- 1Great Lakes Institute for Environmental Research, University of Windsor, Windsor, ON, Canada
- 2Department of Integrative Biology, University of Windsor, Windsor, ON, Canada
Elevated temperatures resulting from climate change are expected to disproportionately affect ectotherms given their biological function has a direct link to environmental temperature. Thus, as climate change leads to rapid increases in water temperatures in rivers, aquatic ectotherms, such as fish may be highly impacted. Organisms can respond to these stressors through flexible and rapid phenotypic change induced via developmental and/or transgenerational plasticity. In oviparous species, gravid females may translate environmental stress across generations via increased exposure of eggs to maternally derived glucocorticoids (i.e., maternal stress), which has been shown to result in diverse phenotypic effects in offspring. Recent studies suggest these phenotypic changes from maternal glucocorticoids may elicit predictive adaptive responses, where offspring exposed to maternal stress may be better prepared for the stressful environment they will encounter (i.e., environmental match hypothesis). We applied the environmental match hypothesis to examine whether a prenatal exogenous increase in egg cortisol may prepare Chinook salmon offspring (Oncorhynchus tshawytscha) to cope with thermal challenges after being reared in chronically elevated temperatures. Specifically, we exposed eggs to aqueous bath of cortisol-dosed (1,000 ng/mL) or control (0 ng/ml) solutions, and then raised both treatments at current (+0°C—contemporary ambient river temperature) or elevated (+3°C—projected future river temperature) thermal regimes. We quantified thermal performance in fish 7–9 month post fertilization using two methods: via critical thermal maximum (CTMax), and energetic responses (in plasma cortisol, glucose, and lactate) to environmentally relevant, but challenging thermal spikes over 3 days. Overall, we found that exposure to elevated rearing temperatures had a large impact on thermal tolerance, where elevated-temperature reared offspring had significantly higher CTMax. In comparison, egg cortisol treatment had little to no clear effects on CTMax and blood energetic response. Our study demonstrates the importance of elevated water temperatures as an inducer of offspring phenotypes (via early developmental cues), and highlights the significance of examining offspring performance in environments with ecologically relevant stressors.
Introduction
Climate change is a major contributor to rapid global changes, whether via increasing average temperatures (Solomon et al., 2012), or increasing frequency of extreme weather events (e.g., droughts: Rahmstorf and Coumou, 2011). Aquatic systems are expected to be highly impacted by climate change, not only through increasing average water temperatures, but by changing the hydrological cycle (e.g., increase in precipitation variation), which causes more extremes in water flow (e.g., droughts and floods), and by increasing daily temperature extremes (e.g., thermal spikes: Mantua et al., 2010; Woodward et al., 2016). Aquatic ectotherms such as reptiles, amphibians and most fish species are potentially sensitive to these alterations in temperature events, which may have lasting effects within and across generations (Deutsch et al., 2008; McCullough et al., 2009; Buckley and Huey, 2016). Indeed, juvenile ectotherms that develop in warmer temperatures have been shown to have altered phenotypes: smaller bodies (Cingi et al., 2010; Sheridan and Bickford, 2011; Whitney et al., 2014), altered immunity (Alcorn et al., 2002; Pérez-Casanova et al., 2008), and increased metabolism (Clarke and Johnston, 1999; Enders and Boisclair, 2016). These responses have been shown to impact performance (i.e., higher thermal tolerance: Bickford et al., 2010; Dillon et al., 2010; Sandblom et al., 2016, but see Chen et al., 2013) and fitness (i.e., survival: Martins et al., 2012; Rohr and Palmer, 2013).
To respond and persist within a rapidly changing world, species require mechanisms such as developmental plasticity (Hendry et al., 2008; Chevin et al., 2010), phenotypically flexible responses (Piersma and Drent, 2003; Franklin et al., 2007; Forsman, 2015), epigenetic inheritance (Lind and Spagopoulou, 2018), and contemporary evolution (Carroll et al., 2007) which act within and across generations. Environmentally induced plasticity can enable organisms to optimize growth, morphology, and physiology in response to current (or expected) environmental conditions to ultimately maximize performance, reproduction, and survival (Seebacher et al., 2014; Fox et al., 2019). Non-genetic maternal effects such as variation in egg quality (Sinervo, 1990; Bernardo, 1996; Einum and Fleming, 1999), variation in parental behavior (Champagne et al., 2003; Koch and Meunier, 2014), and traits such as maternal immune components (e.g., antibodies, Roth et al., 2018), and maternally derived hormones (Dantzer et al., 2013; Ruuskanen, 2015) have long been recognized for their potential to shape offspring phenotype and performance in response to current or expected environmental quality (Mousseau and Fox, 1998; Green, 2008). When a mother is exposed to a stressful environment during gestation or follicular recruitment, she may mount a stress response by elevating her glucocorticoid (GC) levels (Wingfield, 2013; Schreck and Tort, 2016). Recent research has examined the transfer of environmentally elevated GCs from mother to developing offspring (i.e., maternal stress) as a modulator of offspring phenotype and performance (Love et al., 2005, 2009). These GC-induced responses have been interpreted by some researchers as predictive adaptive responses in offspring expected to face with stressful environments (Gluckman et al., 2005; Marshall and Uller, 2007; Sheriff and Love, 2013). These types of adaptive response mechanisms have already been highlighted as potential drivers of flexible responses to warming environments (Meylan et al., 2012), but the role of maternal GCs as a signal of a stressor to offspring—such as warmer waters—has not been fully established. Exposure to increased maternal GCs has been shown to result in phenotypes expected to have lowered energetic demand (i.e., slower growth: Hayward and Wingfield, 2004, smaller body size: Love et al., 2005; Burton et al., 2011, and lower baseline energetics: Capelle, 2017), allowing offspring to outcompete individuals with faster growth or larger size in energetically demanding warmer waters (Messmer et al., 2017). However, this expected increase in performance of offspring exposed to increased maternal GCs within a harsher environment requires further testing (Sopinka et al., 2017). In species where there is spatial or temporal overlap in the maternal and offspring environment (Sheriff and Love, 2013), these stress-induced maternal effects may be particularly relevant for signaling offspring for stressful future environments (Capelle et al., 2017; Sopinka et al., 2017). The environmental match hypothesis suggests that when there is a match between the maternal and offspring environment (i.e., stressful maternal environment, and exposure to maternal stress via elevation in maternal GCs, respectively) the result may be higher than expected offspring performance and fitness. Although we already appreciate that elevated temperatures can lead to altered offspring phenotypes across generations (Burt et al., 2011; Jonsson and Jonsson, 2016; Le Roy et al., 2017), it is unclear whether phenotypes induced by maternal stress signals (via GCs) enable offspring to optimally respond to chronic elevated rearing temperature as well as rapid, extreme changes in water temperature, especially in at-risk species (Love et al., unpublished; Sopinka et al., 2017).
Here we examine the effects of exposure to mimicked prenatal GCs and altered rearing temperatures on the thermal performance of juvenile Chinook salmon (Oncorhynchus tshawytscha). Exploring whether Pacific salmon possess transgenerational stress-induced responses that may mitigate the effects of climate change is relevant from both a mechanistic and a conservation point of view. Mechanistically, Pacific salmon are ectothermic and are highly susceptible to thermal stressors (McCullough, 1999; Geist et al., 2006; Kuehne et al., 2012; Bowerman et al., 2018). Pacific salmon have the capacity to mount a GC stress response (i.e., elevated plasma GCs) to additional environmental stressors during migration and spawning (Cook et al., 2014). Since the maternal spawning and offspring development environments overlap spatially, maternal stress has the potential to act as a reliable signal of the offspring’s future environment (Healey, 1991). From a conservation standpoint, increasing river temperatures and higher frequency of droughts are predicted to highly impact cold-water species such as North American Pacific salmon (McCullough et al., 2009; Cunningham et al., 2018); multiple Pacific salmon species are in (or soon expected to be in) decline due to climate change (Crozier et al., 2008; Ford et al., 2011); and multiple populations of Chinook salmon in particular are in rapid decline (COSEWIC, 2018). Therefore, our study species and system offer an ideal combination in which to investigate environmental inducers and transgenerational signals of offspring stress. Predicting how the underlying regulatory physiology, in addition to thermotolerance capacity, will be impacted in Chinook salmon by future warming scenarios is very challenging. Recent experimental work in fish has suggested that while core physiological processes such as cardiorespiratory functions may indeed be thermally plastic, upper thermal tolerance limits may be much less flexible (Sandblom et al., 2016). Although even more difficult to predict, estimating the degree to which stress-induced transgenerational effects will further influence both of these important performance metrics remains imperative. Additional mechanistic (i.e., transcriptional profiling and physiological performance; e.g., Colson et al., 2019) studies examining the impact of thermally induced developmental plasticity in both these key systems, as well as measuring whole-animal performance under differing thermal scenarios (e.g., Farrell et al., 2008), will be required to answer these complex questions.
To examine how the effects of climate change interact with maternal GCs to generate plastic responses in Pacific salmon, we applied the environmental matching paradigm (Sheriff and Love, 2013). Specifically, we test the adaptive potential of altered offspring phenotypes (due to maternal stress and rearing temperature) to cope with environmentally relevant stressors such as increased rearing temperatures under climate change (Sheriff et al., 2017). Within this framework, we mimicked a maternal stress signal by exogenously elevating egg cortisol (via post-fertilization bathing method), and then raised resultant cortisol-dosed (and control) offspring under a current (+0°C) or an elevated (+3°C) temperature regime (Figure 1). At 7–9 months post fertilization we assessed the thermal performance of fish in two ways. First, we determined the CTMax of the fish, defined as the temperature at which fish lose equilibrium under steadily increasing water temperatures (Chen et al., 2013; McDonnell and Chapman, 2015). This is a standardized approach in the literature to approximate thermal tolerance (Becker and Genoway, 1979; Lutterschmidt and Hutchison, 1997). Second, we quantified the energetic response of the fish following 3 days of environmentally relevant, but challenging, thermal spikes in water temperature. Given previous work that found higher rearing water temperatures lead to higher thermal tolerance (Sandblom et al., 2016), we predicted offspring raised in elevated temperatures would have a higher thermal performance (i.e., higher CTMax, lower energetic cost) in both thermal performance metrics. Based on the environmental matching hypothesis, we predicted that cortisol-dosed offspring raised in elevated rearing temperatures would have a greater thermal performance than control-dosed offspring reared in the same elevated temperatures and facing the same acute thermal challenge.
