- 1Cavanilles Institute for Biodiversity and Evolutionary Biology, University of Valencia, Valencia, Spain
- 2Instituto Clodomiro Picado, Facultad de Microbiología, Universidad de Costa Rica, San José, Costa Rica
- 3Emil Racovita Institute of Speleology, Cluj Napoca, Romania
- 4Departamento de Biología, Facultad de Ciencia y Tecnología, Universidad Nacional Autónoma de Nicaragua, León, Nicaragua
Metacommunities are dynamic systems, but the influence of time independently of environmental change in their configuration has been rarely considered. In temporary ponds, strong temporal effects are expected to influence their metacommunity structure, even in relatively constant environments such as tropical habitats. We therefore expect that time as an independent factor could modulate tropical pond metacommunities, which would be also less affected by niche-related processes than by dispersal-related processes. In addition, good dispersers should be more environmentally structured than bad dispersers, which should be more spatially structured. Finally, the relevance of temporal effects should vary among organisms with different generation times. To test these hypotheses, we surveyed 30 temporary ponds along the dry tropical region of western Costa Rica and Nicaragua at three different moments of their hydroperiod: shortly after the infilling of the water bodies, at the middle of the hydroperiod and just before desiccation. We obtained data on 56 environmental variables and used geographic coordinates to build spatial variables (Moran Eigenvector Maps). We collected biological samples and estimated the specific abundance of phytoplankton, zooplankton and benthic invertebrates. To evaluate the relative role of environmental, spatial and temporal (sequential sampling season) effects for metacommunity organization, we used variation partitioning with distance-based redundancy analyses for each group of organisms. The inclusion of time in the analysis highlighted that pure temporal effects explained part of metacommunity variance in almost every group, being as important as spatial or even environmental effects for some groups of organisms. In contrast to the assumed low environmental constraints in tropical areas (i.e., high and stable temperatures), we found strong environmental effects. Passive dispersers were more influenced by environmental factors than active ones. We also found a positive relationship between the body size of the different groups of organisms and the magnitude of the temporal effects, interpreted as related to generation time. Finally, when analyzing each sampling period separately, we found differences in the relative role of environment and space at different sampling periods, showing that snapshot surveys may not be representative of highly dynamic metacommunities.
Introduction
The establishment of the metacommunity concept as referring to a group of communities linked by dispersal of their interacting species (Hanski and Gilpin, 1991; Wilson, 1992) prompted a turning point in understanding species distributions and abundances. Not only environmental filtering (the species sorting paradigm; Leibold et al., 2004), but other mechanisms related to spatial effects and dispersal rates, play a key role structuring metacommunities. Patch-dynamics (Levins and Culver, 1971), sink-source dynamics (or mass-effects; Holt, 1993) and neutral (Hubbell, 2001) paradigms are complementary to the species sorting approach when studying metacommunity assembly. In this framework, there is however a temporal component that has seldom been considered when testing theoretical expectations with empirical data. The common snapshot-survey studies neglect not only temporal changes in environmental conditions and in the influence of the spatial context, but also direct temporal effects on metacommunity structure due to processes such as life cycles, population growth or priority effects (Brendonck and De Meester, 2003; Fukami, 2015; Leibold and Chase, 2018).
Under the metacommunity framework, freshwater ecosystems are particularly interesting due to their isolation in relation to the terrestrial landscape. Rivers, lakes and ponds have largely been studied in order to understand the role of dispersal limitation and species sorting in such isolated communities (e.g., Soininen et al., 2007; Escrivà et al., 2015; López-Delgado et al., 2019). Pond metacommunities show a high degree of randomness (Chase, 2007), although strong environmental effects are also frequently observed (Leibold and Chase, 2018). Mass and priority effects at small scales (Heino et al., 2015; Castillo-Escrivà et al., 2017b) and dispersal limitation at large (Soininen et al., 2011; Heino et al., 2015) or even small spatial scales (Castillo-Escrivà et al., 2017a) seem also to be important processes affecting pond metacommunity structure. Temporary ponds, as intermittent ecosystems, strongly depend on seasonal dynamics related to their hydroperiod, egg-bank hatching and colonization processes (Williams, 2005; Chase, 2007; Castillo-Escrivà et al., 2017c). Desiccation is a major evolutionary pressure in temporary water bodies, where habitat availability changes cyclically, sometimes unpredictably, and organisms are adapted to this desiccation by means of different life-cycle strategies: while some organisms resist drought in the sediment (resting eggs, anhydrobiosis, seeds, etc.), some others need to abandon the habitat and recolonize from neighboring waterbodies (Brendonck and De Meester, 2003; Richter-Boix et al., 2011; Olmo et al., 2012; Brendonck et al., 2017; Wisnoski et al., 2019). As a consequence, some traits such as generation time, type of dispersal or survival strategy toward desiccation may influence temporal dynamics, which in turn may strongly regulate metacommunity composition (Boix et al., 2004; Holt et al., 2005; Fernandes et al., 2014; Castillo-Escrivà et al., 2017c).
Freshwater metacommunity studies are biased toward temperate regions with strong temperature seasonality, this possibly driving major changes in metacommunity structure. In contrast, tropical regions show a reduced thermal variability, while precipitations, especially in areas with a dry tropical climate, have large annual fluctuations. These fluctuations, consequence of the alternation of rainy and dry seasons, may lead to a high connectivity between ponds through extensive floods, producing a regional environmental homogenization (Thomaz et al., 2007) that locally diverges when the waterbodies become isolated during the dry season (Rojo et al., 2016). Thus, results on spatial and environmental effects on pond metacommunities sampled in temperate regions are expected to notably differ from those of tropical areas, not only because of differences in temperature regimes but also because of heavy rain effects on connectivity.
Previous studies on freshwater metacommunities point toward dispersal mode as an important trait driving metacommunity structure. It is expected that better dispersers will show weaker spatial patterns than those with lower dispersal ability. As a consequence, flying active dispersers and small-size passive dispersers will be more affected by species sorting than non-flying active dispersers or large-bodied passive dispersers (De Bie et al., 2012; Padial et al., 2014). However, this pattern has not been supported by all metacommunity studies (Heino et al., 2012; Schulz et al., 2012; Grönroos et al., 2013; Leibold and Chase, 2018). Perhaps, differences among studies in spatial scales, connectivity or groups of organisms being analyzed may hinder the observation of consistent patterns or, alternatively, dispersal abilities might be more idiosyncratic that one may expect from body size and moving capabilities. For these reasons, a multi-taxon approach in metacommunity research, including groups of different body sizes and dispersal strategies could help to disentangle how metacommunities are actually structured.
In this study, we test the influence of environmental, spatial and temporal factors on metacommunity structure of a wide range of organisms inhabiting tropical temporary ponds, including algae, rotifers, microcrustaceans, and macroinvertebrates (mainly mollusks and insects). Thus, we encompass multiple life-cycle strategies against desiccation (including resting eggs or spores in algae, rotifers and crustaceans, anhydrobiosis in some rotifers and copepods, terrestrial adult stages in insects, etc.) and distinct reproductive strategies (binary fission in cyanobacteria, strict parthenogenesis in some rotifers, cyclic parthenogenesis in rotifers or cladocerans, sexual reproduction in insects, etc.) which are strongly related with dispersal ability (active and passive dispersal) and colonization. In addition, the wide variability of taxa also includes a wide variability in body size, from a few microns in cyanobacteria to several centimeters in adult insects, which is correlated with generation time (Sammarco and Strychar, 2009; Brown et al., 2018). Empirical metacommunity analyses have seldom been carried out surveying multiple taxonomic groups from the same waterbodies, on repeated occasions, and over a wide spatial extent (e.g., Beisner et al., 2006), and even fewer of these at low latitudes (Domis et al., 2013; Padial et al., 2014; Rojo et al., 2016). This work aims at filling these gaps. Considering the standing issues on temporary pond metacommunity dynamics and the differences between groups of organisms and climatic settings we hypothesize that (i) not only spatial and environmental components drive metacommunity structure but also independent temporal factors; (ii) niche-related processes should be relatively less important than dispersal-related processes in structuring tropical pond metacommunities, as compared to published data on more seasonally variable temperate pond metacommunities; (iii) metacommunities of small passive dispersers should be strongly environmentally structured thanks to a high connectivity in tropical ponds through flooding, while metacommunities of larger-bodied organisms with reduced dispersal abilities might still show strong patterns of spatial structure; (iv) the influence of the temporal component should vary between organisms with different generation times, showing a positive relationship between these two variables, and (v) metacommunity patterns observed in snapshot surveys may provide a biased view of the major ecological processes structuring metacommunities because of considerable variation through time.
Materials and Methods
Study Area and Environmental Characterization
We surveyed 30 temporary stagnant freshwater bodies from a dry tropical region on the Pacific watersheds of Costa Rica and Nicaragua. These 30 ponds were selected in order to include a wide range of environmental conditions regarding salt content, nutrient concentration or land use, covering a large spatial extent. Selected waterbodies were grouped in four main clusters over a maximum distance among them of 370 km: Palo Verde National Park (Tempisque River lower basin) and the slopes of Miravalles and Tenorio volcanoes (Tempisque River middle basin), both in Costa Rica, and the delta of Estero Real and the Western region of Nicaragua, both in Nicaragua (Figure 1). Sasa et al. (2015) provide further details on the location, geographical setting and environmental characterization of sampling sites. Due to the temporality of these water bodies, we surveyed them thrice during their hydroperiod: 2 weeks after infilling (June 2010); once again in the middle of the hydroperiod, during the flooding peak (September 2010) and the last time immediately after the end of the rainy season, before the desiccation of the ponds (January 2011).
