonoica maluroides. Acta Ornithol. 52, 51–58. doi: 10.3161/00016454ao2017.52.1.005
Carroll, R. L., Davis, C. A., Fuhlendorf, S. D., Elmore, R. D., DuRant, S. E., and Carroll, J. M. (2018). Avian parental behavior and nest success influenced by temperature fluctuations. J. Therm. Biol. 74, 140–148. doi: 10.1016/j.jtherbio.2018.03.020
Clark, L. (1991). The nest protection hypothesis: the adaptive use of plant secondary compounds by European starlings. Bird Parasite Interact. Ecol. Evol. Behav. 3, 205–221.
Clauser, A. J., and McRae, S. B. (2017). Plasticity in incubation behavior and shading by king rails Rallus elegans in response to temperature. J. Avian Biol. 48, 479–488. doi: 10.1111/jav.01056
Collias, N. E., and Collias, E. C. (1984). Nest Building and Bird Behavior. Princeton: Princeton University Press.
Crossman, C. A., Rohwer, V. G., and Martin, P. R. (2011). Variation in the structure of bird nests between northern Manitoba and Southeastern Ontario. PLoS One 6:e19086. doi: 10.1371/journal.pone.0019086
Deeming, D. (2013). Effects of female body size and phylogeny on avian nest dimensions. Avian Biol. Res. 6, 1–11. doi: 10.3184/175815512x13528955707337
Deeming, D. C. (2011). Importance of nest type on the regulation of humidity in bird nests. Avian Biol. Res. 4, 23–31. doi: 10.3184/175815511x13013963263739
Deeming, D. C. (2016). How does the bird-nest incubation unit work? Avian Biol. Res. 9, 103–113. doi: 10.3184/175815516x14567543242701
Deeming, D. C., and Biddle, L. E. (2015). Thermal properties of bird nests depend on air-gaps between the materials. Acta Ornithol. 50, 121–125. doi: 10.3161/00016454ao2015.50.1.011
Deeming, D. C., Mainwaring, M. C., Hartley, I. R., and Reynolds, S. J. (2012). Local temperature and not latitude determines the design of Blue Tit and Great Tit nests. Avian Biol. Res. 5, 203–208. doi: 10.3184/175815512x13528874959581
Del Hoyo, J., Elliot, A., and Sargatal, J. (2017). Handbook of the Birds of the World Online. Barcelona: Lynx Editions.
Durant, S. E., Hopkins, W. A., Carter, A. W., Stachowiak, C. M., and Hepp, G. R. (2013a). Incubation conditions are more important in determining early thermoregulatory ability than posthatch resource conditions in a precocial bird. Physiol. Biochem. Zool. 86, 410–420. doi: 10.1086/671128
DuRant, S. E., Hopkins, W. A., Hepp, G. R., and Walters, J. (2013b). Ecological, evolutionary, and conservation implications of incubation temperature−dependent phenotypes in birds. Biol. Rev. 88, 499–509. doi: 10.1111/brv.12015
DuRant, S. E., Willson, J. D., and Carroll, R. B. (2019). Parental effects and climate change: Will avian incubation behavior shield embryos from increasing environmental temperatures? Integr. Compar. Biol. 59, 1068–1080. doi: 10.1093/icb/icz083
Duursma, D. E., Gallagher, R. V., Price, J. J., and Griffith, S. C. (2018). Variation in avian egg shape and nest structure is explained by climatic conditions. Sci. Rep. 8:4141.
Ellis, D. H., Craig, T., Craig, E., Postupalsky, S., LaRue, C. T., Nelson, R. W., et al. (2009). Unusual raptor nests around the world. J. Raptor Res. 43, 175–199. doi: 10.3356/jrr-08-110.1
Fang, Y.-T., Tuanmu, M.-N., and Hung, C.-M. (2018). Asynchronous evolution of interdependent nest characters across the avian phylogeny. Nat. Commun. 9:1863.