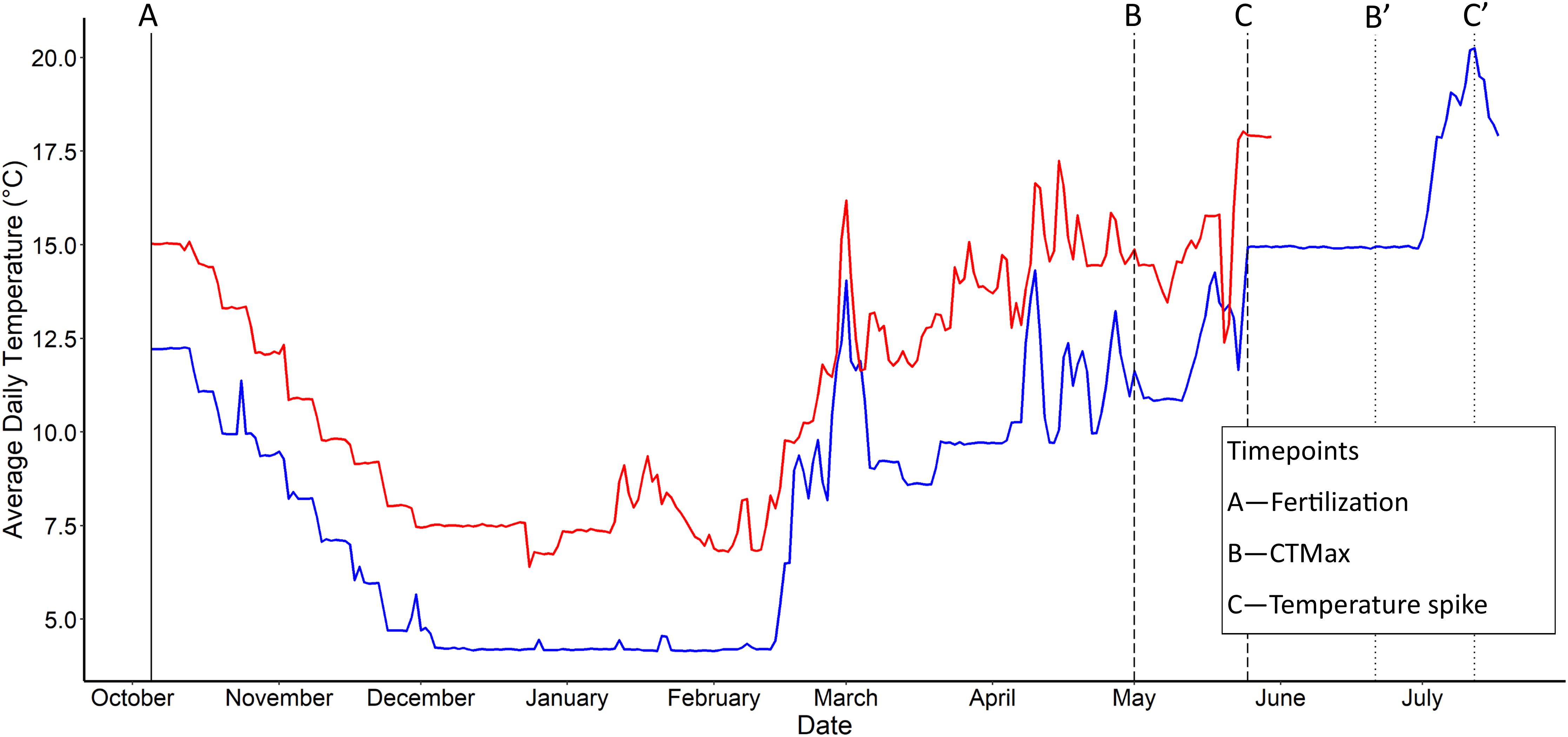
Figure 1. Mean daily water temperature for the two rearing temperature regimes (in aquatic facility) examined in Chinook salmon (Oncorhynchus tshawytscha). Blue—current temperature regime (0°C) and red—elevated temperature regime (+3°C). Vertical lines represent sampling time points: solid—both current and elevated groups; dashed, (B,C)—elevated, dotted, (B′,C′)—current. Time points are: (A)—Fertilization (Oct 4th), (B,B′)—CTMax start date (∼14°C), and (C,C′)—Thermal spike start date (∼18°C). Modified from Figure 2 found in Warriner et al. (2020); Copyright (©) 2020 Warriner, Semeniuk, Pitcher, and Love.
Materials and Methods
Fish Origins and Husbandry
On October 4th, 2016, we caught 15 adult female and 9 adult male spawning Chinook salmon from the Credit River, Ontario, Canada (43°34′40.0″N 79°42′06.3″W), stripped their gametes, and transferred the gametes to the University of Windsor on ice in coolers. We fertilized eggs from each female separately using pooled set of milt in 950 mL containers. We activated the sperm by using 60 mL of river water (Lehnert et al., 2018). Immediately following fertilization, we added river water mixed to 1,000 ng/mL of cortisol (H4001, Sigma-Aldrich Canada Co.) dissolved in 90% ethanol (HPLC grade, Sigma-Aldrich Canada Co.) or 0 ng/mL (ethanol and water only) to each container of eggs for our cortisol-dosed and control-dosed treatments respectively (8 containers per female: 4 cortisol-dosed and 4 control). The cortisol dose concentration was designed to increase egg cortisol levels within a biologically relevant range (within 2 SD of controls) based on previous studies (Auperin and Geslin, 2008; Sopinka et al., 2016; Capelle et al., 2017, reviewed in Sopinka et al., 2017). After a 2-h cortisol treatment, eggs were rinsed using dechlorinated water, and subset of 3 eggs per container were collected for cortisol treatment verification (See Warriner et al., 2020 for full methods and results). Eggs that were cortisol-dosed had (mean ± SD) 75.2 ± 42.4 ng/g cortisol, and control had 22.8 ± 25.4 ng/g. Each container of eggs was then further split into two cells (4-in × 3-in) within a vertical egg-incubation stack. To replicate our cortisol treatment, we placed 2 cortisol-dosed and 2 control treated containers from the same female in the same egg incubation stack (16 incubation cells per female). We then reared eggs under either current (+0°C) or elevated (+3°C) temperature regimes (one incubation stack per temperature treatment; see Figure 1). The current temperature regime was chosen to mimic water temperatures recorded in the Credit River through the Provincial Water Quality Monitoring Network from 2010 to 2014 (PWQMN: Ontario Ministry of Environment and Climate Change), while elevated rearing temperatures were chosen to reflect the higher end of climate change models for river temperatures (van Vliet et al., 2013; also see Zhang et al., 2018; Liu et al., 2020). This resulted in 4 treatment combinations in a 2 × 2 design: (1) current temperature reared—control, (2) current temperature reared—cortisol-dosed, (3) elevated temperature reared—control, and (4) elevated temperature reared—cortisol-dosed. Each female’s eggs were split across all four groups to account for maternal effects. All work described here was approved and completed under University of Windsor Animal Use Project Protocols (AUPPs: #14-25 and #15-15).
On Dec 23rd, 2016 and Feb 16th, 2017, for elevated- and current-reared temperature offspring respectively, we transferred fry at the exogenous-feeding stage (2–4 months post-fertilization) to 320 L recirculation-system housing tanks (five tanks per temperature treatment, with a separate system for each temperature treatment). We separated offspring by maternal identity and cortisol treatment using 10 L perforated buckets placed within the holding tanks (six buckets per tank). Buckets contained 100 offspring each (combined from replicate cortisol treatment containers and replicate incubation cells). During this period, water changes occurred at least daily to maintain water quality. The fish were housed under red light conditions following a 12:12 h light: dark cycle and were fed 3–4 times a day ad libitum. To ensure accurate temperature records, water temperatures in each stack were measured hourly (HOBO® Water Temperature Pro v2 Data Logger; Onset). During this period, the water temperature of the housing tanks continued to follow the current and elevated temperature seasonal regime (Figure 1). Due to mechanical error of an in-line chiller used to control the current-temperature treatment, housing temperatures were slowly raised with drop-in chillers to match that of the elevated-temperature treatment on March 3rd, 2017, to minimize stress. Since this overlap in temperature was for only 5 days until the chiller was repaired, and within the magnitude of temperature fluctuations found in riverine environments (Caissie, 2006), the effects of this period are expected to be minimal. Experiments were performed when fish were at similar accumulated thermal units (ATUs: Table 1), which has been shown to correspond with fish development (Neuheimer and Taggart, 2007).

Table 1. Starting dates for the CTMax and thermal spike experiments in Chinook salmon (Oncorhynchus tshawytscha) with the respective accumulated thermal units (ATUs).
CTMax
We evaluated the acute thermal tolerance of the fish across egg cortisol and rearing temperature treatments by determining their Critical Thermal Maximum (CTMax). CTMax is defined as the temperature at which fish lose equilibrium (i.e., unable to maintain an upright position) under steadily increasing water temperature (Becker and Genoway, 1979; Lutterschmidt and Hutchison, 1997). These trials occurred on May 1st–7th (elevated temperature) and on June 21st–26th, 2017 (current temperature). Two experimental tanks were run concurrently, and trials ran between 08:00 and 19:00 H. Within each experimental tank, four individuals were each placed in separate tapered perforated circular buckets (top diameter 28 cm × bottom diameter 16.5 cm × deep 28 cm), with individuals per experimental tank consisting of the same maternal identity, cortisol- and rearing temperature treatment (ntotal = 234 fish). Two air stones were used per experimental tank to ensure that dissolved oxygen levels remained high throughout the trials. These experimental tanks had the same water temperature as the housing tank at the start of the trial (± 0.7°C, starting temperature range = 13.2–15.1°C), which was controlled by an immersion circulating heater (SC100 Immersion Circulators: Thermo Fisher Scientific). At the end of the 1-h acclimation period, we increased temperature (by ∼0.2°C/min, = 0.20, range = 0.13–0.35; similar to rates in Becker and Genoway, 1979) until the fish lost equilibrium. We measured water temperatures throughout the trial, and we measured dissolved oxygen (DO) pre- and post-trial in a sub-sample of the trials (npre–trial DO = 40, pre–trial DO = 8.70 mg/L, npost–trial DO = 37, post–trial DO = 6.83 mg/L; LabQuest 2, stainless steel temperature probe, optical DO probe: Vernier). Our temperature probe measured temperature to 0.1°C with ± 0.2–0.5°C accuracy at 0–100°C, respectively. The trials were filmed under red light using low-light sensitivity cameras for later video analysis. After each trial, we euthanized the eight fishes using clove oil and pithing, and measured their body mass (to 0.01 g). During video analysis, the experimenter—blind to maternal identity and cortisol treatment—recorded the time when the fish lost equilibrium for a minimum of 10 consecutive seconds, and the temperature at which this occurred.
Energetic Response to Thermal Spikes
As our second measure of thermal performance, we quantified the energetic (physiological) coping response of fish in relation to the egg cortisol and rearing temperature treatments following 3 days of thermal spikes (+9°C—one spike per day). The maximum temperature of our thermal spike was chosen from the results of our CTMax experiment (slightly lower so that thermal spike was challenging but not affecting locomotion). The rate of increase in our environmentally relevant thermal spikes was chosen based on literature examining the effects of diel cycling and temperature spikes on fish energetics and metabolism (Tunnah et al., 2016; Corey et al., 2017; Gallant et al., 2017). Many of these studies have based their ramping protocols on in situ river temperature data. After our experiments, we were able to confirm similar spikes in temperature in the natal stream through water temperature loggers deployed in the Credit River from October 2016 to October 2017 (Figure 2). These trials occurred on May 25th–June 2nd and on July 12th–20th 2017 for the elevated and current treatments, respectively (nreplicate trials = 4; Figure 3). On the evening prior to the first day of thermal spiking, 32 fish were transferred from their housing tanks into two experimental tanks. We conducted two different temperature cycle treatments: first, the spiked group which experienced three environmentally relevant thermal spikes over the 3 days, whereas the second, the constant group, was maintained at a steady temperature (∼18°C, to act as a control for potential transfer stress). In the spiked temperature treatment, thermal spikes were increased and decreased at a rate 1°C/h, resulting in a +9°C temperature spike in 18 h. Within each tank, 4 perforated buckets (top diameter 28 cm × bottom diameter 16.5 cm × deep 28 cm) each contained 3–4 randomly selected fish from the same temperature and cortisol treatment over the experimental period (ntotal = 117). On the night of the final day, buckets were covered with opaque lids to reduce disturbance during sampling planned for the following morning. At 07:00 H of the 4th day, we removed the fish from their containers using a net, and collected their blood (within 3 min of first disturbance for each bucket) by caudal puncture using 10 μL heparinized microcapillary tubes. Each fish was then weighed (0.01 g) and placed in RNA-LaterTM for a future transcriptomics project (Finerty, 2020). We transferred the blood into heparinized microcentrifuge tubes and placed these on ice, and then measured blood glucose and lactate concentrations on-site from whole blood using handheld meters within 8–13 min of whole blood being collected (Freestyle Insulinx: Abbott Diabetes, precision: SD ± 0.1–0.3 on range 2.4–19.2 mmol/L; Lactate Plus: Nova Biochemical, precision SD ± 0.06–0.49 on range 1.6–22.1 mmol/L; Barkley et al., 2016; Beecham et al., 2006; Wells and Pankhurst, 1999). After 1 h on stored on ice, the microcentrifuge tubes were centrifuged at 10,000 rpm for 12 mins, and the plasma collected and stored at −80°C for later cortisol analysis. We assayed the plasma of juvenile fish for baseline cortisol levels using non-extracted plasma and a previously validated enzyme-linked immunosorbent assay (ELISA Cortisol Kit: Cayman Chemical; Capelle, 2017). We ran samples in triplicate at a 1:50 dilution. Assay plates were read at 412 nm on a plate reader, and intra- and inter-plate variation were 2.8 and 17.5%, respectively.
