- 1Department of Biological Sciences, School of Life Sciences and the Environment, Royal Holloway University of London, Egham, United Kingdom
- 2Institut für Biologie, Freie Universität Berlin, Berlin, Germany
- 3Department for Materials and the Environment, BAM Federal Institute for Materials Research and Testing, Berlin, Germany
Social insect colonies can express adaptive, organism-like design. In some cases, colonies so resemble a unique, cohesive and integrated “individual” that they are termed superorganisms. The major evolutionary transitions framework explains, via inclusive fitness theory, how new levels of biological individuality, including genes into genomes within cells, cells into multicellular organisms and organisms into superorganisms can emerge. Importantly, it highlights how at each major transition similar challenges arose and why seemingly convergent solutions evolved. One challenge faced at each transition is exploitation, caused internally by social cheaters and externally by parasites and pathogens. To overcome the problem of exploitation transitions in biological individuality required novel immune systems to maintain the integrity of newly emerged individuals. Multicellular organisms evolved an immune system while social insect colonies evolved a social immune system. In this review, we take a major transitions perspective of immunity to highlight the interdependency between the evolution of immune systems and the emergence of biological individuality. We build on the notion that superorganisms have evolved an immune system to promote the fitness of the colony. We draw parallels between the evolution of the metazoan immune system and the social immune system, and their expression as cognitive networks. Moreover, we discuss how research on other group-living species, such as family based cooperative breeders, can inform our understanding of how social immune systems evolve. We conclude that superorganism immunity is an adaptive suite of organismal traits that evolves to maximize the fitness of advanced social insect colonies, fulfilling the same function as the immune system of Metazoa.
Introduction
“Fixing attention on the honeybee…nothing like the immune system for detecting and combatting microbial enemies is known to exist.”
“The most general organismal character of the ant-colony is its individuality. Like the cell or the person, it behaves as a unitary whole, maintaining its identity in space, resisting dissolution and, as a general rule, any fusion with other colonies of the same or alien species.”
Major evolutionary transitions are a series of defining moments in the history of life on earth where new, more complex forms of life emerged (Smith and Szathmary, 1997; West et al., 2015). At each transition, groups of previously free-living and self-replicating individuals cooperated to such a degree that they lost their independence, coalescing into a single distinct entity that functions and replicates as one. Conflict between lower-level constituent parts is negligible, such that the new higher-level life form becomes the fitness-maximizing “biological individual” (Queller and Strassmann, 2009; West et al., 2015). The underlying process governing each of these transitions is social evolution (Bourke, 2011; West et al., 2015) and examples include: genes into genomes within cells giving rise to single-celled life; cells into organisms resulting in the complex multicellular plants and animals; and organisms into superorganisms, the evolution of social insect colonies with obligate reproductive and worker roles.
One factor that has the potential to both hinder and encourage evolutionary transitions, particularly at the organismal and superorganismal level, are disease-causing pathogens and parasites. Hamilton recognized that, due to low levels of genetic diversity within these groups, disease should be a major constraint on the evolution of multicellularity and insect sociality (Hamilton, 1987). However, as the quote at the beginning of this review reveals, Hamilton did not believe anything comparable to the metazoan immune system to protect against disease had evolved in social insects. Hamilton instead proposed outbreeding to increase genetic diversity as the main mechanism that prevents pathogens driving social insects to extinction (Hamilton, 1987). Although increased genetic diversity via polygyny and polyandry as a means to protect against disease is well supported both theoretically and empirically (Baer and Schmid-Hempel, 1999; Schmid-Hempel and Crozier, 1999; Seeley and Tarpy, 2007), it nevertheless remains true that the majority of social Hymenoptera retain a single-queen genetic bottleneck at some point in their lifecycle, and most queens still mate with fewer than two males on average (Boomsma and Ratnieks, 1996; Queller, 2000; Hughes et al., 2008a). Measures to increase genetic diversity such as polyandry and polygyny seem to be especially rare in the termites as colonies are usually founded by a single monogamous pair (Shellman-Reeve, 1997). Interestingly, although termites from outbred colonies exhibit reduced fungal susceptibility, unrelated monogamous pairs are more likely to perish during colony foundation than related pairs (Calleri et al., 2005, 2006). Genetic diversity has, therefore, clearly not evolved as a universal mechanism to reduce disease susceptibility in insect colonies.
