- 1Department of Geography, The University of Utah, Salt Lake City, UT, United States
- 2Department of Geography, University of Oregon, Eugene, OR, United States
Paleoecological investigations using the pollen-plant functional trait linkage are increasing in value as new insights to past ecological function and dynamics are revealed. These retrospective approaches link pollen sequences to plant functional trait measurements to reveal long-term changes in ecosystem properties that are difficult to resolve using traditional paleoecological methods. Despite these methodological improvements and the newfound perspectives, there has yet to be thorough testing of whether transforming pollen to ecological function tracks functional trait distributions in geographic space. We assess this in North America by linking surface pollen samples to measurements of three functional traits that represent major axes of plant ecological strategy. Pollen-derived estimates of function were first used to investigate occupied trait space at different scales. These estimates were used to reconstruct the latitudinal functional diversity gradient of North America, and results were compared to the continent’s functional diversity gradient estimated from tree assemblages and gradients based on pollen richness and evenness. Results indicated that the patterns in pollen-based function sufficiently track ecological function in trait and geographic space and the macroecological biodiversity pattern was reconstructed, although there were minor differences between the slopes of the functional diversity and each of the pollen index gradients. Taken together, the outcomes of our investigation indicate reliability in extending the pollen-plant functional trait linkage into deeper time, at least for examining North American functional dynamics.
Introduction
Sediment-based sub-fossil pollen is frequently used as a proxy for local and regional changes in vegetation composition over time (Faegri and Iversen, 1989). The application of pollen in paleoecological analysis has been to categorize “who was there” through compositional changes and presence and absence in time (Dodd and Stanton, 1990), with infrequent interpretations of changes in ecosystem function. Yet, these data contain untapped potential. A new tool emerging in paleoecology focuses on measuring ecosystem function by applying ecological theory of modern plant floristics to paleoecological records. Ecosystem function (ecosystem property), or the biological, geochemical, and physical processes that take place within an ecosystem, are those that reflect the capacity of ecosystems to fulfill human needs (ecosystem service) (de Groot et al., 2002). Analysis of long-term functional responses to environmental change, by linking plant functional traits to fossil pollen assemblages, sheds new light on past ecosystem dynamics and provides complementary information to taxonomic-based analyses. Novel interpretations of past functional dynamics that are not typically obtainable from conventional paleoecological methods (Wingard et al., 2017) are increasing in value as a consequence of making the pollen-plant functional trait linkage.
Plant functional traits are defined as any morpho-, physio-, and phenological plant characteristics affecting overall plant fitness through their influence on survival, growth, and reproduction (Violle et al., 2007). These plant characteristics are biological adaptations to the environment and represent fundamental tradeoffs between individual species, as they direct the acquisition, processing, and investment of resources in plants (Chapin et al., 2000). Variations in functional traits impact ecosystem properties (e.g., biologic diversity, cycling of nutrients, soil characteristics) (Hooper et al., 2005) as species respond to and are filtered by climate, disturbance, and biotic interactions (Lavorel and Garnier, 2002). Plant functional trait data, in the form of species-level trait measurements, are increasingly accessible from large databases, such as the Botanical Information and Ecology Network (BIEN Database1; Enquist et al., 2016) and the TRY Plant Trait Database2 (Kattge et al., 2020). Conceptual developments in functional trait theory have led to the identification of a wide variety of plant traits linked to various aspects of ecosystem function and processes (Pérez-Harguindeguy et al., 2013).
Early plant functional trait theory identified three traits as the major axes of plant strategies governing ecosystem function (i.e., plant height, seed mass, leaf area) (Westoby, 1998). These indicators of plant fitness were recently found to occupy primary axes of global plant form and function (Díaz et al., 2016) and were used to identify the latitudinal functional diversity gradient (Lamanna et al., 2014). Tall plant heights are advantageous for dispersing seed and intercepting light (Gaudet and Keddy, 1988; Westoby, 1998; Westoby et al., 2002; Muller-Landau et al., 2008; Moles et al., 2009), although construction and maintenance costs are incurred along with higher risks of breaking (Niklas, 1993; Poorter et al., 2008). High leaf area is advantageous for increasing photosynthetic capacity through capturing light at the expense of increased construction costs and produces large boundary layers responsible for reducing transpiration rates and thus heat exchange and carbon dioxide diffusion to the surrounding air (Díaz et al., 2016). Low seed mass is advantageous for dispersal and colonization ability. Seeds with low weights are generally produced in large numbers that can be dispersed farther, thus allowing for establishing a persistent seed bank, and conversely, high seed mass is advantageous for surviving early recruitment stages and may offer a passive dispersal advantage depending on if and whether fruit is formed (Leishman and Westoby, 1994; Westoby et al., 2002; Muller-Landau, 2010; Thomson et al., 2011; Ben-Hur et al., 2012).
The central tenet of functional analysis is that ecosystem properties can be evaluated through the procedure of classifying plant species by their functional characteristics, information typically unavailable when examining shifts in taxonomic abundances alone (Diaz and Cabido, 2001). Classifying species by their functional characteristics has primarily been an exercise of ecological studies based on direct observation and botanical surveys (e.g., Mccormack et al., 2012; Enright et al., 2014) although the application of plant functional trait theory to remotely sensed imagery is growing (Jetz et al., 2016). In paleoecological contexts, plant functional traits have been previously linked to pollen records to model macro-scale changes in past terrestrial ecosystems making use of broad taxonomic classifications identifying plant functional types (Prentice et al., 1996; Williams et al., 2001, 2004; Marchant et al., 2010; Ni et al., 2010). Functional paleoecology, although in its infancy, links plant functional traits to pollen taxa and transforms pollen percentages (i.e., relative abundances) to represent ecological function variations through space and time. Doing so helps to bridge the gap in paleoecological and neontological analysis (Fritz et al., 2013) and can provide new perspectives of past ecosystem change resulting from biotic and abiotic interactions at various spatial and temporal scales.
Although currently sparse, the field of functional paleoecology is growing. Recent applications have shown the effectiveness of making the pollen-plant functional trait linkage, primarily assessing functional responses to climate variations. Evaluating modern geographic trait distributions related to climate in the European Mediterranean region, Barboni et al. (2004) showed plant functional traits (e.g., life form: tree, shrub; leaf traits: type, size, waxiness; plant traits: leaf phenology: thorniness, phenology) were related to either plant water availability or mean temperature of the coldest month, or a combination of the two. Knight et al. (2020) showed pollen assemblage responses to changing climate were highly variable through the Quaternary in eastern North America and high seed mass within assemblages generally resulted in more matches with climate. Methods for estimating long-term functional trait compositions in fen peatland communities were developed based on the best-fit linear mixed-effects modeling of leaf traits linked to modern pollen samples that acted as a filter for extending back in time (Carvalho et al., 2019). Neogene and Quaternary tree species in eastern North America were found to occupy similar albeit more densely packed trait space compared to Europe despite greater species richness (Ordonez and Svenning, 2018). Within the same time periods, Ordonez and Svenning (2020) developed a conceptual framework for understanding the legacies of environmental changes on ecosystem processes; species diversity variations via response traits were shown to have the potential to impact effect traits that govern ecosystem processes. A regional study on the relationships between pollen-derived function, climate, and human influences in Estonia and Latvia showed cold late-glacial climates were associated with low plant heights as well as small seeds and low leaf area (Reitalu et al., 2015). The transition to a progressively warmer Holocene climate led to taller vegetation with larger seeds and greater leaf area and consequently, functional diversity increased through time.
Functional relationships to disturbances have also been investigated. An analysis of functional trait variations in the Pacific Northwest, United States showed how increased Holocene fire activity led to changes in community-level fire adaptation and structure (Brussel et al., 2018). Site-level functional diversity and overlap estimates provided evidence of an ecosystem shift from seral to stable aspen (Morris et al., 2019). In the Amazon, the effects of precipitation variation and human-caused environmental changes on community traits were estimated and indicated plant functional traits responded more clearly to drivers than pollen taxa, and different anthropogenic disturbances, e.g., erosion and fire, increased the dominance of distinct functional traits (van der Sande et al., 2019).