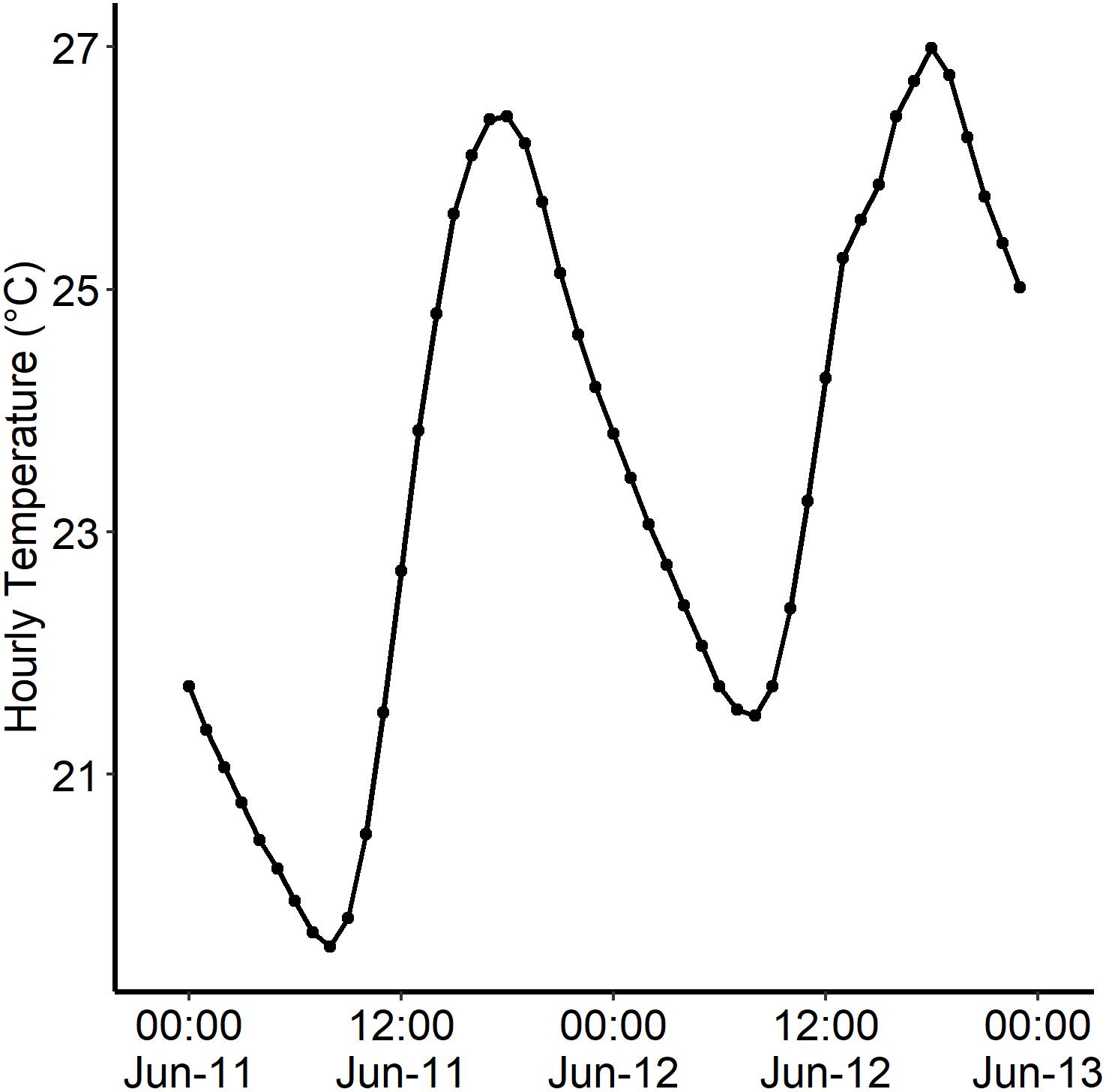
Figure 2. An example of a temperature spike from the Credit River (43°34′40.″N 79°42′06.3″W) during June 11th–13th 2017 (HOBO: Water temp pro v2). Credit River was the origin population for the Chinook offspring. Highest temperature (27.0°C) reached on June 12th, 2016 at 18:00 H.
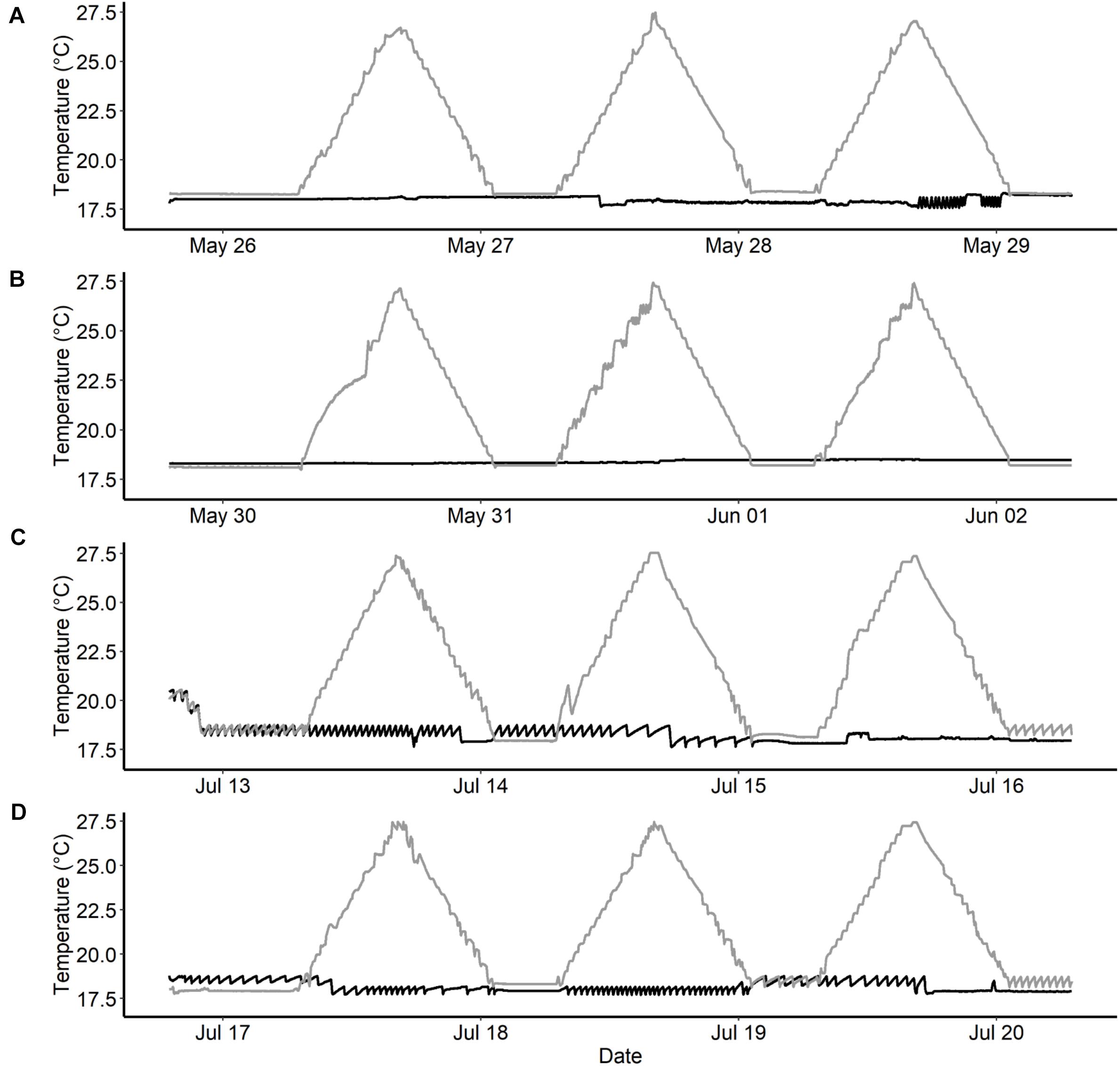
Figure 3. Temperature recording from temperature loggers (HOBO: Water temp pro v2) that measured water temperature once every minute during water thermal spike studies in Chinook salmon. Black lines represent the constant temperature groups (∼18°C) and the gray line represents the spike groups (+9°C per day). (A,B)—represents replicate rounds for fish reared at elevated temperatures, and (C,D)—represents replicate rounds for fish reared at current temperatures.
Statistical Analysis
We conducted all statistical analyses in R version 3.5.1 (R Core Team, 2018). We assessed model assumptions by graphical inspection: quantile-quantile plots of the residuals to verify normality, and residuals vs. fitted values were plotted to verify homogeneity. We transformed data when assumptions were not met using a log transformation, or when needed, a Box-Cox power transformation (Osborne, 2010) in the MASS package (Venables and Ripley, 2003). We ran linear mixed models (LMM) in the lme4 package (Bates et al., 2015; see Supplementary Table 1).
After visually plotting CTMax scores, we detected one individual score to be 3 median absolute deviations (MAD) from the median (median = 28.8°C, MAD = 0.48, datum = 26.6) and thus it was identified as a statistical outlier and removed from the dataset (Leys et al., 2013). This individual was at the smaller body mass end of our range (mass = 0.65 g), and in field notes was recorded as in poor body condition (i.e., frayed tail), which may have contributed to its earlier loss of equilibrium. For CTMax, we examined the interactive effects of rearing temperature and prenatal cortisol using a model that included the fixed effects of rearing temperature × cortisol treatment interaction, and their main effects (rearing temperature + cortisol treatment) and offspring body mass. We included testing tank, testing bucket (nested within tank), start temperature, and maternal identity as random effects for this model.
We analyzed the energetic response of offspring to temperature cycle (thermal spikes) using an LMM after transformation (plasma cortisol: Box-Cox, current—λ = 0.242, elevated—λ = 0.364: Osborne, 2010; glucose and lactate: log transformation). Since we a priori were interested in comparing energetic values within the same rearing temperature treatment, we separated the analyses into separate models for each rearing temperature. This decision also avoided the complexity of examining notoriously difficult to interpret 3-way interactions between egg cortisol treatment, water temperature treatment and the spike treatment. Within current and elevated temperature regimes, we tested models for response variables: cortisol, glucose, and lactate that included fixed effects of temperature cycle (constant or spiked), cortisol treatment, their interaction (temperature cycle × cortisol treatment), and body mass. Models also included random effects of replicate round and bucket ID.
We analyzed the interactions (CTMax: rearing temperature × cortisol treatment; thermal spikes: cortisol treatment × temperature cycle) in all models by fitting them with maximum likelihood (ML) estimations, and conducting a likelihood ratio test (LRT). If the interaction was significant (p < 0.05), the model was refitted with restricted maximum likelihood estimation (REML), and we conducted post-hoc analyses using false discovery rates (FDR, sharpened method) on pairwise comparison of interest (Verhoeven et al., 2005; Pike, 2011). Using this FDR post-hoc approach, we report q-values, which are adjusted p-values (Pike, 2011). We calculated q-values, using p-values from the emmeans package (Lenth, 2020) and using the Excel file from Pike (2011). We calculated the difference in marginal (variance of fixed effects only) and conditional (variance of fixed and random) R2 values of significant interactions against model without interaction (using MuMIn package; Nakagawa and Schielzeth, 2013; Barton, 2019), as a method of estimating the interaction effect size (this difference denoted by ΔR). If the interaction was instead determined to be non-significant (p > 0.05), it was removed from the model, and main effects were tested using LRT with ML estimations. After the final model was established for CTMax, we tested the effects of maternal identity in the model (included as a random factor) using LRT. Maternal identity was not added to the statistical model of the energetic response of offspring to the temperature cycle since maternal identity could not be tracked due to experimental constraints.