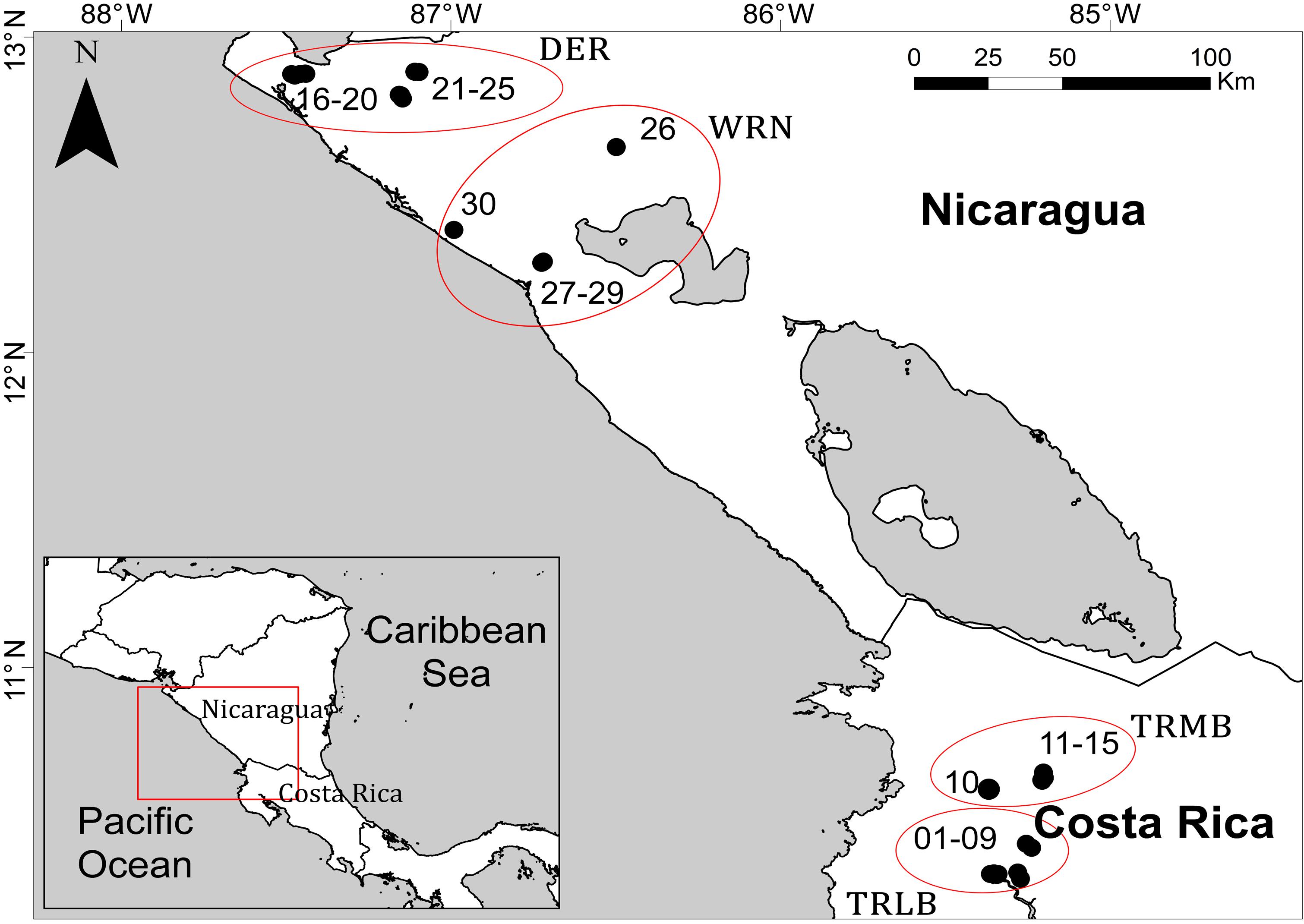
Figure 1. Map of the study area with the location of the studied ponds, grouped in four main clusters: DER (Delta of Estero Real), WRN (Western Region of Nicaragua), TRMB (Tempisque River Medium Basin), and TRLB (Tempisque River Low Basin). Based on Figure 1 in Sasa et al. (2015).
We assessed a set of 56 environmental variables for each pond, including limnological, hydrogeomorphological, biotic, climatic, landscape, and conservation status. In every survey, we measured in situ water temperature, total dissolved solids (TDS), electric conductivity (EC) and pH using a Hanna pH/EC meter HI 98130; oxygen concentration was measured with the Winkler method and transparency with a Snell tube. In addition, we took water samples in order to analyze nutrient and ion concentrations in the lab: 250 ml of unfiltered water for anion analyses (bicarbonate and carbonate alkalinity (Alk), chloride and sulfate), 100 ml of unfiltered water, fixed with nitric acid, for cation analysis (Na+, K+, Mg+2, Ca+2), and 100 ml of filtered water (through GF/F Whatman filters) for nutrient (PO43–, NO3–, NO2–, NH4+) concentration analysis (Rice et al., 1992). The used GF/F filters were analyzed for chlorophyll-a concentration following Jeffrey and Humphrey (1975). Further details on sampling and analytical methods and limnological results are described in Sasa et al. (2015). We calculated some ratios between nutrient or ionic concentrations to be used as possible explanatory variables (Alk/Ca+2, Alk/(Cl– + SO42–), (Ca+2 + Mg+2)/(Na– + K–), Ca+2/Mg+2, NO3–/NO2–, NO3–/NH4+). As for hydrogeomorphological variables, we measured the maximum and average depth of each water body, using a graduated stick, and gathered information from field data on the origin of the water (rain, streams, phreatic) and hydroperiod length (seasonal or semipermanent). Furthermore, we measured the area, perimeter, morphology {shoreline development: DL = L/[2√(πA)]; Aronow, 1982} and altitude. Regarding biotic variables (besides chlorophyll-a concentration), we visually estimated the percentage of water surface and shoreline covered by macrophytes and helophytes, respectively, and recorded the presence of livestock. We downloaded climatic variables, including maximum annual temperature, minimum annual temperature, average annual temperature, temperature range, annual average precipitation, and precipitation seasonality from the online server worldclim.org (using historical climate data from 1970 to 2000; Fick and Hijmans, 2017) and extracted these data by means of ArcGis 10.0 (ESRI, 2006). As for the landscape and land use of the watershed surrounding each pond, we estimated the percentage of land surface occupied by agriculture, buildings, forest, scrub, low grass and high grass, and landscape heterogeneity. For this purpose, we manually measured the percentage cover of these categories in a buffer area of 100 m of diameter around the sampling point using Google Earth (Google Inc.) satellite images. The landscape heterogeneity was calculated with a Shannon index of the proportions of the above-mentioned landscape categories. Finally, we determined the conservation status of each wetland through the ECELS (ECELS1-5 and total ECELS) index (Boix et al., 2010; Sasa et al., 2015). Instead of using latitude and longitude as spatial variables, we calculated Moran’s Eigenvector Maps (MEMs) (Dray et al., 2006), consisting of a matrix of positively autocorrelated orthogonal variables of different spatial scales. The environmental characterization is summarized in Supplementary Table S1.
We built three different matrices with the data gathered: (i) a spatial matrix, including MEMs, presence or absence of connectivity with other neighboring waterbodies and a categorical factor corresponding to the region (Costa Rica or Nicaragua), (ii) a temporal matrix, with the sampling period, as a dummy variable, and (iii) an environmental matrix, with all the variables explained above. These matrices were further used in statistical analyses together with the biological communities data (see below).
Biological Communities
We collected biological samples of phytoplankton, zooplankton, and benthic invertebrates at each site and sampling period. Phytoplankton samples were collected in 100-ml amber-colored glass bottles, directly from the water column, and fixed with Lugol’s iodine solution. Zooplankton quantitative samples were taken by filtering a volume of water (2–20 L measured with a graded jar, until filter got plugged) through a 35-μm mesh filter in order to ensure the capture of the smallest rotifers and microcrustaceans, and fixed with 4% formaldehyde. These samples were collected from the different microhabitats observed, including different depths, substrate or vegetation types and coverages. Benthic invertebrates were collected using a 250 μm pore-size hand net, taking samples from every distinct microhabitat. These samples where fixed with ethanol 96%. In the lab, all the collected groups of organisms were identified and counted using a Leica Leitz Biomed microscope, a Leica DMIL Led inverted microscope and a Leica M205C stereomicroscope, up to the maximum taxonomic resolution possible using a variety of taxonomic works, mostly the following: Huber-Pestalozzi (1976–1982) and Wołowski and Hindák (2005) for phytoplankton; Koste (1978) and Segers (1995) for rotifers in the zooplankton samples; Elías-Gutiérrez et al. (2008) and references therein for cladocerans and copepods (Cyclopoida and Calanoida); Meisch (2000) and Karanovic (2012) and references therein for ostracods; and Domínguez and Fernández (2009), Springer et al. (2010), and Thorp and Covich (2010), and references therein, for benthic invertebrates other than ostracods.
With these data, we built species abundance matrices of all three sampling periods for a series of (nested) groups of organisms: the whole phytoplankton dataset, and separately for Cyanobacteria, Chlorophyceae, mixotrophic phytoplankton (Chrysophyceae, Cryptophyceae, Euglenophyta, and Dinoflagellata) and Diatomea; Rotifera; non-Decapoda nor Isopoda crustaceans (from now on, Crustacea), and separately for Branchiopoda, Copepoda, and Ostracoda; all benthic macroinvertebrates (excluding Ostracoda) and separately for Mollusca, Insecta, Paleoptera (Ephemeroptera and Odonata), Heteroptera, Coleoptera, and Diptera.
Statistical Analysis
In order to determine the role of environmental, temporal and spatial factors over the structure of the metacommunity, we carried out variation partitioning analyses (Peres-Neto et al., 2006). The relative abundance matrices were Hellinger-transformed in order to reduce the influence of rare and ubiquitous species (Legendre and Gallagher, 2001). Environmental variables were transformed depending on their initial frequency distribution, using either logarithms, the arcsine of the square root, or the square-root, in order to reduce the leverage effect of outliers and to approach them to a normal distribution.
We implemented 17 partial distance-based redundancy analyses (dbRDA) with the purpose of explaining the variation of each species matrix in relation to the environmental [E], spatial [S], and temporal [T] matrices. Variation partitioning allows quantifying the percentage of variation explained purely by the environmental component [E| (S + T)], purely by the spatial component [S| (E + T)] and purely by the temporal component [T| (E + S)]. Furthermore, part of the metacommunity variation can also be explained by an overlap between two or more components: environmental and spatial overlap [(E ∩ S)| T], environmental and temporal overlap [(E ∩ T)| S], spatial and temporal overlap [(S ∩ T)| E] and environmental, spatial and temporal overlap [E ∩ S ∩ T]. Variables from environmental, spatial and temporal data matrices went through a forward selection process prior to each variation partitioning analysis, with a double stopping criterion (Blanchet et al., 2008). To further study the relative effects of the temporal component on metacommunity organization across organisms, we compared three groups of taxa with varying generation times, which are highly correlated with body size (Sammarco and Strychar, 2009; Brown et al., 2018): phytoplankton, microinvertebrates (rotifers, branchiopods, copepods and ostracods) and macroinvertebrates (remaining groups of analyzed benthic invertebrates).
In order to check if snapshot survey results are representative of the whole metacommunity dynamics through time, we performed a variation partitioning analysis for selected groups, following the same method as explained above but now performing a test separately for each sampling period and group of organisms, and therefore excluding temporal variables from analyses.
To test for significant differences in the pure temporal component between groups with different generation times (phytoplankton, micro-, and macroinvertebrates), and in pure components between groups of organisms with different dispersal strategies (passive/active), we performed Kruskal-Wallis tests (Kruskal and Wallis, 1952). All analyses were performed with R (v3.6.0; R Core Team, 2019) and R packages vegan (Oksanen et al., 2019), ade4 (Bougeard and Dray, 2018) and adespatial (Dray et al., 2019).
Results
Altogether, we found 295 phytoplankton taxa, most of them identified to species level (Cyanobacteria: 44 taxa; Chlorophyceae: 114 taxa; mixotrophic phytoplankton: 56 taxa; Diatomea: 77 taxa), 102 rotifer taxa, most of them identified to species level, so as the 80 crustacean taxa (Branchiopoda: 34 taxa, Copepoda: 15 taxa, including 13 Cyclopoida and 2 Calanoida, Ostracoda: 31 taxa) and 169 macroinvertebrate taxa, including 19 mollusks, 34 paleopterans, 19 heteropterans, 28 coleopterans, and 32 dipterans (121 insect taxa). The list of identified species can be found in Supplementary Table S2.