Since Hamilton’s predictions, our understanding of how social insects avoid, tolerate and resist pathogens and parasites has expanded greatly. We now know that social insects have evolved a variety of mechanisms to prevent and/or mitigate the impact of disease. In 2007, Cremer et al. (2007) coined the term social immunity to describe the collective immune defenses present in insect colonies. Later, Cremer and Sixt (2009) took the immunity analogy further, pointing out the many ways in which social immunity in superorganisms plays a functionally equivalent role to metazoan immunity (see also Cremer et al., 2018; Cremer, 2019). In this review, our aim is to build on the original hypothesis asserted by Cremer et al. (2007) that, in superorganisms, social disease defense has evolved beyond simple cooperation to function instead as a systemic and indispensable “social immune system.” As we will see, the evolution of biological individuality has repeatedly resulted in the parallel emergence of a bespoke defense system that operates at the level of the new individual (Bourke, 2011; Pradeu, 2013). This defense system maintains the integrity of the individual, be it a cell, organism or superorganism, by providing protective functions that extend beyond disease defense. It may, therefore, be possible to talk of a true immune system that provides superorganism immunity in advanced social insect colonies (Aanen, 2018), which could be used as a criterion for defining what a biological individual is in social insects. We hope that our review will be able to contribute positively to the debate on the defining features of major evolutionary transitions (Godfrey-Smith and Goodnight, 2013; Pradeu, 2013; Boomsma and Gawne, 2018), whilst providing clear directions for future research on superorganism evolution, the most recent and arguably least understood of the major evolutionary transitions.
Superorganisms as Biological Individuals
Before we can explore the role of immunity in superorganism evolution, we first need to discuss what we mean when we talk of superorganisms, and, more broadly, organisms and individuality (Figure 1). Debate and discussion surrounding these terms abound, and there is seemingly no one answer that satisfies both philosophers and biologists (Godfrey-Smith and Goodnight, 2013). Most people have an intuitive understanding of the term organism, which is exemplified by the paradigmatic metazoan animal, yet universal classification remains difficult. An often accepted hallmark of multicellular organismality is the permanent sequestration of the germline early in development, but this criterion excludes many plants, fungi and even some animals, including sponges (Funayama, 2010), cnidarians (Nishimiya-Fujisawa and Kobayashi, 2012), Acoela (De Mulder et al., 2009), some helminths (Rink, 2013; Fields and Levin, 2018), and possibly echinoderms and annelid worms (Solana, 2013; Dannenberg and Seaver, 2018). These all appear to harbor totipotent somatic cells but are clearly organisms (Clarke and Okasha, 2013 and references therein). Such examples support the view that the germline has not been unambiguously sequestrated in either the metazoan or the urbilaterian ancestor of animals (Extavour, 2007; Clarke and Okasha, 2013; Solana, 2013; Fierro-Constaín et al., 2017). Similarly, Wheeler defined superorganisms as advanced social insects societies where worker and queen castes are permanently differentiated into “soma” and “germline” components, such that the worker can never mate nor give rise to a new superorganism (Wheeler, 1911; Boomsma and Gawne, 2018). However, cases that are difficult to classify exist: many termite species – for example, most multi-piece foraging/subterranean lower termites in the families Mastotermitidae and Rhinotermitidae (Roisin, 2000) – form complex, thousands-strong nests that are maintained, protected and supplied with resources by a “true worker” caste. True termite workers split early on in development from the winged dispersing caste that do not work (Shellman-Reeve, 1997; Korb and Thorne, 2017). Nonetheless, true workers, including those in some higher termite species (Roisin, 1990; Myles, 1999; da Silva et al., 2019), can yield reproductively competent ergatoid neotenics under certain conditions, such as the death of the primary reproductive, which seems analogous to emergency queen rearing in honeybees (Shellman-Reeve, 1997; Myles, 1999). Despite this totipotency, true workers typically behave as altruistically as the permanently differentiated workers in other social insect lineages: they perform brood care, leave the colony to forage, and engage in altruistic hygiene (Chouvenc and Su, 2012; Korb et al., 2012; Davis et al., 2018). True workers also have an extremely limited chance of independent reproduction; less than 1% are estimated to become reproductive (Shellman-Reeve, 1997; Thorne, 1997; Korb et al., 2012; Korb and Thorne, 2017). In contrast, less advanced single-piece nesting/wood-dwelling lower termites have helper offspring (“false workers”) that are fully totipotent: helpers and even pre-soldiers instars can reproduce through nest inheritance, colony fusion events, or dispersal (Shellman-Reeve, 1997; Thorne, 1997; Myles, 1999; Korb and Schmidinger, 2004; Korb, 2006, 2007; Hoffmann and Korb, 2011; Korb and Roux, 2012). Hence, termites with true workers appear as superorganismal as species with permanently differentiated castes, in that the reproductive-worker role is obligate and specialized. To avoid excluding these potential superorganisms and to aid comparisons across the major transitions, we will adopt the biological individual terminology used extensively in evolutionary biology, which defines the unit of selection as a meaningful measure of individuality. A biological individual can thus be a gene, cell, organism, or superorganism (Bourke, 2011; West et al., 2015). The important point is that natural selection acts on and between these biological individuals, so that it is the biological individual as a consolidated whole that responds most to selection, accrues adaptions to maximize its fitness, and so evolves over time (Queller, 2000; Bourke, 2011). One such adaptation that is thought to be essential for biological individuals to emerge is immunity (Pradeu, 2013). In the following section, we will see that immunity is an evolutionary widespread adaptation, which can also help to define when a major evolutionary transition has occurred.
