Corrigendum: Plant-Induced Transgenerational Plasticity Affecting Performance but Not Preference in a Polyphagous Moth
- 1Division of Chemical Ecology, Department of Plant Protection Biology, Swedish University of Agricultural Sciences, Uppsala, Sweden
- 2Biodiversity and Crop Improvement Program, International Center of Agricultural Research in the Dry Areas, Rabat, Morocco
- 3Department of Evolutionary Neuroethology, Max Planck Institute for Chemical Ecology, Jena, Germany
Environmental variation experienced by a single genotype can induce phenotypic plasticity in various traits, such as behavioural, physiological and developmental characteristics. It can occur within the lifetime of an individual through within-generation phenotypic plasticity (WGP) or vertically across generations through transgenerational phenotypic plasticity (TGP). However, knowledge about TGP and the co-occurrence of WGP and TGP is still limited. In insect host-plant selection, the ability to alter phenotypic traits through WGP is well documented while the importance of TGP and the possible co-occurrence between the two is largely unknown. Host-plant selection of both larvae and adults of the polyphagous moth Spodoptera littoralis can be modified by previous experience through WGP. Thus, the aim of this study was to investigate if parental host-plant experience can influence host-plant choice behaviour and performance of S. littoralis offspring through TGP. For this, we tested effects of rearing parents on different host plants on the offspring’s first instar larval migration and host plant choice, larval development and adult oviposition. A transgenerational effect on larval development was found, with increased pupal weight on a matching host-plant diet to that of the parent, when larvae were reared on cotton (good larval host plant) while no such effect was found on maize (poor larval host plant). These findings indicate that TGP of S. littoralis progeny development traits may only occur under favourable conditions. Parental diet did not affect larval host plant choice or migration. Furthermore, no effect of parental diet was found on offspring oviposition behaviour, indicating that adult female host-plant selection is governed by innate preference hierarchy and WGP, rather than TGP. Thus, parental diet may influence offspring performance but not behaviour, indicating that WGP is most important for host-plant selection behaviours in S. littoralis, but TGP can affect progeny development. If so, the importance of different types of plasticity may vary among traits of S. littoralis associated with host plant utilisation.
Introduction
Phenotypic plasticity is the ability of individual genotypes to modify traits, such as physiological, morphological and behavioural characteristics, quickly in response to biotic and abiotic environmental variation (West-Eberhard, 1989; Agrawal, 2001a; Whitman and Agrawal, 2009). It can increase the fitness of individuals in their experienced environments (Lande, 2009), and may include changes in both behaviour and development within the lifetime of an organism. Such within-generation plasticity (WGP) has been observed in diverse taxa, and theoretical models of the phenomenon have been widely supported with empirical data (West-Eberhard, 1989; Lande, 2009). Furthermore, phenotypic traits can be transferred vertically across generations through transgenerational plasticity (TGP), a non-genetic process that has been described in various ways, e.g., as parental effects, maternal effects, paternal effects, non-genetic inheritance, epigenetic inheritance, and prenatal learning (Mousseau and Fox, 1998; Uller, 2008; Bonduriansky et al., 2012; Peralta Quesada and Schausberger, 2012). TGP has been documented in plants (Herman and Sultan, 2011), vertebrates (Salinas and Munch, 2012), and invertebrates (Mousseau and Fox, 1998). However, there is still much less evidence of TGP than WGP, and theoretical models of TGP are not well supported by empirical data. Thus, there are substantial gaps in knowledge of TGP’s roles and importance (Bonduriansky et al., 2012). Some empirical support for TGP in morphological and physiological traits has been reported (Herman and Sultan, 2011; Donelson et al., 2018; Yin et al., 2019). For example, herbivory of Raphanus raphanistrum plants may increase their offspring’s leaf trichome density (Agrawal et al., 1999; Agrawal, 2001b) and changes in defensive features, earlier maturation and increased reproductive output have been observed in progeny of Daphnia parents exposed to predator cues (Agrawal et al., 1999; Walsh et al., 2015). Effects of parental experience on offspring behaviour have also been found, for example, fear conditioning in mice (Dias and Ressler, 2014) and feeding preferences of predatory mites (Peralta Quesada and Schausberger, 2012). However, although behaviour is considered to show high phenotypic plasticity, and organisms’ behavioural traits are often the first to change in response to environmental changes (West-Eberhard, 1989), effects of TGP on behaviour have received less attention.
Predictive models have indicated that both WGP and TGP are favoured by spatial and temporal environmental heterogeneity, environmental cues that are reliable predictors of upcoming environmental conditions, and low costs of plasticity (Uller, 2008; Bonduriansky et al., 2012; Dury and Wade, 2019). However, there are differences in the theoretical frameworks regarding the kinds of conditions that favour WGP, TGP and their possible co-occurrence. Studies on Daphnia spp. have supported the decoupling of WGP and TGP, indicating that selective pressures tend to favour either one or the other (Walsh et al., 2015, 2016). It has also been argued that if either WGP or TGP can optimise a trait there is no need for a combination of the two (Donelson et al., 2018). Contrarily, other models have suggested that WGP and TGP can co-exist and that information from environmental cues can be integrated for a specific phenotypic trait (Leimar and McNamara, 2015). Empirical support for this theory has been provided, e.g., by indications of their co-occurrence in Daphnia defence mechanisms and development (Agrawal et al., 1999; Mikulski and Pijanowska, 2010) and drought adaptations of Polygonium persicaria (Sultan et al., 2009). Furthermore, it has been suggested that species with high ability to express WGP should also have high ability to express TGP (Woestmann and Saastamoinen, 2016), thus potentially favouring their co-occurrence.
Behavioural changes in foraging and host-finding induced by WGP have been well documented in various insects, including parasitoids (Turlings et al., 1993), honeybees (Menzel and Müller, 1996) and herbivores (Anderson and Anton, 2014). There is also evidence of insects’ morphological and physiological traits being altered by TGP (Woestmann and Saastamoinen, 2016; Donelson et al., 2018). For example, parental exposure to UV light has been shown to affect wing coloration of offspring of the butterfly Papilio polytes (Katoh et al., 2018). Moreover, feeding on host plants of similar quality to plants their parents fed on has been found to promote development of offspring of both Coenonympha pamphilus and Pieris rapae (Cahenzli and Erhardt, 2013; Cahenzli et al., 2015). In addition, a study on the moth Bicyclus anynana showed that offspring preference for a synthetic odour was increased if the parents were reared on plant material coated with high doses of the same odour (Gowri et al., 2019). However, studies on effects of TGP on behaviour, particularly host-plant choice, using natural plant material are still lacking.
The Egyptian cotton leaf worm, Spodoptera littoralis Boisduval (Lepidoptera: Noctuidae), is a generalist phytophagous insect (Pogue, 2002), with an innate preference hierarchy for host plants that can be modified by WGP in both larval and adult stages. Larval host plant feeding experience has been found to induce a preference for the experienced host plant in later larval stages (Carlsson et al., 1999), and subsequent adult moth oviposition and mating behaviour (Anderson et al., 2013; Thöming et al., 2013; Proffit et al., 2015). Moreover, mating experience affects subsequent reproductive behaviour of both male and female adults (Proffit et al., 2015). As the importance of WGP for host plant choice in S. littoralis is well established it provides a good model to investigate the occurrence of TGP and possible interactions between, and co-occurrence of, WGP and TGP. Thus, the objective of this study was to investigate whether TGP, induced by parental experience to host plants, can affect preferences and performance of the species’ offspring. First, we investigated whether first instar larval host-plant choice and migratory behaviour are influenced by parental diet. We then followed the performance of larvae reared on the parental host plant or a different plant in a cross-comparison experiment. Finally, we tested whether the oviposition preference of the offspring was influenced by the parental diet.
