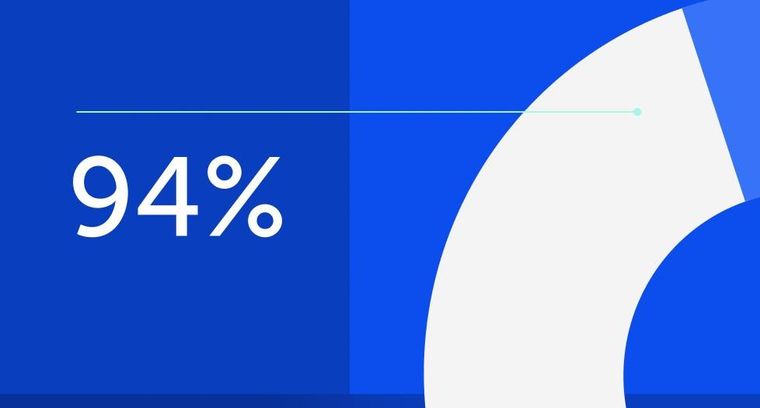
94% of researchers rate our articles as excellent or good
Learn more about the work of our research integrity team to safeguard the quality of each article we publish.
Find out more
ORIGINAL RESEARCH article
Front. Ecol. Evol., 24 September 2020
Sec. Biogeography and Macroecology
Volume 8 - 2020 | https://doi.org/10.3389/fevo.2020.565708
This article is part of the Research TopicConsequences of Climate Change for Plant Biodiversity in High Mountain EcosystemsView all 18 articles
Some of the largest impacts of climate change are expected in the environmentally heterogeneous and species rich high mountain ecosystems. Among those, the Neotropical alpine grassland above the tree line (c. 2,800 m), known as Páramo, is the fastest evolving biodiversity hotspot on earth, and one of the most threatened. Yet, predicting climate responses of typically slow-growing, long-lived plant linages in this unique high mountain ecosystem remains challenging. Here we coupled climate sensitivity modeling and adaptive potential inferences to efficiently assess climate vulnerability of Espeletia, Páramo’s most iconic, predominant and rapidly evolving plant complex. In order to estimate climate sensitivity, we first modeled the distribution of 28 Espeletia taxa under a niche conservatism scenario using altitude and five current (1970–2000) and future (2050 RCP 8.5) bioclimatic variables across 36 different Páramo complexes in the northern Andes (49% of the world’s Páramo area). As an alternative to range shifts via migration, we also computed the adaptive capacity of these Páramo complexes by considering three enhancing factors of the biodiversity’s adaptive potential as well as three environmental limiting factors of the populations’ plastic response. These predictors showed that diverse Páramos in the Eastern Cordillera were more vulnerable likely because the counteracting effects of the adaptive potential (r = −0.93 ± 0.01) were not sufficient to buffer higher distribution losses (r = 0.39 ± 0.01). Agriculture (r = −0.48 ± 0.01), mining (r = −0.36 ± 0.01), and rural population density (r = −0.23 ± 0.01) also weakened the adaptive capacity. These results speak for a limited persistence via migration in the short-term responses of Espeletia to climate change, even though the past population dynamics in concert with glacial cycling is indicative of a predominant role of range shifts. Furthermore, changing climate, together with a general inability to adapt, may eventually constrain the rapid diversification in the Espeletia complex. Our integrative modeling illustrates how future climate may impact plant populations in a mega diverse and highly threatened ecosystem such as the Páramo, and encourages carrying out similar estimates in diverse plant complexes across other high mountain and island-like ecosystems.
How tropical alpine biodiversity hotspots are affected and will react to climate change is among the most pressing questions in current biological research. Most studies on responses to changing climate assume populations migration to higher altitudes so that organisms would track their ecological niche (Lenoir et al., 2008; Steinbauer et al., 2018), and in some cases even to lower altitudes due to competitive release (Lenoir et al., 2010). Yet, when migration potential is restricted (Jump and Penuelas, 2005; Lees et al., 2020), the only way left for organisms to persist is by adjusting to the new environmental conditions, a traditionally neglected alternative in niche modeling. Adjustment via phenotypic plasticity is presumably important in long-lived taxa because plastic responses can happen within the lifetime of an individual (Nicotra et al., 2010). However, plasticity may be hampered when populations face novel anthropic conditions not found in their evolutionary history (Fox et al., 2019; Kelly, 2019), e.g., agriculture, mining and population density. Organisms can also adjust to climate change through adaptation (Waldvogel et al., 2020). Still, populations may not have enough adaptive potential (e.g. limited diversity) to keep up with the pace of climate change (Hoffmann and Sgro, 2011). Therefore, assessing biodiversity hotspots’ vulnerability to climate change not only requires tracing ecological niche gaps under future scenarios – i.e., sensitivity (Berry et al., 2003), but also needs to explicitly account for the adaptive potential (Razgour et al., 2019) and plasticity to anthropic pressures. These improvements may make predictions more congruent with empirical data, even in potential climate refugia (Sweet et al., 2019).
One of the fastest evolving biodiversity hotspots is the Páramo (Madriñán et al., 2013), a highly threated (Vásquez et al., 2015; Pérez-Escobar et al., 2018) alpine ecosystem dominated by diverse grasslands above the treeline in the American tropics (at elevations of ca. 2,800–5,000 m). It is a main water supplier of wetland ecosystems and densely populated areas in the northern Andes (Cuesta et al., 2019a; Llambí et al., 2020). Despite it has a relatively small surface area (35,000 km2), it contains over 3,000 plant species, several of which are endemic (Luteyn, 1999; Hughes and Atchison, 2015) and emerged as unique adaptations to extreme environments that evolved during the Andean uplift in the last five million years (Antonelli et al., 2009; Madriñán et al., 2013; Mutke et al., 2014). Unparalleled diversification rates across plant groups at these tropical “sky islands” (Sklenáø et al., 2014) has further been attributed to colonization by pre-adapted linages (Muellner-Riehl, 2019), and Pleistocene glacial cycling (Nevado et al., 2018; Perrigo et al., 2019; Rahbek et al., 2019) in the last 2.4 Myr that led to repeated periods of connectivity and spatial isolation (i.e., “species pump hypothesis”). Population fluctuations in concert with past glacial cycling indicate a general inability to adapt and a major role of range shifts (Martín-Bravo et al., 2010; Ronikier, 2011; Hazzi et al., 2018; Muellner-Riehl, 2019). Yet, it remains to be explored whether the rapidly evolving Páramo can keep pace with the quick rate of climate change and human expansion.
The most iconic, abundant and diverse group in the Páramo is the Espeletia complex (Monasterio and Sarmiento, 1991; Madriñán et al., 2013; Mavárez, 2019b). It originated in the Venezuelan Andes (Pouchon et al., 2018) from where it colonized southwards the Colombian Eastern Cordillera and the Ecuadorian Andes (Mavárez, 2019b), followed by a northwards migration into the Colombian Central and Western Cordilleras (Cuatrecasas, 2013). Espeletia taxa (ca. 120) are ecologically abundant across the Páramo landscape (Luteyn, 1999), occurring in highly heterogeneous habitats. Espeletia populations are adapted to grow in the wet depressions of high valleys, in the dry exposed slopes or even within the forests at the tree line, therefore experiencing a wide range of climatic conditions within elevations and localities (Peyre et al., 2018). This environmental heterogeneity may have important implications for the reaction of Espeletia to changing climatic conditions. For example, habitat variation may provide suitable locations for migrants within only a few meters of their current locations (Scherrer and Körner, 2011). However, populations adapted to a narrow range of conditions may respond poorly to future threats. In other words, environmental heterogeneity effects on the responses to climate change may be mixed, and sometime contradictory, especially for a long living tropical alpine plant with limited seed dispersal, such as Espeletia. Hence, a much-needed research is to balance climate sensitivity under the current ecological niche heterogeneity with the adaptive capacity of Espeletia to assess the climate vulnerability of this plant complex.
In order to bridge this gap, our goals in this study were to (1) quantify climate sensitivity in the Espeletia complex by comparing current and future (2050 RCP 8.5) distributions under a niche conservatism scenario (i.e., range shift via migration), (2) compute the adaptive capacity by incorporating enhancing factors of the biodiversity’s adaptive potential (i.e., diversity, protected areas, and forest area in the buffer zone of the Páramo) as well as limiting factors of the plastic response (i.e., agricultural area, mining, and population density), and (3) estimate climate vulnerability of Espeletia by simultaneously coupling its climate sensitivity with its adaptive capacity. This work will help establishing how plant populations may respond to environmental change in a mega diverse tropical alpine region, where the largest impacts of climate pressures are expected (Körner, 2003; Rumpf et al., 2018).
The study area comprised the Colombian Andean Páramo biogeographical province (2,732–5,212 m, Figure 1), including the high-elevation northern Andes and the Sierra Nevada de Santa Marta, but excluding areas with similar altitudes in the Cordillera de Merida and Central American mountains (Figure 2). It was delimited in the north by the Sierra Nevada of Santa Marta (11°N), and in the south by the Colombia-Ecuador border (1 N). It spanned 36 Páramo complexes, as in Morales et al. (2007) and Londoño et al. (2014), denoting 49% of the world’s Páramos. The surveyed Páramo zones were covered in a 33% by protected areas1, and surrounded in a 30% by forest patches2. Meanwhile, agriculture and mining, respectively, disturbed 6 and 7%3 of these Páramos and their buffer belt (2 km around the tree line). The handling of these data sources is detailed below as part of the computation of the “Adaptive Capacity at the Study Area.”
Figure 1. Altitudinal profile (m asl) of 28 Espeletia taxa across Páramo sky islands in the Colombian Andes. A total of 1,432 occurrences, originally gathered as georeferenced specimen data from GBIF, were retained after cleaning for georeferencing consistency (i.e., non-redundant records located within the study area) and number of records per species (to avoid distributions with less than 10 points, following Van Proosdij et al. (2016) for narrow-ranged species). Espeletia taxonomy is in accordance with Cuatrecasas (2013).
Figure 2. Climate sensitivity estimates of Espeletia across 36 Páramo complexes in the Colombian Andes. Climate sensitivity was modeled by comparing (A) current (baseline period 1970–2000) and (B) future (2050 RCP 8.5) average distribution probabilities across 28 taxa, as obtained with Maxent v.3.4.1 (Phillips et al., 2017) under a niche conservatism scenario (i.e., range shift via migration). Values close to zero in the sensitivity index (C) indicate few changes in the forecasted distribution of Espeletia due to climate change, while negative and positive values correspond to range losses and gains, respectively, based on the averaged presence probabilities. Five non-collinear WorldClim bioclimatic variables (Supplementary Table S3) were considered at a 30 s (1 km2) spatial resolution for the current and future scenarios, besides a Digital Elevation Model (DEM). Espeletia taxonomy follows Cuatrecasas (2013). Páramo complexes are numbered in (A) according to Morales et al. (2007) and Londoño et al. (2014), specifically: (1) Perijá, (2) Jurisdicciones-Santurbán, (3) Tamá, (4) Almorzadero, (5) Yariguíes, (6) Cocuy, (7) Pisba, (8) Tota-Bijagual-Mamapacha, (9) Guantiva-Rusia, (10) Iguaque-Merchán, (11) Guerrero, (12) Rabanal y río Bogotá, (13) Chingaza, (14) Cruz Verde-Sumapaz, (15) Los Picachos, (16) Miraflores, (17) Belmira, (18) Nevados, (19) Chilí-Barragán, (20) Las Hermosas, (21) Nevado del Huila-Moras, (22) Guanacas-Puracé-Coconuco, (23) Sotará, (24) Doña Juana-Chimayoy, (25) La Cocha-Patascoy, (26) Chiles-Cumbal, (27) Paramillo, (28) Frontino-Urrao, (29) Citará, (30) Tatamá, (31) Duende, (32) Farallones de Cali, (33) Cerro Plateado, (34) Santa Marta, (35) Sonsón, and (36) Altiplano Cundiboyacense.
Climate sensitivity in the Espeletia complex was modeled by comparing current and future (2050 RCP 8.5) distributions under a niche conservatism scenario (i.e., range shift via migration). Occurrences of Espeletia samples for the 36 Colombian Páramo complexes were downloaded as georeferenced specimen data from providers served by GBIF4. From an initial set of 15,466 specimen records downloaded, a total of 1,432 (Supplementary Table S1), comprising 28 different Espeletia taxa according to Cuatrecasas (2013) – Supplementary Table S2, were retained after filtering for georeferencing consistency (i.e., non-redundant records within the study area) and number of records. In order to avoid bias due to distributions with low number of input points (Wisz et al., 2008), specially for presumably rare taxa (Fois et al., 2018), only putative species with at least 10 original records were retained (three taxa with more than 100 records, Supplementary Figure S1A). This threshold follows Van Proosdij et al. (2016) recommendation for narrow-ranged species, that is those with a moderate prevalence class (i.e., 27 taxa were present in less than seven different Páramo complexes, and only one taxon was present in 20 Páramos, Supplementary Figure S1B). This latter trend is expected in Espeletia species, for which distance (Diazgranados and Barber, 2017; Padilla-González et al., 2017) and ecology (Cortés et al., 2018a) are the major speciation drivers.