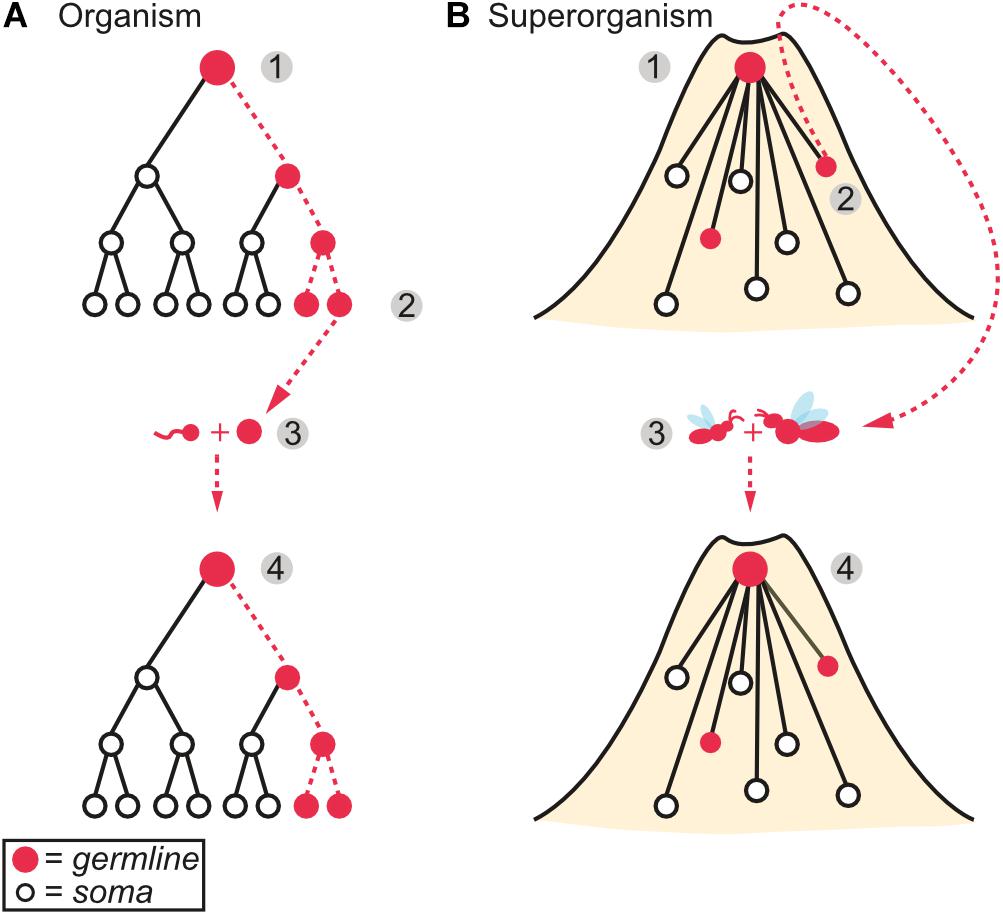
Figure 1. Analogous features between (A) multicellular organisms and (B) social insect superorganisms. (1) An organismal/colony existence is obligate with distinct germline (red dots) and soma (white dots) roles. The germline gives rise to both the sterile soma and additional germline entities. (2) The germline reproduces the (super)organism. (3) Lifetime commitment, through the fusion of gametes as a zygote in organisms and strict lifetime monogamy between mated pairs in superorganisms, creates the conditions necessary for the evolution of germline-soma differentiation, which in many, but not all, metazoan and superorganismal lineages has also become fully sequestered (see main text). (4) Superorganism fitness, akin to the fitness of an organism, is measured not by the number of new insects it creates, but by the number of new, independent superorganisms it produces.