Materials and Methods
Plants and Insects
Plants of three species – cotton (Gossypium hirsutum, cv. Delta Pineland 90, Malvaceae), cowpea (Vigna unguiculata subsp. Unguiculata, Fabaceae) and maize (Zea mays, cv. Sweet Nugget, Poaceae) – were used in this study. They were cultivated until use (before flowering) in experiments in a commercial substrate (Kronmull, Weibull Trädgård AB, Hammenhög, Sweden) in 1.5 L pots for 5–6 weeks at 25 ± 2°C, 70 ± 5% RH in a greenhouse with artificial light provided by Osram Powerstar HQI-T, 400 W/D lamps in 16:8 h L:D cycles.
The rearing strain of S. littoralis was founded from moths collected in Alexandria, Egypt, in 2008 and has been regularly refreshed with new wild-collected specimens from Egypt. Larvae were fed a potato-based artificial diet (Hinks and Byers, 1976) and kept at 25 ± 2°C, RH 65 ± 2% with 17:7 h L:D cycles. Adults were kept at 25 ± 2°C, RH 50 ± 2% with 16:8 h L:D cycles. Adult males and females were separated at the pupal stage and kept separate until mating.
Adult Oviposition Preference Rearing Procedure
The hypothesis that parental experience of this moth may affect oviposition preference of the offspring was tested in a four-generation rearing experiment, as follows. First-generation (F0) insects were reared on the artificial diet. Resulting pupae were sexed and kept separate. Sugar solution was provided as an energy source for the merging adults. Two to three days after hatching, single couples were mated in the absence of plants (F0). Mating of all replicates was observed and directly after mating the females were introduced to a cage with cotton plants to lay eggs. The offspring was then fed on cotton and adult females were introduced to a cage with cotton plants to mate and lay eggs. This was repeated for three generations (F1–F3). Females of the fourth generation were introduced to either cages with cotton plants or in the absence of plants for mating and oviposition, creating two separate rearing lines. One, consisting of larvae from eggs laid on cotton, was kept on cotton for the fourth generation (designated F4 cotton) while larvae of the other line, from eggs laid in cages with no plants, were kept on artificial diet (F4 artificial diet).
Adult Oviposition Preference
In this experiment, the females were allowed to choose to lay eggs on either cotton or cowpea plants. Larval experience of feeding on cotton has been previously shown to induce a preference for cotton over the innately preferred cowpea (Thöming et al., 2013). Females that had not been exposed to plant odours as adults of F0, F1, F2, F3, F4 generations fed on cotton and F4 fed on artificial diet were mated with unexposed males from the same feeding background and put in mating cages (length and width 28 cm; height 29 cm, N = 25 per treatment) containing detached cotton and cowpea leaves in water-filled vials (diameter 2 cm, height 9 cm). Detached leaves were used because they have previously given comparable results to intact plants in preference experiments (Thöming et al., 2013). Females were left in the cages and were able to oviposit on the leaves for 3 days. Cages were checked for eggs on a daily basis and eggs oviposited on the plants were removed and weighed.
Rearing Procedure for Larval Behaviour and Performance Assays
Eggs produced by the parental generation (F0) reared on artificial diet were collected and placed in plastic boxes (width 24 cm, height 7 cm, depth 18 cm) until hatching. Hatched larvae were randomly divided into two groups, one of which was fed on detached maize leaves and the other on detached cotton leaves. The plants were chosen partly because third and fourth instar larvae have different innate preferences for them, and partly because they differ in suitability as hosts, with cotton being considered a good host and maize a poor host (Anderson et al. unpublished data). Larvae were reared in groups of 60 individuals. Males and females were separated at the pupal stage, then after emergence males and females were transferred to a mating cage where mating occurred in the presence of the larval host plant. Eggs deposited on the plants were then removed and left to hatch in plastic boxes with no plant material.
Larval Host-Plant Choice Assay
Naïve first instar larvae (F1), from parents reared on either cotton (N = 318) or maize (N = 326), were placed in Petri dishes (diameter 8.5 cm, height 1.5 cm), each containing a maize leaf disc and a cotton leaf disc (both 0.5 cm diameter). Leaf discs were placed 5 cm apart in diametric opposition. To avoid positional effects, alternate replicates were rotated at 180° with respect to the others, so the leaf discs had different orientations. Since S. littoralis is known to use olfactory cues for host-plant identification (Salloum et al., 2011), the first choice was noted when a larva had oriented towards one of the leaf discs and touched it. Twenty-four batches of thirty randomly selected larvae were used in the tests, to ensure that the behaviour could be successfully observed. Larvae that did not make a choice within 4 h were excluded from further analysis.
Migration Assay
Naïve first instar larvae (F1) from parents reared on either cotton or maize were put in boxes (length 24 cm, width 18 cm, height 7 cm) containing cotton and maize leaves placed in water-filled vials (diameter 2 cm, height 9 cm). The larvae were put on either the plant that their parent was reared on or the other one. This resulted in four possible combinations, designated cotton × cotton, maize × maize, maize × cotton and cotton × maize, where the first and second plants are those that the parents and offspring were reared on, respectively. There were 20 replicates of each combination except cotton-maize (19) and 20 larvae in each replicate. To avoid positional effects, alternate replicates were rotated at 180° with respect to the others, so the leaves had different orientations. After 72 h, the number of larvae present on each plant was counted. Larvae that were not present on any of the plants were excluded from the experiment.
Performance Assay
Individual first instar larvae (F1), from parents fed on cotton or maize, were transferred to individual plastic cups (30 ml) containing either cotton or maize, creating four possible parent-offspring combinations (maize × maize, maize × cotton, cotton × maize, cotton × cotton: N = 80 in each case). Food was provided ad libitum during their entire development, and their mortality, larval development time (period from hatching to pupation) and pupal weight were recorded. The larvae were checked daily during the later larval instars to see if pupation had occurred, and pupae were weighed 24 h after pupation.
Statistics
A preference index, based on the total egg weight oviposited on the two plants by each female in the adult oviposition preference assay, was defined as follows:
The index ranges from 1 (absolute preference for cotton) to −1 (absolute preference for cowpea), with 0 indicating no preference. Larval migration was calculated as the percentage of larvae that migrated from one plant to the other:
This variable ranges from 0 (no migration) to 1 (migration of all larvae).
The Wilcoxon signed rank test was used to test the significance of between-treatment differences in non-parametric datasets, such as the female oviposition preference, larval migration and development time values (which did not satisfy the normal distribution null hypothesis of the Shapiro-Wilk test, at P = 0.009, P < 0.001 and P = 0.003, respectively). Post hoc pairwise comparisons were conducted using Dunn’s test as implemented in the R package dunn. test (Dinno, 2017), with Bonferroni correction for the larval migration and development time. In the female oviposition bioassay, the response of the first generation (F0) was compared to the response of every other generation (F1–F4). To compensate for mass-significance, the P-values were multiplied by the number of relevant comparisons. A chi-square test was performed to assess the significance of preference differences in the larval host plant choice bioassay.
As pupal weight data obtained in the performance bioassay were not normally distributed (according to the Shapiro-Wilk test; P < 0.001) they were subjected to square-root transformation, then fitted using a linear model. Differences in pupal weight were analysed using ANOVA, with post hoc (Tukey’s HSD) pairwise comparisons implemented using the glht function in the multicomp R package (Hothorn et al., 2008).
A chi-square test was applied to assess the significance of between-treatment differences in mortality rates in the performance bioassay. In all tests, P < 0.05 were considered statistically significant. All statistical analyses were performed using R statistical software version 3.6.1 (R Core Team, 2019), and figures were created with the software packages ggplot2 version 3.2.1 (Wickham, 2016) and ggpubr version 0.3.0 (Kassambara, 2020).