The utility of making pollen-plant functional trait linkages requires evaluating how well transforming pollen data to ecological function captures the spatial distribution of modern functional traits. The overarching question we work to address is, does the pollen-plant functional trait linkage represent ecological function in North America? We test the linkage by integrating surface pollen samples from the Neotoma Paleoecology Database (Williams et al., 2018) and plant functional traits from the BIEN Database (Enquist et al., 2016). Functional trait data representing primary axes of plant strategy are used to assess spatial relationships between the pollen-plant functional trait linkage (i.e., estimates of site-level function via individual traits) and the functional trait data used to inform these pollen-derived values. We then use these functional estimates from North American sites to quantify functional diversity, the biodiversity metric that governs ecosystem function, and therefore the ability of ecosystems to provide services for humans (Diaz et al., 2007). To present a macroecological example of the utility of making the pollen-plant functional linkage, we compared pollen-based functional diversity estimates to those from modern tree assemblages in the form of latitudinal functional diversity gradient, a pattern found to be embedded within the latitudinal biodiversity gradient for the western hemisphere (i.e., North and South America) (Lamanna et al., 2014). We hypothesize that pollen-derived functional diversity estimates will corroborate the recently observed latitudinal gradient in functional diversity of North America, as there is a non-linear relationship between plants and pollen. The pollen-based functional diversity gradient is next compared to gradients in the richness and evenness of pollen taxa through latitude, as these correspond to measures of the number and distribution of species abundances, respectively. We then briefly discuss potential avenues for investigating biogeographic questions that are difficult to resolve by employing traditional paleoecological methods.
Materials and Methods
Pollen Data and Plant Functional Trait Measurements
The Neotoma Paleoecology Database (accessed November 2019) was queried for North American surface pollen samples using the R package “neotoma” version 1.7.2 (Goring et al., 2015; Williams et al., 2018) returning pollen sequences for 3033 sites. Each pollen count dataset with standard taxonomies was converted to pollen percentages for tree and herb taxa. Sample density was low in Mexico, west of the United States’ Rocky Mountains, the west coast of Canada and southern Alaska. A request for trait measurements of leaf area, plant height, and seed mass from the BIEN Database (R package “bien” version 4.1.1, Enquist et al., 2016; Maitner et al., 2017) returned over 10 million standardized trait observations. The list of species with trait measurements was restricted to only those that are native to continental North America (Mexico, United States, and Canada) in a multi-step process. A list of standardized plant species native to the United States and Canada was obtained from the PLANTS Database (USDA and NRCS, 2019) and species lists of native and introduced plants of Mexico were obtained (Villaseñor and Espinosa-Garcia, 2004; Villaseñor, 2016), which were then combined. Plants of the World Online3 was consulted to reduce the list of species with trait measurements to only those plant species that are native to the entire continent. If a species was listed as introduced to Mexico, but native to the United States or Canada, the species was retained. Conversely, if a species was listed as introduced to one of the countries but not designated as a native species in one of the others, it was removed from the list. The final data set for North America included 9,487,396 plant height measurements from 2,146 species, 13,103 leaf area measurements from 1,016 species, and 16,621 seed mass measurements from 3,580 species.
Pollen Taxa X Species Functional Trait Matrix
Following the identification of functional trait measurements for plant species native to North America, the species within the list were then linked to their respective pollen taxonomies (i.e., species-, genus-, and family-level pollen types) by constructing a species with trait measurements X pollen taxon matrix. The “taxize” R package version 0.7.5 (Chamberlain et al., 2016) allows for retrieving both higher and lower standardized taxonomies by accessing different databases that house these datasets, and so the National Center for Biotechnology Information (NCBI) and Integrated Taxonomic Information System (ITIS) databases were employed to link individual species to their respective taxonomies. The surface pollen dataset contains pollen grains varying in taxonomic resolution. Some pollen types are identified to the species level and others to the pollen-type level, such that they could be linked to multiple species (e.g., Acer spicatum, Acer undifferentiated). We opted to retain the original pollen taxonomy as identified by pollen analysts that were submitted to the Neotoma Paleoecology Database (Williams et al., 2018), opposed to harmonizing pollen taxa to standardize pollen types (see, e.g., Giesecke et al., 2019). This makes the assumption pollen analysts use an informed approach to identify pollen types to the species level (e.g., local species presence surrounding a depositional environment; a regional absence of any other species; geographic context). Doing so maximizes the amount of data that was used to inform trait value estimates while also estimating the most precise trait values.
Trait measurements were log-transformed and scaled to normalize and standardize distributions. We then assigned values for the three traits to each pollen taxon. As the majority of pollen taxa are associated with multiple observations in the BIEN Database, due to repeated measurements or from linking lower taxonomic resolution pollen taxa to species names, we represent the possible range of functional trait values for each taxon as the mean and standard deviation of all associated values. Remarkable efforts have been put forward to catalog plant functional traits and changes in these through time (Meeus, 2018; Meineke et al., 2018; Kissling et al., 2019). However, and because there has yet to be a comprehensive list of measurements for species-level functional traits across different geographic localities compiled, we assume these values are stationary across space. The result was a list of pollen taxa and the mean and standard deviation of their trait values (Supplementary Table 1).
Functional Diversity Estimates
Functional diversity estimates were calculated using multidimensional hypervolumes that were based on the set of trait values for each pollen taxon at each surface pollen site using box kernel density estimations in the R package “hypervolume” version 2.0.8 (Blonder et al., 2018). These estimates quantify the range of occupied trait space of a set of functional traits at each pollen sample, consistent with a measure of functional richness (Villéger et al., 2008) and correspond to a realized niche (Hutchinson, 1957). To quantify accurate representations of functional diversity on the landscape, we opted to use only surface sample sites that had all traits quantified for no less than five pollen taxa (i.e., each pollen taxon had a trait value estimated in earlier steps), and from these, only those taxa with pollen percentages greater than 0.5%. Retaining only those pollen taxa that had estimates for each trait and sites that had no less than five pollen taxon level functional estimates strives to represent accurate site-scale functional diversity estimates. The threshold is used to restrict pollen species that may not be present on the landscape from diversity estimates. To represent the uncertainty in the trait assignments, we used a Monte Carlo approach, where at each site, we resample a trait value for each taxon from a normal distribution defined by the mean and standard deviation described above, and then calculate the hypervolume of each surface sample. This is then repeated 500 times with the result as pollen-derived, occupied trait space at each site measured in standard deviations (Blonder et al., 2018).
The set of functional diversity estimates were then gridded at a 0.5° resolution by taking the median values of the full set of Monte Carlo estimates for all sites within each grid cell, resulting in 828 gridded functional diversity estimates. Doing so minimizes variability that may have arisen from inconsistent pollen sampling spatially and taxonomically. These gridded functional diversity estimates were then used to reconstruct the latitudinal functional diversity gradient (Lamanna et al., 2014) by estimating a linear model against latitude (Supplementary Table 2).
Comparisons: Pollen-Derived Function and BIEN Data; Latitudinal Functional Diversity Gradient and Pollen Indices
To identify the capacity of the pollen-plant functional trait linkage to accurately represent ecological function, we compared the occupied trait space (i.e., range of functional estimates) of those sites that had hypervolumes quantified, the subset of plant species that are native to North America from the BIEN Database (Enquist et al., 2016) that were used for informing functional diversity estimations, and also just the BIEN Database’s North American tree species. We also made comparisons between the North American latitudinal functional diversity gradients estimated from the full set of terrestrial pollen taxa and tree assemblages (Lamanna et al., 2014), and pollen-based richness and evenness gradients. Constituents of the functional diversity gradient of the western hemisphere, the estimates from tree assemblages were quantified based on the presence of tree species with diameters at breast height > 10 cm in 0.1 ha plots (see Lamanna et al., 2014 for their detailed methods). We limited their dataset to only functional diversity estimates of North America. Evenness was calculated as the slope of the rank-order versus log-abundance curve for each surface pollen sample, and richness was calculated using rarefaction to 500 pollen grain counts (Giesecke et al., 2014). Each surface sample’s richness and evenness estimates were gridded as previously described and all pollen-based estimates were mapped against the continent’s Level 1 ecoregions (Omernik, 1987). Generalized least squares regression was used to estimate all latitudinal gradients, including trends in the traits themselves. An exponential spatial covariance function was incorporated into each model to account for spatial dependency (Supplementary Table 2). Table 1 reports the model coefficients and the results of Moran’s I tests for residual spatial autocorrelation. Pollen-based functional diversity, richness, and evenness were correlated using Pearson’s product-moment correlation with the R package “Hmisc” (Harrell, 2004).