Results
CTMax
CTMax was influenced by rearing temperature, where fish that were raised in elevated temperatures had significantly higher CTMax than those raised in current temperatures (χ2 = 77.9, p < 0.001). There was also a marginally significant (at the 10% level—p < 0.1) effect of rearing temperature by cortisol treatment interaction on CTMax (LMM, LRT: χ2 = 2.92, p = 0.087; Figure 4 and Table 2). Cortisol dose alone did not significantly affect CTMax (χ2 = 0.13, p = 0.72). Body mass had a marginally significant effect (at the 10% level) on CTMax (χ2 = 2.78, p = 0.095), and thus was retained within the model. In the final model, maternal identity was a significant random effect for CTMax (χ2 = 14.0, p < 0.001, variance = 0.018; see Supplementary Table 2).
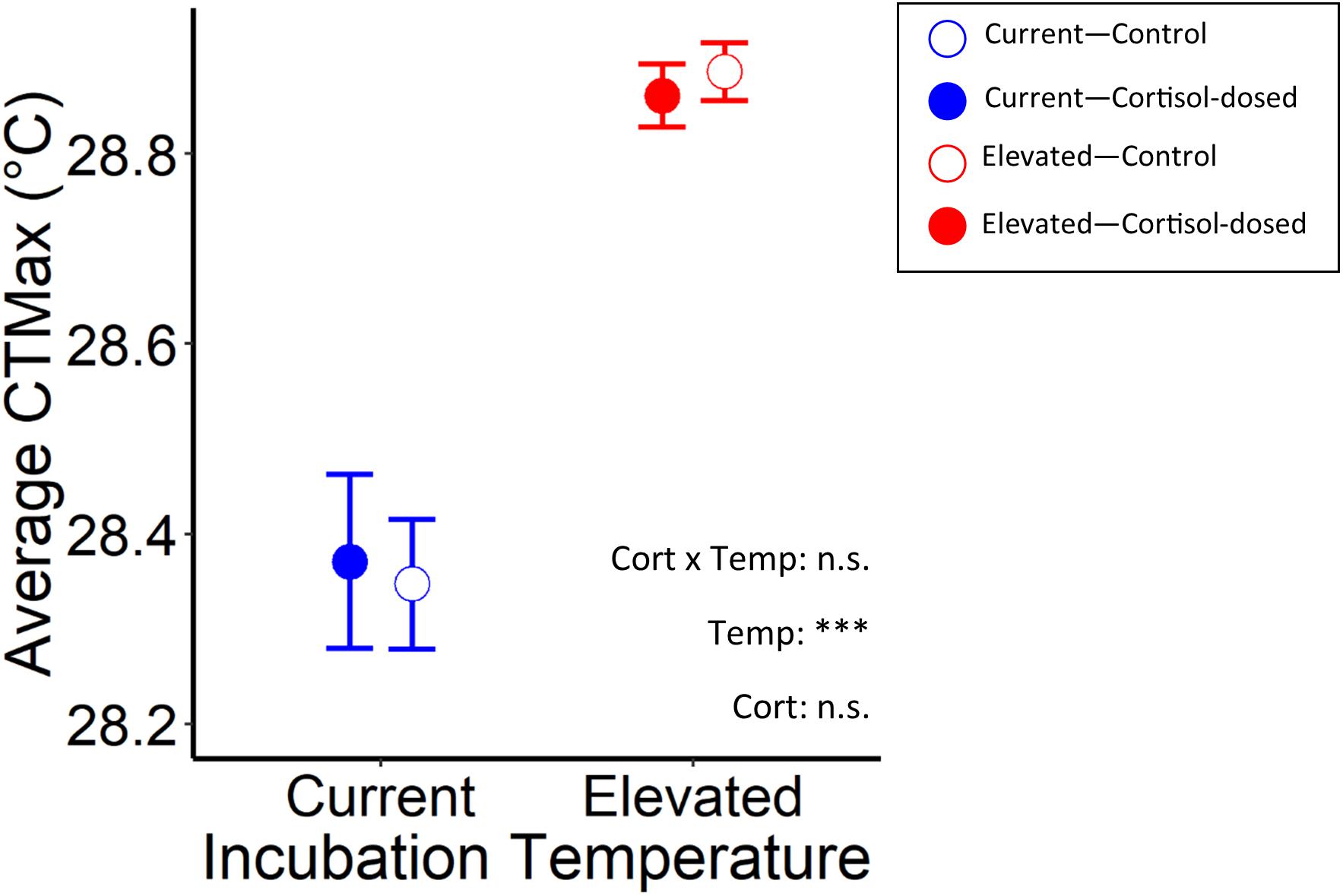
Figure 4. Effects of rearing temperature and prenatal cortisol on average CTMax in juvenile Chinook salmon exposed to control (open circles) and cortisol-exposed (closed circles) treatments and raised in current (blue), or elevated (red) temperature regimes (n.s. and *** represent p-values that were >0.05 and <0.001, respectively).
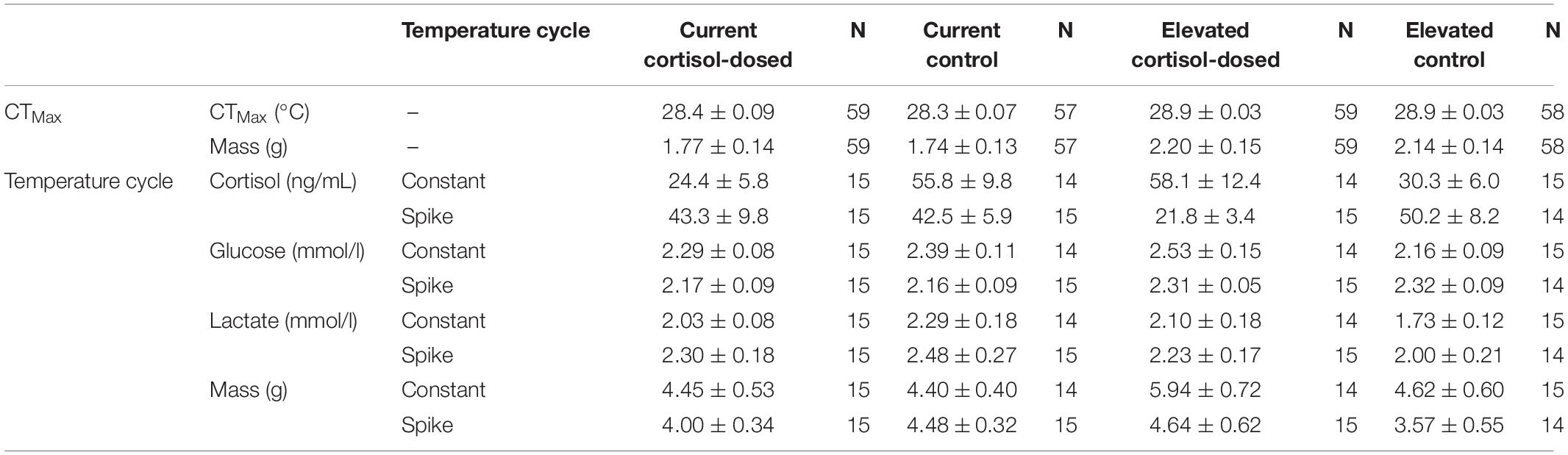
Table 2. Thermal tolerance performance and phenotype metrics (mean ± SE) of juvenile Chinook salmon.
Energetic Response to Thermal Spikes
Plasma Cortisol
Within the elevated temperature treatment, there was a significant cortisol treatment by temperature cycle interaction on plasma cortisol (LMM: χ2 = 4.47, p = 0.034; marginal, conditional R2 with interaction: 11.8%, 40.2%; without interaction: 0.82%, 39.7%, ΔR: 11.0%, 0.5%; Figure 5A and Table 2). Despite this overall global effect, FDR post-hoc analysis was unable to differentiate significant differences between all pairwise comparisons (q≥0.32), although the pattern of results suggests cortisol-exposed offspring exhibit lower plasma cortisol concentrations than control-dosed fish within the spiked temperature, and the opposite under the constant-temperature controls. Within the current water temperature treatment, the interaction of cortisol and spike treatments was non-significant (χ2 = 1.46, p = 0.23), nor was the main effect of temperature cycles on plasma cortisol levels (χ2 = 0.14, p = 0.71). However, cortisol treatment alone was marginally significant (at 10% level: χ2 = 2.90, p = 0.089), where cortisol-dosed offspring had lower plasma cortisol levels than control-dosed. Body mass did not have a significant effect on plasma cortisol in both rearing temperatures (elevated: χ2 = 0.01, p = 0.91; current: χ2 = 0.91, p = 0.34).
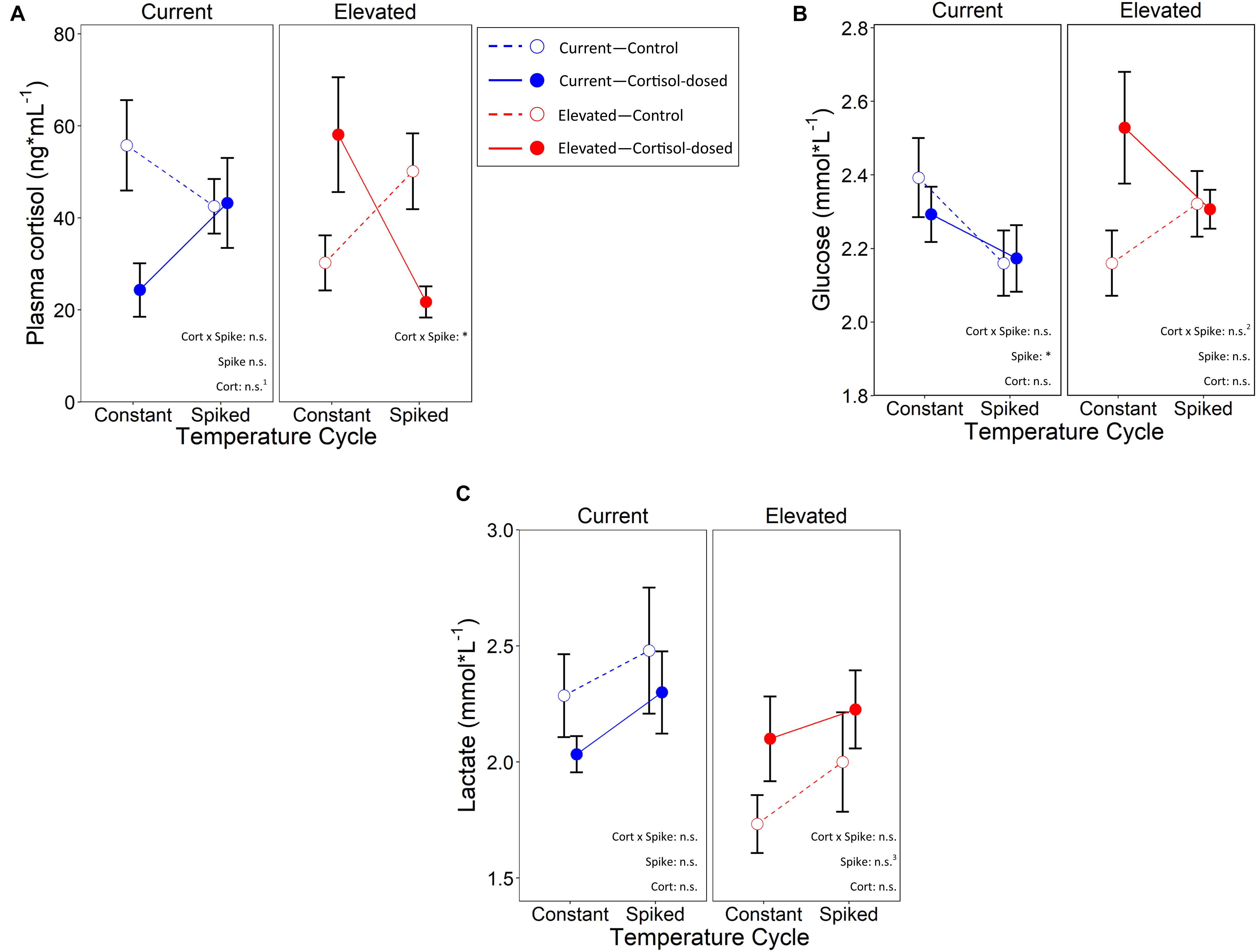
Figure 5. Effects of cortisol treatment and temperature cycle on (A)—plasma cortisol, (B)—whole blood glucose, and (C)—whole blood lactate in juvenile Chinook salmon. Open circles depict control and closed circles depict cortisol-dosed. For the temperature cycle groups, constant indicates fish remained at a constant temperature, and spiked are fish who were exposed to 3 days of thermal spikes. n.s. and *represent a p-value of >0.05 and <0.05, respectively. n.s.1, n.s.2, and n.s.3 represents a marginal significance of p = 0.089, p = 0.056, and p = 0.090 respectively.