Results
Adult Oviposition Preference
We detected significant between-treatment differences in adults’ host plant oviposition preferences (Wilcoxon signed rank test, Z = −14.456, P < 0.001; Figure 1). These included differences between the generation (F0) reared on the artificial diet and subsequent generations (F1–F4) reared on cotton (N = 25 in each case, Dunn’s test: Z = −2.884, P = 0.019; Z = −3.749, P < 0.001; Z = −2.950, P = 0.016; and Z = −3.470, P = 0.003). In contrast, no difference in oviposition preference was found between the F0 and F4 generations reared on artificial diet (N = 25; Z = 1.240, P = 1).
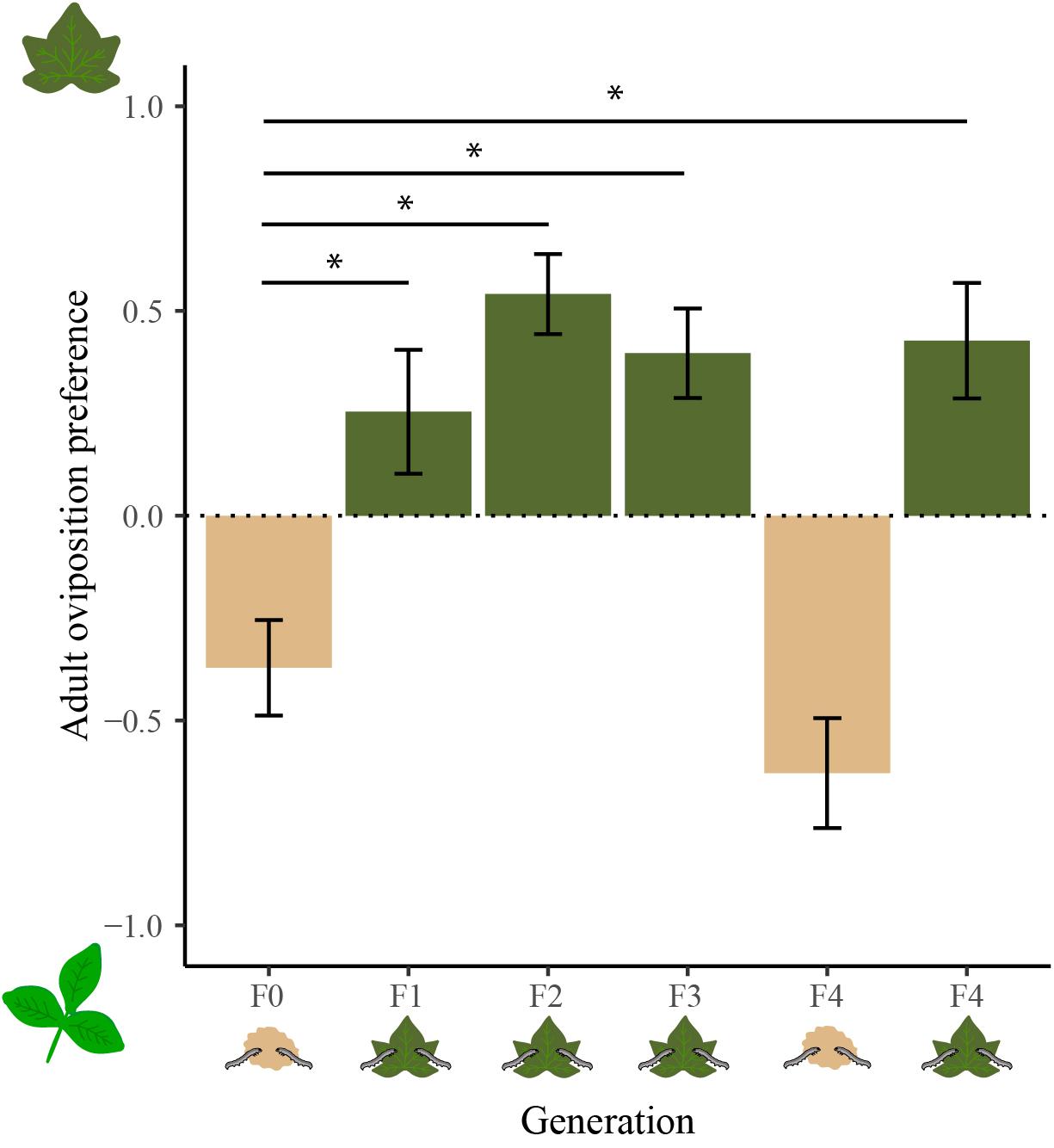
Figure 1. Effects of larval diet experience on oviposition preference (mean ± SE of the preference index) of the five generations (F0–F4) reared on either the artificial diet (beige bars) or cotton (dark green bars) when given a choice of cotton (> 0) or cowpea (< 0) plants. Asterisks indicate significant difference between treatments (Dunn’s test, P < 0.05).
Larval Host Plant Choice
Parental host plant experience had no significant effect on offspring host plant choice [χ2(1) = 0.585, P = 0.444; Figure 2]. Offspring from parents reared on cotton (N = 318) choose maize 41% and cotton 59% of the time while offspring from parents reared on maize (N = 326) choose maize 44% and cotton 56% of the time.
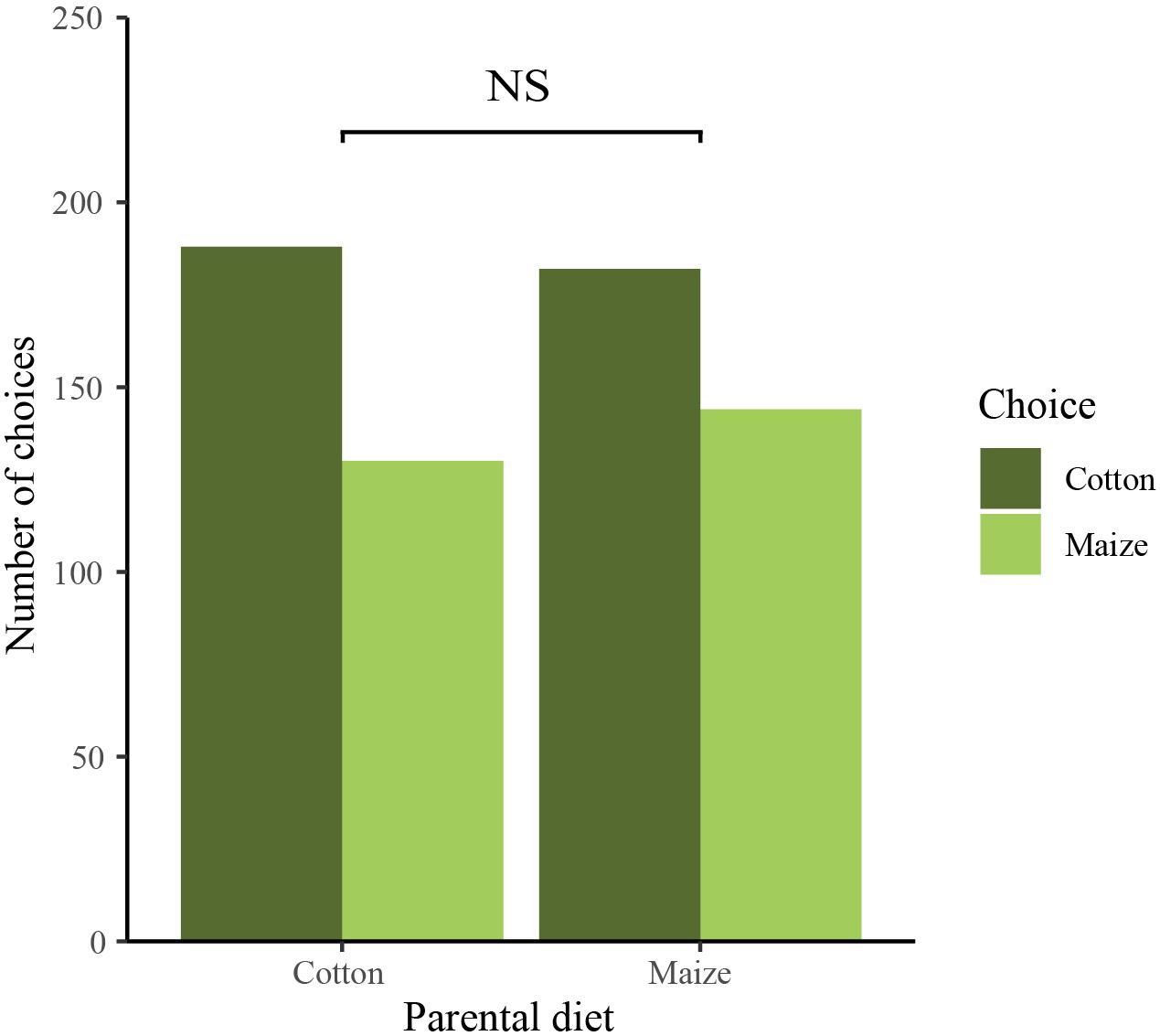
Figure 2. Feeding preferences of first instar larvae: number of offspring that chose maize (light green bar) and cotton (dark green bar) of parents reared on cotton or maize. No significant effect of parental host plant experience was detected (χ2 > 0.05).