Table 1. Outcomes of generalized least squares models of functional traits, functional diversity, and pollen evenness and richness against latitude in North America.
Results and Discussion
Comparison of Pollen-Based Trait Space and Ecological Datasets
As a first test of the pollen-plant functional trait linkage, we compare the trait space of North America derived from pollen samples to the full list of BIEN trait measurements for native species and a subset of the measurements for native trees (Enquist et al., 2016). Compared to the full list of native BIEN species functional trait measurements, all three aspects of pollen-based function occupy the upper ranges of the BIEN data. The pollen-plant functional trait linkage collapses the spread of interquartile ranges of all three traits with few outliers (Figure 1). As related to a subset of BIEN Database tree species, pollen-derived values for leaf area were lower and seed mass values were matched more similarly. We thus posit linking surface pollen samples to plant functional traits reconstructs ecological function, such that the method may be carried forward for further analysis and into recent geologic time. Further, these results allow for an ability to examine the dynamics of ecological function in addition to taxonomic composition.
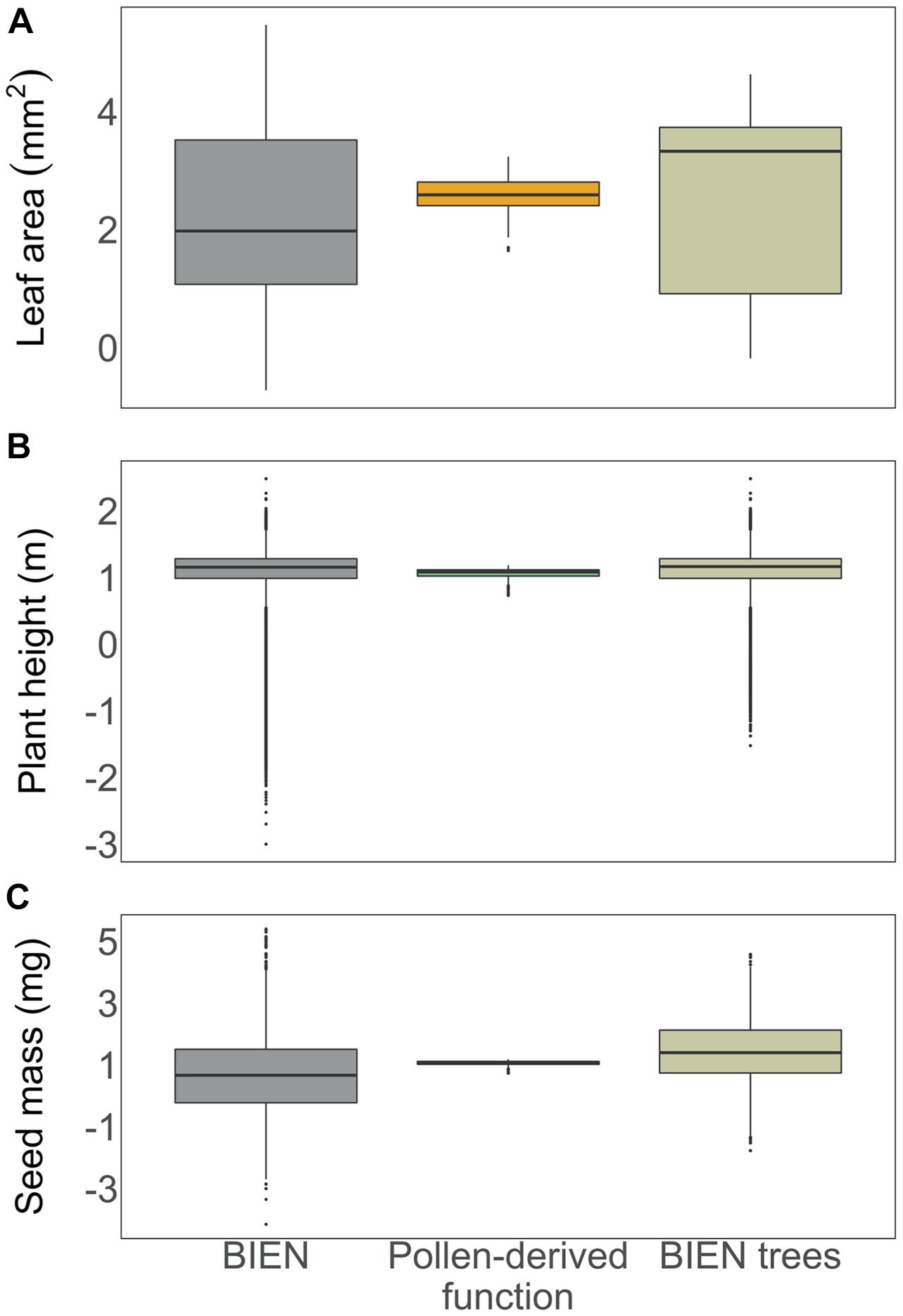
Figure 1. Comparisons between the trait spaces of leaf area (A), plant height (B), and seed mass (C) from the list of trait measurements for species native to North America from the BIEN Database (Enquist et al., 2016), surface pollen samples (Williams et al., 2018), and native tree species (Enquist et al., 2016). Ranges of functional estimates from the pollen-plant functional trait linkage were generated using median trait values in each 0.5° × 0.5° grid cell. All traits were log-transformed. See Supplementary Table 1 for pollen-based trait values.
Spatial Patterns of the Pollen-Plant Functional Trait Linkage
The distribution of pollen-derived ecological function in geographic space is more complicated, but a useful comparison can be made at the scale of ecoregions (Omernik, 1987). Leaf area values were highest in the Eastern Temperate Forest and the eastern margins of the Great Plains (Figure 2A). Leaves with the smallest area were found primarily in the southern ranges of Northwestern Forested Mountains and the Marine West Coast Forest, ecoregions dominated by coniferous trees, and North American Deserts, where small leaves are advantageous for limiting water loss during transpiration. Pollen-derived leaf area was intermediate in high latitude ecoregions, where temperate coniferous trees dominate and smaller leaf area would be expected.