Historical climate data was extracted from the WorldClim5 database, at a 30 s resolution, using the georeferencing of each record. All 19 bioclimatic variables were downloaded in the baseline period 1970–2000. The same variables projected to 2050, under the IPCC RCP 8.5 scenario, were gathered using CCAFS’s downscaled delta method (Navarro-Racines et al., 2020). This projection averages 33 different global climatic models at a 1 km2 resolution. A Digital Elevation Model (DEM) variable was further included. Prioritization of environmental variables (Zeng et al., 2016) is crucial to enhance model complexity (Warren and Seifert, 2011) for climate sensitivity assessments. By improving variable selection (Cobos et al., 2019), collinearity (Feng et al., 2019) and sampling bias (Syfert et al., 2013) can be minimized. Collinearity in particular refers to a strong correlation between two or more predictor variables, and may cause instability in parameter estimation (Dormann et al., 2013). A measure to diagnose collinearity is the Variance Inflation Factor (VIF), the square of the multiple correlation coefficient resulting from regressing a predictor variable against all other predictor variables (Chatterjee and Hadi, 2006). Hence, we performed variable selection by computing the VIF score using the usdm (Uncertainty Analysis for Species Distribution Models) package (Naimi et al., 2014) in R v.3.3.1 (R Core Team), and drew Pairwise Pearson correlations (r) among all variables by means of the same software (Supplementary Figure S2). Five bioclimatic variables and DEM did not exhibit collinearity (Naimi et al., 2014) at VIF < 10 (Supplementary Table S3), with absolute r-values below 0.7 from 0.05 to 0.65, and therefore were retained hereinafter.
The six non-collinear variables fed Maxent model v.3.4.1. (Phillips et al., 2017), a “machine learning” outline that uses maximum entropy and Bayesian inference to estimate occurrence probability distributions. Ten split-sample models were run, after checking for stabilization in the ROC curves (Spiers et al., 2018), with an average of 10 k pseudo-absences for each of the 28 putative taxa. Each replicated run type was set to “cross-validate” with 500 iterations (Buffum et al., 2015). Presence ≥ 95% was binary reclassified (Liu et al., 2005) and the average across repetitions was recorded in each case. This procedure was performed for the historical dataset (1970–2000). To predict distribution changes of Espeletia, we projected trained models on current presence localities and present background climate to 2050 under the RCP 8.5 scenario through Maxent’s “projection layer” tool. Current model validation considered AUC, an aggregate measure of performance across all possible classification thresholds. Minimum average testing AUC was above 0.98 (Supplementary Table S4). As AUC alone (Supplementary Table S5) may be deceiving (Lobo et al., 2008; West et al., 2016), the AIC and BIC criteria were also computed and checked for consistency (Supplementary Table S6).
All 56 distribution maps were converted to binary data, using a threshold of the 20th percentile, as suggested by the Maxent v.3.4.1 software (Phillips et al., 2017). These maps were then averaged to generate spatial information of current and future presence probabilities across all 28 taxa. Finally, the current distribution map was subtracted to the one obtained under a climate change scenario in order to estimate climate sensitivity in the Espeletia complex. Values close to zero in the sensitivity index meant few changes in the forecasted distribution of Espeletia due to climatic variation. On the other hand, negative and positive values corresponded to areas where the presence probabilities were, respectively, diminished or boosted by climate change.
We computed the adaptive capacity as the scaled additive effects of six main variables that expressed the capacity of the overall Espeletia biodiversity to respond without migration to the effects of climate change. Enhancing factors of the biodiversity’s adaptive potential were diversity, protected areas, and forest area around the Páramo. On the contrary, limiting factors of the plastic response were agriculture, mining, and population density.
Diversity may buffer the effects of climate change by enriching complex interactions, such as adaptive inter-specific introgression (Marques et al., 2019) and hybrid speciation (Coyne and Orr, 2004; Abbott et al., 2013; Payseur and Rieseberg, 2016). Hybridization may allow for local adaptation in transitional environments and bi-directional transfer of adaptive variation through introgression (Isabel et al., 2020). Espeletia in particular exhibits weak species boundaries, rampant gene-flow and reticulate evolution (Cortés et al., 2018a; Pouchon et al., 2018), leading to a high incidence of natural hybrids (Rauscher, 2002; Cuatrecasas, 2013). In order to account for the enhancing role of diversity on the adaptive potential, the number of Espeletia taxa was totalized in a 1 km grid using the occurrence data downloaded from GBIF (see footnote), as described in the previous section, and scaled from 0 to 1.
Another enhancing factor of the biodiversity’s adaptive potential is protected areas, where human intervention does not represent a direct threat, allowing biodiversity to be conserved while naturally coping with climate change. Protected areas selected for this analysis spanned 1,050 natural areas, according to the System of National Natural Parks (see footnote), and included 43 National Natural Parks, 663 Civil Society Reserves, 54 Regional Natural Parks, and 57 National Protected Forest Reserves. These categories were, respectively, rated as 1, 0.3, 0.1, and 0.1, following CIAT (2017). Higher ratings were assigned to areas that presumably provide greater protection for biodiversity due to their low anthropogenic pressure and extent.
Forest patches around the Páramo complexes (Henao-Díaz, 2019) may also contribute buffering climate change effects by facilitating (Bueno and Llambí, 2015) migration and gene-flow, which could increase the frequency of existing genetic variants adapted to particular conditions (Bridle and Vines, 2007). Additionally, forests provide conditions that may favor thermal and water regulation, decreasing the magnitude of climate change itself. Therefore, forest area in the buffer zone of the Páramo (2 km around the tree line) was gathered from the IDEAM (see footnote), and converted to binary points (1 = presence) in a 1 km grid.
On the other hand, major limiting factors of the plastic response are agriculture (Avellaneda-Torres et al., 2020), mining (Pérez-Escobar et al., 2018) and population density within the study area and in its buffer zone (Vásquez et al., 2015). In order to account for these threats, agricultural and mining areas were downloaded from the Geographic Institute Agustín Codazzi (see footnote) for the period 2010–2012, and transformed to binary data (0 = presence) in a 1 km grid within the study area and in its buffer zone (2 km around the tree line). The population density indicator was computed as the inverse value of the normalized rural population density, scaled from 0 to 1, so that 1 meant absence of human settlements and 0 was the population density at the most populated rural zones in the study area. The population information was gathered from data projected to 2015 by the National Administrative Department of Statistics6.
In order to estimate climate vulnerability at the overall Espeletia complex, we simultaneously coupled its climate sensitivity with its adaptive capacity. Negative values of the sensitivity index meant areas where the presence probabilities of Espeletia were diminished due to climate change. Meanwhile, lower adaptive capacity scores spoke for a prominent role of limiting factors of the plastic response (i.e., agriculture, mining, and population density) as compared to enhancing drivers of the biodiversity’s adaptive potential (i.e., diversity, protected areas, and forest area around the Páramo). Therefore, the climate vulnerability score was computed as the complement value of the additive contributions of the sensitivity and the adaptive capacity indices. The climate vulnerability score was scaled from 0 to 1 for comparative purposes. Pairwise Pearson correlations (r) among all estimated scores were computed, and boxplots and ridgeline plots were drawn for the three indices in all 36 Páramo complexes. Computing and plotting were done in R v.3.3.1 (R Core Team).
Climate sensitivity was estimated across all 36 Colombian Páramo complexes by comparing current (baseline period 1970–2000, Figure 2A) and future (2050 RCP 8.5, Figure 2B) average distribution probabilities of 28 different Espeletia taxa (nine had average sensitivity scores below zero). Values surrounding zero in the sensitivity index (−0.029 to 0.030, 42% of the study area, Figure 2C) stood for few changes in the forecasted distribution of Espeletia due to climate change, and were predominant in the north of the Eastern Cordillera, the Cruz Verde-Sumapaz Páramo complex south of the Bogotá montane savanna (the southwestern wetland complex of the larger Eastern Cordillera’s Andean highland plateau), and the small sky islands of the Western Cordillera.
Given a niche conservatism scenario, negative estimates of the climate sensitivity (≤−0.030, 32% of the study area, Figure 2C) predicted range losses based on the averaged presence probabilities. Páramo areas more likely to experience range losses were those in the Sierra Nevada de Santa Marta, and the Central Cordillera South of Las Hermosas (i.e., Nevado del Huila-Moras, and Guanacas-Puracé-Coconuco), as well as those in the northern corner of the Eastern Cordillera (i.e., Sierra Nevada del Cocuy, Pisba, and Tota-Bijagual-Mamapacha), where diverse summits surrounded the topographically intricate Chichamocha inter-Andean canyon. On the other hand, range shifts via migration enriched presence probabilities in other localities (positive estimates of the climate sensitivity ≥ 0.031, 27% of the study area, Figure 2C). Range gains were only predicted for Páramo areas in the Central Cordillera (Los Nevados, Chilí-Barragán, Las Hermosas, and La Cocha-Patascoy, Supplementary Figure S3) where the dominant taxon was E. hartwegiana, as well as in the summits of the northern tip of the Eastern Cordillera (Perijá).
The adaptive capacity across 36 Colombian Páramo complexes was computed using a scaled additive model of enhancing (Figures 3A–C) and limiting (Figures 3D–F) factors of the biodiversity’s adaptive potential and the plastic response. The adaptive capacity index (Figures 4B, 5A, Supplementary Figure S3A, and Supplementary Table S7) was higher (≥0.72, 34% of the study area) in species-rich Páramo areas (Pearson’s r = 0.19 ± 0.01, Figure 3A and Supplementary Figure S4), and matched extensive National Natural Parks (r = 0.59 ± 0.01, Figure 3B and Supplementary Figure S4), like the Eastern Cordillera’s Sierra Nevada del Cocuy, Chingaza and Sumapaz. The Páramo adaptive capacity index was also moderately superior (0.62–0.71, 29% of the study area, Figure 4B) in the most representative National Natural Parks in the Central Cordillera (Los Nevados and Nevado del Huila) as well as in the Sierra Nevada de Santa Marta. In turn, the western slopes of the Páramo complexes at the Eastern and Central Cordilleras exhibited lower adaptive capacity values (≤0.61, 37% of the study area), and were enclosed by less forest patches (30% on average, Figure 3C, r = 0.39 ± 0.01, Supplementary Figure S4).
Figure 3. Components of the adaptive capacity across 36 Páramo complexes in the Colombian Andes. Enhancing (A–C) and limiting (D–F) factors of the adaptive capacity are shown in green and red, respectively. Enhancing factors of the biodiversity’s adaptive potential were (A) diversity, (B) protected areas, and (C) forest area around the Páramo, while limiting factors of the plastic response were (D) agriculture, (E) mining and (F) population density. Diversity (A), which is the number of Espeletia taxa totalized in a 1 km grid based on the occurrence data downloaded from GBIF, and the inverse value of the normalized rural population density (F) were scaled from 0 to 1. A total of 1,050 protected areas (B) were rated as 1, 0.3, 0.1, and 0.1 for National Natural Parks, Civil Society Reserves, Regional Natural Parks and National Protected Forest Reserves, respectively, following (CIAT, 2017). Forest patches (C) around the Páramo complexes (2 km around the tree line, 1 km grid) were recorded as presence points (1 = presence), while agriculture (D) and mining (E) for the period 2010–2012 were marked as null data points (0 = presence) within the study area and in its buffer zone (2 km around the tree line, 1 km grid). Páramo complexes numbers in (A) follow Morales et al. (2007) and Londoño et al. (2014): (1) Perijá, (2) Jurisdicciones-Santurbán, (3) Tamá, (4) Almorzadero, (5) Yariguíes, (6) Cocuy, (7) Pisba, (8) Tota-Bijagual-Mamapacha, (9) Guantiva-Rusia, (10) Iguaque-Merchán, (11) Guerrero, (12) Rabanal y río Bogotá, (13) Chingaza, (14) Cruz Verde-Sumapaz, (15) Los Picachos, (16) Miraflores, (17) Belmira, (18) Nevados, (19) Chilí-Barragán, (20) Las Hermosas, (21) Nevado del Huila-Moras, (22) Guanacas-Puracé-Coconuco, (23) Sotará, (24) Doña Juana-Chimayoy, (25) La Cocha-Patascoy, (26) Chiles-Cumbal, (27) Paramillo, (28) Frontino-Urrao, (29) Citará, (30) Tatamá, (31) Duende, (32) Farallones de Cali, (33) Cerro Plateado, (34) Santa Marta, (35) Sonsón, and (36) Altiplano Cundiboyacense.
Figure 4. Climate sensitivity, adaptive capacity and climate vulnerability in the Espeletia complex across 36 Páramo sky island complexes in the Colombian Andes. Climate sensitivity (A) is already depicted in Figure 2C and is brought here for comparative purposes. Adaptive capacity (B) was computed as the scaled additive effects of all six variables shown in Figure 3. Climate vulnerability (C) was calculated as the complement value of the additive contributions of the sensitivity and the adaptive capacity indices, scaled from 0 to 1. Negative values in the sensitivity index (A) suggest range losses in the presence probabilities of Espeletia due to climate change. Low values in the adaptive capacity score (B) indicate a major role of limiting factors of the plastic response (i.e., agriculture, mining, and population density, Figures 3D–F) as compared to enhancing drivers of the biodiversity’s adaptive potential (i.e., diversity, protected areas, and forest area around the Páramo, Figures 3A–C). Páramo complexes are numbered in (A) following Morales et al. (2007) and Londoño et al. (2014), that is: (1) Perijá, (2) Jurisdicciones-Santurbán, (3) Tamá, (4) Almorzadero, (5) Yariguíes, (6) Cocuy, (7) Pisba, (8) Tota-Bijagual-Mamapacha, (9) Guantiva-Rusia, (10) Iguaque-Merchán, (11) Guerrero, (12) Rabanal y río Bogotá, (13) Chingaza, (14) Cruz Verde-Sumapaz, (15) Los Picachos, (16) Miraflores, (17) Belmira, (18) Nevados, (19) Chilí-Barragán, (20) Las Hermosas, (21) Nevado del Huila-Moras, (22) Guanacas-Puracé-Coconuco, (23) Sotará, (24) Doña Juana-Chimayoy, (25) La Cocha-Patascoy, (26) Chiles-Cumbal, (27) Paramillo, (28) Frontino-Urrao, (29) Citará, (30) Tatamá, (31) Duende, (32) Farallones de Cali, (33) Cerro Plateado, (34) Santa Marta, (35) Sonsón, and (36) Altiplano Cundiboyacense.