Larval Migration
We detected between-treatment differences in migratory behaviour of first instar larvae (Wilcoxon rank sum test, Z = −6.782, P < 0.001; Figure 3). Larvae placed on maize migrated more frequently [on average 73% migration in the maize × maize treatment (N = 20) and 59% migration in the cotton × maize treatment (N = 20)] than those placed on cotton [on average 4.5% migration in the maize × cotton treatment (N = 20) and 5% migration in the cotton × cotton treatment (N = 20)]. This applied to offspring of parents reared on both maize (maize × cotton versus maize × maize, Dunn’s test, Z = −5.783, P < 0.001) and cotton (cotton × cotton versus cotton × maize, Dunn’s test, Z = −4.896, P < 0.001). However, the parental diet had no effect on the migratory behaviour of offspring larvae (cotton × cotton versus maize × cotton, Dunn’s test, Z = 0.069, P = 1; maize × maize versus cotton × maize, Dunn’s test, Z = −0.744, P = 1).
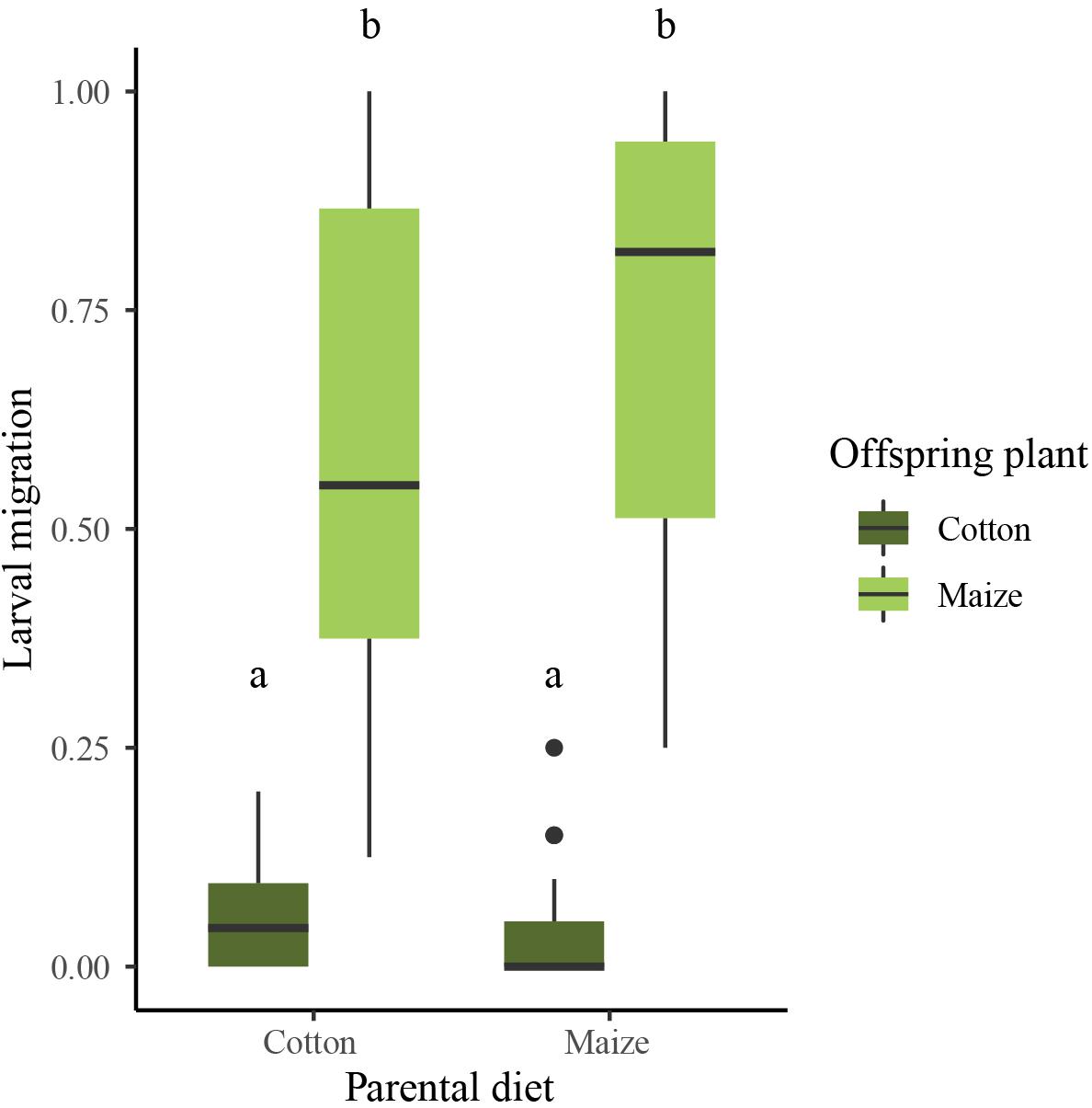
Figure 3. Indices of migration from maize (light green boxes) and cotton (dark green boxes), ranging from 0 (no migration) to 1 (migration of all the larvae) of first instar larvae with matching and mismatching parental diets. Boxplots show means (black lines) and 25–75% percentiles. Whiskers show data ranges, excluding outliers (black circles, defined as values more than one box length from the upper and lower edges of the corresponding boxes). Different letters indicate significant between-treatment differences (Dunn’s test, P < 0.05).
Development Time
Development time from first larval instar to pupation differed between the treatments (Wilcoxon rank sum test, Z = −21.6, P < 0.001; Figure 4). Offspring on cotton developed faster than offspring on maize independently of parental diet [maize × cotton (N = 79) versus maize × maize (N = 78), Dunn’s test, Z = −7.154, P < 0.001; cotton × cotton (N = 77) versus cotton × maize (N = 76), Dunn’s test, Z = −9.678, P < 0.001]. The parental diet did not affect the development time when offspring were reared on cotton (cotton × cotton versus maize × cotton, Dunn’s test, Z = −1.884, P = 0.179) or maize (maize × maize, cotton × maize, Dunn’s test, Z = 0.752, P = 1).