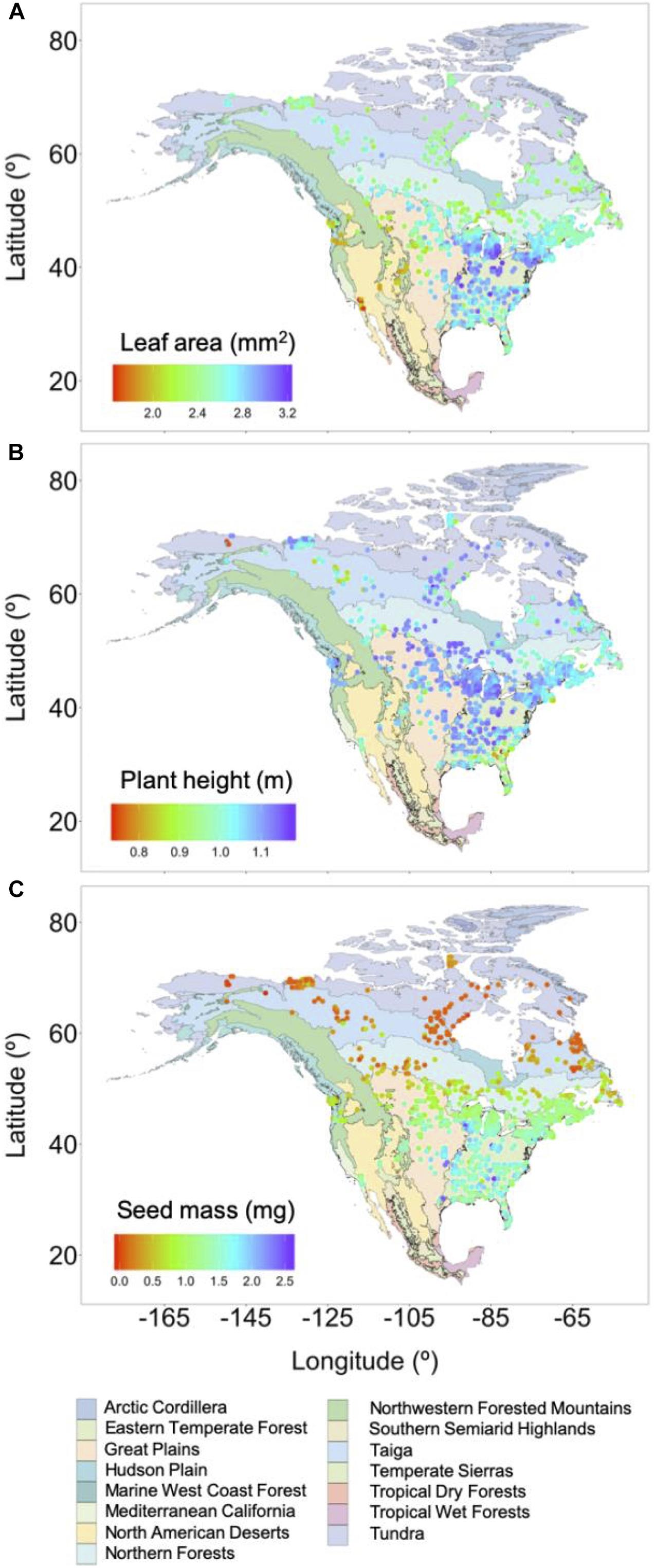
Figure 2. Gridded and log-transformed leaf area (A), plant height (B), and seed mass (C) estimates from surface pollen samples against a backdrop of the continent’s ecoregions (Omernik, 1987) with mean grid cell values colored on a log scale. Generalized least squares regression found the seed mass trend was significantly related to latitude.
The pollen-plant functional trait linkage indicated most tree vegetation occupies the mid- to upper- ranges of pollen-derived plant height trait space (Figure 2B). The shortest plant heights were found generally at the southern extent of the Eastern Temperate Forest and northern Alaska. The general consistency of plant height through geographic space in North America suggests competition for light is generally low. A different climatic forcing, potentially water availability through precipitation in the wettest month, may govern the spatial distribution of plant heights in North America (Moles et al., 2009).
The continent’s spatial distribution of seed mass estimates from pollen showed a largely coherent latitudinal trend (Moles and Westoby, 2003) (Figure 2C and Supplementary Figure 1), as this was the sole pollen-based functional trait to decline significantly with movement toward the pole (Table 1). Heavy seeds were found at southern latitudes, and with movement to the north, seeds became lighter across all ecoregions. Plants bearing seeds with light weights are expected to invest resources for producing large numbers of seeds that can disperse farther distances and also develop persistent seed banks. Low seed mass values at high latitudes may suggest environmental controls (e.g., the timing of spring snowmelt, poor soil conditions, and frequent fires) influence plants to establish and grow rapidly once an opportunity arises. Greater seed weights in southern latitudes indicate longer-term investments to capitalize on canopy openings when opportunities arise.
Post-glacial Dispersal as an Interpretation for the Spatial Patterns of Seed Mass
Many examples have shown individual tree species migrated from southern latitudes at different rates in post-glacial North America and Europe (Davis, 1976; Cwynar and MacDonald, 1987; Webb, 1987; Birks, 1989). It is generally accepted that periodic, long-distance dispersal characterizes colonization and range expansion events from the late-glacial to present (Cain et al., 1998; Clark, 1998). Considering this, the nearly systematic decline in seed mass through latitude (Figure 2C and Supplementary Figure 1) may be a result of functional selection that occurred from the late-glacial period to modern day, opposed to species selection. Cwynar and MacDonald (1987) showed directional selection for lighter seeds of Pinus contorta spp. latifolia as the species migrated in post-glacial western Canada; as new populations established northward, allelic diversity decreased and seeds became more readily dispersible. With the modern distribution of seed mass in North America, and the results of Cwynar and MacDonald (1987), we provisionally suggest the consistent trend in seed mass may be a factor of directional selection, such that species with low seed weights at high latitudes may have been under the strongest selection for light seeds in post-glacial North America. To make this conclusion, though, would require continued phylogenetic investigations of post-glacial migrations (Abbott et al., 2000; Griffin and Barrett, 2004) yet the potential for range expansion and colonization via birds assisting in seed dispersal in post-glacial North America may be a constraint (Porter, 1984). Another potential cause for the seed mass gradient, which may be either independent of or entwined within the notion of functional selection, is the prospect of a macroscale driver such as temperature, precipitation, or insolation (Lacourse, 2009; Knight et al., 2020).
Macroecological Application Using the Pollen-Plant Functional Trait Linkage
We used estimates of pollen-based function to quantify functional diversity for reconstructing the latitudinal functional diversity gradient of North America. This macroecological pattern was recently found to coincide with the latitudinal biodiversity gradient and showed that functional diversity in the western hemisphere trees declined with increasing latitude, supporting environmental filtering theory (Lamanna et al., 2014). We hypothesized the functional diversity gradient estimated from surface pollen samples would exhibit a similar slope compared to that estimated from modern tree assemblages (Lamanna et al., 2014). Estimated from surface pollen samples, the latitudinal functional diversity gradient exhibited a stronger slope compared to the gradient estimated from tree assemblages (Figure 3A and Table 1, slope from surface pollen samples = −1.251, slope from tree assemblages = −0.2; Lamanna et al., 2014).