Selected variables in dbRDA are shown in the Supplementary Table S3. The proportion of metacommunity variation explained by the selected significant variables, considering all three components (E + S + T) together, varied between 0.09 in Insecta and 0.33 in Ostracoda (average 0.20 ± 0.06). The results of the variance partitioning analyses show a significant effect of environmental, spatial and temporal components for most taxonomic groups (Figure 2 and Table 1), with a predominance of pure environmental over pure spatial and temporal effects. Especially remarkable are the mixotrophic phytoplankton, with a stronger pure temporal component than any other phytoplankton group; Heteroptera, with a large pure spatial component; and Diptera, with the highest pure temporal component. We did not find significant pure environmental effects only in Heteroptera and Insecta (probably influenced by Heteroptera). Part of the variation of all phytoplankton groups was significantly explained by pure spatial factors, but none in the case of Rotifera, Branchiopoda and Paleoptera. Finally, we found non-significant pure temporal effects only in small body size taxa (Chlorophyceae, Diatomea, Branchiopoda and Ostracoda), but these effects were always significant in macroinvertebrates.
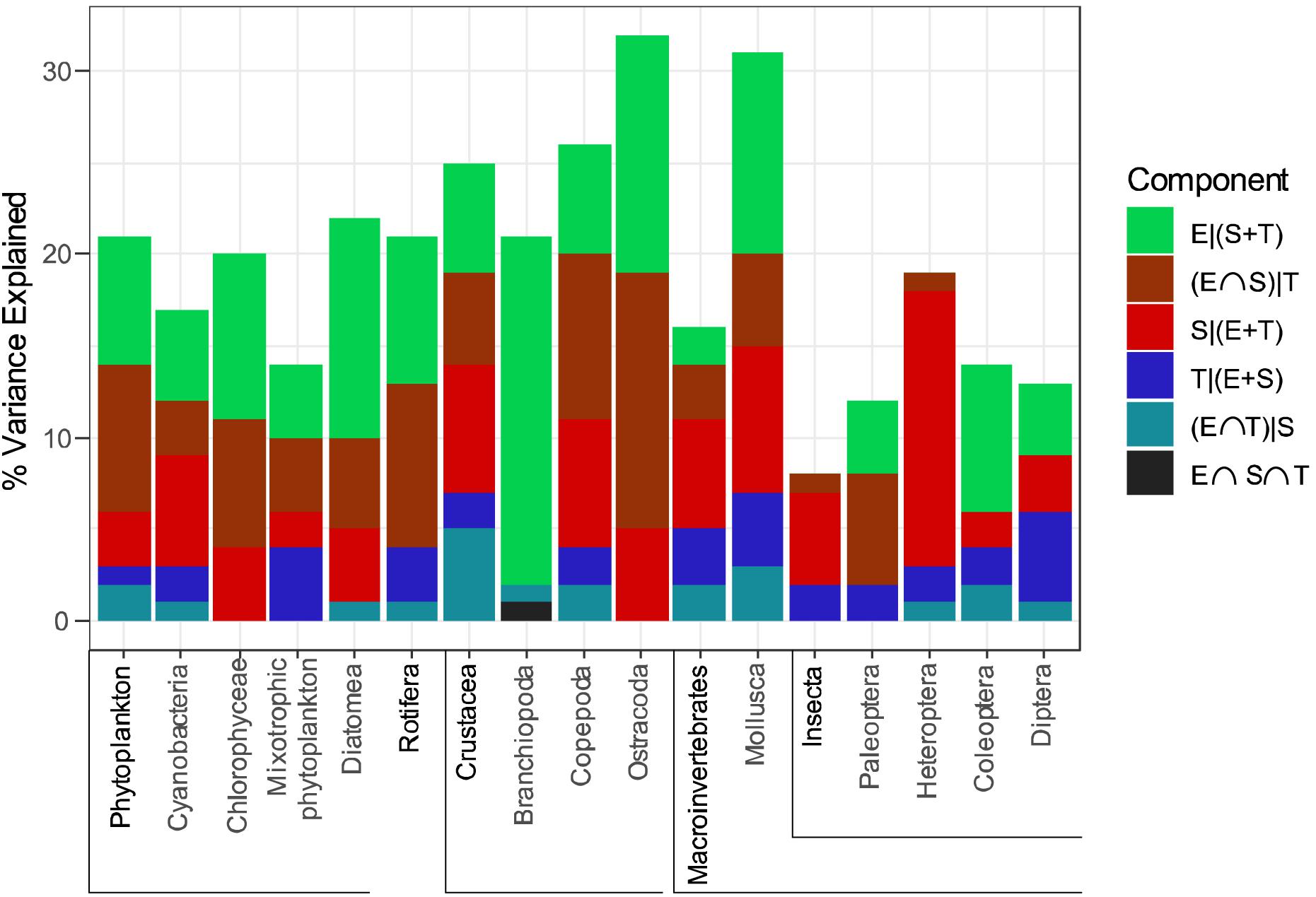
Figure 2. Results of variation partitioning analysis for each group of organisms. The percentage of variation explained by each component is represented with a different color (green: pure environment; brown: environment-space overlap; red: pure space; dark blue: pure time; cyan: environment-time overlap; black: environment-space-time overlap). Only significant components (P < 0.05) are shown. Taxa in bold type include species from the following underlined groups.
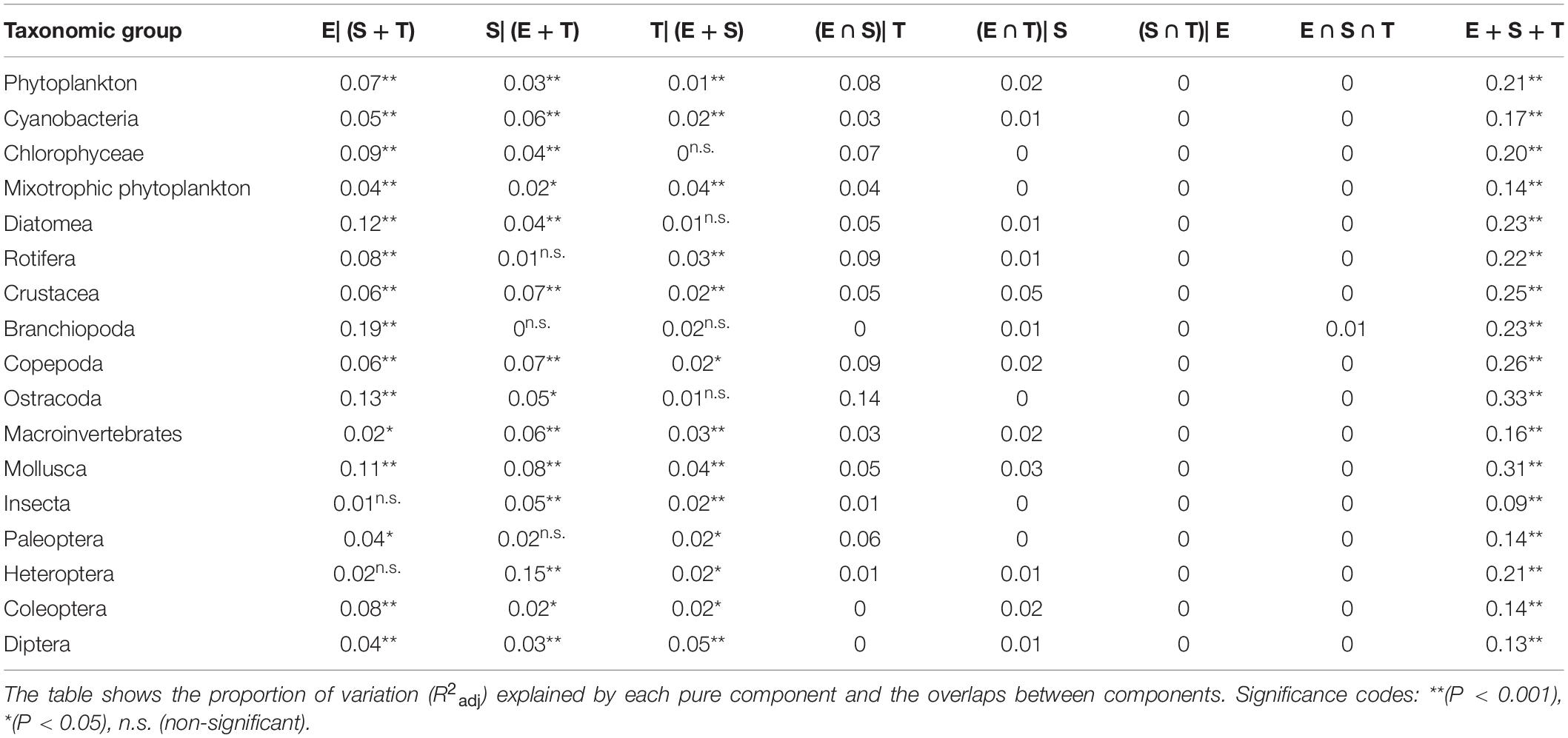
Table 1. Results of variation partitioning analysis for each group, where E, environmental component; S, spatial component; T, temporal component.
When comparing passive and active dispersers, the pure environmental component was significantly higher in the former group (Figure 3, Kruskal-Wallis: P = 0.037). On the other hand, there were no differences neither in pure spatial nor temporal effects between these groups. Therefore, although the spatial structure and temporal dynamics seem to have the same influence for both types of dispersal strategies, passive dispersers seem to be more environmentally structured than active dispersers.
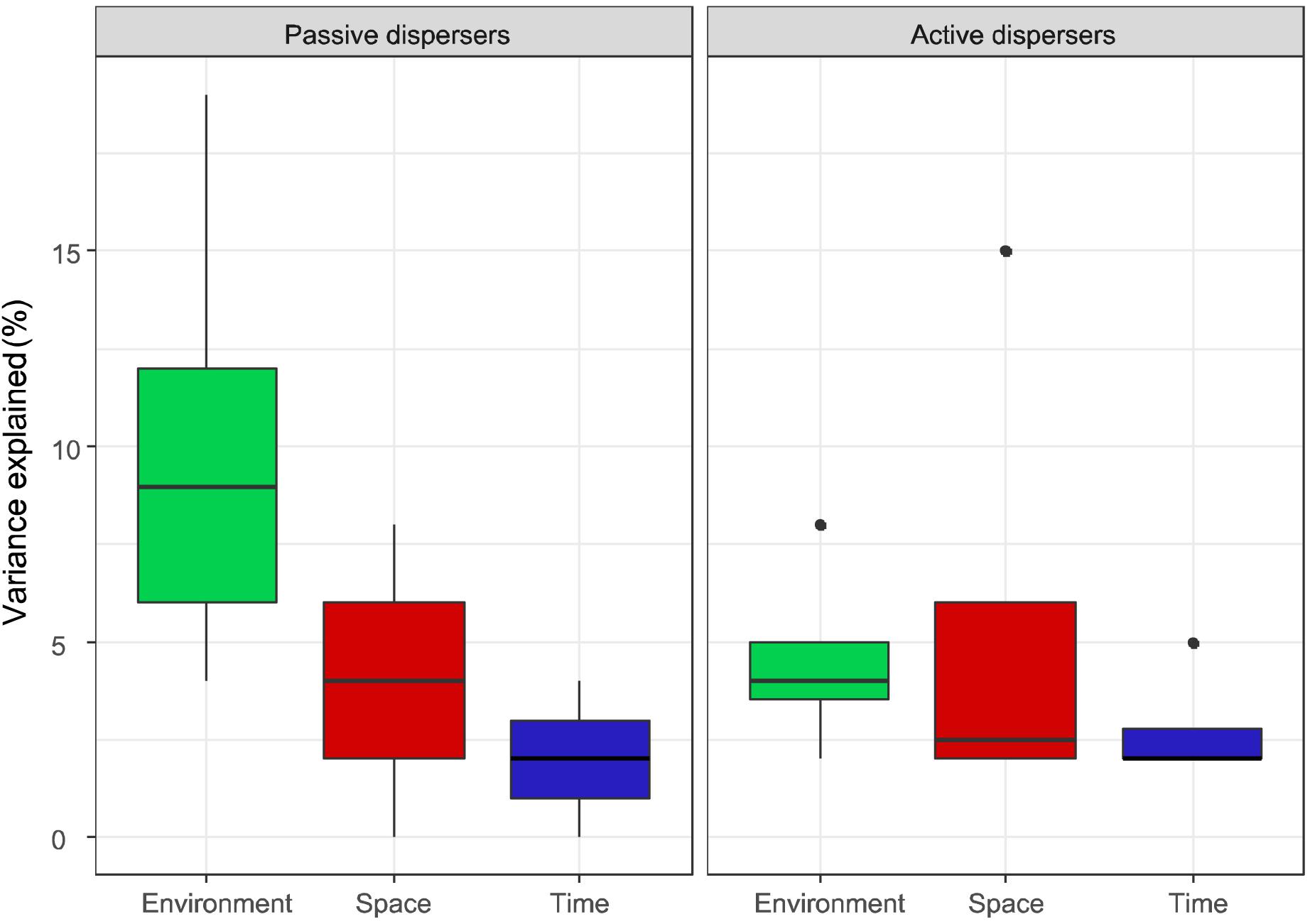
Figure 3. Percentage of metacommunity variance explained by pure environmental, spatial and temporal components, according to the type of dispersal of the studied groups of organisms.