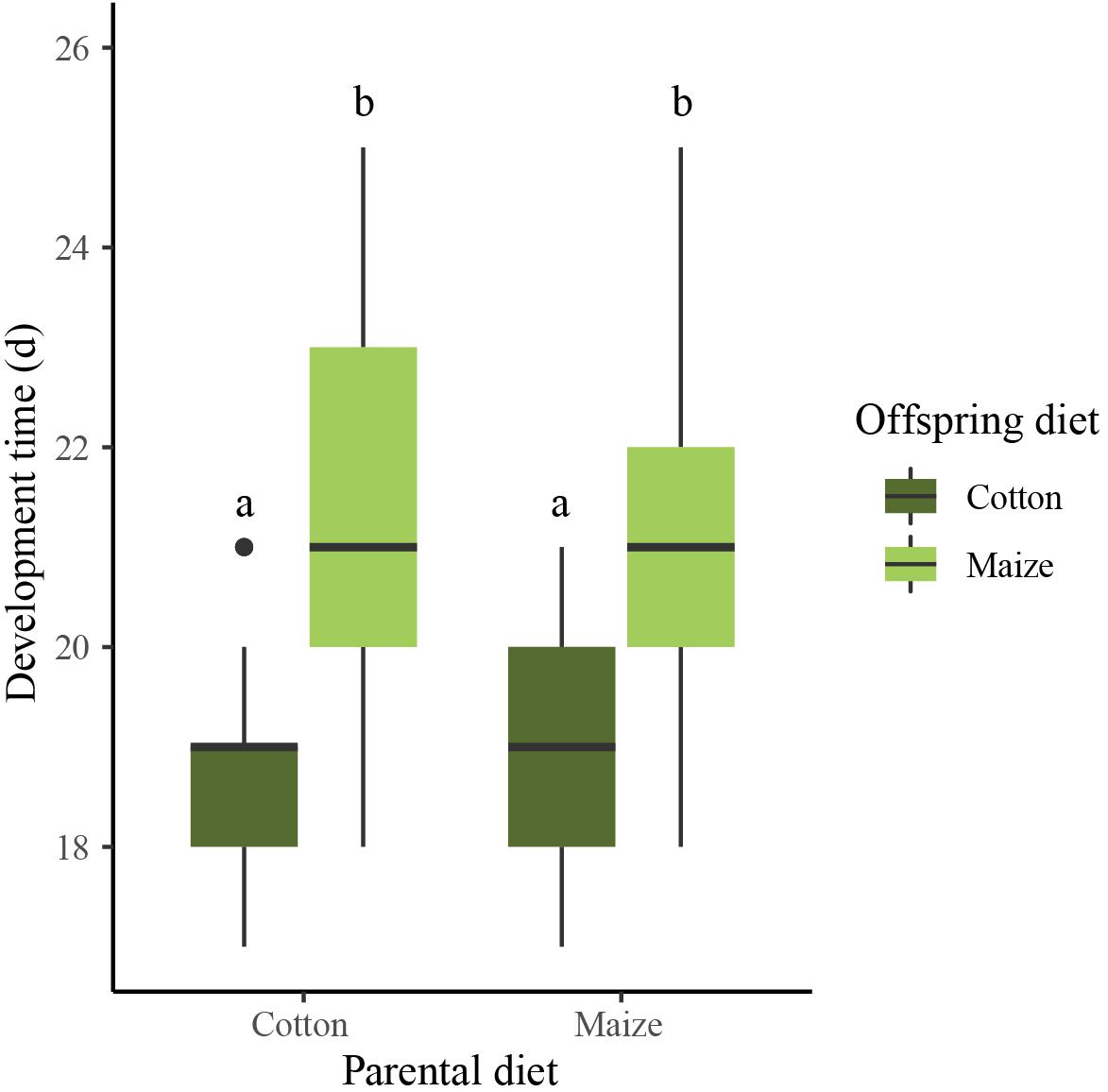
Figure 4. Development time from first instar to pupation of larvae on maize (light green boxes) and cotton (dark green boxes), of parents reared on either a matching or mismatching diet. Boxplots show means (black lines) and 25–75% percentiles. Whiskers show data ranges, excluding outliers (black circles, defined as values more than one box length from the upper and lower edges of the corresponding boxes). Different letters indicate significant between-treatment differences (Dunn’s test, P < 0.05).
Pupal Weight
Results of the larval performance bioassay showed that pupal weight differed between the treatments (LM, F = 389, df = 3/306, P < 0.001; Figure 5). Larvae reared on cotton had a higher pupal weight than larvae reared on maize, independently of parental diet (maize × cotton versus maize × maize, t = −20.925, P < 0.001; cotton × cotton versus cotton × maize, t = −26.889, P < 0.001). Offspring reared on cotton with a matching parental diet had a higher pupal weight than offspring reared on cotton with mismatching parental diet (cotton × cotton versus maize × cotton, t = −4.919, P < 0.001). In contrast, no effect of parental diet on pupal weight was detected in offspring reared on maize (cotton × maize versus maize × maize, t = 1.365, P = 0.523).
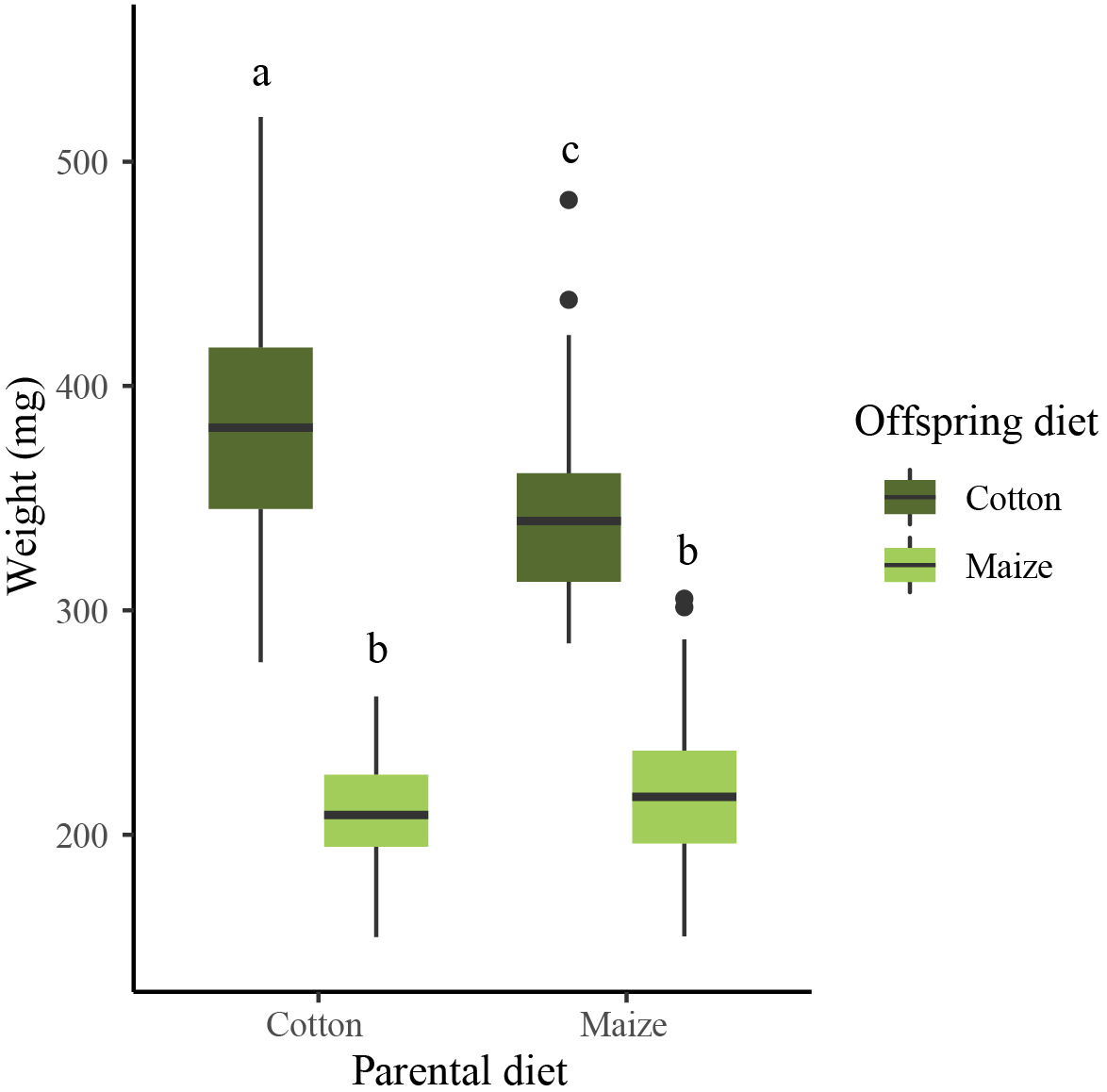
Figure 5. Pupal weight of larvae reared on maize (light green boxes) and cotton (dark green boxes) of parents reared on either a matching or mismatching diet. Boxplots show means (black lines) and 25–75% percentiles. Whiskers show data ranges, excluding outliers (black circles, defined as values more than one box length from the upper and lower edges of the corresponding boxes). Different letters indicate significant between-treatment differences (LM, Tukey’s HSD test, P < 0.05).