Whole Blood Glucose
Within the elevated temperature treatment, there was a marginally significant effect of cortisol and temperature cycle interaction on glucose (LMM: χ2 = 3.66, p = 0.056; Figure 5B). However, when we examined cortisol and temperature cycle as main effects, they did not significantly affect blood glucose (cortisol: χ2 = 2.47, p = 0.12, spike: χ2 = 0.025, p = 0.88). Under the current temperature regime, glucose levels were not significantly impacted by cortisol and temperature cycle interaction (χ2 = 0.37, p = 0.54), nor cortisol treatment as a main effect (χ2 = 0.15, p = 0.70). However, temperature cycle as a main effect did significantly affect glucose (χ2 = 4.14, p = 0.042), where offspring in the spiked treatment had significantly lower glucose levels. Body mass did not have a significant effect on glucose in both rearing temperatures (elevated: χ2 = 0.93, p = 0.34; current: χ2 = 0.06, p = 0.81).
Whole Blood Lactate
Within the elevated temperature treatment, there was no cortisol by temperature cycle interaction on lactate concentrations (χ2 = 0.94, p = 0.63; Figure 5C), nor a main effect of cortisol treatment (χ2 = 2.48, p = 0.12). Temperature cycle had a marginally significant effect at the 10% level (χ2 = 2.90, p = 0.09), where fish that underwent the thermal spike treatment had marginally higher lactate levels. Overall lactate levels were also higher in fish with a larger body mass regardless of treatment (χ2 = 5.68, p = 0.017). Under the current rearing temperature, cortisol by temperature cycle interaction did not significantly affect lactate (χ2 = 0.21, p = 0.65). Lactate also did not significantly differ across cortisol treatment (χ2 = 1.02, p = 0.31), temperature cycle (χ2 = 0.86, p = 0.35), or with variation in body mass (χ2 = 1.57, p = 0.21).
Discussion
With climate change leading to increased water temperatures and elevated daily temperature fluctuations (see Introduction), we aimed to test whether maternal stress (i.e., exogenously increased egg cortisol) mitigates the effects of chronically elevated rearing temperatures to enhance offspring responses to extreme temperature variation (i.e., spikes) in juvenile salmon. Under the environmental match hypothesis (Love et al., 2013; Sheriff and Love, 2013), when offspring were reared under elevated water temperatures (stressful environment) we predicted that exposure to elevated egg cortisol (resulting from mimicking maternal stress) would improve thermal performance (environmental match) compared to control-dosed individuals (mismatch). Similar to established work on acclimation temperature (McDonnell and Chapman, 2015), we demonstrated that elevated rearing temperatures can indeed enhance offspring tolerance to rapid increases in temperature (i.e., CTMax). Contrary to our predictions, mimicking a signal of maternal stress (exogenous increase in egg cortisol) did not noticeably modulate offspring thermal sensitivity (both CTMax and energetic response) within either rearing temperature regime, with the exception of marginally lower plasma cortisol in cortisol-dosed offspring reared under current temperatures.
CTMax
Although rearing temperature impacted offspring maximum thermal tolerance, thermal tolerance was not further altered by exposure to elevated egg cortisol: fish raised in elevated temperatures had a higher mean CTMax than fish reared in current temperatures. Previous work has shown that short-term acclimation to higher temperatures results in a higher CTMax (Zhang and Kieffer, 2014; McDonnell and Chapman, 2015). Long-term rearing (from early development) in elevated temperatures also led to increased CTMax (He et al., 2014; Muñoz et al., 2017; Del Rio et al., 2019; but see Chen et al., 2013). Thus, acclimation to higher temperatures may allow for organisms to persist within a warmer world under climate change. However, acclimation to higher rearing temperature does not increase CTMax in a 1:1 ratio (i.e., a ceiling threshold exists; Sandblom et al., 2016), thus acclimation to higher temperatures may have a limited capacity to increase thermal tolerance. Unlike elevated rearing temperatures, exposure to elevated egg cortisol did not further augment juvenile salmon CTMax. To our knowledge, this is the first study to test the effects of prenatal stress (i.e., exogenously elevated egg cortisol) on CTMax. Previous studies have proposed that intergenerational or transgenerational effects may be a mechanism by which fish may increase their thermal tolerance under climate change (Munday, 2014), although exposure to exogenously elevated prenatal cortisol does not appear to be a significant maternal effect contributor to thermal tolerance and performance. Overall, we also found that CTMax was shaped by variation in juvenile body mass, where larger individuals had a higher CTMax. Mass has previously been shown to alter CTMax, with some studies reporting that larger fish (higher mass, length, or body condition) have higher CTMax, which is thought to be driven by higher energetic reserves (Chen et al., 2013; Gallant et al., 2017). While others have found the opposite, with smaller fish having higher CTMax, potentially due to the have lower oxygen limited energetic demand of smaller fish (Di Santo and Lobel, 2017; Messmer et al., 2017). Although not the main aim of our study, we also found maternal identity to be highly influential on offspring CTMax. Maternal effects are thought to play a significant role in determining how organisms respond to elevated temperatures (Burt et al., 2011), and our study provides further evidence that the role of maternal identity on offspring phenotype should be further studied.
Energetic Coping to Thermal Spikes
Overall, our study suggests that exposure to elevated egg cortisol may decrease plasma cortisol responses during thermal spikes for fish raised in elevated temperatures; however, these pair-wise, independent effects require further investigation as they were not detectable in post-hoc analysis. By rearing these fish under elevated temperatures, the offspring may be more prepared for thermal spikes, which is why we may not have seen an impact of thermal spikes on plasma cortisol. However, within the current (i.e., benign) temperature treatment, cortisol-dosed offspring had marginally lower plasma cortisol regardless of whether the offspring had undergone the thermal spike treatment. According to the environmental match hypothesis, the cortisol-exposed offspring should be mismatched to the current rearing environment, and yet, they had significantly lower baseline plasma cortisol (i.e., a lower energetic demand) than control fish. Previous work has shown that cortisol-dosed salmonid offspring had a lower plasma cortisol in a semi-natural postnatal environment (compared to low water conditions: Capelle, 2017) and in laboratory conditions compared to controls (Colson et al., 2015). However, for each group in the thermal spikes experiment, the average plasma cortisol was >25 ng/ml, with the exception of cortisol-dosed fish in the constant group reared at current temperatures (24.4 ng/mL). Compared to previous studies in juvenile Chinook salmon (Capelle, 2017; Dender et al., 2018), the combined average for cortisol-dosed offspring (33.9 ng/mL) is within the biologically relevant range for this species. This suggests that the lower plasma cortisol responses we report for the cortisol-dosed fish may be an adaptive energy-saving response compared to control offspring, which in turn may have long-term phenotypic consequences such as higher growth (Wendelaar-Bonga, 1997).
Among offspring reared under elevated temperature, there was a marginally significant (p < 0.06) interaction between cortisol dose and temperature cycle on blood glucose, suggesting cortisol-dosed offspring had higher blood glucose than controls when kept at constant temperatures. It is somewhat difficult to determine the origin of this response since having a higher glucose level may be indicative of both glucose mobilization as part of a stress response, or higher food intake (since fish were fed throughout the experiment; Polakof et al., 2012). Previous studies have found that fish cease feeding in warmer temperatures (Breau et al., 2011), and thus continued feeding may indicate that cortisol-dosed fish are retaining the ability to maintain homeostasis after exposure to a chronic thermal stressor. Regardless, the temperature cycle treatment itself did not impact blood glucose levels, suggesting that living in chronically warmer waters may allow fish to recover more quickly (i.e., via acclimation) to thermal spikes and maintain higher blood glucose levels. In support of this, Barton et al. (1987) found that rainbow trout (Oncorhynchus mykiss) exposed to 10 weeks of daily handling stress acclimated to additional handling and did not increase their glucose levels as a result. Similarly, a study comparing energetic responses to exposure between one and multiple thermal spikes found that after multiple thermal spikes, fish were able to maintain glucose levels by inducing anabolic metabolism and replenishing glycogen reserves (Callaghan et al., 2016). Since elevated-temperature reared fish were chronically exposed to increased temperatures, they may be using an anabolic phenotype to respond to thermal spikes, potentially allowing for the maintenance of higher glucose levels. Alternatively, when reared under current temperatures, fish undergoing thermal spikes had lower glucose levels than fish under stable temperatures, regardless of egg cortisol treatment. This suggests that fish undergoing the thermal spikes may have incurred an energetic cost, thus reducing their reserves and glucose homeostatic concentrations when raised in a benign environment.
Across both rearing temperatures, neither cortisol treatment nor temperature cycle influenced offspring whole blood lactate, although among fish reared in elevated temperatures, blood lactate was higher in fish with a larger body size. Since we ensured that oxygen levels remained high during the thermal spikes (via air stones), anaerobic metabolism may not have been necessary for the fish to persist at the higher, spiking temperatures, which is why we did not detect differences across temperature cycle treatments. Furthermore, since lactate is a by-product of anaerobic metabolism in muscle tissues (Dando, 1969), larger fish having higher lactate levels may be due to a higher proportion of muscle tissue available.