Figure 5. Boxplots for the adaptive capacity (A), climate sensitivity (B), and vulnerability (C) across 36 Páramo complexes in the Colombian Andes. For comparative purposes, Páramo complexes are sorted in (A) from those with a low adaptive potential to anthropic pressures according to the enhancing and limiting factors depicted in Figure 3, in (B) from those likely to experience a range loss (distributions below zero) to those predicted to exhibit a range gain (distributions above zero) by 2050 (under RCP 8.5), while in (C) Páramo complexes at the top are the most vulnerable. Boxplots summarize grid estimates depicted in Figure 4.
However, positive effects due to protected areas and forest coverage on the adaptive potential were offset by limiting factors of the plastic response, primarily agricultural (6% of the study area and its buffer zone, Figure 3D, r = −0.47 ± 0.01, Supplementary Figure S4) and mining (7% of the study area and its buffer zone, Figure 3E, r = −0.41 ± 0.01, Supplementary Figure S4) pressures, mainly in the western slopes of the Páramo areas at the Eastern and Central Cordilleras. This let to the lowest adaptive potential estimates (≤0.52, 20% of the study area, Figure 4B) at the western slopes of the Páramo complexes in the Cauca province, in the Central Cordillera, and the Boyacá and Santander provinces (i.e., Almorzadero and Altiplano Cundiboyacense Páramo complexes), in the Eastern Cordillera. Rural population (Figure 3F) hampered the adaptive potential in all three Cordilleras and Páramo areas (r = −0.28 ± 0.01, Supplementary Figure S4).
The scaled complement of the additive contributions of the sensitivity (Figures 2C, 5B and Supplementary Figure S3B) and the adaptive capacity (Figure 4B) indices were used to compute the climate vulnerability score (Figures 4C, 5C and Supplementary Figure S3C). The most vulnerable (≥0.57, 11% of the study area, Figure 4C) Páramo areas coincided with diverse Páramos in the Eastern Cordillera (Figure 3A) because of more distribution losses (r = 0.39 ± 0.01, Supplementary Figure S4). Agriculture (r = 0.48 ± 0.01, Supplementary Figure S4), mining (r = 0.36 ± 0.01, Supplementary Figure S4) and rural population density (r = 0.23 ± 0.01, Supplementary Figure S4) also boosted their vulnerability, mainly on the western slopes in the middle of the Central Cordillera (i.e., Guanacas-Puracé-Coconuco), and the northeast corner of the Eastern Cordillera (i.e., Almorzadero, Pisba, and Altiplano Cundiboyacense). On the other hand, the less vulnerable (≤0.38, 57% of the study area, Figure 4C) Páramo areas were those with the highest adaptive capacity (≥ 0.72, Figure 4B, r = −0.93 ± 0.01, Supplementary Figure S4) due to large National Natural Parks (Sumapaz, Chingaza, Sierra Nevada del Cocuy, Figure 3B), as well as those for which predicted range shifts were neutral (−0.029 to 0.030, Figure 4A, i.e., the Cruz Verde-Sumapaz Páramo complexes in the Eastern Cordillera) or positive (≥0.031, Figure 5B, Los Nevados, Chilí-Barragán, and Las Hermosas in Central Cordillera).
The climate sensitivity score of Espeletia to climate change indicated range losses (negative values) widespread across most Páramo complexes. This suggests limited persistence of the Espeletia spp. populations via migration in the majority of Páramos, with some exceptions in the Central Cordillera possibly due to its higher connectivity. An alternative for populations to persist despite changing environments would be adaptation s.l. However, positive effects on the adaptive potential, like those due to protected areas and forests, are likely to be offset by limiting factors of the plastic response, such as rising agriculture and mining in the Páramo areas and their surroundings. Overall, this suggests that diverse Páramo areas of the Eastern Cordillera are the most vulnerable to changing climate. Climate change pushing Espeletia toward mountain summits where there is nowhere else to go (Cuesta et al., 2019a), together with a general inability of those populations to adapt, might constrain the rapid diversification that has made the Espeletia complex so unique.
Widespread range losses in the distribution of Espeletia are expected by 2050, a pessimistic prediction in line with previous modeling efforts (Buytaert et al., 2011; Crandall et al., 2013; Mavárez et al., 2018; Helmer et al., 2019; Peyre et al., 2020; Young and Duchicela, 2020). This tendency is broadly defined as thermophilization, a progressive decline of cold mountain habitats and their biota (Gottfried et al., 2012). Large reductions in modeled area and important upward shifts in the distribution of 28 Espeletiinae are already predicted by 2070 at the Venezuelan Páramo (Mavárez et al., 2018). Despite our projection is carried out for a shorter time frame at another, wider, study area (Colombian Andes spanning the Eastern, Central and Western Cordilleras besides Sierra Nevada de Santa Marta), overall results point toward the same trend and are complementary. Similar predictions of climate sensitivity at the Páramo complexes of the Ecuadorian Cordilleras (1 sp.) were not considered as part of this study because of the difficulties in compiling and comparing data from different national repositories, specifically for some of the variables that compose the adaptive capacity score (i.e., agriculture and mining pressures). Yet, considering analogous approaches in the Ecuadorian Páramo (Crandall et al., 2013; Helmer et al., 2019; Peyre et al., 2020) is essential to gather a more comprehensive understating on the consequences of climate change at high Andean ecosystems north of the Huancabamba depression, a major biogeographical barrier for high-elevation plant taxa (Weigend, 2002).
There are two important exceptions for the widespread range losses in the distribution of Espeletia in the Colombian Andes. First, small and fragmented Páramo sky islands in the Western Cordillera exhibit neutral climate sensitivity, a counterintuitive projection that Mavárez et al. (2018) also pointed out for Espeletiinae in isolated Páramo areas at Cordillera de Mérida. This reiterative finding suggests that scarce topographical complexity (terrain ruggedness) in recently colonized small islands (Flantua et al., 2019) may have favored habitat generalists, while intricate local-scale environmental heterogeneity at larger Páramo complexes could have triggered an explosive radiation (Cortés et al., 2018a; Naciri and Linder, 2020) of habitat specialists, each with a narrow ecological niche for migration to occur (Cuesta et al., 2019b). Modern colonization and limited topographical complexity, together with higher connectivity, may also account for the second exception, which is that range gains are only predicted for Páramo areas in the Central Cordillera (Los Nevados, Chilí-Barragán, Las Hermosas, and La Cocha-Patascoy), where the dominant taxon is E. hartwegiana. In line with this, Páramo areas more likely to exhibit range losses are those in the topographically complex Eastern Cordillera’s northeast corner, where diverse (Luteyn, 1999; Cuatrecasas, 2013; Peyre et al., 2015,2019) summits surround the deep (1,100 m) Chichamocha inter-Andean canyon.
Range shifts presuppose niche conservatism, but also depend on the intrinsic dispersal ability of the taxa (Pelayo et al., 2019; Tovar et al., 2020). In organisms with limited seed dispersal such as Espeletia, other ways for migration are key (Cochrane et al., 2019). For instance, migration via sporadic pollen flow would not be as constrained as seed dispersal (Cuatrecasas, 2013). Alternately, hybrids with intermediate niche requirements (Rieseberg, 2003) may serve as bridges for migration in a plant complex with rampant natural introgression (Rauscher, 2002; Cortés et al., 2018a; Pouchon et al., 2018). Finally, sporadic episodes of habitat connectivity (Flantua et al., 2019) in the lifespan of a long-lived organism such as Espeletia (Cuatrecasas, 2013) could provide a window for migration across modern barriers.
Key traits may relax the niche conservatism assumption by conferring the ability to colonize new habitats. For instance, underground plant-soil interactions (Goh et al., 2013; Sedlacek et al., 2014; Little et al., 2016) across different water levels (Geange et al., 2017) and cellular-anatomical physiological adaptations (Sandoval et al., 2019) may serve as pre-adaptations for a wider range expansion (Cuesta et al., 2017). Plastic traits may also broad the spectrum of novel climates (Arnold et al., 2019). Coupling this modeling with eco-physiological studies in Espeletia (Leon-Garcia and Lasso, 2019; Llambí and Rada, 2019) is hence a key research line to better track future range shifts in the Páramo.
The fact that diverse areas in the Eastern Cordillera are vulnerable to climate change is not only counterintuitive, but it also means that the long-term rapid diversification rate, striking in the Espeletia complex, could be jeopardized. Diversity is regarded as a buffer of the effects of climate change by enriching interactions (Cáceres et al., 2014), such as facilitation (Bueno and Llambí, 2015; Wheeler et al., 2015; Llambí et al., 2018; Mora et al., 2018; Venn et al., 2019), adaptive inter-specific introgression (Schilthuizen et al., 2004; Seehausen, 2004) and hybrid speciation (Payseur and Rieseberg, 2016), of which there is abundant evidence in Espeletia (Rauscher, 2002; Cuatrecasas, 2013; Cortés et al., 2018a; Pouchon et al., 2018). Yet, these positive effects are offset by distribution losses in sensitive Páramo localities, besides rising mining, agriculture and population density. These are Páramo’s current major anthropogenic threats (Vásquez et al., 2015; Pérez-Escobar et al., 2018) primarily in the western slopes of the Eastern and Central Cordilleras.
Permeable species barriers due to pervasive hybridization make the species concept highly debatable within the Espeletia complex. Therefore we refrained from emphasizing single-species responses that are likely to be overwhelmed by meta-population dynamics. Pouchon et al. (2018) suggested that the entire subtribe Espeletiinae requires an in deep taxonomic revision (Mavárez, 2019b) because most of Cuatrecasas’ (2013) species are thought to be paraphyletic. This trend is not unexpected since the available taxonomy greatly relies on potentially plastic morphological and reproductive traits (Mavárez, 2020) that are susceptible to be driven by environmental effects, besides the fact that it disregards rampant hybridization (Rauscher, 2002). Then, a more appropriate scenario for the young rapidly-evolving Espeletia complex with weak species boundaries would be that ecotypes in the same locality, despite depicting microhabitat divergence, are capable of sharing through gene flow and introgression adaptive genetic variants (Cortés et al., 2018a). Gene swapping because of porosities in the species bounds would imply that (1) the effective size of “rare” taxa with few records is higher in terms of standing adaptive variation, and (2) concerted climate responses is feasible across lineages within the same Páramo sky island. Inter-mountain exchange is also plausible, as reported in other tropical sky islands (Chala et al., 2020; Tusiime et al., 2020).
A possible way forward for populations to persist is the very same driver that is thought to contribute with the unbeatable diversification rate in the Páramo flora (Madriñán et al., 2013) – local-scale adaptive variation (Huang et al., 2020; Todesco et al., 2020). As part of this study we considered major enhancers and constrainers of the adaptive potential at a regional scale. However, populations may still harbor intrinsic genetic variation naturally selected to cope with environmental differences at the microhabitat level (Ramírez et al., 2014). In highly heterogeneous mountainous environments, local-scale environmental effects are known for shaping genetic diversity and generating morphological diversity (Hughes and Atchison, 2015), which may prove useful in the reaction to climate change within the same locality. There is already compiling evidence of ecological-driven divergence in the radiation of Andean Espeletia (Cortés et al., 2018a), besides allopatric differentiation and isolation by distance (Diazgranados and Barber, 2017; Padilla-González et al., 2017, 2018). Ecological parapatric speciation is a likely consequence of local patterns of environmental variation (Naciri and Linder, 2020) typically found at the Páramo ecosystem (Monasterio and Sarmiento, 1991). For instance, frost is more extreme in the wind-exposed high valley slopes than in the wind-sheltered depressions of those valleys or in the upper boundary of the cloud forest. Soil moisture content, higher in the well-irrigated high valley depressions and in the upper boundary of the cloud forest than in the drier slopes of the valleys, could also contribute to this divergence. It now remains to be explored whether this mosaic of environmental heterogeneity has enforced enough heritable polygenic (Barghi et al., 2020) phenotypic variation as a pre-adaptation (Nürk et al., 2018) to the new selective forces imposed by climate change.
One potential caveat of our results precisely concerns data availability at the micro-scale level. Bioclimatic data is reliably modeled throughout the Páramo range at a 1 km2 spatial resolution, which unfortunately overlooks micro-environmental drivers of the local-scale genetic adaptation (De La Harpe et al., 2017; Leroy et al., 2020). This is of particular importance at mountain/alpine ecosystems (Ramírez et al., 2014; Cortés and Wheeler, 2018), where microhabitats may serve as refugia (Zellweger et al., 2020), like across the treeline due to active landform processes (Bueno and Llambí, 2015; Arzac et al., 2019; Gentili et al., 2020). Populations may rely on specific small-scale habitat attributes that are likely to be overlooked by SDMs (Sinclair et al., 2010), such as topographic, geomorphic, and edaphic features, as well as the distribution of other taxa – e.g., competitors and facilitators (Yackulc et al., 2015). Due to this, SDMs may exhibit substantial uncertainty (Buisson et al., 2010) and lack of validation (Botkin et al., 2007) at local scales. Therefore, niche models could be insensitive to micro-environmental processes, leading to an underestimation of the mechanisms governing populations’ responses. Even if bioclimatic models aim representing the realized niche, they may then disregard the fundamental niche (Loehle and Leblanc, 1996). Despite statistical imputation-like approaches such as environmental downscaling have been considered to model climate at a higher resolution for specific Páramo complexes (Mavárez et al., 2018), consistently applying such techniques across cordilleras seems unfeasible because accuracy would be constrained by the accessibility of fine-scale bioclimatic data from regional weather stations. These restraints are likely to be overcome soon by incorporating microclimate into SDMs (Lembrechts et al., 2019) through mechanistic algorithms (Lembrechts and Lenoir, 2020) physiological models (Cortés et al., 2013; López-Hernández and Cortés, 2019), hydrological equations (Rodríguez-Morales et al., 2019; Correa et al., 2020), in situ data logging, and remote sensing (Ramón-Reinozo et al., 2019; Zellweger et al., 2019).