Mortality
No difference in mortality rate was detected between the treatments: χ2(3) = 2.06, P = 0.560. Offspring reared on cotton with matching (cotton × cotton) and mismatching (maize × cotton) parental diets had mortality rates of 3.75 and 1.25%, respectively. Offspring reared on maize with matching (maize × maize) and mismatching (cotton × maize) parental diets had mortality rates of 2.5 and 5%, respectively.
Discussion
In this study we found transgenerational effects of parental diet on larval development, but not the behaviour of progeny larvae or adults of S. littoralis. Larvae reared on cotton from parents reared on the same diet reached a higher pupal weight than larvae from parents reared on maize, but there was no difference in their development time. The difference observed between offspring reared on cotton with matching and mismatching parental diets could be explained by anticipatory transgenerational effects affecting offspring weight, e.g., through epigenetic modulations (Glastad et al., 2019) or vertically transferred symbionts (Paniagua Voirol et al., 2018). Increases in the fitness of offspring relative to parental fitness under matching conditions has been predicted in theoretical studies (Uller et al., 2013; Engqvist and Reinhold, 2016) and detected in both plants (Herman and Sultan, 2011) and vertebrates (Salinas and Munch, 2012). Anticipatory transgenerational effects have also been observed in invertebrates, including findings that offspring of the lepidopterans Pieris rapae and Coenonympha pamphilus developed best on food with the same nitrogen content as food that the parental generation had received (Rotem et al., 2003; Cahenzli and Erhardt, 2013). However, in our study a positive effect on offspring development was only found when offspring and parent diet was matched on the good host plant cotton, as no increase on offspring larval weight was found for offspring reared on maize with parents on a matching diet compared to the mismatching diet. When reared on a suboptimal host plant, the stressful environment limits the resources available for the parental generation and could reduce means of cue transfer through TGP to subsequent generations, thereby limiting the adaptive adjustment of the offspring (Uller et al., 2013). Effects of host plant quality have been observed in maritime pine, as Vivas et al. (2013) found that offspring of parents reared in benign conditions had higher pathogen resistance and growth rates than individuals grown in less favourable conditions. Furthermore, we have in S. littoralis found that on high quality food, larval olfactory experience is transferred to the adult through WGP and affect host plant choice while this does not occur on low quality food (Lhomme et al., 2018). Another possible explanation to the results could be silver spoon effects that allow parents from benign environments to give their offspring a heads start in life through transmission of abundant resources that would increase fitness independent of the environment of the offspring (Bonduriansky et al., 2012; Uller et al., 2013; Engqvist and Reinhold, 2016). In our study, we would expect that a silver spoon effect should increase the weight of offspring from parents reared on cotton irrespective of the larval diet, but we only found this effect on progeny that were fed cotton and not on those fed maize. Negative carry-over effects could potentially also be involved, we would then expect detrimental effects on the development of larvae with parents fed on maize. However, we detected no difference in pupal weight of offspring reared on maize related to the parental diet. Thus, we found no clear evidence for either silver spoon or carry-over effects.
The oviposition experiments revealed no transgenerational effects, as cotton versus cowpea preferences did not differ between females reared on the artificial diet after three generations on cotton and females of the first generation with no experience of plants during the larval stage. If transgenerational effects had influenced the oviposition preference, the females transferred to the artificial diet after three generations on cotton should have had a stronger preference for cotton than the first generation reared on the artificial diet. The oviposition results corroborate findings from our earlier studies that host plant selection of S. littoralis relies on an innate preference hierarchy between host plants that is modified through WGP, where larval feeding experience induces a preference for the experienced plant (Thöming et al., 2013).
The migration assay clearly showed that there was a difference in the behaviour of the larvae on the two host plants. First instar larvae that were placed on maize migrated towards cotton at a much higher extent compared to the number of larvae migrating from cotton to maize. This is most likely due to that cotton is a more suitable host plant for larval development than maize (Anderson et al. unpublished data). The lower food quality of maize could induce larval movement and increase their search for an alternative host plant. A difference in migration behaviour has also been shown for larger larvae of S. littoralis, where more larvae were found to leave damaged cotton plants with induced defence than undamaged plants (Anderson et al., 2011). However, although S. littoralis larvae may actively migrate from less suitable plants, and WGP can strongly influence the species’ feeding preference (Carlsson et al., 1999; Salloum et al., 2011), we detected no effect of parental diet on migration behaviour of first instar larvae, or larval host-plant choice.
Recent genetic and mathematical models of WGP, TGP and their possible coexistence predict that the two types of plasticity can operate either separately or additively, depending on the environmental conditions (Lande, 2009; Ezard et al., 2014; Leimar and McNamara, 2015; Dury and Wade, 2019). Empirical support for this has been found in both plants and animals (Agrawal et al., 1999; Sultan et al., 2009; Mikulski and Pijanowska, 2010; Walsh et al., 2015, 2016; Katoh et al., 2018). The experiments reported here provided no evidence of TGP affecting the behaviour of S. littoralis, supporting the hypothesis that the two types of plasticity operate separately for a specific trait, as postulated by Walsh et al. (2015, 2016). Theoretical models of WGP, TGP and their possible co-occurrence indicate that WGP is the dominating type of plasticity (Kuijper and Hoyle, 2015; Leimar and McNamara, 2015). However, the developmental data obtained in this study show that transgenerational effects may be present in S. littoralis when environmental conditions are favourable, and there may be interactions between WGP and TGP under these conditions. Thus, WGP and TGP could potentially influence specific traits connected to host plant utilisation either separately or additively.
WGP may influence behavioural choices of S. littoralis more strongly than TGP because the associated cues are temporally closer to them than parental cues, and more accurate predictors of current availabilities and qualities of host plants (Kuijper and Hoyle, 2015). We have previously identified a sensitive period in the late larval instar of S. littoralis in which larval host plant experience modifies subsequent adult behavioural decisions, while early larval experience is not retained (Lhomme et al., in press). Thus, the information is gathered close to the adult stage, and the decision mechanism takes into account factors that affect larval development. Such WGP increases the salience of the previously experienced plant, reduces risks of mismatching conditions in the ovipositing female’s environment, and could make the transfer of parental experience through TGP redundant (Donelson et al., 2018). Furthermore, the host plant choice is under maternal control and she will likely lay her eggs on the same plant (through WGP), thereby reducing selective pressures favouring the evolution of TGP-mediated effects on the behaviour of offspring larvae. However, under such conditions, a transgenerational transfer of cues that increases the ability of the progeny to develop on that specific plant species could be very valuable and promote TGP of such traits.