Potential for Maternal Stress to Adjust Offspring Thermal Performance
Overall, we did not find that a biologically relevant exogenous elevation of egg cortisol noticeably improved thermal tolerance in fish already raised under elevated water temperatures. Contrary to predictions of the environmental match hypothesis, elevated egg cortisol did not affect CTMax, nor did this treatment consistently interact with rearing temperature to clearly modulate the suite of energetic response in fish to thermal spikes. We did find that exposure to elevated egg cortisol led to increased blood glucose in offspring reared under elevated water temperatures, suggesting these offspring have more energy readily available to cope with additional thermal stressors within elevated temperatures. Maternal GCs are thought to enact phenotypic changes in offspring via GC-glucocorticoid receptor complex induced transcription and/or epigenetic programming (Love et al., 2013; Sopinka et al., 2017). Although prenatal stress has been shown to improve energetic responses to stressful postnatal environments (e.g., low water conditions: Capelle, 2017), and has been shown to alter a host of offspring traits (Sloman, 2010; Burton et al., 2011; Sopinka et al., 2017), our study suggests that maternally derived GCs may not noticeably improve thermal tolerance under elevated water temperature conditions in Chinook salmon. Thus, maternal GCs transferred to the egg may not be able to adjust the thermal tolerances of developing offspring via environmental matching, especially in combination with a powerful modulator of offspring development such as elevated rearing temperatures. However, we examined the effect of only one cortisol dose, which may have not affected offspring thermal tolerance because the chosen cortisol dose may not have matched the intensity of the chronically elevated rearing temperatures. To determine if a prenatal cortisol signal could improve offspring thermal tolerance under elevated temperatures, more research using a variety of prenatal cortisol doses is needed. By designing experiments using only mechanisms of transgenerational effects (such as maternal GCs, epigenetics, and maternal provisioning), researchers can differentiate between the paradigms of predictive adaptive responses and the silver spoon hypothesis (Engqvist and Reinhold, 2016). Other mechanisms of transgenerational effects (parental cues such as epigenetics and maternal provisioning) should be studied as they may allow for a mechanism by which environmental matching can improve offspring performance in warming waters (Munday, 2014; Donelan et al., 2020). In experiments when both parents and offspring are exposed to elevated temperatures, there is evidence for environmental matching which results in improved thermal tolerance in offspring (Sandblom et al., 2016; Le Roy et al., 2017; Le Roy and Seebacher, 2018). For example, Donelson et al. (2012) found that fish reared under elevated water temperatures were able to maintain their aerobic scope (an indicator of ability to do aerobic activities) if their parents were also raised in elevated temperatures. However, meta-analyses of transgenerational effects have shown mixed evidence for environmental matching inducing predictive adaptive responses (Uller et al., 2013; Yin et al., 2019). Thus, further testing the role of transgenerational stress within the environmental context of climate change remains imperative to determine the precise role of mechanisms such as maternally derived hormones (Meylan et al., 2012), and epigenetics (Anastasiadi et al., 2017; Ryu et al., 2018) in mediating adaptive responses to global warming. Future work should include examining which potential mechanisms of transgenerational effects may allow for environmental matching to improve offspring thermal tolerance by testing the effects of elevated temperatures on these parental cues. From an evolutionary perspective, our results show that offspring performance responses may be modulated by environmentally and ecologically relevant stressors (i.e., elevated rearing temperatures) experienced in early life. From an applied point of view, this project demonstrates that developmental plasticity (via early life environmental cues) may enable adaptive organismal responses to the effects of climate change.
Data Availability Statement
The raw data supporting the conclusions of this article will be made available by the authors, without undue reservation, to any qualified researcher.
Ethics Statement
The animal study was reviewed and approved by the Animal Care Committee, University of Windsor AUPP #14-25, #15-15.
Author Contributions
TW, CS, and OL collected the data. TW, CS, and OL ran statistical analysis and led writing of the manuscript with input from all authors. All authors contributed to the concept and design of the study.
Funding
CS and OL were supported by the Natural Sciences and Engineering Research Council of Canada (NSERC) Discovery Grants (Grant Numbers: 06724 and 06768). OL was supported by a Canada Research Chairs (Grant Number: 231897). TW was supported by the NSERC Canada Graduate Scholarships (Grant Number: 627684).
Conflict of Interest
The authors declare that the research was conducted in the absence of any commercial or financial relationships that could be construed as a potential conflict of interest.
Acknowledgments
We would like to thank K. Janisse, S. Currier, P. Capelle, C. Frank, J. Wong for their assistance with fish husbandry and experimental preparation, M. McCabe for assisting with CTMax, C. Harris for piloting equipment, and C. Finerty, I. Smith, S. Currier for aiding in thermal spikes experiment, with additional help from K. Janisse, C. Harris, L. Nguyen-Dang, S. Power, and P. Capelle for data collection. Data presented here was originally constituted as part of TW Master’s thesis (Warriner, 2019).
Supplementary Material
The Supplementary Material for this article can be found online at: https://www.frontiersin.org/articles/10.3389/fevo.2020.548939/full#supplementary-material
References
Alcorn, S. W., Murray, A. L., and Pascho, R. J. (2002). Effects of rearing temperature on immune functions in sockeye salmon (Oncorhynchus nerka). Fish Shellfish Immun. 12, 303–334. doi: 10.1006/fsim.2001.0373
Anastasiadi, D., Díaz, N., and Piferrer, F. (2017). Small ocean temperature increases elicit stage-dependent changes in DNA methylation and gene expression in a fish, the European sea bass. Sci. Rep. 7, 1–12. doi: 10.1038/s41598-017-10861-6
Auperin, B., and Geslin, M. (2008). Plasma cortisol response to stress in juvenile rainbow trout is influenced by their life history during early development and by egg cortisol content. Gen. Comp. Endocrinol. 158, 234–239. doi: 10.1016/j.ygcen.2008.07.002
Barkley, A. N., Cooke, S. J., Fisk, A. T., Hedges, K., and Hussey, N. E. (2016). Capture-induced stress in deep-water Arctic fish species. Polar Biol. 40, 213–220. doi: 10.1007/s00300-016-1928-8
Barton, B., Schreck, C., and Barton, L. (1987). Effects of chronic cortisol administration and daily acute stress on growth, physiological conditions, and stress responses in juvenile rainbow trout. Dis. Aquat. Organ. 2, 173–185. doi: 10.3354/dao002173
Bates, D., Mächler, M., Bolker, B. M., and Walker, S. C. (2015). Fitting linear mixed-effects using lme4. Psychol. Med. 45, 361–373. doi: 10.1017/S0033291714001470
Becker, C. D., and Genoway, R. G. (1979). Evaluation of the critical thermal maximum for determining thermal tolerance of freshwater fish. Env. Biol. Fish 4, 245–256. doi: 10.1007/BF00005481
Beecham, R. V., Small, B. C., and Minchew, C. D. (2006). Using portable lactate and glucose meters for catfish research: acceptable alternatives to established laboratory methods? N. Am. J. Aqu. 68, 291–295. doi: 10.1577/A05-074.1
Bernardo, J. (1996). The particular maternal effect of propagule size, especially egg size: patterns, models, quality of evidence and interpretation. Am. Zool. 36, 216–236. doi: 10.1093/icb/36.2.216
Bickford, D., Howard, S. D., Ng, D. J. J., and Sheridan, J. A. (2010). Impacts of climate change on the amphibians and reptiles of Southeast Asia. Biodivers. Conserv. 19, 1043–1062. doi: 10.1007/s10531-010-9782-4
Bowerman, T., Roumasset, A., Keefer, M. L., Sharpe, C. S., and Caudill, C. C. (2018). Prespawn mortality of female chinook salmon increases with water temperature and percent hatchery origin. Trans. Am. Fish. Soc. 147, 31–42. doi: 10.1002/tafs.10022
Breau, C., Cunjak, R. A., and Peake, S. J. (2011). Behaviour during elevated water temperatures: can physiology explain movement of juvenile Atlantic salmon to cool water? J. Anim. Ecol. 80, 844–853. doi: 10.1111/j.1365-2656.2011.01828.x
Buckley, L. B., and Huey, R. B. (2016). How extreme temperatures impact organisms and the evolution of their thermal tolerance. Integr. Comp. Biol. 56, 98–109. doi: 10.1093/icb/icw004
Burt, J. M., Hinch, S. G., and Patterson, D. A. (2011). The importance of parentage in assessing temperature effects on fish early life history: a review of the experimental literature. Rev. Fish Biol. Fish. 21, 377–406. doi: 10.1007/s11160-010-9179-1
Burton, T., Hoogenboom, M. O., Armstrong, J. D., Groothuis, T. G. G., and Metcalfe, N. B. (2011). Egg hormones in a highly fecund vertebrate: do they influence offspring social structure in competitive conditions? Funct. Ecol. 25, 1379–1388. doi: 10.1111/j.1365-2435.2011.01897.x
Caissie, D. (2006). The thermal regime of rivers: a review. Freshw. Biol. 51, 1389–1406. doi: 10.1111/j.1365-2427.2006.01597.x
Callaghan, N. I., Tunnah, L., Currie, S., and MacCormack, T. J. (2016). Metabolic adjustments to short-term diurnal temperature fluctuation in the rainbow Trout (Oncorhynchus mykiss). Physiol. Biochem. Zool. 89, 498–510. doi: 10.1086/688680
Capelle, P. M. (2017). Interactive Effects of Pre and Post Natal Stressors on Chinook Salmon Performance and Fitness. Master Thesis, University of Windsor, Windsor, ON.
Capelle, P. M., Semeniuk, C. A. D., Sopinka, N. M., Heath, J. W., and Love, O. P. (2017). Prenatal stress exposure generates higher early survival and smaller size without impacting developmental rate in a Pacific salmon. J. Exp. Zool. Part A Ecol. Genet. Physiol. 325, 641–650. doi: 10.1002/jez.2058
Carroll, S. P., Hendry, A. P., Reznick, D. N., and Fox, C. W. (2007). Evolution on ecological time-scales. Funct. Ecol. 21, 387–393. doi: 10.1111/j.1365-2435.2007.01289.x
Champagne, F. A., Francis, D. D., Mar, A., and Meaney, M. J. (2003). Variations in maternal care in the rat as a mediating influence for the effects of environment on development. Physiol. Behav. 79, 359–371. doi: 10.1016/S0031-9384(03)00149-5
Chen, Z., Anttila, K., Wu, J., Whitney, C., Hinch, S., and Farrell, A. (2013). Optimum and maximum temperature of sockeye salmon (Oncorhynchus nerka) populations hatched at different temperatures. Can. J. Zool. 91, 265–274. doi: 10.1139/cjz-2012-0300
Chevin, L. M., Lande, R., and Mace, G. M. (2010). Adaptation, plasticity, and extinction in a changing environment: towards a predictive theory. PLoS Biol. 8:e1000357. doi: 10.1371/journal.pbio.1000357
Cingi, S., Keinänen, M., and Vuorinen, P. J. (2010). Elevated water temperature impairs fertilization and embryonic development of whitefish Coregonus lavaretus. J. Fish Biol. 76, 502–521. doi: 10.1111/j.1095-8649.2009.02502.x
Clarke, A., and Johnston, N. M. (1999). Scaling of metabolic rate with body mass and temperature in teleost fish. J. Anim. Ecol. 68, 893–905. doi: 10.1046/j.1365-2656.1999.00337.x
Colson, V., Nguyen, T., Cousture, M., Damasceno, D., Le Cam, A., Bobe, J., et al. (2019). Maternal temperature exposure impairs emotional and cognitive responses and triggers dysregulation of neurodevelopment genes in fish. PeerJ 7:e6338. doi: 10.7717/peerj.6338
Colson, V., Valotaire, C., Geffroy, B., and Kiilerich, P. (2015). Egg cortisol exposure enhances fearfulness in larvae and juvenile rainbow trout. Ethology 121, 1–11.
Cook, K. V., Crossin, G. T., Patterson, D. A., Hinch, S. G., Gilmour, K. M., and Cooke, S. J. (2014). The stress response predicts migration failure but not migration rate in a semelparous fish. Gen. Comp. Endocrinol. 202, 44–49. doi: 10.1016/j.ygcen.2014.04.008
Corey, E., Linnansaari, T., Cunjak, R. A., and Currie, S. (2017). Physiological effects of environmentally relevant, multi-day thermal stress on wild juvenile Atlantic salmon (Salmo salar). Conserv. Physiol. 5:cox014. doi: 10.1093/conphys/cox014
COSEWIC (2018). COSEWIC Wildlife Species Assessments, November 2018. Available online at: https://www.canada.ca/content/dam/eccc/documents/pdf/cosewic/wsam-results/november-2018/2018-wildlife-species-assessments-detailed-november-en.pdf (accessed March 4, 2019).