In Table 2, we show the number and type of significant environmental variables explaining the variance of each group. In organisms with small body size and passive dispersal (from Phytoplankton to Crustacea), species sorting is dominated by limnological variables. In Mollusca and active dispersers, limnological, climatic, and landscape variables seem to play a similar role, but no hydrogeomorphological variables were selected for insects.
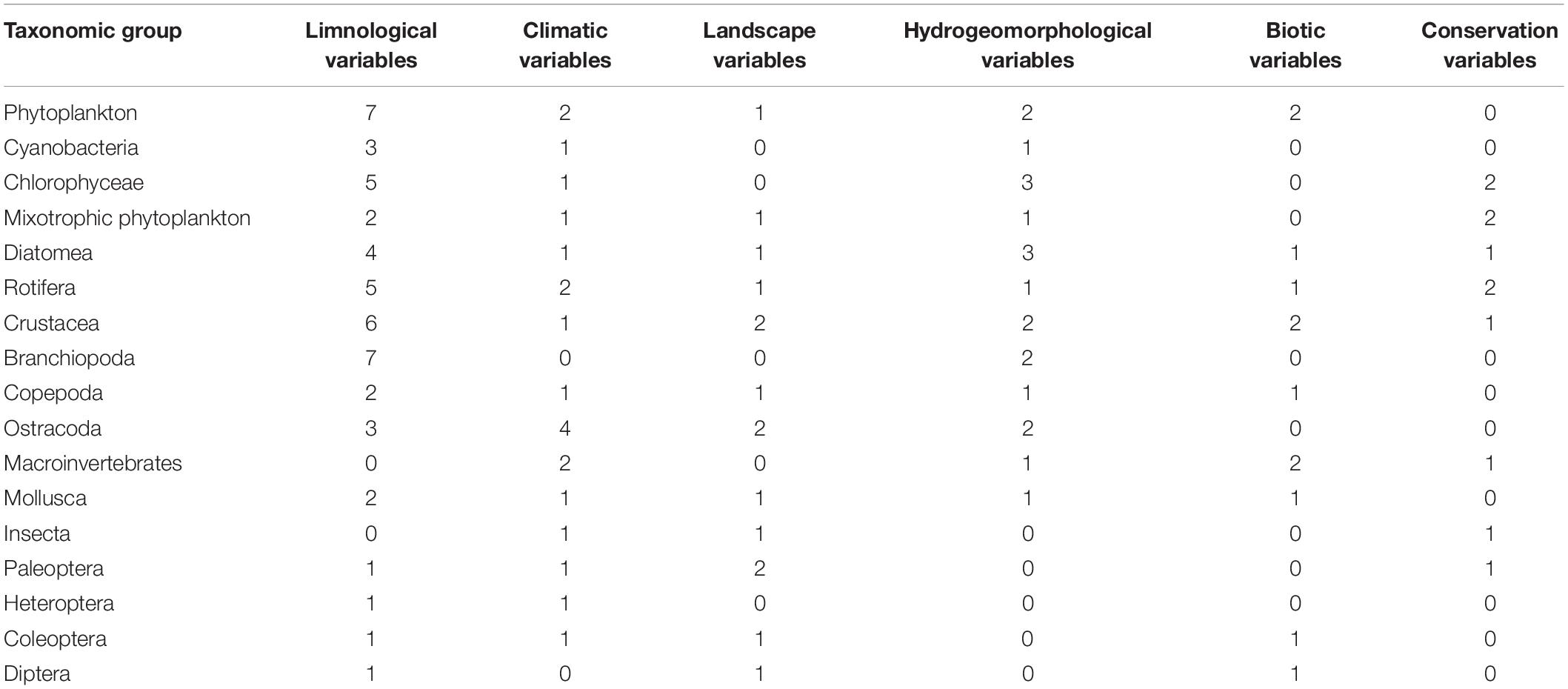
Table 2. Number and type of environmental variables selected in the variation partitioning analyses for each group of organisms.
The influence of the temporal component seems to follow an increasing trend that might be related to increasing length of the life-cycle, from phytoplankton groups to microscopic metazoans to macroinvertebrate groups (Figure 4). However, we did not find significant differences among these three groups (K-W test, P = 0.354).
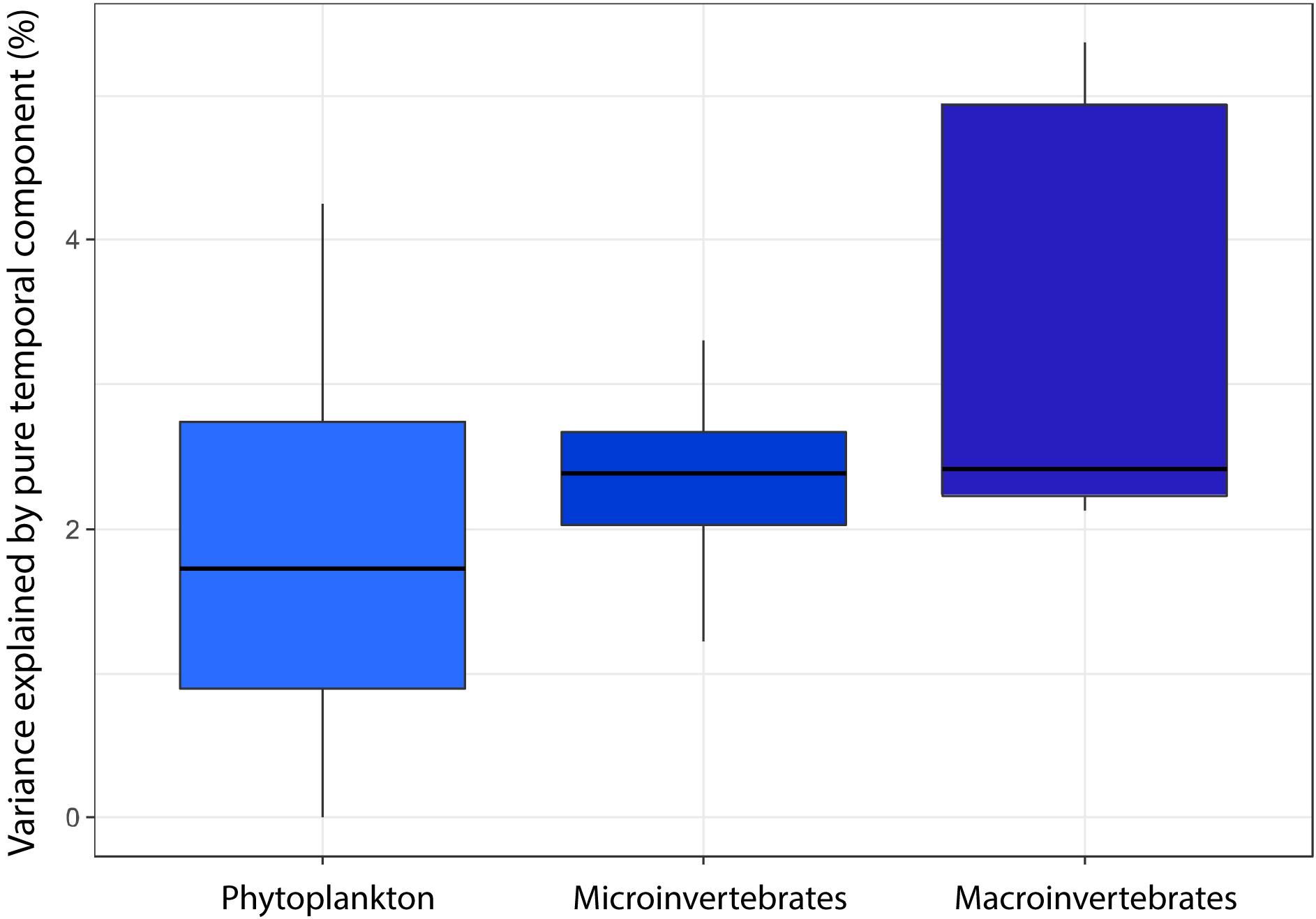
Figure 4. Percentage of variance explained by pure temporal effects for three groups with different generation times: Phytoplankton (Cyanobacteria, Chlorophyceae, mixotrophic phytoplankton, and Diatomea), Microinvertebrates (Rotifera, Branchiopoda, Copepoda, and Ostracoda) and Macroinvertebrates (Mollusca, Paleoptera, Heteroptera, Coleoptera, and Diptera).
Finally, separated variation partitioning analyses for each of the three sampling seasons were carried out for five different groups of organisms (Phytoplankton, Rotifera, Crustacea, Mollusca, and Insecta) (Figure 5 and Table 3) to check for variability of spatial and environmental effects through time. Selected variables are shown in the Supplementary Table S4. We observed a temporal variation in the percentage of variance explained by the pure environmental component, even though this component appears to be significant in every group through time. The pure spatial component decreases with time in every group except for Mollusca, which do not present a significant pure spatial component in any sampling period, and for Insecta, whose pure spatial component remains almost constant.