Another potential caveat of our inferences relate with data availability across the entire Páramo area in the northern Andes. Some components of the adaptive capacity score (i.e., protected areas, agriculture, and mining pressures) are downloadable only through national repositories, making standardizations and comparisons across all Páramo complexes in different countries impractical. Yet, it is worth to clarify that climate sensitivity, adaptation capacity and vulnerability indices are not meant to be absolute scores but rather relative values to compare among Páramo complexes. In this sense, the power of our inferences to rank Páramo areas is unlikely limited by the pessimistic predictions of the future climate scenario RCP 8.5, making our vulnerability index robust. Furthermore, our whole integrative analytical pipeline is in line with the most up-to-date approaches to carry out climate change vulnerability assessment (Ramirez-Villegas et al., 2014; Valladares et al., 2014; Foden et al., 2018).
By assessing climate sensitivity and adaptive capacity across Páramo sky islands, we predicted climate change vulnerability in the rapidly evolving Espeletia complex, a syngameon (Cannon and Petit, 2020) likely shaped by incomplete ecological speciation (Nosil et al., 2009) and adaptive introgression (Fitzpatrick et al., 2015; Martin and Jiggins, 2017). However, in order to gather a more comprehensive view of climate change responses in the high mountain ecosystems of the northern Andes, other diverse and highly abundant plant genera in the Páramo (Hughes et al., 2013) should be considered under a similar analytical framework as the described here. Immediate candidates to follow up this approach are Bartsia (Uribe-Convers and Tank, 2015), Chusquea (Ely et al., 2019), Diplostephium (Vargas et al., 2017), Hypericum (Nürk et al., 2013), Loricaria (Kolar et al., 2016), Lupinus (Hughes and Eastwood, 2006; Vásquez et al., 2016; Contreras-Ortiz et al., 2018), Oreobolus (Chacón et al., 2006; Gómez-Gutiérrez et al., 2017), Puya (Jabaily and Sytsma, 2013), and Senecio (Duskova et al., 2017; Walter et al., 2020). These combined efforts would ultimately reveal whether the fastest evolving biodiversity hotspot on earth, and in general tropical high mountain ecosystems (Hedberg, 1964; Sklenáø et al., 2014; Chala et al., 2016), have a chance to persist under current environmental and anthropogenic threats.
Besides range shifts (Chen et al., 2011; Feeley et al., 2011; Donoghue and Edwards, 2014; Kolar et al., 2016; Freeman et al., 2020) and adaptive responses (Hoffmann and Sgro, 2011; Franks and Hoffmann, 2012; Donoghue, 2019; Ørsted et al., 2019), introgression, hybridization (Lafon-Placette et al., 2016), and polyploidization (Han et al., 2019; Mason and Wendel, 2020; Nieto Feliner et al., 2020) which have not been explicitly explored in this study, may also provide innovative (Donoghue and Sanderson, 2015) adaptive sources in plants (Abbott et al., 2013; Marques et al., 2019). The Espeletia complex is known for a high incidence of natural hybrids as told by phenotypic and genetic markers (Rauscher, 2002; Pouchon et al., 2018). Hence, assessing the role of reticulate evolution and adaptive introgression for the Espeletia complex to face a changing environment will require sampling of hybrids and their putative parental populations across the Páramo’s current micro-ecological mosaic.
Even though hybrids could occupy intermediate niches, persistence of populations in alpine environments that face climate change is regarded as mostly mediated by local-scale (i.e., microhabitat) variation (Cortés et al., 2014; Cortés and Wheeler, 2018). For example, environmental heterogeneity may provide new suitable locations for migrants within only a few meters of their current locations (Scherrer and Körner, 2011), driving in this way plant responses to warming (Zellweger et al., 2020). More localized environmental data must then be gathered (Zellweger et al., 2019), since current climate repositories lack the resolution needed to describe the microhabitat heterogeneity. Topographic and soil properties, often overlooked by climatic models, are presumably key in defining Páramo’s wet and drier microhabitats in the wind-sheltered high valley depressions, and in the more wind-exposed slopes. This local-scale environmental trend has diversity implications for other endemic plant species in the northern Andes (Cortés et al., 2012a,b; Blair et al., 2016). Besides high-resolution environmental data, eco-physiological (Llambí and Rada, 2019; Sandoval et al., 2019) and ecological adaptive traits (e.g., Bruelheide et al., 2018; Cortés and Blair, 2018) must be noted more systematically at local scales in natural surveys and field tests, like reciprocal transplant assays of ecotypes among microhabitats (as in Sedlacek et al., 2015), and space-by-time substitution trials across altitudes (Cuesta et al., 2017) and habitats (Wheeler et al., 2014, 2016). Ultimately, micro-scales keep genetic variance (Cortés et al., 2011, 2018b; Galeano et al., 2012; Kelleher et al., 2012; Blair et al., 2013, 2018; Cortés, 2013; Wu et al., 2020), morphological diversity, and adaptive trait variation (Sedlacek et al., 2016; Pacifici et al., 2017).
Conservation efforts may also buffer the impact of climate change on Páramo ecosystems by enhancing their adaptive capacity. Vast areas of Páramo land now safe from conflict are not being rapidly protected, making them susceptible to deforestation for cattle and agriculture (Avellaneda-Torres et al., 2020), and illegal logging (Vásquez et al., 2015) and mining (Pérez-Escobar et al., 2018). Thus, improving the current network of protected areas would help minimizing ongoing threats (Duchicela et al., 2019), especially in the highly diverse Páramo complexes of the Eastern Cordillera where putatively new species are still being discovered (Diazgranados and Sanchez, 2017; Mavárez, 2019a). Alongside adoption of mitigation policies, adaptation policies (Elsen et al., 2020) may help safeguarding Páramos. Finding more sustainable land uses by empowering local communities and developing ecotourism are then as essential to relieve human impact on tropical-alpine plant diversity in the northern Andes.
Last but not least, extending the modeling approach implemented in this work to other key pressures (e.g., fire, Wu and Porinchu, 2019; Rivadeneira et al., 2020; Zomer and Ramsay, 2020) and plant groups (Luteyn, 1999) in the Páramo, as well as to other sky islands around the mountains of the world (Hoorn et al., 2018; Pausas et al., 2018; Nürk et al., 2019; Testolin et al., 2020), and more broadly to other island-like systems (Papadopoulou and Knowles, 2015; Lamichhaney et al., 2017; Cámara-Leret et al., 2020; Flantua et al., 2020), will help understanding climate change effects on unrelated taxa experiencing similar evolutionary processes (Condamine et al., 2018; Cortés et al., 2020; Nürk et al., 2020). Such systems offer natural experiments to assess the role of colonization and adaptation (Ding et al., 2020; McGee et al., 2020; Tito et al., 2020) in the past and ongoing responses to climate change, which undeniably will complement ecological predictive modeling.
All datasets generated for this study are included in the article/Supplementary Material and in the Dryad repository under https://doi.org/10.5061/dryad.gf1vhhmmp. Further inquiries can be directed to the corresponding author.
AC, JV, and SM conceived the study. JV compiled the data. JV and JM analyzed the data with contributions from AC and JL. AC, JV, and SM interpreted the data. AC wrote the manuscript with contributions from JV, and further edits made by the other co-authors. All authors contributed to the article and approved the submitted version.
Grants 4.1-2016-00418 from Vetenskapsrådet (VR) and BS20170036 from Kungliga Vetenskapsakademien (KVA) are thanked for funding AC. The University of California (CA, United States) Conservation Grant, the Wildlife Conservation Society (WCS, NY, United States) and Facultad de Ingeniería y Administración from Universidad Nacional de Colombia (Palmira, Colombia) are also acknowledged for supporting JV’s presentation at ESRI User Conference during 2018 at San Diego (CA, United States). The editorial fund from the Colombian Corporation for Agricultural Research (AGROSAVIA) is thanked for financing this publication.
The authors declare that the research was conducted in the absence of any commercial or financial relationships that could be construed as a potential conflict of interest.
We thank early discussions on Espeletia ecological divergence with A. Antonelli, C.D. Bacon, I. Calixto-Botía, N. Contreras-Ortiz, I. Jiménez, J. Mavarez, and M. Pineda. J. Ramirez-Villegas, and C.C. Sosa assistance in the prioritization of bioclimatic variables is recognized. V. Bermúdez, L. Gaona, and A. Salamanca are acknowledged for administrative support. M.J. Torres-Urrego aid while drafting this work is deeply appreciated, too. Some of the analyses and ideas presented in this manuscript were polished thanks to comments from participants of the Facultad de Ingeniería y Administración (UNAL) FiaInnova Workshop, and the International Center for Tropical Agriculture (CIAT)’s Decision & Policy Analysis (DAPA) Annual Meeting, respectively, held in November 2018 and April 2019 at Palmira (Colombia). Topic editors and reviewers are also thanked for envisioning a well-timed Research Topic on the “Consequences of Climate Change for Plant Biodiversity in High Mountain Ecosystems,” and for improving an early version of this manuscript through their thoughtful recommendations.
The Supplementary Material for this article can be found online at: https://www.frontiersin.org/articles/10.3389/fevo.2020.565708/full#supplementary-material
Supplementary Figure 1 | Frequency distributions of the number of records per taxon (A), and the number of Páramo complexes (B) spanned by each of the 28 Espeletia taxa across Páramo sky islands in the Colombian Andes. Number of records, and number of Páramo complexes spanned for each taxon are drawn from Supplementary Table S2.
Supplementary Figure 2 | Pairwise Pearson correlation coefficients (r) among 19 WorldClim bioclimatic variables (in the baseline period 1970–2000), and Digital Elevation Model (DEM) at a 30 s (1 km2) spatial resolution. Five bioclimatic variables and DEM did not exhibit collinearity problems (Supplementary Table S3) and were retained.
Supplementary Figure 3 | Ridgeline plots for the adaptive capacity (A), climate sensitivity (B), and vulnerability (C) scores across 36 Páramo complexes in the Colombian Andes. For comparative purposes, Páramo complexes are sorted in (A) from those with a low adaptive potential to anthropic pressures according to the enhancing and limiting factors depicted in Figure 3, in (B) from those likely to experience a range loss (distributions below zero) to those predicted to exhibit a range gain (distributions above zero) by 2050 (under RCP 8.5), while in (C) Páramo complexes at the top are the most vulnerable. Ridgeline plots summarize grid estimates shown in Figure 4.
Supplementary Figure 4 | Pairwise Pearson correlation coefficients (r) among climate sensitivity (S), adaptive capacity (AC), climate vulnerability (V) and the components of the adaptive capacity in the Espeletia complex across Páramo sky islands in the Colombian Andes. The adaptive capacity enhancing components were diversity (D), protected areas (PA) and forest area around the Páramo (F), while its limiting factors were agriculture (A), mining (M) and rural population density (RPD). The area of each of the 36 Páramo complexes is also considered in hectares (Ha). Correlation estimates and 95% confidence intervals are presented above the diagonal, while below the diagonal circles are colored and sized accordingly. Estimates are gathered from Supplementary Table S7 and are negatively transformed for agriculture (A), mining (M) and rural population density (RPD) in order to aid interpretation.
Supplementary Table 1 | Occurrence data of 28 Espeletia taxa across Páramo sky islands in the Colombian Andes. Occurrences were gathered as georeferenced specimen data from GBIF. Given a starting set of 15,466 specimen records, a total of 1,432 were kept after cleaning for georeferencing consistency (i.e., non-redundant records located within the study area) and number of records per species (to avoid distributions with less than 10 points, as suggested by Van Proosdij et al. (2016) for narrow-ranged species). Espeletia taxonomy follows Cuatrecasas (2013).
Supplementary Table 2 | Number of records for each of the 28 Espeletia taxa across Páramo sky islands in the Colombian Andes, and altitudinal range for each taxon. Number of records, number of Páramo complexes spanned, and minimum and maximum altitudes for each taxon are gathered from Supplementary Table S1.
Supplementary Table 3 | Variance Inflation Factor (VIF) for five non-collinear (VIF < 10) WorldClim bioclimatic variables considered for modeling at a 30 s (1 km2) spatial resolution for the current and future scenarios, besides a Digital Elevation Model (DEM). Absolute r-values ranged from 0.05 (BIO18∼DEM) to 0.65 (BIO15∼BIO18).
Supplementary Table 4 | Current model validation based on average Area Under the ROC Curve (AUC) and porcentual contribution of the six non-collinear environmental variables for each of the 28 Espeletia taxa. AUC in the training and testing datasets are an aggregate measure of performance across all possible classification thresholds. Pseudo-absences (number of background points) are also averaged for each of the 28 putative taxa. Un-averaged statistics are kept in Supplementary Table S5. The six non-collinear variables included five WorldClim bioclimatic variables at a 30 s (1 m2) spatial resolution. Environmental variables (Supplementary Table S3) are abbreviated as follows: (BIO2) Mean Diurnal Range, (BIO4) Temperature Seasonality, (BIO15) Precipitation Seasonality, (BIO18) Precipitation of Warmest Quarter, (BIO19) Precipitation of Coldest Quarter, and (DEM) Digital Elevation Model.
Supplementary Table 5 | Current model validation based on Area Under the ROC Curve (AUC) and relative contribution of the six non-collinear environmental variables for each of the 10 runs in the 28 Espeletia taxa. The following overall summary statistics are kept: optimum training subset, regularized training gains, un-regularized training gains, effective iterations, training AUC, optimum testing subset, test gains, test AUC, AUC standard deviation, pseudo-absences (number of background points), and entropy. The following relative contribution scores are kept for the six environmental variables: contribution, permutation importance, training gain, test gain and AUC without and with only each variable. The six non-collinear environmental variables (Supplementary Table S3) included five WorldClim BIO variables at a 30 s (1 km2) spatial resolution, and are coded as: (BIO2) Mean Diurnal Range, (BIO4) Temperature Seasonality, (BIO15) Precipitation Seasonality, (BIO18) Precipitation of Warmest Quarter, (BIO19) Precipitation of Coldest Quarter, and (DEM) Digital Elevation Model.