Crozier, L. G., Hendry, A. P., Lawson, P. W., Quinn, T. P., Mantua, N. J., Battin, J., et al. (2008). Potential responses to climate change in organisms with complex life histories: evolution and plasticity in Pacific salmon. Evol. Appl. 1, 252–270. doi: 10.1111/j.1752-4571.2008.00033.x
Cunningham, C. J., Westley, P. A. H., and Adkison, M. D. (2018). Signals of large scale climate drivers, hatchery enhancement, and marine factors in Yukon River Chinook salmon survival revealed with a Bayesian life history model. Glob. Chang. Biol. 24, 4399–4416. doi: 10.1111/gcb.14315
Dando, P. R. (1969). Lactate metabolism in fish. J. Mar. Biol. Assoc. U.K. 49, 209–223. doi: 10.1017/S002531540004652X
Dantzer, B., Newman, A. E. M., Boonstra, R., Palme, R., Boutin, S., Humphries, M. M., et al. (2013). Density triggers maternal hormones that increase adaptive offspring growth in a wild mammal. Science 1215, 1215–1218. doi: 10.1126/science.1235765
Del Rio, A. M., Davis, B. E., Fangue, N. A., and Todgham, A. E. (2019). Combined effects of warming and hypoxia on early life stage Chinook salmon physiology and development. Conserv. Physiol. 7, 1–14. doi: 10.1093/conphys/coy078
Dender, M. G. E., Capelle, P. M., Love, O. P., Heath, D. D., Heath, J. W., and Semeniuk, C. A. D. (2018). Phenotypic integration of behavioural and physiological traits is related to variation in growth among stocks of Chinook salmon. Can. J. Fish. Aquat. Sci. 75, 2271–2279. doi: 10.1139/cjfas-2017-0367
Deutsch, C. A., Tewksbury, J. J., Huey, R. B., Sheldon, K. S., Ghalambor, C. K., Haak, D. C., et al. (2008). Impacts of climate warming on terrestrial ectotherms across latitude. Proc. Natl. Acad. Sci. U.S.A. 105, 6668–6672. doi: 10.1073/pnas.0709472105
Di Santo, V., and Lobel, P. S. (2017). Body size and thermal tolerance in tropical gobies. J. Exp. Mar. Bio. Ecol. 487, 11–17. doi: 10.1016/j.jembe.2016.11.007
Dillon, M. E., Wang, G., and Huey, R. B. (2010). Global metabolic impacts of recent climate warming. Nature 467, 704–706. doi: 10.1038/nature09407
Donelan, S. C., Hellmann, J. K., Bell, A. M., Luttbeg, B., Orrock, J. L., Sheriff, M. J., et al. (2020). Transgenerational plasticity in human-altered environments. Trends Ecol. Evol. 35, 115–124. doi: 10.1016/j.tree.2019.09.003
Donelson, J. M., Munday, P. L., McCormick, M. I., and Pitcher, C. R. (2012). Rapid transgenerational acclimation of a tropical reef fish to climate change. Nat. Clim. Chang. 2, 30–32. doi: 10.1038/nclimate1323
Einum, S., and Fleming, I. A. (1999). Maternal effects of egg size in brown trout (Salmo trutta): norms of reaction to environmental quality. Proc. R. Soc. B Biol. Sci. 266, 2095–2100. doi: 10.1098/rspb.1999.0893
Enders, E. C., and Boisclair, D. (2016). Effects of environmental fluctuations on fish metabolism: atlantic salmon Salmo salar as a case study. J. Fish Biol. 88, 344–358. doi: 10.1111/jfb.12786
Engqvist, L., and Reinhold, K. (2016). Adaptive trans-generational phenotypic plasticity and the lack of an experimental control in reciprocal match/mismatch experiments. Methods Ecol. Evol. 7, 1482–1488. doi: 10.1111/2041-210X.12618
Farrell, A. P., Hinch, S. G., Cooke, S. J., Patterson, D. A., Crossin, G. T., Lapointe, M., et al. (2008). Pacific salmon in hot water: applying aerobic scope models and biotelemetry to predict the success of spawning migrations. Physiol. Biochem. Zool. 81, 697–708. doi: 10.1086/592057
Finerty, C. J. (2020). A Transcriptomic Approach to Examining the Effects of Prenatal and Thermal Stress on Developmental Plasticity in Chinook Salmon (Oncorhynchus tshawytscha). Master’s thesis, University of Windsor, Windsor, ON.
Ford, M., Cooney, T., Mcelhany, P., Sands, N., Weitkamp, L., Hard, J., et al. (2011). Status Review Update for Pacific Salmon and Steelhead Listed Under the Endangered Species Act. NMFS-NWFSC-113. Washington, DC: U.S. Department of Commerce.
Forsman, A. (2015). Rethinking phenotypic plasticity and its consequences for individuals, populations and species. Heredity 115, 276–284. doi: 10.1038/hdy.2014.92
Fox, R. J., Donelson, J. M., Schunter, C., Ravasi, T., and Gaitán-Espitia, J. D. (2019). Beyond buying time: the role of plasticity in phenotypic adaptation to rapid environmental change. Philos. Trans. R. Soc. B Biol. Sci. 374:20180174. doi: 10.1098/rstb.2018.0174
Franklin, C. E., Davison, W., and Seebacher, F. (2007). Antarctic fish can compensate for rising temperatures: thermal acclimation of cardiac performance in Pagothenia borchgrevinki. J. Exp. Biol. 210, 3068–3074. doi: 10.1242/jeb.003137
Gallant, M. J., Leblanc, S., Maccormack, T. J., and Currie, S. (2017). Physiological responses to a short-term, environmentally realistic, acute heat stress in Atlantic salmon, Salmo salar. Facets 2, 330–341. doi: 10.1139/facets-2016-0053
Geist, D. R., Abernethy, C. S., Hand, K. D., Cullinan, V. I., Chandler, J. A., and Groves, P. A. (2006). Survival, development, and growth of Fall Chinook salmon embryos, alevins, and fry exposed to variable thermal and dissolved oxygen regimes. Trans. Am. Fish. Soc. 135, 1462–1477. doi: 10.1577/T05-294.1
Gluckman, P. D., Hanson, M. A., and Spencer, H. G. (2005). Predictive adaptive responses and human evolution. Trends Ecol. Evol. 20, 527–533. doi: 10.1016/j.tree.2005.08.001
Green, B. S. (2008). “Maternal effects in fish populations,” in Advances in Marine Bology, ed. D. W. Sims (Cambridge, MA: Academic press), 1–105. doi: 10.1016/s0065-2881(08)00001-1
Hayward, L. S., and Wingfield, J. C. (2004). Maternal corticosterone is transferred to avian yolk and may alter offspring growth and adult phenotype. Gen. Comp. Endocrinol. 135, 365–371. doi: 10.1016/j.ygcen.2003.11.002
He, Y., Wu, X., Zhu, Y., Li, H., Li, X., and Yang, D. (2014). Effect of rearing temperature on growth and thermal tolerance of Schizothorax (Racoma) kozlovi larvae and juveniles. J. Therm. Biol. 46, 24–30. doi: 10.1016/j.jtherbio.2014.09.009
Healey, M. C. (1991). “Life history of Chinook Salmon (Oncorhynchus tshawytscha),” in Pacific Salmon Life Histories, eds C. Groot and L. Margolis (Vancouver: University of British Columbia Press).
Hendry, A. P., Farrugia, T. J., and Kinnison, M. T. (2008). Human influences on rates of phenotypic change in wild animal populations. Mol. Ecol. 17, 20–29. doi: 10.1111/j.1365-294X.2007.03428.x
Jonsson, B., and Jonsson, N. (2016). Trans-generational maternal effect: temperature influences egg size of the offspring in Atlantic salmon Salmo salar. J. Fish Biol. 89, 1482–1487. doi: 10.1111/jfb.13040
Koch, L. K., and Meunier, J. (2014). Mother and offspring fitness in an insect with maternal care: phenotypic trade-offs between egg number, egg mass and egg care. BMC Evol. Biol. 14:125. doi: 10.1186/1471-2148-14-125
Kuehne, L. M., Olden, J. D., and Duda, J. J. (2012). Cost of living for juvenile Chinook salmon (Oncorhynchus tshawytscha) in an increasing warming and invaded world. Can. J. Fish. Aquat. Sci. 69, 1621–1630. doi: 10.1139/f2012-094
Le Roy, A., Loughland, I., and Seebacher, F. (2017). Differential effects of developmental thermal plasticity across three generations of guppies (Poecilia reticulata): canalization and anticipatory matching. Sci. Rep. 7, 1–12. doi: 10.1038/s41598-017-03300-z
Le Roy, A., and Seebacher, F. (2018). Transgenerational effects and acclimation affect dispersal in guppies. Funct. Ecol. 32, 1819–1831. doi: 10.1111/1365-2435.13105
Lehnert, S. J., Helou, L., Pitcher, T. E., Heath, J. W., and Heath, D. D. (2018). Sperm competition, but not major histocompatibility divergence, drives differential fertilization success between alternative reproductive tactics in Chinook salmon. J. Evol. Biol. 31, 88–97. doi: 10.1111/jeb.13199
Lenth, R. (2020). emmeans: Estimated Marginal Means, aka Least-Squares Means. R package version 1.4.7.
Leys, C., Ley, C., Klein, O., Bernard, P., and Licata, L. (2013). Detecting outliers: do not use standard deviation around the mean, use absolute deviation around the median. J. Exp. Soc. Psychol. 49, 764–766. doi: 10.1016/j.jesp.2013.03.013
Lind, M. I., and Spagopoulou, F. (2018). Evolutionary consequences of epigenetic inheritance. Heredity 121, 205–209. doi: 10.1038/s41437-018-0113-y
Liu, S., Xie, Z., Liu, B., Wang, Y., Gao, J., Zeng, Y., et al. (2020). Global river water warming due to climate change and anthropogenic heat emission. Glob. Planet. Change 193:103289. doi: 10.1016/j.gloplacha.2020.103289
Love, O. P., Chin, E. H., Wynne-Edwards, K. E., and Williams, T. D. (2005). Stress hormones: a link between maternal condition and sex-biased reproductive investment. Am. Nat. 166, 751–766. doi: 10.1086/497440
Love, O. P., Gilchrist, H. G., Bêty, J., Wynne-Edwards, K. E., Berzins, L., and Williams, T. D. (2009). Using life-histories to predict and interpret variability in yolk hormones. Gen. Comp. Endocrinol. 163, 169–174. doi: 10.1016/j.ygcen.2008.10.001
Love, O. P., McGowan, P. O., and Sheriff, M. J. (2013). Maternal adversity and ecological stressors in natural populations: the role of stress axis programming in individuals, with implications for populations and communities. Funct. Ecol. 27, 81–92. doi: 10.1111/j.1365-2435.2012.02040.x
Love, O. P., Rilett, A., and Capelle, P. M. (n.d.). Maternal stress modulates the effects of rearing environment enrichment on reintroduction phenotypes in Chinook salmon. Conserv. Physiol.
Lutterschmidt, W. I., and Hutchison, V. H. (1997). The critical thermal maximum: History and critique. Can. J. Zool. 75, 1561–1574. doi: 10.1139/z97-783
Mantua, N., Tohver, I., and Hamlet, A. (2010). Climate change impacts on streamflow extremes and summertime stream temperature and their possible consequences for freshwater salmon habitat in Washington State. Clim. Change 102, 187–223. doi: 10.1007/s10584-010-9845-2
Marshall, D. J., and Uller, T. (2007). When is a maternal effect adaptive? Oikos 116, 1957–1963. doi: 10.1111/j.2007.0030-1299.16203.x
Martins, E. G., Hinch, S. G., Patterson, D. A., Hague, M. J., Cooke, S. J., Miller, K. M., et al. (2012). High river temperature reduces survival of sockeye salmon (Oncorhynchus nerka) approaching spawning grounds and exacerbates female mortality. Can. J. Fish. Aquat. Sci. 69, 330–34212. doi: 10.1139/F2011-154
McCullough, D. A. (1999). A Review and Synthesis of Effects of Alterations to the Water Temperature Regime on Freshwater Life Stages of Salmonids, with Special Reference to Chinook Salmon. Seattle: U.S. Environmental Protection Agency.