Feldheim, C. L. (1997). The length of incubation in relation to nest initiation date and clutch size in Dabbling ducks. Condor 99, 997–1001. doi: 10.2307/1370155
Forstmeier, W., and Weiss, I. (2004). Adaptive plasticity in nest−site selection in response to changing predation risk. Oikos 104, 487–499. doi: 10.1111/j.0030-1299.1999.12698.x
Fu, Y., Dai, B., Wen, L., Chen, B., Dowell, S., and Zhang, Z. (2017). Unusual incubation behavior and embryonic tolerance of hypothermia in the Sichuan Partridge (Arborophila rufipectus). J. Ornithol. 158, 707–715. doi: 10.1007/s10336-016-1422-7
Gerson, A. R., Smith, E. K., Smit, B., McKechnie, A. E., and Wolf, B. O. (2014). The impact of humidity on evaporative cooling in small desert birds exposed to high air temperatures. Physiol. Biochem. Zool. 87, 782–795. doi: 10.1086/678956
Greno, J. L., Belda, E. J., and Barba, E. (2008). Influence of temperature during the nestling period on post-fledging survival of great tir Parus major in a Mediterranean habitat. J. Avian Biol. 39, 41–49. doi: 10.1111/j.0908-8857.2008.04120.x
Griffith, S. C., Mainwaring, M. C., Sorato, E., and Beckmann, C. (2016). High atmospheric temperatures and ‘ambient incubation’ drive embryonic development and lead to earlier hatching in a passerine bird. R. Soc. Open Sci. 3, 150371–150371. doi: 10.1098/rsos.150371
Guidi, L., Semprucci, F., Cesaroni, L., and Balsamo, M. (2016). Morphological and functional adaptations of nematodes to habitat types 16 th International Meiofauna Conference. J. Exp. Mar. Biol. Ecol. 502, 1–149.
Gwinner, H., Capilla-Lasheras, P., Cooper, C., and Helm, B. (2018). ‘Green incubation’: Avian offspring benefit from aromatic nest herbs through improved parental incubation behaviour. Proc. R. Soc. B Biol. Sci. 285: 1880.
Hall, Z. J., Street, S. E., and Healy, S. D. (2013). The evolution of cerebellum structure correlates with nest complexity. Biol. Lett. 9:20130687. doi: 10.1098/rsbl.2013.0687
Harnièárová, K., and Adamík, P. (2016). Mammal hair in nests of four cavity-nesting songbirds: occurrence, diversity and seasonality. Bird Study 63, 181–186. doi: 10.1080/00063657.2016.1183584
Heenan, C. B. (2013). An overview of the factors influencing the morphology and thermal properties of avian nests. Avian Biol. Res. 6, 104–118. doi: 10.3184/003685013x13614670646299
Heenan, C. B., and Seymour, R. S. (2011). Structural support, not insulation, is the primary driver for avian cup-shaped nest design. Proc. R. Soc. Lond. B Biol. Sci. 278, 2924–2929. doi: 10.1098/rspb.2010.2798
Heenan, C. B., Goodman, B. A., and White, C. R. (2015). The influence of climate on avian nest construction across large geographical. Glob. Ecol. Biogeogr. 24, 1203–1211. doi: 10.1111/geb.12378
Hepp, G. R., and Kennamer, R. A. (2012). Warm is better: incubation temperature influences apparent survival and recruitment of wood ducks (Aix sponsa). PLoS One 7:e47777. doi: 10.1371/journal.pone.0047777
Hoi, H., Schleicher, B., and Valera, F. (1994). Female mate choice and nest desertion in penduline tits, Remiz pendulinus: The importance of nest quality. Anim. Behav. 48, 743–746. doi: 10.1006/anbe.1994.1296
Horvath, O. (1964). Seasonal differences in Rufous Hummingbird nest height and their relation to nest climate. Ecology 45, 235–241. doi: 10.2307/1933836
Janinga, M., and Višòovská, Z. (2004). The placement and the size and shape of nests of Ring Ouzel (Turdus torquatus) reflect the environmental conditions at different altitude. Oecol. Montana 13, 11–16.
Kelsey, E. C., Bradley, R. W., Warzybok, P., Jahncke, J., and Shaffer, S. A. (2016). Environmental temperatures, artificial nests, and incubation of Cassin’s auklet. J. Wildlife Manag. 80, 292–299. doi: 10.1002/jwmg.1012
Kern, M. D., and Van Riper, C. (1984). Altitudinal variations in nests of the Hawaiian Honeycreeper Hemignathus virens virens. Condor 86, 443–454. doi: 10.2307/1366825
Kern, M. D., Sogge, M. K., Kern, R. B., and van Riper, C. III (1993). Nests and nest sites of the San Miguel Island song sparrow (Melospiza melodia micronyx). J. Field Ornithol. 64, 367–381.