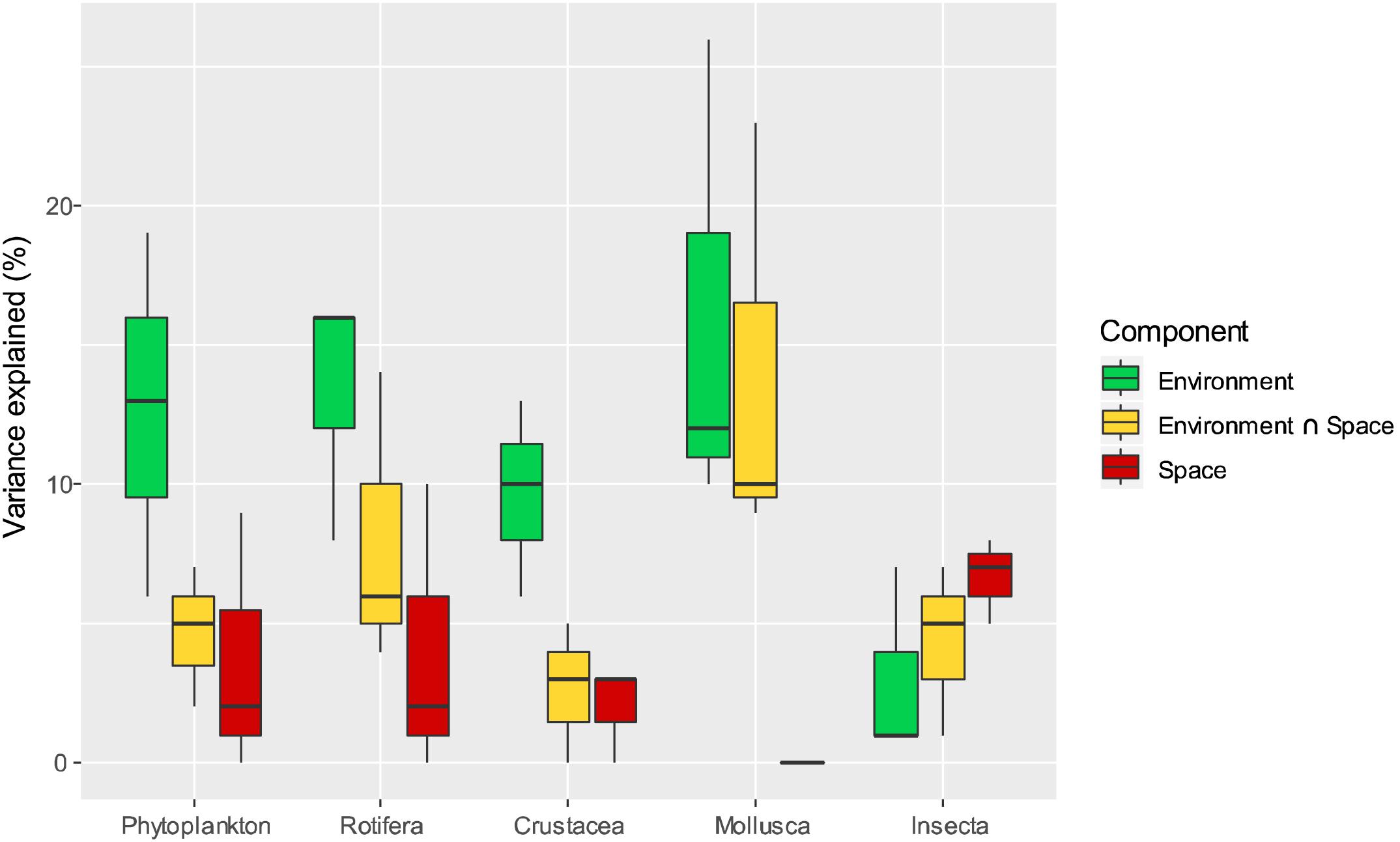
Figure 5. Results of variation partitioning analyses of Phytoplankton, Rotifera, Crustacea, Mollusca, and Insecta studied for each sampling season separately. The variability among seasons is represented in a boxplot graph. The percentage of explained variation for each component is represented with a different color.
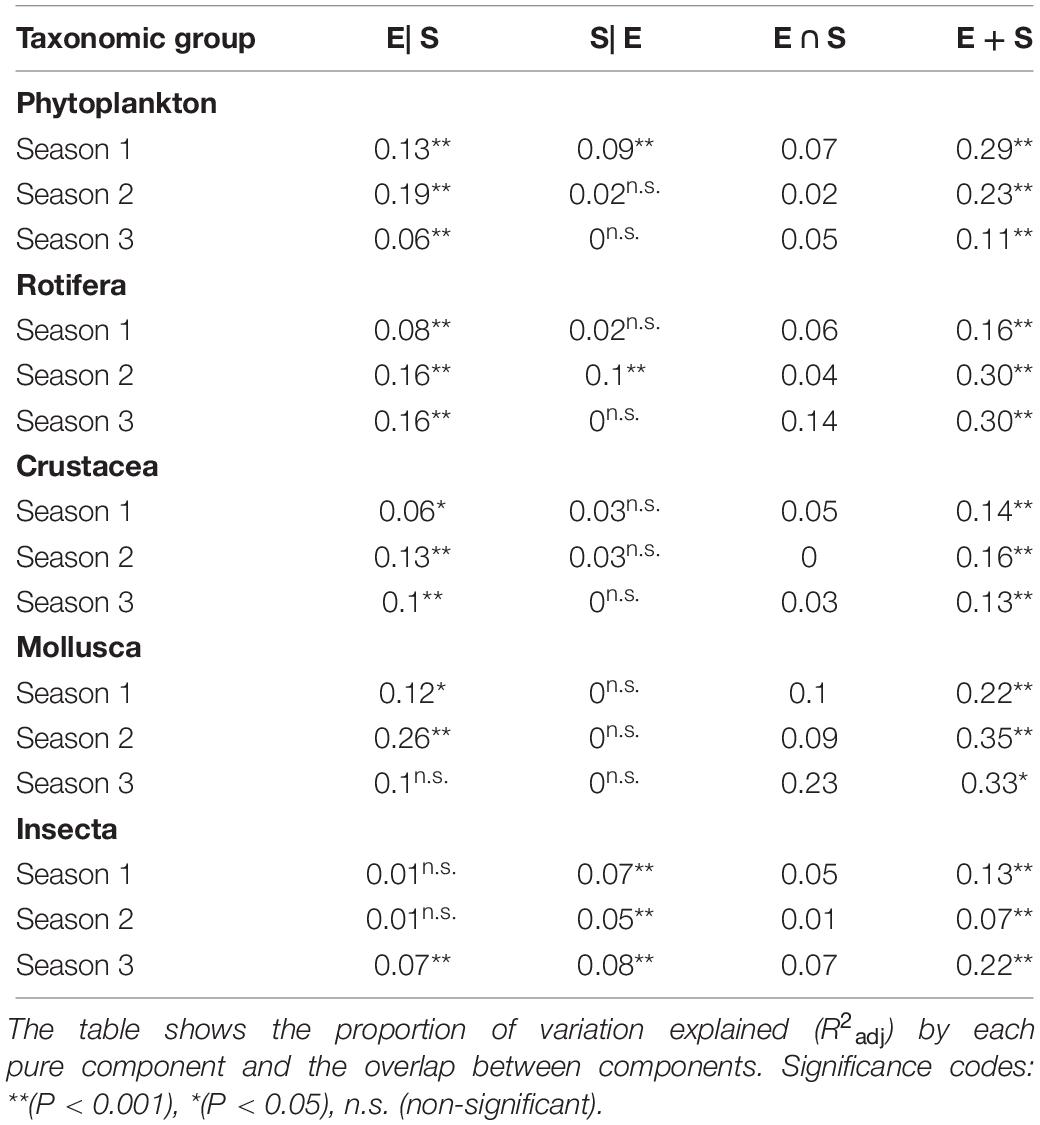
Table 3. Results of variation partitioning analysis for Phytoplankton, Rotifera, Crustacea, Mollusca and Insecta, each sampling campaign analyzed separately, where E, environmental component; S, spatial component.
Discussion
Our results show that species distributions of most of the studied taxa are environmentally, spatially and temporally structured, notwithstanding the relatively low percentage of variances explained by the set of selected variables. Such low values are not uncommon, according to previous studies of freshwater metacommunities (Soininen et al., 2007; De Bie et al., 2012; Padial et al., 2014; Rojo et al., 2016) and, because we made an intensive effort of environmental and spatial characterization, the unexplained variation might be largely attributable to other unmeasured processes. For example, biotic interactions such as predation, competition, facilitation, etc. seem to play an important role structuring metacommunities, increasing the influence of environment (both abiotic and biotic) on metacommunity assembly (Leibold and Chase, 2018; García-Girón et al., 2020). Furthermore, ecological drift, or random variation of species abundances, generating differences between sites, is also a strong process influencing metacommunity structure and dynamics (Jeffries, 1988; Chase, 2007).
Temporal effects were found to be relevant in our analysis of metacommunity dynamics. On the one hand, there is an overlap between temporal and environmental components that suggests that part of the significant environmental variables undergo temporal changes throughout the hydroperiod (Bellier et al., 2013). In this sense, species sorting snapshot studies are not fully representative of the whole metacommunity (Rojo et al., 2016). Actually, in highly dynamic ecosystems such as ponds, temporal changes drive fast variations in community structure through turnover processes. On the other hand, we found significant pure temporal effects on most organisms, which even had a stronger influence than pure spatial or pure environmental components in some cases: the temporal component was higher or equal than environmental or spatial effects in 9 out of 17 taxa, being the most important component in Diptera (5% of variance explained, maybe influenced by seasonal dynamics in some families such as Chironomidae or Culicidae; Yunjun and Xiaoyu, 2007). Most previous studies focused on the temporal change of species sorting and neutral effects by comparing between sampling periods (e.g., Fernandes et al., 2014; Rojo et al., 2016), not checking the proportion of variation explained by time itself and, when they did, they usually found a very weak or non-significant influence of temporal effects on the metacommunity (Anderson and Gribble, 1998; Padial et al., 2014). However, our results show that time per se, can indeed be an important element in metacommunity structure, even more important than space and environment in some cases, as also found by Bortolini et al. (2019) in a study of subtropical phytoplankton.
Species sorting appears to be an important process for most groups, evidenced by the significant percentage of variance explained by the pure environmental component in all the groups except Heteroptera and Insecta. In addition, this component showed the highest explanatory power in 9 out of 17 metacommunity groups being analyzed. These results highlight the idea that pond communities, even in tropical regions with high species richness and relatively low environmental variability, can be structured by the environment. As a consequence of the relatively small overall niche space in relation to the high number of coexisting species responding to this reduced environmental variability, we might expect narrow realized niches due to niche packing (Lamanna et al., 2014). In addition, we found high percentages of overlap between the environmental and spatial components, perhaps attributable to spatially structured environmental gradients (Clappe et al., 2018), such as climate or landscape variables.
The observed niche-related processes seem to be modulated by dispersal capability. According to the selected environmental variables, passive dispersers were strongly influenced primarily by limnological variables. Many phytoplankton and zooplankton species have wide distributions (Vyverman, 1996; Finlay, 2002; Forró et al., 2008; Segers, 2008) (but see Fontaneto, 2011), and their resting forms are highly resistant to environmental stress (Alekseev et al., 2007; Holzinger and Karsten, 2013; Radzikowski, 2013). The distribution of these organisms is therefore unlikely to be controlled by regional environmental variables, such as climate or landscape, so that local environment might play a stronger influence in their colonization success and population dynamics. On the other hand, we found that active dispersers were influenced at a similar intensity by both local (limnological) and regional (landscape, geographic) variables, being sensitive to aquatic and terrestrial environmental conditions, as also found by other authors (Nnoli et al., 2019). Passive dispersers showed higher pure environmental effects compared to active dispersers, in agreement with previous works that found that the distribution of organisms with high dispersal ability, such as phytoplankton, was more influenced by local environmental conditions (Padial et al., 2014), although other authors consider that actively moving organisms should show a stronger relationship with environmental factors than passively dispersing ones (De Bie et al., 2012; Soininen, 2014). Maybe insects, despite being able to fly, are not so easily dispersed at long distances as are passive dispersers such as algae, rotifers or microcrustaceans. These differences may even be stronger in tropical areas due to the expected increase in dispersal limitations (mountains are “higher” in the tropics; Janzen, 1967). The relative influence of space and environment on metacommunity organization depends on the extent of spatial and environmental gradients and on connectivity, not only on organisms’ dispersal traits (Heino et al., 2015; Castillo-Escrivà et al., 2020). One may expect that if we would include semi-permanent ponds and seasonally connected ponds, or other types of water bodies, in the study, the observed influence of species sorting on the structure of aquatic metacommunities would probably increase (Wellborn et al., 1996; Cottenie et al., 2003). We need not to forget that other factors may influence the relative influence of these components, such as the number of measured environmental variables, the way that space is considered in the analyses, the sampling resolution, habitat heterogeneity or species pool size (Leibold and Chase, 2018). In any case, our expectation that small body sized, passively dispersing organisms are proportionally less affected by spatial factors than environmental ones, seem to be supported, although perhaps not so much because of the influence of flood connectivity but rather by regionally related aspects of reduced dispersal in larger bodied organisms such as the abovementioned Janzen’s effect.