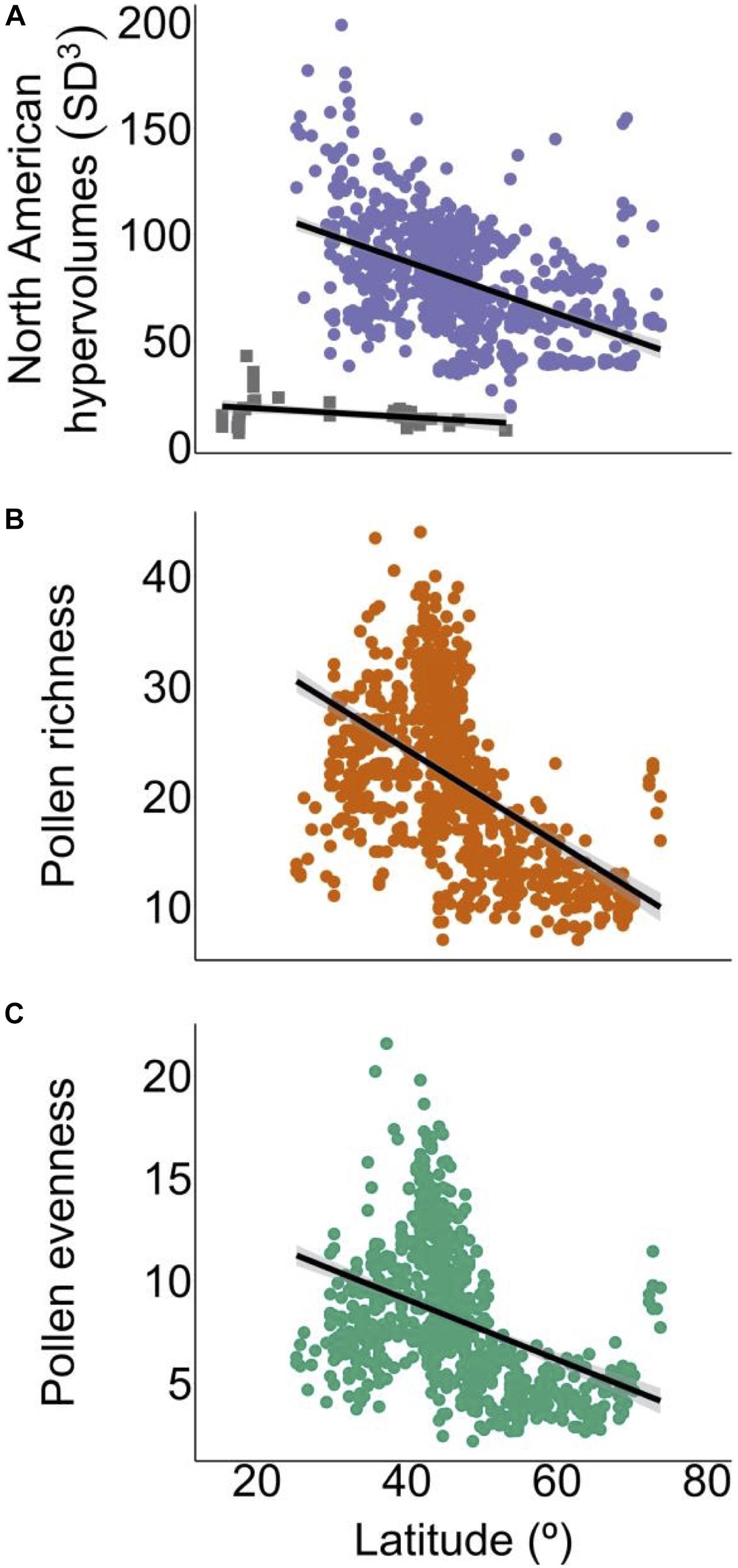
Figure 3. The North American functional diversity gradient estimated from surface pollen samples (purple points, estimated in this study based on data from the Neotoma Paleoecology Database, Williams et al., 2018) and tree assemblages (gray points, Lamanna et al., 2014) (A), with pollen indices of richness (B) and evenness (C). Slopes of each gradient were identified using linear modeling (black lines). Regressions indicated the functional diversity (A) and pollen richness (B) gradients were significantly related to latitude.
The discrepancies in slopes may result from several differences between the studies and datasets used. The reference study we made comparisons to Lamanna et al. (2014) quantified functional diversity based on trees sampled in 0.1 ha plots numbering 47 in North America from the latitudinal range of 15°–53° N with patchy longitudinal coverage. Our estimations were based on a wider set of terrestrial taxa (trees and herbs) that convey vegetation composition effectively sampled in larger areas (Jacobson and Bradshaw, 1981) from 3033 surface pollen samples that after gridding resulted in 828 functional diversity estimates ranging a broader latitudinal transect (25.25°–73.75° N). Individually or combinedly, these differences in estimating the functional diversity gradient may have resulted in wider occupied trait space in grid cells and thus a steeper functional diversity gradient slope. Including both tree and herbaceous pollen types may reflect site openness. Pollen-based trait values for functional diversity estimates and relationships between can be found in Supplementary Table 1 and Supplementary Figure 2. We recommend the criteria employed herein for future investigations of functional diversity dynamics in paleocontexts.
Gradients in Functional Diversity and Pollen Species Richness, Evenness
Following comparisons of the latitudinal functional diversity gradients estimated from surface pollen samples and tree assemblages (Lamanna et al., 2014), we evaluated relationships between pollen-based gradients in functional diversity, richness and evenness (Figures 3, 4). Pollen richness approximates the number of pollen taxa that would be expected using a standardized pollen count. Pollen evenness describes the potential dominance of pollen taxa within an ecosystem, with low evenness suggesting ecosystem function being driven by lower numbers of pollen taxa. Slopes of both pollen indices declined through latitude like that of the functional diversity gradient, suggesting ecosystem function is dominated by high species numbers at low latitudes and low species numbers at high latitudes (Figures 3, 4). Surprisingly, while pollen richness had a significant relationship with latitude, pollen evenness did not, once spatial autocorrelation was accounted for Table 1. As the calculation of evenness incorporates pollen abundances, this may bias the result due to the non-linear representation of plant abundances.
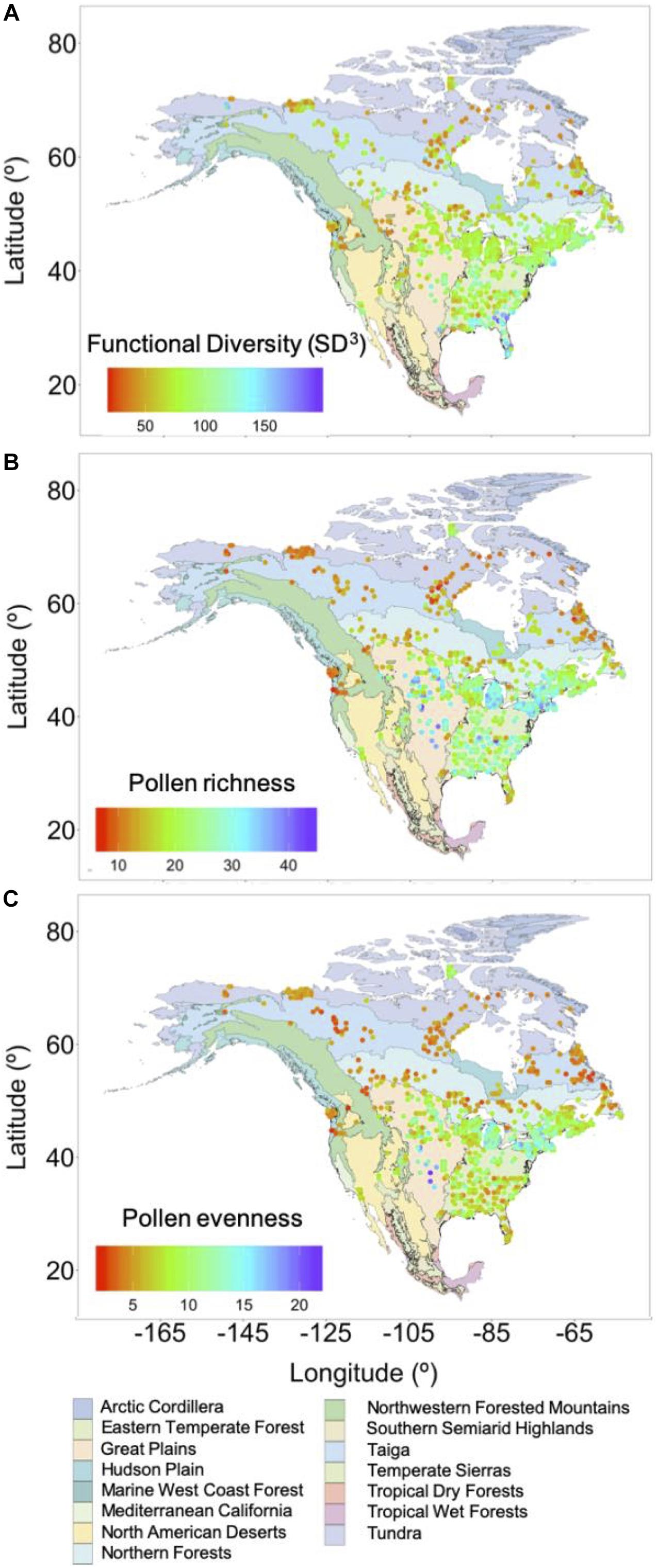
Figure 4. Estimates of functional diversity (A), pollen richness (B), and pollen evenness (C) estimated from surface pollen samples presented as the mean values of all estimates within a grid cell plotted in relation to different ecoregions of North America (Omernik, 1987).