Kreisinger, J., and Albrecht, T. (2008). Nest protection in mallards Anas platyrhynchos: untangling the role of crypsis and parental behaviour. Func. Ecol. 22, 872–879. doi: 10.1111/j.1365-2435.2008.01445.x
Krüger, O., and Davies, N. B. (2004). The evolution of egg size in the brood parasitic cuckoos. Behav. Ecol. 15, 210–218. doi: 10.1093/beheco/arg104
Kubelka, V., Sládeèek, M., and Šálek, M. (2019). Great variability in nest lining size: support for thermoregulation but not for anti-predatory adaptation hypothesis. J. Ornithol. 160, 993–1002. doi: 10.1007/s10336-019-01670-x
Lambrechts, M. M., Blondel, J., de Franceschi, C., and Doutrelant, C. (2017). Nest size is positively correlated with fledging success in Corsican Blue Tits (Cyanistes caeruleus) in an insular oak-dominated habitat mosaic. J. Ornithol. 158, 125–132. doi: 10.1007/s10336-016-1377-8
Lamichhaney, S., Berglund, J., Almén, M. S., Maqbool, K., Grabherr, M., Martinez-Barrio, A., et al. (2015). Evolution of Darwin’s finches and their beaks revealed by genome sequencing. Nature 518:371. doi: 10.1038/nature14181
Larson, E. R., Eastwood, J. R., Micallef, S., Wehbe, J., Bennett, A. T. D., and Berg, M. L. (2018). Nest microclimate predicts bill growth in the Adelaide rosella (Aves: Psittaculidae). Biol. J. Linnean Soc. 124, 339–349.
Lombardo, M. P., Bosman, R. M., Faro, C. A., Houtteman, S. G., and Kluisza, T. S. (1995). Effect of feathers as nest insulation on incubation behavior and reproductive performance of tree swallows (Tachycineta bicolor). Am. Ornithol. 112, 973–981. doi: 10.2307/4089028
Mainwaring, M. C. (2015). “Nest construction and incubation in a changing climate,” in Nests, Eggs, and Incubation: New Ideas About Avian Reproduction, eds D. C. Deeming and S. J. Reynolds (Oxford: Oxford University Press), 65–74. doi: 10.1093/acprof:oso/9780198718666.003.0006
Mainwaring, M. C., and Hartley, I. R. (2008). Seasonal adjustments in nest cup lining in Blue Tits Cyanistes caeruleus. Ardea 96, 278–282. doi: 10.5253/078.096.0213
Mainwaring, M. C., and Hartley, I. R. (2013). The energetic costs of nest building in birds. Avian Biol. Res. 6, 12–17. doi: 10.3184/175815512x13528994072997
Mainwaring, M. C., Hartley, I. R., Bearhop, S., Brulez, K., du Feu, C. R., Murphy, G., et al. (2012). Latitudinal variation in blue tit and great tit nest characteristics indicates environmental adjustment. J. Biogeogr. 39, 1669–1677. doi: 10.1111/j.1365-2699.2012.02724.x
Mainwaring, M. C., Hartley, I. R., Lambrechts, M. M., and Deeming, D. C. (2014). The design and function of birds’ nests. Ecol. Evol. 4, 3909–3928.