Neutral processes seem to have high importance in these isolated systems, as suggested by the residuals and the significant pure spatial component in most of the analyzed groups, in agreement with previous studies (De Bie et al., 2012; Baguette et al., 2013). This component was especially strong for the flying Heteroptera, with about 70% of their total variation explained by pure spatial effects. This was unexpected, given the high dispersal ability and colonization efficiency of many heteropterans (Williams, 2005), so this perhaps reflects high small-scale dispersal among nearby ponds, together with larger-scale dispersal limitation. In this sense, the distribution of some groups with low dispersal ability, such as Mollusca, is expected to be more affected by dispersal limitation than by mass effects dynamics, although this depends on sampling extent and connectivity. On the other hand, groups with high dispersal ability, sometimes with cosmopolitan distributions, also showed a significant pure spatial component, which cannot be directly attributable to dispersal limitation but perhaps to mass effects instead (Leibold et al., 2004; Ng et al., 2009; Winegardner et al., 2012). Due to the similar percentage of variance explained by the pure spatial component in groups with very different dispersal strategies and abilities (e.g., Chlorophyceae: 4%; Cyanobacteria: 6%; Copepoda: 7%; Mollusca: 8%; Diptera: 3%) we interpret that both dispersal limitation and mass effects may contribute to spatially structuring the studied metacommunity (Declerck et al., 2011). However, the relative importance of spatial factors compared to species sorting was not as high as we initially expected from the reduced environmental fluctuations in tropical environments, so we must reject our hypothesis that environmental processes should have a lower influence than spatial processes in tropical metacommunity organization.
Even though the temporal effects were found to widely vary among taxonomic groups with similar generation times, the influence of the temporal component related positively (although this relationship was not significant) to organism generation time (or its surrogate of body size). Many biological processes that strongly affect individuals and populations of (aquatic) organisms, such as maturation, reproduction, senescence, or population growth depend on time spanned (Lahr et al., 1999; Cayrou and Céréghino, 2005; Williams, 2005). In addition, egg-bank hatching and immigrant colonization are also time-dependent (Frisch and Green, 2007; Vanschoenwinkel et al., 2010). We found no significant differences in the temporal component between active and dispersal colonizers, so we cannot state that any of these groups is more strongly structured by time-related processes. We could not provide a strong support for our expectation of higher temporal effects in longer-lived organisms, but the observed (non-significant) trend calls for further research on this possibility, maybe increasing the time extent to be able to detect temporal effects (Castillo-Escrivà et al., 2020). The taxonomic groups with non-significant temporal effects were all passive dispersers, which usually leave diapausing propagules in the sediment and have fast life-cycles. These temporal effects or their lack thereof might therefore be related to colonization processes (faster from the sediment, with certain lag from other ponds), increased turbidity and dilution of planktonic populations during the rainy season, overlapping generations, biotic interactions or metamorphosis and flee from the waterbody by juvenile insects when molting to flying adult instars (Anderson et al., 1999; Williams, 2005; Nursuhayati et al., 2013).
Snapshot surveys are common in metacommunity studies, and high variability of results are observed between them regarding the most influential factors, with even contradictory conclusions (e.g., De Bie et al., 2012; Farjalla et al., 2012). When analyzing our temporal series as three separate snapshots, we found clear differences not only between periods but also compared to our overall results when analyzing the three sampling campaigns altogether. These inconsistencies evidence that single-survey metacommunity studies may drive to misleading or uncomplete conclusions. Our results show an unexpectedly high neutral-based structuring during the seasons corresponding to infilling and maximum flooding, that eventually decreases during the dry season (the desiccation phase), in relation to the relative importance of environmental filtering for all groups (except Mollusca). The observation of relatively high neutral structure at the onset of the hydroperiod was previously observed by Castillo-Escrivà et al. (2017c) in ostracods from temporary lakes, suggesting an initial hatching bloom of opportunistic species from the egg bank (Olmo et al., 2012) provoking more random associations that would later become structured by species filtering, i.e., niche-related processes. As previous studies suggest, seasonal floods produce spatial deconstruction, increasing connectivity and dispersal of many organisms, so as dilution effects and environmental homogenization (Thomaz et al., 2007; Rojo et al., 2016). Thus, in early phases of the hydroperiod, community structure might highly depend on egg-bank hatching and, soon after, also on spatial processes, such as hydrochory via flooding or colonization by immigrants. Conversely, pond isolation and consequent environmental heterogenization as the pond succession proceeds toward the end of the rainy season produce an increment of species sorting effects (Fernandes et al., 2014). However, in organisms without an egg bank, such as most aquatic insects, we observe a constant strong influence of pure spatial components. Insects must leave ponds before desiccation and recolonize other water bodies after infilling, so that colonization events can happen repeatedly all along the hydroperiod (Tronstad et al., 2007), but constrained by life-cycles and distance between sites. Anyway, when comparing snapshot and periodic sampling analyses considering time as a set of variables to partial out different structuring effects, remarkable differences can arise; for instance, in the whole hydroperiod analyses of the mollusk metacommunity, the pure spatial component explained an important percentage of variance, while it appeared to be non-significant in single-period analyses, where there is a higher overlap between spatial and environmental effects. This overlap could then be disentangled when considering three sampling periods together.
We may conclude that time is a relevant factor organizing metacommunities of tropical temporary ponds, becoming almost as important as environment and space (Langenheder et al., 2012), as expected from ecological theories of community assembly and succession (Pickett et al., 2011; Fukami, 2015). Many temporal processes are difficult to study, especially long-term ones, but short-term temporal dynamics seems to modulate tropical (pond) metacommunities on a par with niche and dispersal related processes. In addition, species with larger body sizes (and longer generation times) seem to be more influenced by environment-independent temporal effects than smaller, faster developing, organisms. However, despite the importance of spatio-temporal factors influencing metacommunity structure, environment seems to be the main process in metacommunity assembly, supporting the relevance of the species sorting paradigm. Moreover, this environmental component is higher in passive dispersers, mainly influenced by local environment, than in active dispersers, influenced by local and regional environment. Finally, our results show that in many organisms, environmental and spatial components are highly variable between periods, so snapshot surveys provide only partial information about metacommunity organization.
Data Availability Statement
All datasets generated for this study are included in the article/Supplementary Material.
Author Contributions
FM-J, JM, RR, CR, and MS equally contributed to project design and logistics. FB, JM, RR, MS, FM-J, and CR carried out field work. Phytoplankton identification was supervised by CR. ÁG, CO, and XA identified Rotifera, Branchiopoda, and Calanoida. SI identified Cyclopoida. FM-J and JA-A identified Ostracoda. JR identified macroinvertebrates. ÁG did data analysis and manuscript writing. FM-J supervised and reviewed the manuscript with comments from XA, JR, SI, CR, and CO. All authors contributed to the article and approved the submitted version.
Funding
This research was supported by AECID Grants A1024073/09 and A/031019/10 of the Spanish Ministry of Foreign Affairs to the University of Valencia and by Vicerrectoría de Investigación Universidad de Costa Rica Grant 741-B1-517. This study was also sustained by the Spanish Ministry of Economy, Industry and Competitivity–AEI, and FEDER (EU), through project METACOM-SET (CGL2016-78260-P). The Spanish Ministry of Economy and Competitiveness partially supported this study through project CGL2014-54502-C2-1- ÁG was also supported by an FPI fellowship BES-2017-080022 from the Spanish Ministry of Economy, Industry and Competitiveness.
Conflict of Interest
The authors declare that the research was conducted in the absence of any commercial or financial relationships that could be construed as a potential conflict of interest.
Acknowledgments
We want to thank Matilde Segura for phytoplankton identification. We are also grateful to Sergio Padilla, Arelly Reyes, and Davinia Beneyto for field assistance, Ulises Chavarría and the Palo Verde National Park personnel, Darío Hernández (Vicerrectoría de Investigación, UCR), Julieta Carranza, WalterMarín, Odeth Esquivel, and Yorleny Aguilar (Oficina Asuntos Internacionales, UCR) for logistic support at different stages of the project. We are also very grateful for the comments and suggestions of Jani M. Heino, Man K. Cheung, and Fábio A. Lansac Toha, which improved the present paper.
Supplementary Material
The Supplementary Material for this article can be found online at: https://www.frontiersin.org/articles/10.3389/fevo.2020.558833/full#supplementary-material
References
Alekseev, V. R., de Stasio, B. T., and Gilbert, J. J. (2007). Diapause in Aquatic Invertebrates: Theory and Human Use. Dordretch: Springer.
Anderson, C. R., Peckarsky, B. L., and Wissinger, S. A. (1999). “Tinajas of southeastern Utah: invertebrate reproductive strategies and the habitat templet,” in Invertebrates in Freshwater Wetlands of North America: Ecology and Management, eds D. P. Batzer, R. B. Rader, and S. A. Wissinger (New York, NY: John Wiley and Sons, Inc), 791–810.
Anderson, M. J., and Gribble, N. A. (1998). Partitioning the variation among spatial, temporal and environmental components in a multivariate data set. Austr. J. Ecol. 23, 158–167. doi: 10.1111/j.1442-9993.1998.tb00713.x
Aronow, S. (1982). “Shoreline development ratio,” in Beaches and Coastal Geology, ed. M. Schwartz (Boston: Springer), 754–755. doi: 10.1007/0-387-30843-1_417
Baguette, M., Blanchet, S., Legrand, D., Stevens, V. M., and Turlure, C. (2013). Individual dispersal, landscape connectivity and ecological networks. Biol. Rev. 88, 310–326. doi: 10.1111/brv.12000
Beisner, B. E., Peres-Neto, P. R., Lindström, E. S., Barnett, A., and Longhi, M. L. (2006). The role of environmental and spatial processes in structuring lake communities from bacteria to fish. Ecology 87, 2985–2991. doi: 10.1890/0012-9658(2006)87[2985:troeas]2.0.co;2
Bellier, E., Grøtan, V., Engen, S., Schartau, A. K., Herfindal, I., and Finstad, A. G. (2013). Distance decay of similarity, effects of environmental noise and ecological heterogeneity among species in the spatio-temporal dynamics of a dispersal-limited community. Ecography 37, 172–182. doi: 10.1111/j.1600-0587.2013.00175.x
Blanchet, F. G., Legendre, P., and Borcard, D. (2008). Forward selection of explanatory variables. Ecology 89, 2623–2632. doi: 10.1890/07-0986.1
Boix, D., Caiola, N., Cañedo-Argüelles, M., Gascón, S., Ibàñez, C., Nebra, A., et al. (2010). Avaluació de l’estat Ecològic de les Zones Humides i Ajust Dels Indicadors de Qualitat. Índexs QAELSe 2010, ECELS i EQAT. Barcelona: Agència Catalana de l’Aigua, Departament de Medi Ambient i Habitatge, Generalitat de Catalunya.