Supplementary Table 6 | Current model validation based on Akaike (AIC) and Bayesian (BIC) Information Criteria per repetition of each of the 28 Espeletia taxa. Optimum training and testing subsets are summed under NTR+TS.
Supplementary Table 7 | Climate sensitivity (S), adaptive capacity (AC), and climate vulnerability (V) in the Espeletia complex across Páramo sky islands in the Colombian Andes. The adaptive capacity enhancing components were diversity (D), protected areas (PA) and forest area around the Páramo (F), while its limiting factors were agriculture (A), mining (M) and rural population density (RPD). Diversity (D), which is the number of Espeletia taxa totalized in a 1 km grid based on the occurrence data downloaded from GBIF, and the inverse value of the normalized rural population density (RPD) were scaled from 0 to 1. A total of 1,050 protected areas (PA) were rated as 1, 0.3, 0.1, and 0.1 for National Natural Parks, Civil Society Reserves, Regional Natural Parks and National Protected Forest Reserves, respectively, following (CIAT, 2017). Forest patches (F) around the Páramo complexes (2 km around the tree line, 1 km grid) were recorded as presence points (1 = presence), while agriculture (A) and mining (M) for the period 2010–2012 were marked as null data points (0 = presence) within the study area and in its buffer zone (2 km around the tree line, 1 km grid). The area of each of the 36 Páramo complexes is also presented in hectares (Ha), as well as its name and ID number following Figure 2A, Morales et al. (2007) and Londoño et al. (2014).
Abbott, R., Albach, D., Ansell, S., Arntzen, J. W., Baird, S. J. E., Bierne, N., et al. (2013). Hybridization and speciation. J. Evol. Biol. 26, 229–246.
Antonelli, A., Nylander, J. A. A., Persson, C., and Sanmartin, I. (2009). Tracing the impact of the andean uplift on neotropical plant evolution. Proc. Natl. Acad. Sci. U.S.A. 106, 9749–9754. doi: 10.1073/pnas.0811421106
Arnold, P. A., Kruuk, L. E. B., and Nicotra, A. B. (2019). How to analyse plant phenotypic plasticity in response to a changing climate. New Phytol. 222, 1235–1241. doi: 10.1111/nph.15656
Arzac, A., Llambí, L. D., Dulhoste, R., Olano, J. M., and Chacón-Moreno, E. (2019). Modelling the effect of temperature changes on plant life-form distribution across a treeline ecotone in the tropical Andes. Plant Ecol. Divers. 12, 619–631.
Avellaneda-Torres, L. M., Sicard, T. L., Castro, E. G., and Rojas, E. T. (2020). Potato cultivation and livestock effects on microorganism functional groups in soils from the neotropical high andean páramo. Rev. Brasi. Ciênc. Solo 44:e0190122.
Barghi, N., Hermisson, J., and SchlöTterer, C. (2020). Polygenic adaptation: a unifying framework to understand positive selection. Nat. Rev. Genet. doi: 10.1038/s41576-020-0250-z
CrossRef Full Text. [Epub ahead of print]. | PubMed Abstract | Google Scholar
Berry, P. M., Dawson, T. P., Harrison, P. A., Pearson, R., and Butt, N. (2003). The sensitivity and vulnerability of terrestrial habitats and species in britain and ireland to climate change. J. Nat. Conserv. 11, 15–23. doi: 10.1078/1617-1381-00030
Blair, M. W., Cortes, A. J., Farmer, A. D., Huang, W., Ambachew, D., Penmetsa, R. V., et al. (2018). Uneven recombination rate and linkage disequilibrium across a reference snp map for common bean (Phaseolus vulgaris L.). PLoS One 13:e0189597. doi: 10.1371/journal.pone.0189597
Blair, M. W., Cortés, A. J., Penmetsa, R. V., Farmer, A., Carrasquilla-Garcia, N., and Cook, D. R. (2013). A high-throughput snp marker system for parental polymorphism screening, and diversity analysis in common bean (Phaseolus vulgaris L.). Theor. Appl. Genet. 126, 535–548. doi: 10.1007/s00122-012-1999-z
Blair, M. W., Cortés, A. J., and This, D. (2016). Identification of an erecta gene and its drought adaptation associations with wild and cultivated common bean. Plant Sci. 242, 250–259. doi: 10.1016/j.plantsci.2015.08.004
Botkin, D. B., Saxe, H., Araújo, M. B., Betts, R., Bradshaw, R. H. W., Cedhagen, T., et al. (2007). Forecasting the effects of global warming on biodiversity. Bioscience 57, 227–236.
Bridle, J. R., and Vines, T. H. (2007). Limits to evolution at range margins: when and why does adaptation fail? Trends Ecol. Evol. 22, 140–147. doi: 10.1016/j.tree.2006.11.002
Bruelheide, H., Dengler, J., Purschke, O., Lenoir, J., Jiménez-Alfaro, B., Hennekens, S. M., et al. (2018). Global trait-environment relationships of plant communities. Nat. Ecol. Evol. 2, 1906–1917.
Bueno, A., and Llambí, L. D. (2015). Facilitation and edge effects influence vegetation regeneration in old-fields at the tropical Andean forest line. Appl. Veg. Sci. 18, 613–623.
Buffum, B., Mcgreevy, T. J. Jr., Gottfried, A. E., Sullivan, M. E., and Husband, T. P. (2015). An analysis of overstory tree canopy cover in sites occupied by native and introduced cottontails in the northeastern United States with recommendations for habitat management for new england cottontail. PLoS One 10:e0135067. doi: 10.1371/journal.pone.0135067
Buisson, L., Thuiller, W., Casajus, N., Lek, S., and Grenouillet, G. (2010). Uncertainty in ensemble forecasting of species distribution. Glob. Chang. Biol. 16, 1145–1157. doi: 10.1111/j.1365-2486.2009.02000.x
Buytaert, W., Cuesta-Camacho, F., and Tobón, C. (2011). Potential impacts of climate change on the environmental services of humid tropical alpine regions. Glob. Ecol. Biogeogr. 20, 19–33. doi: 10.1111/j.1466-8238.2010.00585.x
Cáceres, Y., Llambí, L. D., and Rada, F. (2014). Shrubs as foundation species in a high tropical alpine ecosystem: a multi-scale analysis of plant spatial interactions. Plant Ecol. Divers. 8, 147–161. doi: 10.1080/17550874.2014.960173
Cámara-Leret, R., Frodin, D. G., Adema, F., Anderson, C., Appelhans, M. S., Argent, G., et al. (2020). New guinea has the world’s richest Island Flora. Nature 584, 579–583.
Cannon, C. H., and Petit, R. J. (2020). The oak syngameon: more than the sum of its parts. N. Phytol. 226, 978–983. doi: 10.1111/nph.16091
Chacón, J., Madriñán, S., Chase, M. W., and Bruhl, J. J. (2006). Molecular phylogenetics of oreobolus (Cyperaceae) and the origin and diversification of the American species. Taxon 55, 359–366. doi: 10.2307/25065583
Chala, D., Brochmann, C., Psomas, A., Ehrich, D., Gizaw, A., Masao, C. A., et al. (2016). Good-bye to tropical alpine plant giants under warmer climates? loss of range and genetic diversity in lobelia rhynchopetalum. Ecol. Evol. 6, 8931–8941. doi: 10.1002/ece3.2603
Chala, D., Zimmermann, N. E., Brochmann, C., and Bakkestuen, V. (2020). Migration corridors for alpine plants among the ‘sky islands’ of eastern africa: do they, or did they exist? Alp. Bot. 127, 133–144. doi: 10.1007/s00035-017-0184-z
Chen, I. C., Hill, J. K., Ohlemuller, R., Roy, D. B., and Thomas, C. D. (2011). Rapid range shifts of species associated with high levels of climate warming. Science 333, 1024–1026. doi: 10.1126/science.1206432
CIAT (2017). Formulación Del Plan Regional Integral De Cambio Climático Para La Orinoquía, Departamentos De Meta, Casanare, Vichada Y Arauca. Cali: CIAT.
Cobos, M. E., Peterson, A. T., Osorio-Olvera, L., and Jiménez-García, D. (2019). An exhaustive analysis of heuristic methods for variable selection in ecological niche modeling and species distribution modeling. Ecol. Inform. 53:100983. doi: 10.1016/j.ecoinf.2019.100983
Cochrane, A., Nicotra, A., and Ooi, M. (2019). Climate change: alters plant recruitment from seed. Aust. Ecol. 44, 931–934. doi: 10.1111/aec.12728
Condamine, F. L., Antonelli, A., Lagomarsino, L. P., Hoorn, C., and Liow, L. H. (2018). “Teasing apart mountain uplift, climate change and biotic drivers of species diversification,” in Mountains, Climate, and Biodiversity, eds C. Hoorn, A. Perrigo, and A. Antonelli (New York, NY: Wiley).
Contreras-Ortiz, N., Atchison, G. W., Hughes, C. E., and Madriñán, S. (2018). Convergent evolution of high elevation plant growth forms and geographically structured variation in Andean Lupinus (Fabaceae). Bot. J. Linn. Soc. 187, 118–136. doi: 10.1093/botlinnean/box095
Correa, A., Ochoa-Tocachi, B. F., Birkel, C., Ochoa-Sánchez, A., Zogheib, C., Tovar, C., et al. (2020). A concerted research effort to advance the hydrological understanding of tropical páramos. Hydrol. Process. doi: 10.1002/hyp.13904
Cortés, A. J. (2013). On the origin of the common bean (Phaseolus vulgaris L.). Am. J. Plant Sci. 4, 1998–2000.
Cortés, A. J., and Blair, M. W. (2018). Genotyping by sequencing and genome - environment associations in wild common bean predict widespread divergent adaptation to drought. Front. Plant Sci. 9:128. doi: 10.3389/fpls.2018.00128
Cortés, A. J., Chavarro, M. C., and Blair, M. W. (2011). Snp marker diversity in common bean (Phaseolus vulgaris L.). Theor. Appl. Genet. 123, 827–845. doi: 10.1007/s00122-011-1630-8
Cortés, A. J., Chavarro, M. C., Madriñán, S., This, D., and Blair, M. W. (2012a). Molecular ecology and selection in the drought-related asr gene polymorphisms in wild and cultivated common bean (Phaseolus vulgaris L.). BMC Genet. 13:58. doi: 10.1186/1471-2156-13-58
Cortés, A. J., This, D., Chavarro, C., Madriñán, S., and Blair, M. W. (2012b). Nucleotide diversity patterns at the drought-related Dreb2 encoding genes in wild and cultivated common bean (Phaseolus vulgaris L.). Theoret. Appl. Genet. 125, 1069–1085. doi: 10.1007/s00122-012-1896-5
Cortés, A. J., Garzón, L. N., Valencia, J. B., and Madriñán, S. (2018a). On the causes of rapid diversification in the páramos: isolation by ecology and genomic divergence in espeletia. Front. Plant Sci. 9:1700. doi: 10.3389/fpls.2018.01700
Cortés, A. J., Skeen, P., Blair, M. W., and Chacón-Sánchez, M. I. (2018b). Does the genomic landscape of species divergence in phaseolus beans coerce parallel signatures of adaptation and domestication? Front. Plant Sci. 9:1816. doi: 10.3389/fpls.2018.01816
Cortés, A. J., López-Hernández, F., and Osorio-Rodriguez, D. (2020). Predicting thermal adaptation by looking into populations’ genomic past. Front. Genet. doi: 10.3389/fgene.2020.564515
CrossRef Full Text. [Epub ahead of print]. | Google Scholar
Cortés, A. J., Monserrate, F., Ramírez-Villegas, J., Madriñán, S., and Blair, M. W. (2013). Drought tolerance in wild plant populations: the case of common beans (Phaseolus vulgaris L.). PLoS One 8:e62898. doi: 10.1371/journal.pone.062898
Cortés, A. J., Waeber, S., Lexer, C., Sedlacek, J., Wheeler, J. A., Van Kleunen, M., et al. (2014). Small-Scale patterns in snowmelt timing affect gene flow and the distribution of genetic diversity in the alpine dwarf shrub salix herbacea. Heredity 113, 233–239. doi: 10.1038/hdy.2014.19
Cortés, A. J., and Wheeler, J. A. (2018). “The environmental heterogeneity of mountains at a fine scale in a changing world,” in Mountains, Climate, and Biodiversity, eds C. Hoorn, A. Perrigo, and A. Antonelli (New York, NY: Wiley).
Crandall, K. A., Tovar, C., Arnillas, C. A., Cuesta, F., and Buytaert, W. (2013). Diverging responses of tropical andean biomes under future climate conditions. PLoS ONE 8:e63634. doi: 10.1371/journal.pone.0063634
Cuatrecasas, J. (2013). A Systematic Study of the Subtribe Espeletiinae: Heliantheae, Asteraceae. New York, NY: The New York Botanical Garden.