McCullough, D. A., Bartholow, J. M., Jager, H. I., Beschta, R. L., Cheslak, E. F., Deas, M. L., et al. (2009). Research in thermal biology: burning questions for coldwater stream fishes. Rev. Fish. Sci. 17, 90–115. doi: 10.1080/10641260802590152
McDonnell, L. H., and Chapman, L. J. (2015). At the edge of the thermal window: effects of elevated temperature on the resting metabolism, hypoxia tolerance and upper critical thermal limit of a widespread African cichlid. Conserv. Physiol. 3, 1–13. doi: 10.1093/conphys/cov050
Messmer, V., Pratchett, M. S., Hoey, A. S., Tobin, A. J., Coker, D. J., Cooke, S. J., et al. (2017). Global warming may disproportionately affect larger adults in a predatory coral reef fish. Glob. Chang. Biol. 23, 2230–2240. doi: 10.1111/gcb.13552
Meylan, S., Miles, D. B., and Clobert, J. (2012). Hormonally mediated maternal effects, individual strategy and global change. Philos. Trans. R. Soc. L. B Biol. Sci. 367, 1647–1664. doi: 10.1098/rstb.2012.0020
Mousseau, T. A., and Fox, C. W. (1998). Maternal effects as adaptation. Trends Ecol. Evol. 13, 403–407. doi: 10.1017/CBO9781107415324.004
Munday, P. L. (2014). Transgenerational acclimation of fishes to climate change and ocean acidification. F1000Prime Rep. 6, 1–7. doi: 10.12703/p6-99
Muñoz, N. J., Farrell, A. P., Heath, J. W., and Neff, B. D. (2017). Hematocrit is associated with thermal tolerance and modulated by developmental temperature in juvenile chinook salmon. Physiol. Biochem. Zool. 91, 757–762. doi: 10.1086/695556
Nakagawa, S., and Schielzeth, H. (2013). A general and simple method for obtaining R2 from generalized linear mixed-effects models. Methods Ecol. Evol. 4, 133–142. doi: 10.1111/j.2041-210x.2012.00261.x
Neuheimer, A. B., and Taggart, C. T. (2007). The growing degree-day and fish size-at-age: the overlooked metric. Can. J. Fish. Aquat. Sci. 64, 375–385. doi: 10.1139/F07-003
Osborne, J. W. (2010). Improving your data transformations: applying the Box-Cox transformation. Pract. Assess. Res. Eval. 15, 1–9.
Pérez-Casanova, J. C., Rise, M. L., Dixon, B., Afonso, L. O. B., Hall, J. R., Johnson, S. C., et al. (2008). The immune and stress responses of Atlantic cod to long-term increases in water temperature. Fish Shellfish Immun. 24, 600–609. doi: 10.1016/j.fsi.2008.01.012
Piersma, T., and Drent, J. (2003). Phenotypic flexibility and the evolution of organismal design. Trends Ecol. Evol. 18, 228–233. doi: 10.1016/S0169-5347(03)00036-3
Pike, N. (2011). Using false discovery rates for multiple comparisons in ecology and evolution. Methods Ecol. Evol. 2, 278–282. doi: 10.1111/j.2041-210X.2010.00061.x
Polakof, S., Panserat, S., Soengas, J. L., and Moon, T. W. (2012). Glucose metabolism in fish: a review. J. Comp. Physiol. B 182, 1015–1045. doi: 10.1007/s00360-012-0658-7
R Core Team (2018). R: A Language and Environment for Statistical Computing. Vienna: R Foundation for Statistical Computing. Available online at: http://www.R-project.org/
Rahmstorf, S., and Coumou, D. (2011). Increase of extreme events in a warming world. PNAS 109, 17905–17909. doi: 10.1073/pnas.1114368109
Rohr, J. R., and Palmer, B. D. (2013). Climate change, multiple stressors, and the decline of ectotherms. Conserv. Biol. 27, 741–751. doi: 10.1111/cobi.12086
Roth, O., Beemelmanns, A., Barribeau, S. M., and Sadd, B. M. (2018). Recent advances in vertebrate and invertebrate transgenerational immunity in the light of ecology and evolution. Heredity 121, 225–238. doi: 10.1038/s41437-018-0101-2
Ruuskanen, S. (2015). Hormonally-mediated maternal effects in birds: lessons from the flycatcher model system. Gen. Comp. Endocrinol. 224, 283–293. doi: 10.1016/j.ygcen.2015.09.016
Ryu, T., Veilleux, H. D., Donelson, J. M., Munday, P. L., and Ravasi, T. (2018). The epigenetic landscape of transgenerational acclimation to ocean warming. Nat. Clim. Change 8, 504–509. doi: 10.1038/s41558-018-0159-0
Sandblom, E., Clark, T. D., Gräns, A., Ekström, A., Brijs, J., Sundström, L. F., et al. (2016). Physiological constraints to climate warming in fish follow principles of plastic floors and concrete ceilings. Nat. Commun. 7, 1–8. doi: 10.1038/ncomms11447
Seebacher, F., White, C. R., and Franklin, C. E. (2014). Physiological plasticity increases resilience of ectothermic animals to climate change. Nat. Clim. Chang. 5, 61–66. doi: 10.1038/nclimate2457
Sheridan, J. A., and Bickford, D. (2011). Shrinking body size as an ecological response to climate change. Nat. Clim. Chang. 1, 401–406. doi: 10.1038/nclimate1259
Sheriff, M. J., Bell, A., Boonstra, R., Dantzer, B., Lavergne, S. G., McGhee, K. E., et al. (2017). Integrating ecological and evolutionary context in the study of maternal stress. Integr. Comp. Biol. 57, 437–449. doi: 10.1093/icb/icx105
Sheriff, M. J., and Love, O. P. (2013). Determining the adaptive potential of maternal stress. Ecol. Lett. 16, 271–280. doi: 10.1111/ele.12042
Sinervo, B. (1990). The evolution of maternal investment in lizards: an experimental and comparative analysis of egg size and its effects on offspring performance. Evolution 44, 279–294. doi: 10.2307/2409407
Sloman, K. A. (2010). Exposure of ova to cortisol pre-fertilisation affects subsequent behaviour and physiology of brown trout. Horm. Behav. 58, 433–439. doi: 10.1016/j.yhbeh.2010.05.010
Solomon, S., Qin, D., Manning, M., Marquis, M., Averyt, K., Tignor, M. M. B., et al. (eds) (2012). Climate Change 2007: The Physical Science Basis Working Group I Contribution to the Fourth Assessment Report of the Intergovernment on Climate Change. Geneva: IPCC.
Sopinka, N. M., Capelle, P. M., Semeniuk, C. A. D., and Love, O. P. (2017). Glucocorticoids in fish eggs: variation, interactions with the environment, and the potential to shape offspring fitness. Physiol. Biochem. Zool. 90, 15–33. doi: 10.1086/689994
Sopinka, N. M., Hinch, S. G., Healy, S. J., Raby, G. D., and Patterson, D. A. (2016). Effects of experimentally elevated egg cortisol on offspring traits in two species of wild Pacific salmon. Environ. Biol. Fishes 99, 717–728. doi: 10.1007/s10641-016-0513-x
Tunnah, L., Currie, S., and MacCormack, T. J. (2016). Do prior diel thermal cycles influence the physiological response of Atlantic salmon (Salmo salar) to subsequent heat stress? Can. J. Fish. Aquat. Sci. 139, 1–13. doi: 10.1139/cjfas-2016-0157
Uller, T., Nakagawa, S., and English, S. (2013). Weak evidence for anticipatory parental effects in plants and animals. J. Evol. Biol. 26, 2161–2170. doi: 10.1111/jeb.12212
van Vliet, M. T. H., Ludwig, F., and Kabat, P. (2013). Global streamflow and thermal habitats of freshwater fishes under climate change. Clim. Change 121, 739–754. doi: 10.1007/s10584-013-0976-0
Venables, W. N., and Ripley, B. D. (2003). Modern applied statistics with S. Technometrics 45, 1–495. doi: 10.1198/tech.2003.s33
Verhoeven, K. J. F., Simonsen, K. L., and McIntyre, L. (2005). Implementing false discovery rate control: increasing your power. Oikos 108, 643–647. doi: 10.1111/j.0030-1299.2005.13727.x
Warriner, T. R. (2019). Prenatal Stress As a Potential Modulator of Offspring Survival, Phenotype, and Performance in Response to Elevated Temperatures. master’s thesis, University of Windsor, Windsor, ON.
Warriner, T. R., Semeniuk, C. A. D., Pitcher, T. E., and Love, O. P. (2020). Exposure to exogenous egg cortisol does not rescue juvenile Chinook salmon body size, condition, or survival from the effects of elevated water temperatures. Ecol. Evol. 10, 2466–2477. doi: 10.1002/ece3.6073
Wells, R. M. G., and Pankhurst, N. W. (1999). Evaluation of simple instruments for the measurement of blood glucose and lactate, and plasma protein as stress indicators in fish. J. World Aquac. Soc. 30, 276–284. doi: 10.1111/j.1749-7345.1999.tb00876.x
Wendelaar-Bonga, S. E. (1997). The stress response in fish. Physiol. Rev. 77, 591–625. doi: 10.1152/physrev.1997.77.3.591
Whitney, C. K., Hinch, S. G., and Patterson, D. A. (2014). Population origin and water temperature affect development timing in embryonic Sockeye salmon. Trans. Am. Fish. Soc. 143, 1316–1329. doi: 10.1080/00028487.2014.935481
Wingfield, J. C. (2013). Ecological processes and the ecology of stress: the impacts of abiotic environmental factors. Funct. Ecol. 27, 37–44. doi: 10.1111/1365-2435.12039
Woodward, G., Bonada, N., Brown, L. E., Death, R. G., Durance, I., Gray, C., et al. (2016). The effects of climatic fluctuations and extreme events on running water ecosystems. Philos. Trans. R. Soc. B 371:20150274. doi: 10.1098/rstb.2015.0274
Yin, J., Zhou, M., Lin, Z., Li, Q. Q., and Zhang, Y. Y. (2019). Transgenerational effects benefit offspring across diverse environments: a meta-analysis in plants and animals. Ecol. Lett. 22, 1976–1986. doi: 10.1111/ele.13373
Zhang, L., Zhao, Y., Hein-Griggs, D., and Ciborowski, J. J. (2018). Projected monthly temperature changes of the Great Lakes Basin. Environ. Res. 167, 453–467. doi: 10.1016/j.envres.2018.08.017
Keywords: maternal stress, prenatal stress, thermal stress, climate change, CTMax, plasma cortisol, glucose, lactate
Citation: Warriner TR, Semeniuk CAD, Pitcher TE, Heath DD and Love OP (2020) Mimicking Transgenerational Signals of Future Stress: Thermal Tolerance of Juvenile Chinook Salmon Is More Sensitive to Elevated Rearing Temperature Than Exogenously Increased Egg Cortisol. Front. Ecol. Evol. 8:548939. doi: 10.3389/fevo.2020.548939
Received: 04 April 2020; Accepted: 30 September 2020;
Published: 30 October 2020.
Edited by:
Olivia Roth, GEOMAR Helmholtz Center for Ocean Research Kiel, GermanyReviewed by:
Bram Kuijper, University of Exeter, United KingdomAnne Beemelmanns, Memorial University of Newfoundland, Canada
Copyright © 2020 Warriner, Semeniuk, Pitcher, Heath and Love. This is an open-access article distributed under the terms of the Creative Commons Attribution License (CC BY). The use, distribution or reproduction in other forums is permitted, provided the original author(s) and the copyright owner(s) are credited and that the original publication in this journal is cited, in accordance with accepted academic practice. No use, distribution or reproduction is permitted which does not comply with these terms.
*Correspondence: Theresa R. Warriner, d2FycmluZXRAdXdpbmRzb3IuY2E=; d2FycmludHJAZ21haWwuY29t