Amongst the three pollen-based estimates, Pearson’s product-moment correlation coefficients indicated significant but weak correlations between functional diversity, and pollen richness and evenness estimates (richness: r = 0.36, P < 0.001; evenness: r = 0.31, P < 0.001). These results are contrary to what might be presumed regarding species richness and correspondingly, the dominance of individual pollen taxa within ecosystems as they relate to the range of function within ecosystems along latitudinal gradients (e.g., Lamanna et al., 2014). Instead, weak correlations suggest there may be different drivers of each gradient, for instance, environmental constraints (Klopfer and MacArthur, 1961; Janzen, 1967; Kerkhoff et al., 2014) or competition (Dobzhansky, 1950; Tilman et al., 1997).
When looking to functional diversity estimates and their values in each ecoregion, significant differences in ecoregion’s hypervolumes generally occurred between ecoregions with large geographic distance between them (Supplementary Table 3). Median functional diversity varied by ecoregion with the highest and lowest average hypervolumes found in Tropical Wet Forests (median hypervolume = 147.0) and Arctic Cordillera (median hypervolume = 45.4; the ecoregion with the most outliers was Eastern Temperate Forests that also had the highest number of surface samples (Supplementary Figure 3).
Future Directions Using the Pollen-Plant Functional Trait Linkage
The analysis of pollen-based functional trait space in principle would entail the use of georeferenced functional trait measurements, although there is a dearth of these datasets. As such, we estimated pollen-based functional traits and diversity based on all species that belong to a pollen taxon notwithstanding variation in functional trait variation through geographic space, which may influence these estimates (e.g., low variation in pollen-derived plant height). Future research may look to incorporate plant species distributions in large-scale (i.e., continental) analyses of ecosystem function, to constrain the trait measurements associated with any pollen assemblage.
Given suitable functional datasets, the pollen-plant functional trait linkage can be applied to capture enhanced explanations of ecological, evolutionary, and biodiversity dynamics, and place ecological theory based on modern plant floristics into the dynamic perspective of long-term analysis that was previously difficult to obtain. Many of these aspects are aligned with priority research questions for the paleoecological community (Seddon et al., 2014) and include complex community-level processes such as competition and succession, and also ecosystem processes like decomposition and biogeochemical cycling. Past ecosystem services can also be explored, such as the capacity to provide climate regulation, pollination, or soil stability. Gaining these new perspectives of long-term, ecosystem function changes provides a new route by which paleoecological analysis can contribute to sustainable conservations and management (Willis et al., 2007). The prospective assessment of functional responses to natural ranges of climate variability, disturbances, and anthropogenic pressure at varying spatiotemporal scales, especially in comparison to modern landscape changes, offers a new avenue of using the past to understand how the future of terrestrial ecosystems may unravel.
Conclusion
The focus of our investigation was to determine if pollen-derived aspects of ecological function track functional trait distributions in North America. Results showed that pollen-based function sufficiently tracks ecological function at the continental-scale on account of site-level estimates. At large-regional scales, the analysis shows strong linkages with pollen-inferred ecological function across a latitudinal gradient of functional diversity, which also followed the latitudinal trends of pollen richness and evenness. When considered with the robust interpretations of functional paleoecological analyses to date, our results indicate the transformation of pollen abundances to ecological function can be extended to deeper time, at least for North American functional dynamics. Making pollen-plant functional trait linkages allows for new interpretations of spatiotemporal biogeographic dynamics beyond the observational record. Functional paleoecology provides new perspectives that have great potential for placing the field into important discussions on how current and future ecosystems should be conserved and managed.
Data Availability Statement
The surface pollen trait values (Supplementary Table 1) generated for this study are available at: https://doi.org/10.1594/PANGAEA.922040.
Author Contributions
TB and SB designed and performed the research. TB led the analysis and writing. Both authors contributed to writing the manuscript.
Conflict of Interest
The authors declare that the research was conducted in the absence of any commercial or financial relationships that could be construed as a potential conflict of interest.
Acknowledgments
The study has been supported by the Neotoma Paleoecology Database (http://neotomadb.org) and the Botanical Information and Ecology Network (BIEN) (http://bien.nceas.ucsb.edu/bien/). The work of the data contributors and stewards of Neotoma and BIEN is gratefully acknowledged.
Supplementary Material
The Supplementary Material for this article can be found online at: https://www.frontiersin.org/articles/10.3389/fevo.2020.564609/full#supplementary-material
Footnotes
References
Abbott, R. J., Smith, L. C., Milne, R. I., Crawford, R. M. M., Wolff, K., and Balfour, J. (2000). Molecular analysis of plant migration and refugia in the arctic. Science 289, 1343–1346. doi: 10.1126/science.289.5483.1343
Barboni, D., Harrison, S. P., Bartlein, P. J., Jalut, G., New, M., Prentice, I. C., et al. (2004). Relationships between plant traits and climate in the Mediterranean region: a pollen data analysis. J. Veg. Sci. 15, 635–646. doi: 10.1111/j.1654-1103.2004.tb02305.x
Ben-Hur, E., Fragman-Sapir, O., Hadas, R., Singer, A., and Kadmon, R. (2012). Functional trade-offs increase species diversity in experimental plant communities. Ecol. Lett. 15, 1276–1282. doi: 10.1111/j.1461-0248.2012.01850.x
Birks, H. J. B. (1989). Holocene isochrone maps and patterns of tree-spreading in the British Isles. J. Biogeogr. 16, 503–540. doi: 10.2307/2845208
Blonder, B., Morrow, C. B., Maitner, B., Harris, D. J., Lamanna, C., Violle, C., et al. (2018). New approaches for delineating n-dimensional hypervolumes. Methods Ecol. Evol. 9, 305–319. doi: 10.1111/2041-210X.12865
Brussel, T., Minckley, T. A., Brewer, S. C., and Long, C. J. (2018). Community-level functional interactions with fire track long-term structural development and fire adaptation. J. Veg. Sci. 29, 450–458. doi: 10.1111/jvs.12654
Cain, M. L., Damman, H., and Muir, A. (1998). Seed dispersal and the Holocene migration of woodland herbs. Ecol. Monogr. 68, 325–347. doi: 10.1890/0012-9615(1998)068[0325:sdathm]2.0.co;2
Carvalho, F., Brown, K. A., Waller, M. P., Bunting, M. J., Boom, A., and Leng, M. J. (2019). A method for reconstructing temporal changes in vegetation functional trait composition using Holocene pollen assemblages. PLoS One 14:e0216698. doi: 10.1371/journal.pone.0216698
Chamberlain, S., Szoecs, E., Foster, Z., Arendsee, Z., Boettiger, C., Ram, K., et al. (2016). taxize: Taxonomic Information From Around the Web. R Package Version 0.7.5. 2. Available online at: https://github.com/ropensci/taxize (accessed December, 2019).
Chapin, F. S., Zavaleta, E. S., Eviner, V. T., Naylor, R. L., Vitousek, P. M., Reynolds, H. L., et al. (2000). Consequences of changing biodiversity. Nature 405, 234–242. doi: 10.1038/35012241
Clark, J. S. (1998). Why trees migrate so fast: confronting theory with dispersal biology and the paleorecord. Am. Nat. 152, 204–224. doi: 10.1086/286162
Cwynar, L. C., and MacDonald, G. M. (1987). Geographical variation of Lodgepole Pine in relation to population history. Am. Nat. 129, 463–469. doi: 10.1086/284651
Davis, M. B. (1976). Pleistocene biogeography of temperate deciduous forests. Geosci. Man 13, 13–26.
de Groot, R. S., Wilson, M. A., and Boumans, R. M. J. (2002). A typology for the classification, description and valuation of ecosystem functions, goods and services. Ecol. Econ. 41, 393–408. doi: 10.1016/s0921-8009(02)00089-7
Diaz, S., and Cabido, M. (2001). Vive la différence: plant functional diversity matters to ecosystem processes. Trends Ecol. Evol. 16, 646–655. doi: 10.1016/S0169-5347(01)02283-2
Díaz, S., Kattge, J., Cornelissen, J. H. C., Wright, I. J., Lavorel, S., Dray, S., et al. (2016). The global spectrum of plant form and function. Nature 529, 167–171. doi: 10.1038/nature16489
Diaz, S., Lavorel, S., de Bello, F., Quetier, F., Grigulus, K., and Robson, T. M. (2007). Incorporating plant functional diversity effects in ecosystem service assessments. Proc. Natl. Acad. Sci. U.S.A. 104, 20684–20689. doi: 10.1073/pnas.0704716104
Dodd, J. R., and Stanton, R. J. (1990). Paleoecology: Concepts and Applications. New York, NY: John Wiley & Sons.