Cuesta, F., Llambí, L. D., Huggel, C., Drenkhan, F., Gosling, W. D., Muriel, P., et al. (2019a). New land in the neotropics: a review of biotic community, ecosystem, and landscape transformations in the face of climate and glacier change. Reg. Environ. Chang. 19, 1623–1642. doi: 10.1007/s10113-019-01499-3
Cuesta, F., Tovar, C., Llambí, L. D., Gosling, W. D., Halloy, S., Carilla, J., et al. (2019b). Thermal niche traits of high alpine plant species and communities across the tropical andes and their vulnerability to global warming. J. Biogeogr. 47, 408–420. doi: 10.1111/jbi.13759
Cuesta, F., Muriel, P., Llambí, L. D., Halloy, S., Aguirre, N., Beck, S., et al. (2017). Latitudinal and altitudinal patterns of plant community diversity on mountain summits across the tropical andes. Ecography 40, 1381–1394. doi: 10.1111/ecog.02567
De La Harpe, M., Paris, M., Karger, D. N., Rolland, J., Kessler, M., Salamin, N., et al. (2017). Molecular ecology studies of species radiations: current research gaps, opportunities and challenges. Mol. Ecol. 26, 2608–2611. doi: 10.1111/mec.14110
Diazgranados, M., and Barber, J. C. (2017). Geography shapes the phylogeny of frailejones (Espeletiinae Cuatrec., Asteraceae): a remarkable example of recent rapid radiation in sky Islands. PeerJ 5:e2968. doi: 10.7717/peerj.2968
Diazgranados, M., and Sanchez, L. R. (2017). Espeletia praesidentis, a new species of espeletiinae (Millerieae, Asteraceae) from Northeastern colombia. Phytokeys 76, 1–12. doi: 10.3897/phytokeys.76.11220
Ding, W.-N., Ree, R. H., Spicer, R. A., and Xing, Y. W. (2020). Ancient orogenic and monsoon-driven assembly of the world’s richest temperate alpine Flora. Science 369, 578–581. doi: 10.1126/science.abb4484
Donoghue, M. J. (2019). Adaptation meets dispersal: legumes in the land of succulents. N. Phytol. 222, 1667–1616. doi: 10.1111/nph.15834
Donoghue, M. J., and Edwards, E. J. (2014). Biome shifts and niche evolution in plants. Annu. Rev. Ecol. Evol. Syst. 45, 547–572. doi: 10.1146/annurev-ecolsys-120213-091905
Donoghue, M. J., and Sanderson, M. J. (2015). Confluence, synnovation, and depauperons in plant diversification. New Phytol. 207, 260–274. doi: 10.1111/nph.13367
Dormann, C. F., Elith, J., Bacher, S., Buchmann, C., Carl, G., Carré, G., et al. (2013). Collinearity: a review of methods to deal with it and a simulation study evaluating their performance. Ecography 36, 27–46. doi: 10.1111/j.1600-0587.2012.07348.x
Duchicela, S. A., Cuesta, F., Pinto, E., Gosling, W. D., and Young, K. R. (2019). Indicators for assessing tropical alpine rehabilitation practices. Ecosphere 10:e02595.
Duskova, E., Sklenáø, P., Kolar, F., Vasquez, D. L. A., Romoleroux, K., Fer, T., et al. (2017). Growth form evolution and hybridization in senecio (Asteraceae) from the high equatorial andes. Ecol. Evol. 7, 6455–6468. doi: 10.1002/ece3.3206
Elsen, P. R., Monahan, W. B., Dougherty, E. R., and Merenlender, A. M. (2020). Keeping pace with climate change in global terrestrial protected areas. Sci. Adv. 6:eaay0814. doi: 10.1126/sciadv.aay0814
Ely, F., Rada, F., Fermin, G., and Clark, L. G. (2019). Ecophysiology and genetic diversity in species of the bamboo Chusquea in the high Andes, Venezuela. Plant Ecol. Divers. 12, 555–572.
Feeley, K. J., Silman, M. R., Bush, M. B., Farfan, W., Cabrera, K. G., Malhi, Y., et al. (2011). Upslope migration of andean trees. J. Biogeogr. 38, 783–791. doi: 10.1111/j.1365-2699.2010.02444.x
Feng, X., Park, D. S., Liang, Y., Pandey, R., and Papes, M. (2019). Collinearity in ecological niche modeling: confusions and challenges. Ecol. Evol. 9, 10365–10376. doi: 10.1002/ece3.5555
Fitzpatrick, S. W., Gerberich, J. C., Kronenberger, J. A., Angeloni, L. M., and Funk, W. C. (2015). Locally adapted traits maintained in the face of high gene flow. Ecol. Lett. 18, 37–47. doi: 10.1111/ele.12388
Flantua, S. G. A., O’dea, A., Onstein, R. E., Giraldo, C., and Hooghiemstra, H. (2019). The Flickering connectivity system of the North Andean Páramos. J. Biogeogr. 46, 1808–1825. doi: 10.1111/jbi.13607
Flantua, S. G. A., Payne, D., Borregaard, M. K., Beierkuhnlein, C., Steinbauer, M. J., Dullinger, S., et al. (2020). Snapshot isolation and isolation history challenge the analogy between mountains and islands used to understand endemism. Glob. Ecol. Biogeogr. doi: 10.1111/geb.13155
CrossRef Full Text. [Epub ahead of print]. | Google Scholar
Foden, W. B., Young, B. E., Akçakaya, H. R., Garcia, R. A., Hoffmann, A. A., Stein, B. A., et al. (2018). Climate change vulnerability assessment of species. Wiley Interdiscipl. Rev. Clim. Chang. 10:e551.
Fois, M., Cuena-Lombraña, A., Fenu, G., and Bacchetta, G. (2018). Using species distribution models at local scale to guide the search of poorly known species: review, methodological issues and future directions. Ecol. Model. 385, 124–132. doi: 10.1016/j.ecolmodel.2018.07.018
Fox, R. J., Donelson, J. M., Schunter, C., Ravasi, T., and Gaitan-Espitia, J. D. (2019). Beyond buying time: the role of plasticity in phenotypic adaptation to rapid environmental change. Philos. Trans. R. Soc. Lond. B Biol. Sci. 374:20180174.
Franks, S. J., and Hoffmann, A. A. (2012). Genetics of climate change adaptation. Annu. Rev. Genet. 46, 185–208.
Freeman, B. G., Song, Y., Feeley, K. J., and Zhu, K. (2020). Montane species and communities track recent warming more closely in the tropics. bioRxiv doi: 10.1101/2020.05.18.102848
Galeano, C. H., Cortés, A. J., Fernandez, A. C., Soler, A., Franco-Herrera, N., Makunde, G., et al. (2012). Gene-based single nucleotide polymorphism markers for genetic and association mapping in common bean. BMC Genet. 13:48. doi: 10.1186/1471-2156-13-48
Geange, S. R., BricenÞO, V. F., Aitken, N. C., Ramirez-Valiente, J. A., Holloway-Phillips, M. M., and Nicotra, A. B. (2017). Phenotypic plasticity and water availability: responses of alpine herb species along an elevation gradient. Clim. Chang. Respon. 4:5.
Gentili, R., Baroni, C., Panigada, C., Rossini, M., Tagliabue, G., Armiraglio, S., et al. (2020). Glacier shrinkage and slope processes create habitat at high elevation and microrefugia across treeline for alpine plants during warm stages. Catena 193:104626. doi: 10.1016/j.catena.2020.104626
Goh, C. H., Veliz-Vallejos, D. F., Nicotra, A. B., and Mathesius, U. (2013). The impact of beneficial plant-associated microbes on plant phenotypic plasticity. J. Chem. Ecol. 39, 826–839. doi: 10.1007/s10886-013-0326-8
Gómez-Gutiérrez, M. C., Pennington, R. T., Neaves, L. E., Milne, R. I., Madriñán, S., and Richardson, J. E. (2017). Genetic diversity in the andes: variation within and between the south American species of oreobolus R. Br. (Cyperaceae). Alp. Bot. 127, 155–170. doi: 10.1007/s00035-017-0192-z
Gottfried, M., Pauli, H., Futschik, A., Akhalkatsi, M., Baranèok, P., Benito Alonso, J. L., et al. (2012). Continent-wide response of mountain vegetation to climate change. Nat. Clim. Chang. 2, 111–115. doi: 10.1038/nclimate1329
Han, T. S., Zheng, Q. J., Onstein, R. E., Rojas-Andrés, B. M., Hauenschild, F., Muellner-Riehl, A. N., et al. (2019). Polyploidy promotes species diversification of Allium through ecological shifts. New Phytol. 225, 571–583.
Hazzi, N. A., Moreno, J. S., Ortiz-Movliav, C., and Palacio, R. D. (2018). biogeographic regions and events of isolation and diversification of the endemic biota of the tropical andes. Proc. Natl. Acad. Sci. U.S.A. 115, 7985–7990. doi: 10.1073/pnas.1803908115
Henao-Díaz, F., Arrroyo, S., Cárdenas-Posada, G., Fernández, M., López, J. P., Martínez, D. C., et al. (2019). Biotic characterization of the forest-paramo transition zone in Chingaza Páramo Complex, Colombia. Biota Colombiana 20, 132–145.
Helmer, E. H., Gerson, E. A., Baggett, L. S., Bird, B. J., Ruzycki, T. S., and Voggesser, S. M. (2019). Neotropical cloud forests and páramo to contract and dry from declines in cloud immersion and frost. PLoS ONE 14:e0213155. doi: 10.1371/journal.pone.0213155
Hoffmann, A. A., and Sgro, C. M. (2011). Climate change and evolutionary adaptation. Nature 470, 479–485.
Hoorn, C., Perrigo, A., and Antonelli, A. (2018). “Mountains, climate and biodiversity: an introduction,” in Mountains, Climate, and Biodiversity, eds C. Hoorn, A. Perrigo, and A. Antonelli (New York, NY: Wiley).
Huang, K., Andrew, R. L., Owens, G. L., Ostevik, K. L., and Rieseberg, L. H. (2020). Multiple chromosomal inversions contribute to adaptive divergence of a dune sunflower ecotype. Mol. Ecol. 2020, 1–15.
Hughes, C., and Eastwood, R. (2006). Island radiation on a continental scale: exceptional rates of plant diversification after uplift of the andes. Proc. Natl. Acad. Sci. U.S.A. 103, 10334–10339. doi: 10.1073/pnas.0601928103
Hughes, C., Pennington, R. T., and Antonelli, A. (2013). Neotropical plant evolution: Assembling the big picture. Bot. J. Linn. Soc. 171, 1–18. doi: 10.1111/boj.12006
Hughes, C. E., and Atchison, G. W. (2015). The ubiquity of alpine plant radiations: from the andes to the hengduan mountains. New Phytol. 207, 275–282. doi: 10.1111/nph.13230
Isabel, N., Holliday, J. A., and Aitken, S. N. (2020). Forest genomics: Advancing climate adaptation, forest health, productivity, and conservation. Evol. Appl. 13, 3–10. doi: 10.1111/eva.12902
Jabaily, R. S., and Sytsma, K. J. (2013). Historical biogeography and life-history evolution of Andean Puya (Bromeliaceae). Bot. J. Linnea. Soc. 171, 201–224. doi: 10.1111/j.1095-8339.2012.01307.x
Jump, A. S., and Penuelas, J. (2005). Running to stand still: adaptation and the response of plants to rapid climate change. Ecol. Lett. 8, 1010–1020. doi: 10.1111/j.1461-0248.2005.00796.x
Kelleher, C. T., Wilkin, J., Zhuang, J., Cortés, A. J., Quintero, ÁL. P., Gallagher, T. F., et al. (2012). Snp discovery, gene diversity, and linkage disequilibrium in wild populations of populus tremuloides. Tree Genet. Genom. 8, 821–829. doi: 10.1007/s11295-012-0467-x
Kelly, M. (2019). Adaptation to climate change through genetic accommodation and assimilation of plastic phenotypes. Philos. Trans. R. Soc. Lond. B Biol. Sci. 374:20180176. doi: 10.1098/rstb.2018.0176
Kolar, F., Duskova, E., and Sklenáø, P. (2016). Niche shifts and range expansions along cordilleras drove diversification in a high-elevation endemic plant genus in the tropical andes. Mol. Ecol. 25, 4593–4610. doi: 10.1111/mec.13788
Körner, C. (2003). Alpine Plant Life: Functional Plant Ecology of High Mountain Ecosystems. Berlin: Springer.
Lafon-Placette, C., Vallejo-Marín, M., Parisod, C., Abbott, R. J., and Köhler, C. (2016). Current Plant speciation research: unravelling the processes and mechanisms behind the evolution of reproductive isolation barriers. New Phytol. 209, 29–33. doi: 10.1111/nph.13756
Lamichhaney, S., Han, F., Webster, M. T., Andersson, L., Grant, B. R., and Grant, P. R. (2017). Rapid hybrid speciation in Darwin’s finches. Science 359, 224–228. doi: 10.1126/science.aao4593
Lees, A. C., Attwood, S., Barlow, J., and Phalan, B. (2020). Biodiversity scientists must fight the creeping rise of extinction denial. Nat. Ecol. Evol. doi: 10.1038/s41559-020-01285-z
CrossRef Full Text. [Epub ahead of print]. | PubMed Abstract | Google Scholar
Lembrechts, J. J., and Lenoir, J. (2020). Microclimatic conditions anywhere at any time! Glob. Chang. Biol. 26, 337–339. doi: 10.1111/gcb.14942
Lembrechts, J. J., Nijs, I., and Lenoir, J. (2019). Incorporating microclimate into species distribution models. Ecography 42, 1267–1279. doi: 10.1111/ecog.03947
Lenoir, J., Gégout, J.-C., Guisan, A., Vittoz, P., Wohlgemuth, T., Zimmermann, N. E., et al. (2010). Going against the flow: potential mechanisms for unexpected downslope range shifts in a warming climate. Ecography 33, 295–303.
Lenoir, J., Gegout, J. C., Marquet, P. A., De Ruffray, P., and Brisse, H. (2008). A significant upward shift in plant species optimum elevation during the 20th century. Science 320, 1768–1771. doi: 10.1126/science.1156831
Leon-Garcia, I. V., and Lasso, E. (2019). High heat tolerance in plants from the Andean highlands: Implications for paramos in a warmer world. PLoS One 14:e0224218. doi: 10.1371/journal.pone.0224218
Leroy, T., Louvet, J. M., Lalanne, C., Le Provost, G., Labadie, K., Aury, J. M., et al. (2020). Adaptive introgression as a driver of local adaptation to climate in european white oaks. N. Phytol. 226, 1171–1182. doi: 10.1111/nph.16095
Little, C. J., Wheeler, J. A., Sedlacek, J., Cortés, A. J., and Rixen, C. (2016). Small-scale drivers: the importance of nutrient availability and snowmelt timing on performance of the alpine shrub salix herbacea. Oecologia 180, 1015–1024. doi: 10.1007/s00442-015-3394-3
Liu, C., Berry, P. M., Dawson, T. P., and Pearso, R. G. (2005). Selecting thresholds of occurrence in the prediction of species distributions. Ecography 28, 385–393. doi: 10.1111/j.0906-7590.2005.03957.x
Llambí, L. D., Becerra, M. T., Peralvo, M., Avella, A., Baruffol, M., and Díaz, L. J. (2020). Monitoring biodiversity and ecosystem services in Colombia’s high andean ecosystems: toward an integrated strategy. Mt. Res. Dev. 39:3.