Mainwaring, M. C., Wolfenden, A., Read, J. E., Robson, J. M., Tomlinson, C. J., and Hartley, I. R. (2016). Feathering the nest: the effects of feather supplementation to Blue Tit nests. Avian Biol. Res. 9, 89–95. doi: 10.3184/175815516x14551240159329
Malzer, I., and Hansell, M. (2017). Nest timing, nest site selection and nest structure in a high latitude population of Bearded Reedlings Panurus biarmicus. Bird Study 64, 51–61. doi: 10.1080/00063657.2016.1271771
Martin, T. E. (2014). Consequences of habitat change and resource selection specialization for population limitation in cavity-nesting birds. J. Appl. Ecol. 52, 475–485. doi: 10.1111/1365-2664.12375
Martin, T. E., and Li, P. (1992). Life history traits of open- vs. cavity-nesting birds. Ecology. 73, 579–592. doi: 10.2307/1940764
Martin, T. E., Boyce, A. J., Fierro-calder, K., Mitchell, A. E., Armstad, C. E., Mouton, J. C., et al. (2017). Enclosed nests may provide greater thermal than nest predation benefits compared with open nests across latitudes. Func. Ecol. 31, 1231–1240. doi: 10.1111/1365-2435.12819
Matysiokov, B., and Remes, V. (2018). Evolution of parental activity at the nest is shaped by the risk of nest predation and ambient temperature across bird species. Evolution 72, 2214–2224. doi: 10.1111/evo.13580
McClintock, M. E., Hepp, G. R., and Kennamer, R. A. (2014). Plasticity of incubation behaviors helps Wood Ducks (Aix sponsa) maintain an optimal thermal environment for developing embryos. Auk 131, 672–680. doi: 10.1642/auk-14-57.1
McGowan, A., Sharp, S. P., and Hatchwell, B. J. (2004). The structure and function of nests of long-tailed tits Aegithalos caudatus. Func. Ecol. 18, 578–583. doi: 10.1111/j.0269-8463.2004.00883.x
Meachen, J. A., Dunn, R. H., and Werdelin, L. (2016). Carnivoran postcranial adaptations and their relationships to climate. Ecography 39, 553–560. doi: 10.1111/ecog.01656
Medina, I. (2019). The role of the environment in the evolution of nest shape in Australian passerines. Sci. Rep. 9:157.
Medina, I., Kilner, R., and Langmore, N. (2020). From micro- to macro-evolution: brood parasitism as a driver of phenotypic diversity in birds. Curr. Zool. 03, 1–12. doi: 10.1007/978-3-319-73138-4_1
Mennerat, A., Mirleau, P., Blondel, J., Perret, P., Lambrechts, M. M., and Heeb, P. (2009). Aromatic plants in nests of the blue tit Cyanistes caeruleus protect chicks from bacteria. Oecologia 161, 849–855. doi: 10.1007/s00442-009-1418-6
Merrill, L., Ospina, E. A., Santymire, R. M., and Benson, T. J. (2019). Egg Incubation Temperature Affects Development of Innate Immune Function in Nestling American Robins (Turdus migratorius). Physiol. Biochem. Zool. 93, 1–12. doi: 10.1086/705361
Møller, A. P. (1990). Nest predation selects for small nest size in the Blackbird. Oikos 57, 237–240. doi: 10.2307/3565945
Møller, A. P. (2005). Rapid change in nest size of a bird related to change in a secondary sexual character. Behav. Ecol. 17, 108–116. doi: 10.1093/beheco/arj003
Møller, A. P., and Nielsen, J. T. (2015). Large increase in nest size linked to climate change: an indicator of life history, senescence and condition. Oecologia 179, 913–921. doi: 10.1007/s00442-015-3385-4
Moreno, J. (2012). Avian nests and nest-building as signals. Avian Biol. Res. 5, 238–251. doi: 10.3184/175815512x13534385822786
Mougeot, F., Benítez-López, A., Casas, F., Garcia, J. T., and Viñuela, J. (2014). A temperature-based monitoring of nest attendance patterns and disturbance effects during incubation by ground-nesting sandgrouse. J. Arid Environ. 102, 89–97. doi: 10.1016/j.jaridenv.2013.11.010
Mouton, J. C., and Martin, T. E. (2019). Nest structure affects auditory and visual detectability, but not predation risk, in a tropical songbird community. Func. Ecol. 33, 1973–1981. doi: 10.1111/1365-2435.13405
Mueller, A. J., Miller, K. D., and Bowers, E. K. (2019). Nest microclimate during incubation affects posthatching development and parental care in wild birds. Sci. Rep. 9, 5161–5161.