In conclusion, this study suggests that TGP may modify the physiological state of S. littoralis offspring in a manner that enhances their performance on the parental host plant. However, such enhancement may only occur when conditions are favourable. The results also indicate that host plant-mediated behaviours of both adult females and first instar larvae are strongly influenced by innate preferences and WGP, but not by the parental diet. However, mechanisms underlying the higher pupal weight of offspring reared on cotton with a matching parental diet are still unknown, and further studies on anticipatory TGP, WGP and their possible co-occurrence in S. littoralis focused on these mechanisms in both favourable and unfavourable conditions are warranted.
Data Availability Statement
All datasets generated for this study are included in the article/Supplementary Material.
Author Contributions
AR, PA, PL, and MK designed the study. AR, PL, and MK collected and analysed the data. AR wrote the manuscript together with PL and PA. PA obtained the funding. All authors contributed to revisions.
Funding
Funding was provided by the Swedish Research Council for Environment, Agricultural Sciences and Spatial Planning (Formas) (Linnaeus grant “Insect Chemical Ecology, Ethology and Evolution” 217-2006-1750) and the Swedish Research Council (VR) (2018-03560-3).
Conflict of Interest
The authors declare that the research was conducted in the absence of any commercial or financial relationships that could be construed as a potential conflict of interest.
Acknowledgments
We thank Elisabeth Marling for her technical assistance in rearing the moths; Adam Flöhr and Jan-Erik Englund for comments on the data analysis; Kristina Green, Audrey Bras, and Björn Eriksson in the Chemical Ecology Agriculture group for their valuable comments on the manuscript; and Gunda Thöming for discussions on concepts of the study.
Supplementary Material
The Supplementary Material for this article can be found online at: https://www.frontiersin.org/articles/10.3389/fevo.2020.00254/full#supplementary-material
References
Agrawal, A. A. (2001a). Phenotypic plasticity in the interactions and evolution of species. Science 294, 321–326. doi: 10.1126/science.1060701
Agrawal, A. A. (2001b). Transgenerational consequences of plant responses to herbivory: an adaptive maternal effect? Am. Natural. 157, 555–569. doi: 10.1086/319932
Agrawal, A. A., Laforsch, C., and Tollrian, R. (1999). Transgenerational induction of defences in animals and plants. Nature 401, 60–63. doi: 10.1038/43425
Anderson, P., and Anton, S. (2014). Experience-based modulation of behavioural responses to plant volatiles and other sensory cues in insect herbivores. Plant Cell Environ. 37, 1826–1835. doi: 10.1111/pce.12342
Anderson, P., Sadek, M. M., Larsson, M., Hansson, B. S., and Thöming, G. (2013). Larval host plant experience modulates both mate finding and oviposition choice in a moth. Anim. Behav. 85 1169–1175. doi: 10.1016/j.anbehav.2013.03.002
Anderson, P., Sadek, M. M., and Wackers, F. L. (2011). Root herbivory affects oviposition and feeding behavior of a foliar herbivore. Behav. Ecol. 22, 1272–1277. doi: 10.1093/beheco/arr124
Bonduriansky, R., Crean, A. J., and Day, T. (2012). The implications of nongenetic inheritance for evolution in changing environments. Evol. Appl. 5, 192–201. doi: 10.1111/j.1752-4571.2011.00213.x
Cahenzli, F., and Erhardt, A. (2013). Transgenerational acclimatization in an herbivore-host plant relationship. Proc. R. Soc. Lond. B Biol. Sci. 280:2856. doi: 10.1098/rspb.2012.2856
Cahenzli, F., Wenk, B. A., and Erhardt, A. (2015). Female butterflies adapt and allocate their progeny to the host-plant quality of their own larval experience. Ecology 96, 1966–1973. doi: 10.1890/14-1275.1
Carlsson, M. A., Anderson, P., Hartlieb, E., and Hansson, B. S. (1999). Experience-dependent modification of orientational response to olfactory cues in larvae of Spodoptera littoralis. J. Chem. Ecol. 25, 2445–2454. doi: 10.1023/A:1020865922827
Dias, B. G., and Ressler, K. J. (2014). Parental olfactory experience influences behavior and neural structure in subsequent generations. Nat. Neurosci. 17, 89–96. doi: 10.1038/nn.3594
Dinno, A. (2017). Dunn.test: Dunn’s Test of Multiple Comparisons Using Rank Sums. Available online at: https://CRAN.R-project.org/package=dunn.test (accessed June 1, 2020).
Donelson, J. M., Salinas, S., Munday, P. L., and Shama, L. N. (2018). Transgenerational plasticity and climate change experiments: where do we go from here? Glob. Chang. Biol. 24, 13–34. doi: 10.1111/gcb.13903
Dury, G. J., and Wade, M. J. (2019). When mother knows best: a population genetic model of transgenerational versus intragenerational plasticity. J. Evol. Biol. 33, 127–137. doi: 10.1111/jeb.13545
Engqvist, L., and Reinhold, K. (2016). Adaptive trans-generational phenotypic plasticity and the lack of an experimental control in reciprocal match/mismatch experiments. Methods Ecol. Evol. 7, 1482–1488. doi: 10.1111/2041-210x.12618
Ezard, T. H. G., Prizak, R., Hoyle, R. B., and Marshall, D. (2014). The fitness costs of adaptation via phenotypic plasticity and maternal effects. Funct. Ecol. 28, 693–701. doi: 10.1111/1365-2435.12207
Glastad, K. M., Hunt, B. G., and Goodisman, M. A. D. (2019). Epigenetics in insects: genome regulation and the generation of phenotypic diversity. Annu. Rev. Entomol. 64, 185–203. doi: 10.1146/annurev-ento-011118-111914
Gowri, V., Dion, E., Viswanath, A., Piel, F. M., and Monteiro, A. (2019). Transgenerational inheritance of learned preferences for novel host plant odors in Bicyclus anynana butterflies. Evolution 73, 2401–2414. doi: 10.1111/evo.13861
Herman, J. J., and Sultan, S. E. (2011). Adaptive transgenerational plasticity in plants: case studies, mechanisms, and implications for natural populations. Front. Plant Sci. 2:102. doi: 10.3389/fpls.2011.00102
Hinks, C., and Byers, J. (1976). Biosystematics of the genus Euxoa (Lepidoptera: Noctuidae): V. rearing procedures, and life cycles of 36 species. Can. Entomol. 108, 1345–1357. doi: 10.4039/Ent1081345-12
Hothorn, T., Bretz, F., and Westfall, P. (2008). Simultaneous inference in general parametric models. Biometr. J. 50, 346–363. doi: 10.1002/bimj.200810425
Kassambara, A. (2020). ggpubr: ‘ggplot2’ Based Publication Ready Plots. Available online at: https://rpkgs.datanovia.com/ggpubr/ (accessed June 1, 2020).