Enquist, B. J., Condit, R., Peet, R. K., Schildhauer, M., and Thiers, B. M. (2016). Cyberinfrastructure for an integrated botanical information network to investigate the ecological impacts of global climate change on plant biodiversity. PeerJ Prepr. 4:e2615v2.
Enright, N. J., Fontaine, J. B., Lamont, B. B., Miller, B. P., and Westcott, V. C. (2014). Resistance and resilience to changing climate and fire regime depend on plant functional traits. J. Ecol. 102, 1572–1581. doi: 10.1111/1365-2745.12306
Fritz, S. A., Schnitzler, J., Eronen, J. T., Hof, C., Böhning-Gaese, K., and Graham, C. H. (2013). Diversity in time and space: wanted dead and alive. Trends Ecol. Evol. 28, 509–516. doi: 10.1016/j.tree.2013.05.004
Gaudet, C. L., and Keddy, P. A. (1988). A comparative approach to predicting competitive ability from plant traits. Nature 334, 242–243. doi: 10.1038/334242a0
Giesecke, T., Ammann, B., and Brande, A. (2014). Palynological richness and evenness: insights from the taxa accumulation curve. Veg. Hist. Archaeobot. 23, 217–228. doi: 10.1007/s00334-014-0435-5
Giesecke, T., Wolters, S., van Leeuwen, J. F. N., van der Knaap, P. W. O., Leydet, M., and Brewer, S. (2019). Postglacial change of the floristic diversity gradient in Europe. Nat. Commun. 10:5422. doi: 10.1038/s41467-019-13233-y
Goring, S., Dawson, A., Simpson, G. L., Ram, K., Graham, R. W., Grimm, E. C., et al. (2015). neotoma: a programmatic interface to the Neotoma paleoecological database. OpenQuaternary 1, 1–17. doi: 10.5334/oq.ab
Griffin, S. R., and Barrett, S. C. H. (2004). Post-glacial history of Trillium grandiflorum (Melanthiaceae) in Eastern North America: inferences from phylogeography. Am. J. Bot. 91, 465–473. doi: 10.3732/ajb.91.3.465
Harrell, F. E. (2004). Hmisc S Function Library. Available online at: http://math.furman.edu/~dcs/courses/math47/R/library/Hmisc/html/Overview.html (accessed March, 2020).
Hooper, D. U., Chapin, F. S. III, and Ewel, J. J. (2005). Effects of biodiversity on ecosystem functioning: a consensus of current knowledge. Ecol. Monogr. 75, 3–35. doi: 10.1890/04-0922
Hutchinson, G. E. (1957). Concluding remarks. Cold Spring Harb. Symp. Quant. Biol. 22, 415–427. doi: 10.1101/SQB.1957.022.01.039
Jacobson, G., and Bradshaw, R. (1981). The selection of sites for paleovegetational studies. Quat. Res. 16, 80–96. doi: 10.1016/0033-5894(81)90129-0
Janzen, D. H. (1967). Why mountain passes are higher in the tropics. Am. Nat. 101, 233–249. doi: 10.1086/282487
Jetz, W., Cavender-Bares, J., Pavlick, R., Schimel, D., Davis, F. W., Asner, G. P., et al. (2016). Monitoring plant functional diversity from space. Nat. plants 2:16024. doi: 10.1038/nplants.2016.24
Kattge, J., Bönisch, G., Diìaz, S., Lavorel, S., Prentice, I. C., Leadley, P., et al. (2020). TRY plant trait database – enhanced coverage and open access. Glob. Chang. Biol. 26, 119–188. doi: 10.1111/gcb.14904
Kerkhoff, A. J., Moriarty, P. E., and Weiser, M. D. (2014). The latitudinal species richness gradient in New World woody angiosperms is consistent with the tropical conservatism hypothesis. Proc. Natl. Acad. Sci. U.S.A. 111, 8125–8130. doi: 10.1073/pnas.1308932111
Kissling, W. D., Balslev, H., Baker, W. J., Dransfield, J., Göldel, B., Lim, J. Y., et al. (2019). PalmTraits 1.0, a species-level functional trait database of palms worldwide. Sci. data 6:178. doi: 10.1038/s41597-019-0189-0
Klopfer, P. H., and MacArthur, R. H. (1961). On the causes of tropical species diversity: niche overlap. Am. Nat. 95, 223–226. doi: 10.1086/282179
Knight, C. A., Blois, J. L., Blonder, B., Macias-Fauria, M., Ordonez, A., and Svenning, J. C. (2020). Community assembly and climate mismatch in late quaternary eastern North American pollen assemblages. Am. Nat. 195, 166–180. doi: 10.1086/706340
Lacourse, T. (2009). Environmental change controls postglacial forest dynamics through interspecific differences in life-history traits. Ecology 90, 2149–2160. doi: 10.1890/08-1136.1
Lamanna, C. A., Blonder, B., Violle, C., Kraft, N. J. B., Sandel, B., Simova, I., et al. (2014). Functional trait space and the latitudinal diversity gradient. Proc. Natl. Acad. Sci. U.S.A. 111, 13745–13750. doi: 10.1073/pnas.1317722111
Lavorel, S., and Garnier, E. (2002). Predicting changes in community composition and ecosystem functioning from plant traits: revisiting the Holy Grail. Funct. Ecol. 16, 545–556. doi: 10.1046/j.1365-2435.2002.00664.x
Leishman, M. R., and Westoby, M. (1994). Hypotheses on seed size: tests using the semiarid flora of western New South Wales. Australia. Am. Nat. 143, 890–906. doi: 10.1086/285638
Maitner, B., Boyle, B., Casler, N., Condit, R., Donoghe, J. C., Duran, S. M., et al. (2017). The BIEN R package: a tool to access the Botanical Information and Ecology Network (BIEN) database. Methods Ecol. Evol. 9, 373–379. doi: 10.1111/2041-210X.12861
Marchant, R., Harrison, S. P., Hooghiemstra, H., Markgraf, V., van Boxel, J. H., Ager, T., et al. (2010). Pollen-based biome reconstructions for Latin America at 0, 6000 and 18 000 radiocarbon years ago. Clim. Past Discuss. 5, 725–767. doi: 10.5194/cp-5-725-2009
Mccormack, M. L., Adams, T. S., Smithwick, E. A. H., and Eissenstat, D. M. (2012). Predicting fine root lifespan from plant functional traits in temperate trees. New Phytol. 195, 823–831. doi: 10.1111/j.1469-8137.2012.04198.x
Meeus, S. (2018). Herbaria as functional trait databases. Biodivers. Inf. Sci. Stand. 2:e25766. doi: 10.3897/biss.2.25766
Meineke, E. K., Davis, C. C., and Davies, T. J. (2018). The unrealized potential of herbaria for global change biology. Ecol. Monogr. 88, 505–525. doi: 10.1002/ecm.1307
Moles, A. T., Warton, D. I., Warman, L., Swenson, N. G., Laffan, S. W., Zanne, A. E., et al. (2009). Global patterns in plant height. J. Ecol. 97, 923–932. doi: 10.1111/j.1365-2745.2009.01526.x
Moles, A. T., and Westoby, M. (2003). Latitude, seed predation and seed mass. J. Biogeogr. 30, 105–128. doi: 10.1046/j.1365-2699.2003.00781.x
Morris, J. L., Derose, R. J., Brussel, T., Brewer, S., Brunelle, A., and Long, J. N. (2019). Stable or seral? Fire-driven alternative states in aspen forests of western North America. Biol. Lett. 15:20190011. doi: 10.1098/rsbl.2019.0011
Muller-Landau, H. C. (2010). The tolerance-fecundity trade-off and the maintenance of diversity in seed size. Proc. Natl. Acad. Sci. U.S.A. 107, 4242–4247. doi: 10.1073/pnas.0911637107
Muller-Landau, H. C., Wright, S. J., Calderón, O., Condit, R., and Hubbell, S. P. (2008). Interspecific variation in primary seed dispersal in a tropical forest. J. Ecol. 96, 653–667. doi: 10.1111/j.1365-2745.2008.01399.x
Ni, J., Yu, G., Harrison, S. P., and Prentice, I. C. (2010). Palaeovegetation in China during the late Quaternary: Biome reconstructions based on a global scheme of plant functional types. Palaeogeogr. Palaeoclimatol. Palaeoecol. 289, 44–61. doi: 10.1016/j.palaeo.2010.02.008
Niklas, K. J. (1993). Influence of tissue density-specific mechanical properties on the scaling of plant height. Ann. Bot. 72, 173–179. doi: 10.1006/anbo.1993.1096
Omernik, J. M. (1987). Ecoregions of the conterminous United States. Map (scale 1:7,500,000). Ann. Assoc. Am. Geogr. 77, 118–125.