Muth, F., and Healy, S. D. (2014). Zebra finches select nest material appropriate for a building task. Anim. Behav. 90, 237–244. doi: 10.1016/j.anbehav.2014.02.008
Oniki, Y. (1979). Is nesting success of birds low in the tropics? Biotropica 11, 60–69. doi: 10.2307/2388174
Ospina, E. A., Merrill, L., and Benson, T. J. (2018). Incubation temperature impacts nestling growth and survival in cup nesting passerine. Ecol. Evol. 8, 3270–3279. doi: 10.1002/ece3.3911
Peluc, S. I., Sillett, T. S., Rotenberry, J. T., and Ghalambor, C. K. (2008). Adaptive phenotypic plasticity in an island songbird exposed to a novel predation risk. Behav. Ecol. 19, 830–835. doi: 10.1093/beheco/arn033
Powell, L. A., and Rangen, K. L. (2000). Nest in Wood Thrush nest construction and dimensions. North Am. Bird Bander 25, 89–96.
Price, J. J., and Griffith, S. C. (2017). Open cup nests evolved from roofed nests in the early passerines. Proc. R. Soc. Lond. B Biol. Sci. 284:20162708. doi: 10.1098/rspb.2016.2708
Prokop, P., and Trnka, A. (2011). Why do grebes cover their nests? Laboratory and field tests of two alternative hypotheses. J. Ethol. 29, 17–22. doi: 10.1007/s10164-010-0214-4
Reddy, S., Driskell, A., Rabosky, D., Hackett, S., and Schulenberg, T. (2012). Diversification and the adaptive radiation of the vangas of Madagascar. Proc. R. Soc. B Biol. Sci. 279, 2062–2071. doi: 10.1098/rspb.2011.2380
Reid, J. M., Monaghan, P., and Ruxton, G. D. (2000). Resource allocation between reproductive phases: the importance of thermal conditions in determining the cost of incubation. Proc. R. Soc. B 267, 37–41. doi: 10.1098/rspb.2000.0963
Rodríguez, S., and Barba, E. (2016). Nestling growth is impaired by heat stress: an experimental study in a mediterranean great tit population. Zool. Stud. 55, 40–40.
Rohwer, V. G., and Law, J. S. (2010). Geographic variation in nests of Yellow Warblers breeding in Churchill, Manitoba, and Elgin, Ontario. Condor 112, 596–604. doi: 10.1525/cond.2010.090229
Ruiz-Castellano, C., Tomás, G., Ruiz-Rodríguez, M., and Soler, J. J. (2018). Nest material preferences by spotless starlings. Behav. Ecol. 29, 137–144. doi: 10.1093/beheco/arx139
Šálek, M. E., and Zárybnická, M. (2015). Different temperature and cooling patterns at the blunt and sharp egg poles reflect the arrangement of eggs in an avian clutch. PLoS One 10:e0117728. doi: 10.1371/journal.pone.0117728
Seymour, R. S., and Bradford, D. F. (1992). Temperature regulation in the incubation mounds of the Australian Brush-turkey. Condor 94, 134–150. doi: 10.2307/1368803
Skowron, C., and Kern, M. D. (1980). The insulation in nests of selected North American songbirds. Auk. 97, 816–824. doi: 10.1093/auk/97.4.816
Slagsvold, T. (1989). On the evolution of clutch size and nest size in passerine birds. Oecologia 79, 300–305. doi: 10.1007/bf00384308
Smith, M. G., Kaiser, S. A., Sillett, T. S., and Webster, M. S. (2018). Variation in nest characteristics and brooding patterns of female Black-throated Blue Warblers is associated with thermal cues. Auk 135, 733–747. doi: 10.1642/auk-17-195.1
Smith, W. K., Roberts, S. W., and Miller, P. C. (1974). Calculating the nocturnal energy expenditure of an incubating Anna’s Hummingbird. Condor 76, 176–183. doi: 10.2307/1366728
Soler, J. J., Møller, A. P., and Soler, M. (1998). Nest building, sexual selection and parental investment. Evolutionary. Ecology 12, 427–441. doi: 10.1023/a:1006520821219
Suárez, F., Morales, M. B., Mínguez, I., and Herranz, J. (2005). Seasonal variation in nest mass and dimensions in an open-cup ground-nesting shrub-steppe passerine: the tawny pipit Anthus campestris. Ardeola 52, 43–51.