Boix, D., Sala, J., Quintana, X. D., and Moreno-Amich, R. (2004). Succession of the animal community in a Mediterranean temporary pond. J. N. Am. Benthol. Soc. 23, 29–49. doi: 10.1899/0887-3593(2004)023<0029:sotaci>2.0.co;2
Bortolini, J. C., da Silva, P. R. L., Baumgartner, G., and Bueno, N. C. (2019). Response to environmental, spatial, and temporal mechanisms of the phytoplankton metacommunity: comparing ecological approaches in subtropical reservoirs. Hydrobiologia 830, 45–61. doi: 10.1007/s10750-018-3849-8
Bougeard, S., and Dray, S. (2018). Supervised multiblock analysis in R with the ade4 package. J. Statist. Softw. 86, 1–17. doi: 10.18637/jss.v086.i01
Brendonck, L., and De Meester, L. (2003). Egg banks in freshwater zooplankton: evolutionary and ecological archives in the sediment. Hydrobiologia 491, 65–84. doi: 10.1023/A:1024454905119
Brendonck, L., Pinceel, T., and Ortells, R. (2017). Dormancy and dispersal as mediators of zooplankton population and community dynamics along a hydrological disturbance gradient in inland temporary pools. Hydrobiologia 796, 201–222. doi: 10.1007/s10750-016-3006-1
Brown, J. H., Hall, C. A. S., and Sibly, R. M. (2018). Equal fitness paradigm explained by a trade-off between generation time and energy production rate. Nat. Ecol. Evol. 2, 262–268. doi: 10.1038/s41559-017-0430-1
Castillo-Escrivà, A., Mesquita-Joanes, F., and Rueda, J. (2020). Effects of the temporal scale of observation on the analysis of aquatic invertebrate metacommunities. Front. Ecol. Evol. 8:561838. doi: 10.3389/fevo.2020.561838
Castillo-Escrivà, A., Aguilar-Alberola, J. A., and Mesquita-Joanes, F. (2017a). Spatial and environmental effects on a rock pool metacommunity depend on landscape setting and dispersal mode. Freshw. Biol. 62, 1004–1011. doi: 10.1111/fwb.12920
Castillo-Escrivà, A., Valls, L., Rochera, C., Camacho, C., and Mesquita-Joanes, F. (2017b). Disentangling environmental, spatial and historical effects on ostracod communities in shallow lakes. Hydrobiologia 787, 61–72. doi: 10.1007/s10750-016-2945-x
Castillo-Escrivà, A., Valls, L., Rochera, C., Camacho, A., and Mesquita-Joanes, F. (2017c). Metacommunity dynamics of Ostracoda in temporary lakes: overall strong niche effects except at the onset of the flooding period. Limnologica 62, 104–110. doi: 10.1016/j.limno.2016.11.005
Cayrou, J., and Céréghino, R. (2005). Life-cycle phenology of some aquatic insects: implications for pond conservation. Aquat. Conserv. Mar. Freshw. Ecosyst. 15, 559–571. doi: 10.1002/aqc.739
Chase, J. M. (2007). Drought mediates the importance of stochastic community assembly. Proc. Natl. Acad. Sci. U.S.A. 104, 17430–17434. doi: 10.1073/pnas.0704350104
Clappe, S., Dray, S., and Peres-Neto, P. R. (2018). Beyond neutrality: disentangling the effects of species sorting and spurious correlations in community analysis. Ecology 99, 1737–1747. doi: 10.1002/ecy.2376
Cottenie, K., Michels, E., Nuytten, N., and de Meester, L. (2003). Zooplankton metacommunity structure: regional vs local processes in highly interconnected ponds. Ecology 84, 991–1000. doi: 10.1890/0012-9658(2003)084[0991:zmsrvl]2.0.co;2
De Bie, T., De Meester, L., Brendonck, L., Martens, K., Goddeeris, B., Ercken, D., et al. (2012). Body size and dispersal mode as key traits determining metacommunity structure of aquatic organisms. Ecol. Lett. 15, 740–747. doi: 10.1111/j.1461-0248.2012.01794.x
Declerck, S. A. J., Coronel, J. S., Legendre, P., and Brendonck, L. (2011). Scale dependency of processes structuring metacommunities of cladocerans in temporary pools of High-Andes wetlands. Ecography 34, 296–305. doi: 10.1111/j.1600-0587.2010.06462.x
Domínguez, E., and Fernández, H. R. (2009). Macroinvertebrados Bentónicos Sudamericanos: Sistemática y Biología. Tucumán: Fundación Miguel Lillo.
Domis, L. N. D. S., Elser, J. J., Gsell, A. S., Huszar, V. M., Ibelings, B. W., Jeppesen, E., et al. (2013). Plankton dynamics under different climatic conditions in space and time. Freshw. Biol. 58, 463–482. doi: 10.1111/fwb.12053
Dray, S., Bauman, D., Blanchet, G., Borcard, D., Clappe, S., Guenard, G., et al. (2019). Adespatial: Multivariate Multiscale Spatial Analysis. R Package Version 0.3-7. Available online at: https://CRAN.R-project.org/package=adespatial (accessed November 12, 2019).
Dray, S., Legendre, P., and Peres-Neto, P. R. (2006). Spatial modelling: a comprehensive framework for principal coordinate analysis of neighbour matrices (PCNM). Ecol. Model. 196, 483–493. doi: 10.1016/j.ecolmodel.2006.02.015
Elías-Gutiérrez, M., Suárez-Morales, E., Gutiérrez-Aguirre, M., Silva-Briano, M., Granados-Ramírez, J., and Garfias-Espejo, T. (2008). Cladocera y Copepoda de las Aguas Continentales de México: Guía Ilustrada. Mexico City: Universidad Nacional Autonoma de México.
Escrivà, A., Poquet, J. M., and Mesquita-Joanes, F. (2015). Effects of environmental and spatial variables on lotic ostracod metacommunity structure in the Iberian Peninsula. Inland Waters 5, 283–294. doi: 10.5268/IW-5.3.771
Farjalla, V. F., Srivastava, D. S., Marino, N. A. C., Azevedo, F. D., Dib, V., Lopes, P. M., et al. (2012). Ecological determinism increases with organism size. Ecology 93, 1752–1759. doi: 10.1890/11-1144.1
Fernandes, I. M., Henriquez-Silva, R., Penha, J., Zuanon, J., and Peres-Neto, P. R. (2014). Spatiotemporal dynamics in a seasonal metacommunity structure is predictable: the case of floodplain-fish communities. Ecography 37, 464–475. doi: 10.1111/j.1600-0587.2013.00527.x
Fick, S. E., and Hijmans, R. J. (2017). WorldClim 2: new 1-km spatial resolution climate surfaces for global land areas. Intern. J. Climatol. 37, 4302–4315. doi: 10.1002/joc.5086
Finlay, B. J. (2002). Global dispersal of free-living microbial eukaryote species. Science 296, 1061–1063. doi: 10.1126/science.1070710
Fontaneto, D. (2011). Biogeography of Microscopic Organisms, Is Everything Small Everywhere?. Cambridge: Cambridge University Press.
Forró, L., Korovchinsky, N. M., Kotov, A. A., and Petrusek, A. (2008). Global diversity of cladocerans (Cladocera; Crustacea) in freshwater. Hydrobiologia 595, 177–184. doi: 10.1007/978-1-4020-8259-7_19
Frisch, D., and Green, A. J. (2007). Copepods come in first: rapid colonization of new temporary ponds. Fundamen. Appl. Limnol. Archiv. Hydrobiol. 168, 289–297. doi: 10.1127/1863-9135/2007/0168-0289
Fukami, T. (2015). Historical contingency in community assembly: integrating niches, species pools, and priority effects. Annu. Rev. Ecol Evol. Syst. 46, 1–23. doi: 10.1146/annurev-ecolsys-110411-160340
García-Girón, J., Heino, J., García-Criado, F., Fernández-Aláez, C., and Alahuhta, J. (2020). Biotic interactions hold the key to understanding metacommunity organisation. Ecography 43, 1180–1190. doi: 10.1111/ecog.05032
Grönroos, M., Heino, J., Siqueira, T., Landeiro, V. L., Kotanen, J., and Bini, L. M. (2013). Metacommunity structuring in stream networks: roles of dispersal mode, distance type and regional environmental context. Ecol. Evol. 3, 4473–4487. doi: 10.1002/ece3.834
Hanski, I., and Gilpin, M. (1991). Metapopulation dynamics: brief history and conceptual domain. Biol. J. Linnea. Soc. 42, 3–16. doi: 10.1111/j.1095-8312.1991.tb00548.x
Heino, J., Grönroos, M., Soininen, J., Virtanen, R., and Muotka, T. (2012). Context dependency and metacommunity structuring in boreal headwater streams. Oikos 121, 537–544. doi: 10.1111/j.1600-0706.2011.19715.x
Heino, J., Melo, A. S., Siqueira, T., Soininen, J., Valanko, S., and Bini, L. M. (2015). Metacommunity organisation, spatial extent and dispersal in aquatic systems: patterns, processes and prospects. Freshw. Biol. 60, 845–869. doi: 10.1111/fwb.12533
Holt, R. D. (1993). “Ecology at the mesoscale: the influence of regional processes on local communities,” in Species Diversity: Historical and Geographical Perspectives, eds R. Ricklefs and D. Schluter (Chicago: University of Chicago Press), 77–88.
Holt, R. D., Holyoak, M., and Leibold, M. A. (2005). “Future directions in metacommunity ecology,” in Metacommunities: Spatial Dynamics and Ecological Communities, eds M. A. Holyoak, A. Leibold, and R. D. Holt (Chicago: University of Chicago Press), 465–489.
Holzinger, A., and Karsten, U. (2013). Desiccation stress and tolerance in green algae: consequences for ultrastructure, physiological and molecular mechanisms. Front. Plant Sci. 4:327. doi: 10.3389/fpls.2013.00327
Hubbell, S. P. (2001). The Unified Neutral Theory of Biodiversity and Biogeography (MPB-32). Princeton: Princeton University Press.
Huber-Pestalozzi, G. (1976–1982). Das Phytoplankton Des SüSswassers: Systematik und Biologie. Stuttgart: Schweizerbart.
Janzen, D. H. (1967). Why mountain passes are higher in the tropics. Am. Nat. 101, 233–249. doi: 10.1086/282487
Jeffrey, S. W., and Humphrey, G. F. (1975). New spectrophotometric equations for determining chlorophylls a, b, c1 and c2 in higher plants, algae and natural phytoplankton. Biochem. Physiol. Pflanzen 167, 191–194. doi: 10.1016/S0015-3796(17)30778-3
Jeffries, M. (1988). Measuring Talling’s “elements of chance” in pond populations. Freshw. Biol. 20, 383–393. doi: 10.1111/j.1365-2427.1988.tb00464.x
Karanovic, I. (2012). Recent Freshwater Ostracods of the World: Crustacea, Ostracoda, Podocopida. Berlin: Springer Science & Business Media.