Ordonez, A., and Svenning, J. C. (2018). Greater tree species richness in eastern North America compared to Europe is coupled to denser, more clustered functional trait space filling, not to trait space expansion. Glob. Ecol. Biogeogr. 27, 1288–1299. doi: 10.1111/geb.12785
Ordonez, A., and Svenning, J. C. (2020). The potential role of species and functional composition in generating historical constraints on ecosystem processes. Glob. Ecol. Biogeogr. 29, 207–219. doi: 10.1111/geb.13033
Pérez-Harguindeguy, N., Díaz, S., Lavorel, S., Poorter, H., Jaureguiberry, P., Bret-Harte, M. S., et al. (2013). New handbook for standardized measurement of plant functional traits worldwide. Aust. J. Bot. 23, 167–234. doi: 10.1071/BT12225
Poorter, L., Wright, S. J., Paz, H., Ackerly, D. D., Condit, R., Ibarra-Manríquez, G., et al. (2008). Are functional traits good predictors of demographic rates? Evidence from five neotropical forests. Ecology 89, 1908–1920. doi: 10.1890/07-0207.1
Porter, D. M. (1984). Relationships of the Galapagos flora. Biol. J. Linn. Soc. 21, 243–251. doi: 10.1111/j.1095-8312.1984.tb02065.x
Prentice, C., Guiot, J., Huntley, B., Jolly, D., and Cheddadi, R. (1996). Reconstructing biomes from palaeoecological data: a general method and its application to European pollen data at 0 and 6 ka. Clim. Dyn. 12, 185–194. doi: 10.1007/BF00211617
Reitalu, T., Gerhold, P., Poska, A., Pärtel, M., Väli, V., and Veski, S. (2015). Novel insights into post-glacial vegetation change: functional and phylogenetic diversity in pollen records. J. Veg. Sci. 26, 911–922. doi: 10.1111/jvs.12300
Seddon, A. W. R., Mackay, A. W., Baker, A. G., Birks, H. J. B., Breman, E., Buck, C. E., et al. (2014). Looking forward through the past: identification of 50 priority research questions in palaeoecology. J. Ecol. 102, 256–267. doi: 10.1111/1365-2745.12195
Thomson, F. J., Moles, A. T., Auld, T. D., and Kingsford, R. T. (2011). Seed dispersal distance is more strongly correlated with plant height than with seed mass. J. Ecol. 99, 1299–1307. doi: 10.1111/j.1365-2745.2011.01867.x
Tilman, D., Lehman, C. L., and Thomson, K. T. (1997). Plant diversity and ecosystem productivity: theoretical considerations. Proc. Natl. Acad. Sci. U.S.A. 94, 1857–1861. doi: 10.1073/pnas.94.5.1857
van der Sande, M. T., Gosling, W., Correa-Metrio, A., Prado-Junior, J., Poorter, L., Oliveira, R. S., et al. (2019). A 7000-year history of changing plant trait composition in an Amazonian landscape; the role of humans and climate. Ecol. Lett. 22, 925–935. doi: 10.1111/ele.13251
Villaseñor, J. L. (2016). Checklist of the native vascular plants of Mexico. Rev. Mex. Biodivers. 87, 559–902. doi: 10.1016/j.rmb.2016.06.017
Villaseñor, J. L., and Espinosa-Garcia, F. J. (2004). The alien flowering plants of Mexico. Divers. Distrib. 10, 113–123. doi: 10.1111/j.1366-9516.2004.00059.x
Villéger, S., Mason, N. W. H., and Mouillot, D. (2008). New multidimensional functional diversity indices for a multifaceted framework in functional ecology. Ecology 89, 2290–2301. doi: 10.1890/07-1206.1
Violle, C., Navas, M. L., Vile, D., Kazakou, E., Fortunel, C., Hummel, I., et al. (2007). Let the concept of trait be functional! Oikos 116, 882–892. doi: 10.1111/j.2007.0030-1299.15559.x
Webb, S. L. (1987). Beech range extension and vegetation history: pollen stratigraphy of two Wisconsin lakes. Ecology 68, 1993–2005. doi: 10.2307/1939890
Westoby, M. (1998). A leaf-height-seed (LHS) plant ecology strategy scheme. Plant Soil 199, 213–227. doi: 10.1023/A:1004327224729
Westoby, M., Falster, D. S., Moles, A. T., Vesk, P. A., and Wright, I. J. (2002). Plant ecological strategies: some leading dimensions of variation between species. Annu. Rev. Ecol. Syst. 33, 125–159. doi: 10.1146/annurev.ecolsys.33.010802.150452
Williams, J. W., Grimm, E. C., Blois, J. L., Charles, D. F., Davis, E. B., Goring, S. J., et al. (2018). The neotoma paleoecology database, a multiproxy, international, community-curated data resource. Quat. Res. 89, 156–177. doi: 10.1017/qua.2017.105
Williams, J. W., Shuman, B. N., and Webb, T. (2001). Dissimilarity analyses of late-quaternary vegetation and climate in eastern North America. Ecology 82, 3346–3362. doi: 10.1890/0012-9658(2001)082[3346:daolqv]2.0.co;2
Williams, J. W., Shuman, B. N., Webb, T., Bartlein, P. J., and Leduc, P. L. (2004). Late-Quaternary vegetation dynamics in North America: scaling from taxa to biomes. Ecol. Monogr. 74, 309–334. doi: 10.1890/02-4045
Willis, K. J., Araújo, M. B., Bennett, K. D., Figueroa-Rangel, B., Froyd, C. a, and Myers, N. (2007). How can a knowledge of the past help to conserve the future? Biodiversity conservation and the relevance of long-term ecological studies. Philos. Trans. R. Soc. Lond. B Biol. Sci. 362, 175–186. doi: 10.1098/rstb.2006.1977
Keywords: ecological function, functional diversity, latitudinal functional diversity gradient, paleoecology, plant functional traits, pollen-derived function, pollen-plant functional trait linkage, trait space
Citation: Brussel T and Brewer SC (2021) Functional Paleoecology and the Pollen-Plant Functional Trait Linkage. Front. Ecol. Evol. 8:564609. doi: 10.3389/fevo.2020.564609
Received: 22 May 2020; Accepted: 14 December 2020;
Published: 13 January 2021.
Edited by:
Alistair William Robin Seddon, University of Bergen, NorwayReviewed by:
Triin Reitalu, Tallinn University of Technology, EstoniaSandra Nogué, University of Southampton, United Kingdom
Copyright © 2021 Brussel and Brewer. This is an open-access article distributed under the terms of the Creative Commons Attribution License (CC BY). The use, distribution or reproduction in other forums is permitted, provided the original author(s) and the copyright owner(s) are credited and that the original publication in this journal is cited, in accordance with accepted academic practice. No use, distribution or reproduction is permitted which does not comply with these terms.
*Correspondence: Thomas Brussel, dGhvbWFzYnJ1c3NlbEBnbWFpbC5jb20=