Suárez-Rodríguez, M., López-Rull, I., and Macias Garcia, C. (2013). Incorporation of cigarette butts into nests reduces nest ectoparasite load in urban birds: new ingredients for an old recipe? Biol. Lett. 9:20120931. doi: 10.1098/rsbl.2012.0931
Tomás, G., Merino, S., Martínez-de la Puente, J., Moreno, J., Morales, J., and Rivero-de Aguilar, J. (2013). Nest size and aromatic plants in the nest as sexually selected female traits in blue tits. Behav. Ecol. 24, 926–934. doi: 10.1093/beheco/art015
Tortosa, F. S., and Villafuerte, R. (1999). Effect of nest microclimate on effective endothermy in white stork Ciconia ciconia nestlings. Bird Study 46, 336–341.
van Riper, C. (1980). The phenology of the dryland forest of Mauna Kea, Hawaii, and the impact of recent environmental perturbations. Biotropica 12, 282–291. doi: 10.2307/2387700
Veiga, J. P., Polo, V., and Viñuela, J. (2006). Nest green plants as a male status signal and courtship display in the spotless starling. Ethology 112, 196–204. doi: 10.1111/j.1439-0310.2006.01148.x
Wada, H., Kriengwatana, B. P., Steury, T. D., and Macdougall-Shackleton, S. A. (2018). Incubation temperature influences sex ratio and offspring’s body composition in Zebra finches (Taeniopygia guttata). Canad. J. Zool. 96, 1010–1015. doi: 10.1139/cjz-2017-0099
Walsh, P. T., Hansell, M., Borello, W. D., and Healy, S. D. (2010). Repeatability of nest morphology in African weaver birds. Biol. Lett. 6, 149–151. doi: 10.1098/rsbl.2009.0664
Walsh, P. T., Hansell, M., Borello, W. D., and Healy, S. D. (2011). Individuality in nest building: do southern masked weaver (Ploceus velatus) males vary in their nest-building behaviour? Behav. Proces. 88, 1–6. doi: 10.1016/j.beproc.2011.06.011
Walters, L. A., Webber, J. A., Jones, B. A., and Volker, C. L. (2016). Taking a break: the relationship between ambient temperature and nest attendance patterns of incubating carolina chickadees (Poecile carolinensis). Wilson J. Ornithol. 128, 719–726. doi: 10.1676/15-115.1
Wang, Y., Chen, S., Blair, R. B., Jiang, P., and Ding, P. (2009). Nest composition adjustments by Chinese Bulbuls Pycnonotus sinensis in an urbanized landscape of Hangzhou (E China). Acta Ornithol. 44, 185–192. doi: 10.3161/000164509x482768
Windsor, R. L., Fegely, J. L., and Ardia, D. R. (2013). The effects of nest size and insulation on thermal properties of tree swallow nests. J. Avian Biol. 44, 305–310. doi: 10.1111/j.1600-048x.2013.05768.x
Winkler, D. W., and Sheldon, F. H. (1993). Evolution of nest construction in swallows (Hirundinidae): a molecular phylogenetic perspective. Proc. Natl. Acad. Sci. 90, 5705–5707. doi: 10.1073/pnas.90.12.5705
Keywords: nest microclimate, environmental condition, phenotipic plasticity, extended phenotype, evolution
Citation: Perez DM, Gardner JL and Medina I (2020) Climate as an Evolutionary Driver of Nest Morphology in Birds: A Review. Front. Ecol. Evol. 8:566018. doi: 10.3389/fevo.2020.566018
Received: 26 May 2020; Accepted: 10 November 2020;
Published: 03 December 2020.
Edited by:
Stuart Peter Sharp, Lancaster University, United KingdomReviewed by:
James Mouton, Smithsonian Migratory Bird Center, United StatesMark C. Mainwaring, University of Montana, United States
Copyright © 2020 Perez, Gardner and Medina. This is an open-access article distributed under the terms of the Creative Commons Attribution License (CC BY). The use, distribution or reproduction in other forums is permitted, provided the original author(s) and the copyright owner(s) are credited and that the original publication in this journal is cited, in accordance with accepted academic practice. No use, distribution or reproduction is permitted which does not comply with these terms.
*Correspondence: Daniela M. Perez, ZGFuaS5tYWxnYXJpbmlAZ21haWwuY29t