Katoh, M., Tatsuta, H., and Tsuji, K. (2018). Ultraviolet exposure has an epigenetic effect on a Batesian mimetic trait in the butterfly Papilio polytes. Sci. Rep. 8:31738. doi: 10.1038/s41598-018-31732-31738
Kuijper, B., and Hoyle, R. B. (2015). When to rely on maternal effects and when on phenotypic plasticity? Evolution 69, 950–968. doi: 10.1111/evo.12635
Lande, R. (2009). Adaptation to an extraordinary environment by evolution of phenotypic plasticity and genetic assimilation. J. Evolut. Biol. 22, 1435–1446. doi: 10.1111/j.1420-9101.2009.01754.x
Leimar, O., and McNamara, J. M. (2015). The evolution of transgenerational integration of information in heterogeneous environments. Am. Natural. 185, E55–E69. doi: 10.1086/679575
Lhomme, P., Carrasco, D., Larsson, M., Hansson, B., and Anderson, P. (2018). A context-dependent induction of natal habitat preference in a generalist herbivorous insect. Behav. Ecol. 29, 360–367. doi: 10.1093/beheco/arx173
Lhomme, P., Khallaf, M., Larsson, M., and Anderson, P. (in press). Sensitive period for the induction of host plant preference in a generalist herbivorous insect. Anim. Behav.
Menzel, R., and Müller, U. (1996). Learning and memory in honeybees: from behavior to neural substrates. Annu. Rev. Neurosci. 19, 379–404. doi: 10.1146/annurev.ne.19.030196.002115
Mikulski, A., and Pijanowska, J. (2010). When and how can Daphnia prepare their offspring for the threat of predation? Hydrobiologia 643, 21–26. doi: 10.1007/s10750-010-0131-130
Mousseau, T. A., and Fox, C. W. (1998). Maternal Effects AS Adaptations. New York, NY: Oxford University Press.
Paniagua Voirol, L. R., Frago, E., Kaltenpoth, M., Hilker, M., and Fatouros, N. E. (2018). Bacterial symbionts in lepidoptera: their diversity, transmission, and impact on the host. Front. Microbiol. 9:556. doi: 10.3389/fmicb.2018.00556
Peralta Quesada, P. C., and Schausberger, P. (2012). Prenatal chemosensory learning by the predatory mite Neoseiulus californicus. PLoS One 7:e53229. doi: 10.1371/journal.pone.0053229
Pogue, M. G. (2002). A world revision of the genus Spodoptera Guenée:(Lepidoptera: Noctuidae). Memoirs Am. Entomol. Soc. 43, 1–202.
Proffit, M., Khallaf, M. A., Carrasco, D., Larsson, M. C., and Anderson, P. (2015). ‘Do you remember the first time?’ Host plant preference in a moth is modulated by experiences during larval feeding and adult mating. Ecol. Lett. 18, 365–374. doi: 10.1111/ele.12419
R Core Team (2019). R: A Language and Environment for Statistical Computing. Vienna: R Foundation for Statistical Computing.
Rotem, K., Agrawal, A. A., and Kott, L. (2003). Parental effects in Pieris rapae in response to variation in food quality: adaptive plasticity across generations? Ecol. Entomol. 28, 211–218. doi: 10.1046/j.1365-2311.2003.00507.x
Salinas, S., and Munch, S. B. (2012). Thermal legacies: transgenerational effects of temperature on growth in a vertebrate. Ecol. Lett. 15, 159–163. doi: 10.1111/j.1461-0248.2011.01721.x
Salloum, A., Colson, V., and Marion-Poll, F. (2011). Appetitive and Aversive Learning in Spodoptera littoralis Larvae. Chem. Senses 36, 725–731. doi: 10.1093/chemse/bjr041
Sultan, S. E., Barton, K., and Wilczek, A. M. (2009). Contrasting patterns of transgenerational plasticity in ecologically distinct congeners. Ecology 90, 1831–1839. doi: 10.1890/08-1064.1
Thöming, G., Larsson, M. C., Hansson, B. S., and Anderson, P. (2013). Comparison of plant preference hierarchies of male and female moths and the impact of larval rearing hosts. Ecology 94, 1744–1752. doi: 10.1890/12-0907.1
Turlings, T. C. L., Wäckers, F. L., Vet, L. E. M., Lewis, W. J., and Tumlinson, J. H. (1993). “Learning of host-finding cues by hymenopterous parasitoids,” in Insect Learning: Ecological and Evolutionary Perspectives, eds D. R. Papaj and A. C. Lewis (Boston, MA: Springer), 51–78. doi: 10.1007/978-1-4615-2814-2_3
Uller, T. (2008). Developmental plasticity and the evolution of parental effects. Trends Ecol. Evol. 23, 432–438. doi: 10.1016/j.tree.2008.04.005
Uller, T., Nakagawa, S., and English, S. (2013). Weak evidence for anticipatory parental effects in plants and animals. J. Evolut. Biol. 26, 2161–2170. doi: 10.1111/jeb.12212
Vivas, M., Zas, R., Sampedro, L., and Solla, A. (2013). Environmental maternal effects mediate the resistance of maritime pine to biotic stress. PLoS One 8:e70148. doi: 10.1371/journal.pone.0070148
Walsh, M. R., Castoe, T., Holmes, J., Packer, M., Biles, K., Walsh, M., et al. (2016). Local adaptation in transgenerational responses to predators. Proc. R. Soc. B Biol. Sci. 283:2271. doi: 10.1098/rspb.2015.2271
Walsh, M. R., Cooley, F., Biles, K., and Munch, S. B. (2015). Predator-induced phenotypic plasticity within-and across-generations: a challenge for theory? Proc. R. Soc. B Biol. Sci. 282:2205. doi: 10.1098/rspb.2014.2205
West-Eberhard, M. J. (1989). Phenotypic plasticity and the origins of diversity. Annu. Rev. Ecol. Syst. 20, 249–278. doi: 10.1146/annurev.es.20.110189.001341
Whitman, D. W., and Agrawal, A. A. (2009). “What is phenotypic plasticity and why is it important,” in Phenotypic Plasticity Of Insects: Mechanisms And Consequences, 1st Edn, ed. D. Whitman (Boca Raton: CRC Press), 1–63.
Woestmann, L., and Saastamoinen, M. (2016). The importance of trans-generational effects in Lepidoptera. Curr. Zool. 62, 489–499. doi: 10.1093/cz/zow029
Keywords: within-generation phenotypic plasticity, transgenerational phenotypic plasticity, anticipatory plasticity, larval performance, insect behaviour, Lepidoptera, Spodoptera littoralis
Citation: Rösvik A, Lhomme P, Khallaf MA and Anderson P (2020) Plant-Induced Transgenerational Plasticity Affecting Performance but Not Preference in a Polyphagous Moth. Front. Ecol. Evol. 8:254. doi: 10.3389/fevo.2020.00254
Received: 14 February 2020; Accepted: 13 July 2020;
Published: 31 July 2020.
Edited by:
Olivia Roth, GEOMAR Helmholtz Center for Ocean Research Kiel, GermanyReviewed by:
Jens Joschinski, Ghent University, BelgiumRalf Friedrich Schneider, GEOMAR Helmholtz Center for Ocean Research Kiel, Germany
Copyright © 2020 Rösvik, Lhomme, Khallaf and Anderson. This is an open-access article distributed under the terms of the Creative Commons Attribution License (CC BY). The use, distribution or reproduction in other forums is permitted, provided the original author(s) and the copyright owner(s) are credited and that the original publication in this journal is cited, in accordance with accepted academic practice. No use, distribution or reproduction is permitted which does not comply with these terms.
*Correspondence: Axel Rösvik, YXhlbC5yb3N2aWtAc2x1LnNl
†These authors have contributed equally to this work
‡ORCID: Axel Rösvik, orcid.org/0000-0003-4481-1828; Patrick Lhomme, orcid.org/0000-0001-6735-9104; Mohammed A. Khallaf, orcid.org/0000-0002-1402-3858; Peter Anderson, orcid.org/0000-0003-4105-8236