Kruskal, W. H., and Wallis, W. A. (1952). Use of ranks in one-criterion variance analysis. J. Am. Statist. Assoc. 47, 583–621. doi: 10.2307/2280779
Lahr, J., Diallo, A. O., Ndour, K. B., Badji, A., and Diouf, P. S. (1999). Phenology of invertebrates living in a temporary pond. Hydrobiologia 405, 189–205. doi: 10.1023/A:1003879611584
Lamanna, C., Blonder, B., Violle, C., Kraft, N. J. B., Sandel, B., Šímová, I., et al. (2014). Functional trait space and the latitudinal diversity gradient. Proc. Natl. Acad. Sci. U.S.A. 111, 13745–13750. doi: 10.1073/pnas.1317722111
Langenheder, S., Berga, M., Östman, Ö, and Székely, A. J. (2012). Temporal variation of β-diversity and assembly mechanisms in a bacterial metacommunity. ISME J. 6, 1107–1114. doi: 10.1038/ismej.2011.177
Legendre, P., and Gallagher, E. D. (2001). Ecologically meaningful transformations for ordination of species data. Oecologia 129, 271–280. doi: 10.1007/s004420100716
Leibold, M. A., and Chase, J. M. (2018). Metacommunity Ecology, V.59. Princeton: Princeton University Press.
Leibold, M. A., Holyoak, M., Mouquet, N., Amarasekare, P., Chase, J. M., Hoopes, M. F., et al. (2004). The metacommunity concept: a framework for multi-scale community ecology. Ecol. Lett. 7, 601–613. doi: 10.1111/j.1461-0248.2004.00608.x
Levins, R., and Culver, D. (1971). Regional coexistence of species and competition between rare species. Proc. Natl. Acad. Sci. U.S.A. 68, 1246–1248. doi: 10.1073/pnas.68.6.1246
López-Delgado, E. O., Winemiller, K. O., and Villa-Navarro, F. A. (2019). Do metacommunity theories explain spatial variation in fish assemblage structure in a pristine tropical river? Freshw. Biol. 64, 367–379. doi: 10.1111/fwb.13229
Meisch, C. (2000). Freshwater Ostracoda of Western and Central Europe. Heidelberg: Spektrum Akademischer Verlag.
Ng, I. S. Y., Carr, C. M., and Cottenie, K. (2009). Hierarchical zooplankton metacommunities: distinguishing between high and limiting dispersal mechanisms. Hydrobiologia 619, 133–143. doi: 10.1007/s10750-008-9605-8
Nnoli, H., Kyerematen, R., Adu-Acheampong, S., and Hynes, J. (2019). Change in aquatic insect abundance: evidence of climate and land-use change within the Pawmpawm River in Southern Ghana. Cogent Environ. Sci. 5, 1–14. doi: 10.1080/23311843.2019.1594511
Nursuhayati, A. S., Yusoff, F. M., and Shariff, M. (2013). Spatial and temporal distribution of phytoplankton in Perak Estuary, Malaysia, during monsoon season. J. Fish. Aquat. Sci. 8, 480–493. doi: 10.3923/jfas.2013.480.493
Oksanen, J., Blanchet, F. G., Friendly, M., Kindt, R., Legendre, P., McGlinn, D., et al. (2019). Vegan: Community Ecology Package. R Package Version 2.5-4. Available online at: https://Cran.R-project.org/package=vegan (accessed November 12, 2019).
Olmo, C., Armengol, X., and Ortells, R. (2012). Re-establishment of zooplankton communities in temporary ponds after autumn flooding: does restoration age matter? Limnologica 42, 310–319. doi: 10.1016/j.limno.2012.08.005
Padial, A. A., Ceschin, F., Declerck, S. A. J., De Meester, L., Bonecker, C. C., Lansac-Tôha, F. A., et al. (2014). Dispersal ability determines the role of environmental, spatial and temporal drivers of metacommunity structure. PLoS One 9:e111227. doi: 10.1371/journal.pone.0111227
Peres-Neto, P. R., Legendre, P., Dray, S., and Borcard, D. (2006). Variation partitioning of species data matrices: estimation and comparison of fractions. Ecology 87, 2614–2625. doi: 10.1890/0012-9658(2006)87[2614:vposdm]2.0.co;2
Pickett, S. T. A., Meiners, S. J., and Cadenasso, M. L. (2011). “Domain and propositions of succession theory,” in The Theory of Ecology, eds S. M. Scheiner and M. R. Willig (Chicago: University of Chicago Press), 185–216.
R Core Team (2019). R: A Language and Environment for Statistical Computing. Vienna: R Foundation for Statistical Computing.
Radzikowski, J. (2013). Resistance of dormant stages of planktonic invertebrates to adverse environmental conditions. J. Plankton Res. 35, 707–723. doi: 10.1093/plankt/fbt032
Rice, E. W., Baird, R. B., and Eaton, A. D. (1992). Standard Methods for the Examination of Water and Wastewater. Washington, DC: American Public Health Association.
Richter-Boix, A., Tejedo, M., and Rezende, E. L. (2011). Evolution and plasticity of anuran larval development in response to desiccation. A comparative analysis. Ecol. Evol. 1, 15–25. doi: 10.1002/ece3.2
Rojo, C., Mesquita-Joanes, F., Monrós, J. S., Armengol, J., Sasa, M., Bonilla, F., et al. (2016). Hydrology affects environmental and spatial structuring of microalgal metacommunities in tropical Pacific coast wetlands. PLoS One 11:e0149505. doi: 10.1371/journal.pone.0149505
Sammarco, P. W., and Strychar, K. B. (2009). Effects of climate change/global warming on coral reefs: adaptation/exaptation in corals, evolution in zooxanthellae, and biogeographic shifts. Environ. Bioindic. 4, 9–45. doi: 10.1080/15555270902905377
Sasa, M., Armengol, X., Bonilla, F., Mesquita-Joanes, F., Piculo, R., Rojo, C., et al. (2015). Seasonal wetlands in the Pacific coast of Costa Rica and Nicaragua: environmental characterization and conservation state. Limnetica 34, 1–16. doi: 10.23818/limn.34.01
Schulz, G., Siqueira, T., Stefan, G., and Roque, F. O. (2012). Passive and active dispersers respond similarly to environmental and spatial processes: an example from metacommunity dynamics of tree hole invertebrates. Fundament. Appl. Limnol. 181, 315–326. doi: 10.1127/1863-9135/2012/0365
Segers, H. (1995). Rotifera: vol. 2: The Lecanidae (Monogononta). Guides to the Identification of the Microinvertebrates of the Continental Waters of the World. North Duncan: Balogh Scientific Books.
Segers, H. (2008). Global diversity of rotifers (Rotifera) in freshwater. Hydrobiologia 595, 49–59. doi: 10.1007/s10750-007-9003-7
Soininen, J. (2014). A quantitative analysis of species sorting across organisms and ecosystems. Ecology 95, 3284–3292. doi: 10.1890/13-2228.1
Soininen, J., Kokocinski, M., Estlander, S., Kotanen, J., and Heino, J. (2007). Neutrality, niches, and determinants of plankton metacommunity structure across boreal wetland ponds. Écoscience 14, 146–154. doi: 10.2980/1195-6860(2007)14[146:nnadop]2.0.co;2
Soininen, J., Korhonen, J. J., Karhu, J., and Vetterli, A. (2011). Disentangling the spatial patterns in community composition of prokaryotic and eukaryotic lake plankton. Limnol. Oceanogr. 56, 508–520. doi: 10.4319/lo.2011.56.2.0508
Springer, M., Ramírez, A., and Hanson, P. (2010). Macroinvertebrados de agua dulce de Costa Rica I. Rev. Biol. Trop. 58:rbt.v58i4. doi: 10.15517/rbt.v58i4
Thomaz, S. M., Bini, L. M., and Bozelli, R. L. (2007). Floods increase similarity among aquatic habitats in river-floodplain systems. Hydrobiologia 579, 1–13. doi: 10.1007/s10750-006-0285-y
Thorp, J. H., and Covich, A. P. (2010). Ecology and Classification of North American Freshwater Invertebrates. London: Academic Press.
Tronstad, L. M., Tronstad, B. P., and Benke, A. C. (2007). Aerial colonization and growth: rapid invertebrate responses to temporary aquatic habitats in a river floodplain. J. N. Am. Benthol. Soc. 26, 460–471. doi: 10.1899/06-057.1
Vanschoenwinkel, B., Seaman, M., and Brendock, L. (2010). Hatching phenology, life history and egg bank size of fairy shrimp Branchipodopsis spp. (Branchiopoda, Crustacea) in relation to the ephemerality of their rock pool habitat. Ecology 44, 771–780. doi: 10.1007/s10452-010-9315-y
Vyverman, W. (1996). “The Indo-Malaysian North-Australian phycogeographical region revised,” in Biogeography of Freshwater Algae Developments in Hydrobiology, ed. J. Kristiansen (Dordrecht: Springer), 107–120. doi: 10.1007/978-94-017-0908-8_10
Wellborn, G. A., Skelly, D. K., and Werner, E. E. (1996). Mechanisms creating community structure across a freshwater habitat gradient. Annu. Rev. Ecol. Syst. 27, 337–363. doi: 10.1146/annrev.ecolsys.27.1.337
Wilson, D. S. (1992). Complex interactions in metacommunities, with implications for biodiversity and higher levels of selection. Ecology 73, 1984–2000. doi: 10.2307/1941449
Winegardner, A. K., Jones, B. K., Ng, I. S. Y., Siqueira, T., and Cottenie, K. (2012). The terminology of metacommunity ecology. Trends Ecol. Evol. 27, 253–254. doi: 10.1016/j.tree.2012.01.007
Wisnoski, N. I., Leibold, M. A., and Lennon, J. T. (2019). Dormancy in metacommunities. Am. Nat. 194, 135–151. doi: 10.1086/704168
Keywords: multi-taxon study, dbRDA, MEM analysis, dispersal limitation, species sorting, temporal effects, tropical limnology
Citation: Gálvez Á, Aguilar-Alberola JA, Armengol X, Bonilla F, Iepure S, Monrós JS, Olmo C, Rojo C, Rueda J, Rueda R, Sasa M and Mesquita-Joanes F (2020) Environment and Space Rule, but Time Also Matters for the Organization of Tropical Pond Metacommunities. Front. Ecol. Evol. 8:558833. doi: 10.3389/fevo.2020.558833
Received: 04 May 2020; Accepted: 24 September 2020;
Published: 22 October 2020.
Edited by:
Jani Markus Heino, Finnish Environment Institute (SYKE), FinlandReviewed by:
Man Kit Cheung, The Chinese University of Hong Kong, ChinaFábio Amodêo Lansac Toha, State University of Maringá, Brazil
Copyright © 2020 Gálvez, Aguilar-Alberola, Armengol, Bonilla, Iepure, Monrós, Olmo, Rojo, Rueda, Rueda, Sasa and Mesquita-Joanes. This is an open-access article distributed under the terms of the Creative Commons Attribution License (CC BY). The use, distribution or reproduction in other forums is permitted, provided the original author(s) and the copyright owner(s) are credited and that the original publication in this journal is cited, in accordance with accepted academic practice. No use, distribution or reproduction is permitted which does not comply with these terms.
*Correspondence: Ángel Gálvez, YW5nZWwuZ2FsdmV6QHV2LmVz