Llambí, L. D., Hupp, N., Saez, A., and Callaway, R. (2018). Reciprocal interactions between a facilitator, natives, and exotics in tropical alpine plant communities. Perspect. Plant Ecol. Evol. Syst. 30, 82–88. doi: 10.1016/j.ppees.2017.05.002
Llambí, L. D., and Rada, F. (2019). Ecological research in the tropical alpine ecosystems of the venezuelan páramo: past, present and future. Plant Ecol. Divers. 12, 519–538. doi: 10.1080/17550874.2019.1680762
Lobo, J. M., Jiménez-Valverde, A., and Real, R. (2008). Auc: a misleading measure of the performance of predictive distribution models. Glob. Ecol. Biogeogr. 17, 145–151. doi: 10.1111/j.1466-8238.2007.00358.x
Loehle, C., and Leblanc, D. (1996). Model-based assessments of climate change effects on forests: a critical review. Ecol. Model. 90, 1–31. doi: 10.1016/0304-3800(96)83709-4
Londoño, C., Cleef, A., and Madriñán, S. (2014). Angiosperm flora and biogeography of the páramo region of colombia, Northern Andes. Flora Morphol. Distribut. Funct. Ecol. Plants 209, 81–87. doi: 10.1016/j.flora.2013.11.006
López-Hernández, F., and Cortés, A. J. (2019). Last-generation genome-environment associations reveal the genetic basis of heat tolerance in common bean (Phaseolus vulgaris L.). Front. Genet. 10:22. doi: 10.3389/fpls.2018.00022
Luteyn, J. L. (1999). Páramos: A Checklist of Plant Diversity, Geographic Distribution and Botanical Literature. New York, NY: The New York Botanical Garden Press.
Madriñán, S., Cortés, A. J., and Richardson, J. E. (2013). Páramo is the world’s fastest evolving and coolest biodiversity hotspot. Front. Genet. 4:192. doi: 10.3389/fpls.2018.00192
Marques, D. A., Meier, J. I., and Seehausen, O. (2019). A combinatorial view on speciation and adaptive radiation. Trends Ecol. Evol. 34, 531–544. doi: 10.1016/j.tree.2019.02.008
Martin, S. H., and Jiggins, C. D. (2017). Interpreting the genomic landscape of introgression. Curr. Opin. Genet. Dev. 47, 69–74. doi: 10.1016/j.gde.2017.08.007
Martín-Bravo, S., Valcárcel, V., Vargas, P., and Luceño, M. (2010). Geographical speciation related to pleistocene range shifts in the Western Mediterranean Mountains (Reseda Sect. Glaucoreseda, Resedaceae). Taxon 59, 466–482. doi: 10.1002/tax.592012
Mason, A. S., and Wendel, J. F. (2020). Homoeologous exchanges, segmental allopolyploidy, and polyploid genome evolution. Front. Genet. 11:1014. doi: 10.3389/fgene.2020.01014
Mavárez, J. (2019a). A taxonomic revision of Espeletia (Asteraceae). The venezuelan radiation. Harvard Pap. Bot. 24:131. doi: 10.3100/hpib.v24iss2.2019.n8
Mavárez, J. (2019b). Taxonomic novelties in páramo plants. Espeletia ramosa (Asteraceae), a new species from Colombia. Phytologia 101, 222–230.
Mavárez, J., Bézy, S., Goeury, T., Fernández, A., and Aubert, S. (2018). Current and future distributions of espeletiinae (Asteraceae) in the venezuelan andes based on statistical downscaling of climatic variables and niche modelling. Plant Ecol. Divers. 12, 633–647. doi: 10.1080/17550874.2018.1549599
Mavárez, J. S. (2020). An illustrated diagnostic key to species in the venezuelan clade of Espeletia (Asteraceae). Harv. Pap. Bot. 25, 79–93.
McGee, M. D., Borstein, S. R., Meier, J. I., Marques, D. A., Mwaiko, S., Taabu, A., et al. (2020). The ecological and genomic basis of explosive adaptive radiation. Nature doi: 10.1038/s41586-020-2652-7
Monasterio, M., and Sarmiento, L. (1991). Adaptive radiation of espeletia in the cold andean tropics. Trends Ecol. Evol. 6, 387–391. doi: 10.1016/0169-5347(91)90159-u
Morales, M., Otero-García, J., Van-Der-Hammen, T., Torres-Perdigón, A., Cadena-Vargas, C. E., Pedraza-Peñaloza, C. A., et al. (2007). Atlas De Páramos De Colombia. Bogotaì: Instituto de Investigación de Recursos Biológicos Alexander von Humboldt.
Mora, M. A., Llambí, L. D., and Ramírez, L. (2018). Giant stem rosettes have strong facilitation effects on alpine plant communities in the tropical Andes. Plant Ecol. Divers. 12, 593–606.
Muellner-Riehl, A. N. (2019). Mountains as evolutionary arenas: patterns, emerging approaches, paradigm shifts, and their implications for plant phylogeographic research in the tibeto-himalayan region. Front. Plant Sci. 10:195. doi: 10.3389/fpls.2018.00195
Mutke, J., Jacobs, R., Meyers, K., Henning, T., and Weigend, M. (2014). Diversity patterns of selected Andean plant groups correspond to topography and habitat dynamics, not orogeny. Front. Genet. 5:351. doi: 10.3389/fgene.2014.00351
Naciri, Y., and Linder, H. P. (2020). The genetics of evolutionary radiations. Biol. Rev. 95, 1055–1072.
Naimi, B., Hamm, N. A. S., Groen, T. A., Skidmore, A. K., and Toxopeus, A. G. (2014). where is positional uncertainty a problem for species distribution modelling? Ecography 37, 191–203. doi: 10.1111/j.1600-0587.2013.00205.x
Navarro-Racines, C., Tarapues, J., Thornton, P., Jarvis, A., and Ramirez-Villegas, J. (2020). High-resolution and bias-corrected Cmip5 projections for climate change impact assessments. Sci. Data 7:7.
Nevado, B., Contreras-Ortiz, N., Hughes, C., and Filatov, D. A. (2018). Pleistocene glacial cycles drive isolation, gene flow and speciation in the high-elevation Andes. New Phytol. 219, 779–793. doi: 10.1111/nph.15243
Nicotra, A. B., Atkin, O. K., Bonser, S. P., Davidson, A. M., Finnegan, E. J., Mathesius, U., et al. (2010). Plant phenotypic plasticity in a changing climate. Trends Plant Sci. 15, 684–692. doi: 10.1016/j.tplants.2010.09.008
Nieto Feliner, G., Casacuberta, J., and Wendel, J. F. (2020). Genomics of evolutionary novelty in hybrids and polyploids. Front. Genet. 11:792. doi: 10.3389/fgene.2020.00792
Nosil, P., Harmon, L. J., and Seehausen, O. (2009). Ecological explanations for (Incomplete) speciation. Trends Ecol. Evol. 24, 145–156. doi: 10.1016/j.tree.2008.10.011
Nürk, N. M., Atchison, G. W., and Hughes, C. E. (2019). Island woodiness underpins accelerated disparification in plant radiations. New Phytol. 224, 518–531. doi: 10.1111/nph.15797
Nürk, N. M., Michling, F., and Linder, H. P. (2018). Are the radiations of temperate lineages in tropical alpine ecosystems pre-adapted? Glob. Ecol. Biogeogr. 27, 334–345. doi: 10.1111/geb.12699
Nürk, N. M., Scheriau, C., and Madrinan, S. (2013). Explosive radiation in high andean hypericum-rates of diversification among new world lineages. Front. Genet. 4:175. doi: 10.3389/fpls.2018.00175
Nürk, N. M., Linder, H. P., Onstein, R. E., Larcombe, M. J., Hughes, C. E., and Pineiro Fernandez, L. (2020). Diversification in evolutionary arenas-assessment and synthesis. Ecol. Evol. 10, 6163–6182. doi: 10.1002/ece3.6313
Ørsted, M., Hoffmann, A. A., Rohde, P. D., Sørensen, P., and Kristensen, T. N. (2019). Strong impact of thermal environment on the quantitative genetic basis of a key stress tolerance trait. Heredity 122, 315–325. doi: 10.1038/s41437-018-0117-7
Pacifici, M., Visconti, P., Butchart, S. H. M., Watson, J. E. M., Cassola, F. M., and Rondinini, C. (2017). Species’ traits influenced their response to recent climate change. Nat. Clim. Chang. 7, 205–208. doi: 10.1038/nclimate3223
Padilla-González, G. F., Diazgranados, M., and Costa, F. B. D. (2017). Biogeography shaped the metabolome of the genus espeletia: a phytochemical perspective on an andean adaptive radiation. Sci. Rep. 7:8835.
Padilla-González, G. F., Diazgranados, M., Oliveira, T. B., Chagas-Paula, D. A., and Costa, F. B. D. (2018). Chemistry of the subtribe espeletiinae (Asteraceae) and its correlation with phylogenetic data: an in silico chemosystematic approach. Bot. J. Linnea. Soc. 186, 18–46. doi: 10.1093/botlinnean/box078
Papadopoulou, A., and Knowles, L. L. (2015). Genomic tests of the species-pump hypothesis: recent island connectivity cycles drive population divergence but not speciation in caribbean crickets across the virgin Islands. Evolution 69, 1501–1517. doi: 10.1111/evo.12667
Pausas, J. G., Bond, W. J., and Sankaran, M. (2018). Humboldt and the reinvention of nature. J. Ecol. 107, 1031–1037. doi: 10.1111/1365-2745.13109
Payseur, B. A., and Rieseberg, L. H. (2016). A Genomic perspective on hybridization and speciation. Mol. Ecol. 25, 2337–2360. doi: 10.1111/mec.13557
Pelayo, R. C., Soriano, P. J., Márquez, N. J., and Navarro, L. (2019). Phenological patterns and pollination network structure in a Venezuelan páramo: a community-scale perspective on plant-animal interactions. Plant Ecol. Divers. 12, 607–618.
Pérez-Escobar, O. A., Cámara-Leret, R., Antonelli, A., Bateman, R., Bellot, S., Chomicki, G., et al. (2018). Mining threatens colombian ecosystems. Science 359:1475.
Perrigo, A., Hoorn, C., and Antonelli, A. (2019). Why mountains matter for biodiversity. J. Biogeogr. 47, 315–325. doi: 10.1111/jbi.13731
Peyre, G., Balslev, H., and Font, X. (2018). Phytoregionalisation of the andean paramo. PeerJ 6:e4786. doi: 10.7717/peerj.4786
Peyre, G., Balslev, H., Font, X., and Tello, J. S. (2019). Fine-scale plant richness mapping of the andean páramo according to macroclimate. Front. Ecol. Evol. 7:12. doi: 10.3389/ffgc.2020.00012
Peyre, G., Balslev, H., Martí, D., Sklenář, P., Ramsay, P., Lozano, P., et al. (2015). VegPáramo, a flora and vegetation database for the Andean páramo. Phytocoenologia 45, 195–201. doi: 10.1127/phyto/2015/0045
Peyre, G., Lenoir, J., Karger, D. N., Gomez, M., Gonzalez, A., Broennimann, O., et al. (2020). The fate of páramo plant assemblages in the sky islands of the northern Andes. J. Veg. Sci. doi: 10.1111/jvs.12898
Phillips, S. J., Anderson, R. P., Dudík, M., Schapire, R. E., and Blair, M. E. (2017). Opening the black box: an open-source release of maxent. Ecography 40, 887–893. doi: 10.1111/ecog.03049
Pouchon, C., Fernández, A., Nassar, J. M., Boyer, F. D. R., Aubert, S., Lavergne, S. B., et al. (2018). Phylogenomic analysis of the explosive adaptive radiation of the espeletia complex (Asteraceae) in the tropical andes. Syst. Biol. 67, 1041–1060. doi: 10.1093/sysbio/syy022
Rahbek, C., Borregaard, M. K., Antonelli, A., Colwell, R. K., Holt, B. G., Nogues-Bravo, D., et al. (2019). Building mountain biodiversity: geological and evolutionary processes. Science 365, 1114–1119. doi: 10.1126/science.aax0151
Ramírez, L. A., Rada, F., and Llambí, L. D. (2014). Linking patterns and processes through ecosystem engineering: effects of shrubs on microhabitat and water status of associated plants in the high tropical Andes. Plant Ecol. 216, 213–225.
Ramirez-Villegas, J., Cuesta, F., Devenish, C., Peralvo, M., Jarvis, A., and Arnillas, C. A. (2014). Using species distributions models for designing conservation strategies of Tropical Andean biodiversity under climate change. J. Nat. Conserv. 22, 391–404.
Ramón-Reinozo, M., Ballari, D., Cabrera, J. J., Crespo, P., and Carrillo-Rojas, G. (2019). Altitudinal and temporal evapotranspiration dynamics via remote sensing and vegetation index-based modelling over a scarce-monitored, high-altitudinal Andean páramo ecosystem of Southern Ecuador. Environ. Earth Sci. 78:340.
Rauscher, J. T. (2002). Molecular phylogenetics of the espeletia complex (Asteraceae): evidence from nrdna its sequences on the closest relatives of an andean adaptive radiation. Am. J. Bot. 89, 1074–1084. doi: 10.3732/ajb.89.7.1074
Razgour, O., Forester, B., Taggart, J. B., Bekaert, M., Juste, J., Ibanez, C., et al. (2019). Considering adaptive genetic variation in climate change vulnerability assessment reduces species range loss projections. PNAS 116, 10418–10423. doi: 10.1073/pnas.1820663116
Rieseberg, L. H. (2003). Major ecological transitions in wild sunflowers facilitated by hybridization. Science 301, 1211–1216. doi: 10.1126/science.1086949
Rivadeneira, G., Ramsay, P. M., and Montúfar, R. (2020). Fire regimes and pollinator behaviour explain the genetic structure of Puya hamata (Bromeliaceae) rosette plants. Alp. Botany 130, 13–23. doi: 10.1007/s00035-020-00234-7
Rodríguez-Morales, M., Acevedo-Novoa, D., Machado, D., Ablan, M., Dugarte, W., and Dávila, F. (2019). Ecohydrology of the Venezuelan páramo: water balance of a high Andean watershed. Plant Ecol. Diver. 12, 573–591.
Ronikier, M. (2011). Biogeography of high-mountain plants in the carpathians: an emerging phylogeographical perspective. Taxon 6, 373–389. doi: 10.1002/tax.602008
Rumpf, S. B., Hulber, K., Klonner, G., Moser, D., Schutz, M., Wessely, J., et al. (2018). Range dynamics of mountain plants decrease with elevation. Proc. Natl. Acad. Sci. U.S.A. 115, 1848–1853. doi: 10.1073/pnas.1713936115
Sandoval, D., Rada, F., and Sarmiento, L. (2019). Stomatal response functions to environmental stress of dominant species in the tropical andean Páramo. Plant Ecol. Divers. 12, 649–661. doi: 10.1080/17550874.2019.1683094
Scherrer, D., and Körner, C. (2011). Topogaphically controlled thermal-habitat differentiation buffers alpine plant diversity against climate warming. J. Biogeogr. 38, 406–416. doi: 10.1111/j.1365-2699.2010.02407.x
Schilthuizen, M., Hoekstra, R. F., and Gittenberger, E. (2004). Hybridization, rare alleles and adaptive radiation. Trends Ecol. Evol. 19, 404–405. doi: 10.1016/j.tree.2004.06.005
Sedlacek, J., Bossdorf, O., Cortés, A. J., Wheeler, J. A., and Van-Kleunen, M. (2014). What role do plant-soil interactions play in the habitat suitability and potential range expansion of the alpine dwarf shrub Salix Herbacea? Basic Appl. Ecol. 15, 305–315. doi: 10.1016/j.baae.2014.05.006
Sedlacek, J., Cortés, A. J., Wheeler, J. A., Bossdorf, O., Hoch, G., Klapste, J., et al. (2016). Evolutionary potential in the alpine: trait heritabilities and performance variation of the dwarf willow Salix Herbacea from different elevations and microhabitats. Ecol. Evol. 6, 3940–3952. doi: 10.1002/ece3.2171
Sedlacek, J., Wheeler, J. A., Cortés, A. J., Bossdorf, O., Hoch, G., Lexer, C., et al. (2015). The response of the alpine dwarf shrub Salix Herbacea to altered snowmelt timing: lessons from a multi-site transplant experiment. PLoS One 10:e0122395. doi: 10.1371/journal.pone.0122395
Seehausen, O. (2004). Hybridization and adaptive radiation. Trends Ecol. Evol. 19, 198–207. doi: 10.1016/j.tree.2004.01.003
Sinclair, S. J., White, M. D., and Newell, G. R. (2010). How useful are species distribution models for managing biodiversity under future climates? Ecol. Soc. 15:8.
Sklenáø, P., Hedberg, I., and Cleef, A. M. (2014). Island biogeography of tropical alpine floras. J. Biogeogr. 41, 287–297. doi: 10.1111/jbi.12212
Spiers, J. A., Oatham, M. P., Rostant, L. V., and Farrell, A. D. (2018). Applying species distribution modelling to improving conservation based decisions: a gap analysis of Trinidad and Tobago’s endemic vascular plants. Biodivers. Conserv. 27, 2931–2949. doi: 10.1007/s10531-018-1578-y
Steinbauer, M. J., Grytnes, J. A., Jurasinski, G., Kulonen, A., Lenoir, J., Pauli, H., et al. (2018). Accelerated increase in plant species richness on mountain summits is linked to warming. Nature 556, 231–234.
Sweet, L. C., Green, T., Heintz, J. G. C., Frakes, N., Graver, N., Rangitsch, J. S., et al. (2019). Congruence between future distribution models and empirical data for an iconic species at joshua tree national park. Ecosphere 10:e0276.
Syfert, M. M., Smith, M. J., and Coomes, D. A. (2013). The effects of sampling bias and model complexity on the predictive performance of maxent species distribution models. PLoS One 8:e55158. doi: 10.1371/journal.pone.0055158
Testolin, R., Attorre, F., and Jiménez-Alfaro, B. (2020). Global distribution and bioclimatic characterization of alpine biomes. Ecography 43, 779–788.
Tito, R., Vasconcelos, H. L., and Feeley, K. J. (2020). Mountain ecosystems as natural laboratories for climate change experiments. Front. For. Glob. Chang. 3:38. doi: 10.3389/ffgc.2020.00038
Todesco, M., Owens, G. L., Bercovich, N., Légaré, J. S., Soudi, S., Burge, D. O., et al. (2020). Massive haplotypes underlie ecotypic differentiation in sunflowers. Nature 584, 602–607. doi: 10.1038/s41586-020-2467-6
Tovar, C., Melcher, I., Kusumoto, B., Cuesta, F., Cleef, A., Meneses, R. I., et al. (2020). Plant dispersal strategies of high tropical alpine communities across the Andes. J. Ecol. 108, 1910–1922.
Tusiime, F. M., Gizaw, A., Gussarova, G., Nemomissa, S., Popp, M., Masao, C. A., et al. (2020). Afro-alpine flagships revisited: parallel adaptation, intermountain admixture and shallow genetic structuring in the giant senecios (Dendrosenecio). PLoS One 15:e0228979. doi: 10.1371/journal.pone.00228979
Uribe-Convers, S., and Tank, D. C. (2015). Shifts in diversification rates linked to biogeographic movement into new areas: an example of a recent radiation in the Andes. Am. J. Bot. 102, 1854–1869. doi: 10.3732/ajb.1500229
Valladares, F., Matesanz, S., Guilhaumon, F., Araujo, M. B., Balaguer, L., Benito-Garzon, M., et al. (2014). The effects of phenotypic plasticity and local adaptation on forecasts of species range shifts under climate change. Ecol. Lett. 17, 1351–1364. doi: 10.1111/ele.12348
Van Proosdij, A. S. J., Sosef, M. S. M., Wieringa, J. J., and Raes, N. (2016). Minimum required number of specimen records to develop accurate species distribution models. Ecography 39, 542–552. doi: 10.1111/ecog.01509
Vargas, O. M., Ortiz, E. M., and Simpson, B. B. (2017). Conflicting phylogenomic signals reveal a pattern of reticulate evolution in a recent high-andean diversification (Asteraceae: Astereae: Diplostephium). New Phytol. 214, 1736–1750. doi: 10.1111/nph.14530
Vásquez, D. L. A., Balslev, H., Hansen, M. M., Sklenáø, P., and Romoleroux, K. (2016). Low genetic variation and high differentiation across sky island populations of Lupinus alopecuroides (Fabaceae) in the Northern Andes. Alp. Bot. 126, 135–142. doi: 10.1007/s00035-016-0165-7
Vásquez, D. L. A., Balslev, H., and Sklenáø, P. (2015). Human impact on tropical-alpine plant diversity in the Northern Andes. Biodivers. Conserv. 24, 2673–2683. doi: 10.1007/s10531-015-0954-0
Venn, S., Myers-Smith, I., Camac, J., and Nicotra, A. (2019). Climate change: alpine shrubs as ecosystem engineers. Austr. Ecol. 44, 927–930. doi: 10.1111/aec.12727
Waldvogel, A. M., Feldmeyer, B., Rolshausen, G., Exposito-Alonso, M., Rellstab, C., Kofler, R., et al. (2020). Evolutionary genomics can improve prediction of species’ responses to climate change. Evol. Lett. 4, 4–18. doi: 10.1002/evl3.154
Walter, G. M., Abbott, R. J., Brennan, A. C., Bridle, J. R., Chapman, M., Clark, J., et al. (2020). Senecio as a model system for integrating studies of genotype, phenotype and fitness. New Phytol. 226, 326–344. doi: 10.1111/nph.16434
Warren, D. L., and Seifert, S. N. (2011). Ecological niche modeling in maxent: the importance of model complexity and the performance of model selection criteria. Ecol. Appl. 21, 335–342. doi: 10.1890/10-1171.1
Weigend, M. (2002). Observations on the biogeography of the amotape-huancabamba zone in Northern Peru. Bot. Rev. 68, 38–54. doi: 10.1663/0006-8101(2002)068[0038:ootbot]2.0.co;2
West, A. M., Kumar, S., Brown, C. S., Stohlgren, T. J., and Bromberg, J. (2016). Field validation of an invasive species maxent model. Ecol. Inform. 36, 126–134. doi: 10.1016/j.ecoinf.2016.11.001
Wheeler, J. A., Cortés, A. J., Sedlacek, J., Karrenberg, S., Van Kleunen, M., Wipf, S., et al. (2016). The snow and the willows: accelerated spring snowmelt reduces performance in the low-lying alpine shrub Salix Herbacea. J. Ecol. 104, 1041–1050. doi: 10.1111/1365-2745.12579
Wheeler, J. A., Hoch, G., Cortés, A. J., Sedlacek, J., Wipf, S., and Rixen, C. (2014). Increased spring freezing vulnerability for alpine shrubs under early snowmelt. Oecologia 175, 219–229. doi: 10.1007/s00442-013-2872-8
Wheeler, J. A., Schnider, F., Sedlacek, J., Cortés, A. J., Wipf, S., Hoch, G., et al. (2015). With a little help from my friends: community facilitation increases performance in the dwarf shrub Salix Herbacea. Basic Appl. Ecol. 16, 202–209. doi: 10.1016/j.baae.2015.02.004
Wisz, M. S., Hijmans, R. J., Li, J., Peterson, A. T., Graham, C. H., and Guisan, A. (2008). Effects of sample size on the performance of species distribution models. Divers. Distrib. 14, 763–773. doi: 10.1111/j.1472-4642.2008.00482.x
Wu, J., and Porinchu, D. F. (2019). A high-resolution sedimentary charcoal- and geochemistry-based reconstruction of late Holocene fire regimes in the páramo of Chirripó National Park, Costa Rica. Quat. Res. 93, 314–329.
Wu, X., Islam, A. S. M. F., Limpot, N., Mackasmiel, L., Mierzwa, J., Cortés, A. J., et al. (2020). Genome-wide snp identification and association mapping for seed mineral concentration in mung bean (Vigna radiata L.). Front. Genet. 11:656. doi: 10.3389/fgene.2020.00656
Yackulc, C. B., Nichols, J. D., Reid, J., and Der, R. (2015). To predict the niche, model colonization and extinction. Ecology 96, 16–23. doi: 10.1890/14-1361.1
Young, K. R., and Duchicela, S. (2020). Abandoning holocene dreams: proactive biodiversity conservation in a changing world. Ann. Am. Assoc. Geogr. 1–9. doi: 10.1080/24694452.2020.1785833
Zellweger, F., De Frenne, P., Lenoir, J., Rocchini, D., and Coomes, D. (2019). Advances in microclimate ecology arising from remote sensing. Trends Ecol. Evol. 34, 327–341. doi: 10.1016/j.tree.2018.12.012
Zellweger, F., De Frenne, P., Lenoir, J., Vangansbeke, P., Verheyen, K., Bernhardt-Römermann, M., et al. (2020). Forest microclimate dynamics drive plant responses to warming. Science 368, 772–775.
Zeng, Y., Low, B. W., and Yeo, D. C. J. (2016). Novel methods to select environmental variables in maxent: a case study using invasive crayfish. Ecol. Model. 341, 5–13. doi: 10.1016/j.ecolmodel.2016.09.019
Keywords: Neotropical alpine region, biodiversity hotspots, species distribution modeling, niche conservatism, range shifts, adaptation, Espeletiinae Cuatrec. (Asteraceae)
Citation: Valencia JB, Mesa J, León JG, Madriñán S and Cortés AJ (2020) Climate Vulnerability Assessment of the Espeletia Complex on Páramo Sky Islands in the Northern Andes. Front. Ecol. Evol. 8:565708. doi: 10.3389/fevo.2020.565708
Received: 26 May 2020; Accepted: 26 August 2020;
Published: 24 September 2020.
Edited by:
Domenico Gargano, University of Calabria, ItalyReviewed by:
Rodolfo Gentili, University of Milano-Bicocca, ItalyCopyright © 2020 Valencia, Mesa, León, Madriñán and Cortés. This is an open-access article distributed under the terms of the Creative Commons Attribution License (CC BY). The use, distribution or reproduction in other forums is permitted, provided the original author(s) and the copyright owner(s) are credited and that the original publication in this journal is cited, in accordance with accepted academic practice. No use, distribution or reproduction is permitted which does not comply with these terms.
*Correspondence: Andrés J. Cortés, YWNvcnRlc0BhZ3Jvc2F2aWEuY28=
†These authors share senior authorship
Disclaimer: All claims expressed in this article are solely those of the authors and do not necessarily represent those of their affiliated organizations, or those of the publisher, the editors and the reviewers. Any product that may be evaluated in this article or claim that may be made by its manufacturer is not guaranteed or endorsed by the publisher.
Research integrity at Frontiers
Learn more about the work of our research integrity team to safeguard the quality of each article we publish.