Immunology and Individuality
The concept of biological individuality is tightly linked to immunology (Pradeu, 2012). For biological individuals to emerge, evolve and adapt, they need to: (i) suppress the independent evolution of their constituent parts, (ii) develop a clear delineation of the “self” that makes them biologically unique, and (iii) prevent exploitation by infectious diseases (Bourke, 2011; Pradeu, 2013). In the evolution of multicellularity it is the immune system that acts as “policer,” eliminating non-cooperating cells to prevent conflicts of fitness; “delineator, setting the limits and boundaries of the organism (i.e., acting as gatekeeper); and “eradicator,” preventing infections from spreading within, and causing the dissolution of, clonal aggregations (Pradeu, 2013). It is hypothesized that multicellular immunity may have first evolved to prevent selfish mutations arising that replicate at cost to the whole organism (though modeling indicates this probably only occurs under specific circumstances or as multicellular organisms grew larger; Queller, 2000) and to prevent fusion with, or invasion by, nonkin cells, before secondarily evolving the ability to fight pathogens (Pradeu, 2013).
We, like Pradeu (2013), argue that since immune systems are so important for maintaining individual integrity – and are universally present among all multicellular organisms including plants, fungi and animals – that they may even precede other more patchily distributed criteria, such as a sequestered germline (Clarke and Okasha, 2013). Immune systems should hence be considered key mechanisms that enable and maintain transitions in individuality. For example, some form of crude immunity seems to even be present in facultatively multicellular organisms, such as Dictyostelium, suggesting that immunity evolves concomitantly with the emergence of multicellularity (Chen et al., 2007). Moreover, Pradeu (2013) reasons that immunity has likely played a similarly pivotal role in other major evolutionary transitions, in particular, the emergence of unicellularity and superorganismality: in prokaryotes, Pradeu hypothesizes that their form of immunity (the CRISPR-Cas system) may have evolved to protect the biological individuality of unicellular life (Horvath and Barrangou, 2010); whilst in superorganisms, “the immune system of the colony will make it strongly cohesive in such a way that the colony will qualify as an organism.”
Wheeler, as quoted at the beginning of this review, recognized that social insect colonies possess the fundamental characteristics of a biological individual, exhibiting a tight unity and functional integration of its constituents (Figure 1). Additionally, he noted that they remain the same whilst changing through time and rarely fuse with other colonies. In Wheeler’s era, less was known about the cooperative disease defenses of social insects. Now, we are a better position to examine whether superorganismal social insect colonies have evolved an immune system that perpetuates the individuality of colonies, and, whether this “social immune system” was as instrumental in the evolution of superorganismality as immunity is thought to have been for multicellularity (Pradeu, 2013).
Superorganism Immunity
If superorganisms possess an immune system, we expect it to exhibit certain properties. Chief among these is an ability to police its constituent parts, mechanisms to maintain the superorganism’s uniqueness and an ability to detect and eradicate harmful entities; namely, parasites and pathogens (Bourke, 2011; Pradeu, 2013). Based on the best studied immune systems, those of the vertebrates, we might also predict other qualities and phenomena, such as decentralized control and immunological memory (Hofmeyr and Forrest, 1999). Moreover, we would expect information sharing and the emergence of similar network-based rules (Moses et al., 2019; Piñero and Solé, 2019). In Table 1 we summarize some of the convergent properties of multicellular and superorganismal immunity (based partly on: Hofmeyr and Forrest, 1999). Below we discuss some of these aspects in more detail. Although originally considered in early examinations of colony-level immune systems (Cremer et al., 2007; Cremer and Sixt, 2009), the role of immune policing and the immunological delineation of the individual have been largely neglected in recent discussions of social immune systems (Cremer et al., 2018), which is likely due to a research focus on microbial pathogens. Thus, in the following section, we highlight the broader protective role of superorganism immunity for superorganism integrity, expanding on earlier assessments by Cremer et al. (2007), Cremer and Sixt (2009), and Bourke (2011).
Immune Policing of the Superorganism
Biological individuals cannot emerge and evolve if there is significant selection and evolution of their lower-level constituent parts (Gardner, 2013; Pradeu, 2013; West et al., 2015). Preventing social cheaters with differential fitness is thus a reoccurring challenge across the major evolutionary transitions (Queller, 2000; Bourke, 2011; Ågren et al., 2019). Genetic bottlenecking in modern-day multicellular organisms severely limits selective opportunities for selfish mutants (e.g., cancers) beyond one generation (Queller, 2000; Michod, 2007). However, there was likely to be more opportunity for selfishness and conflict in the early stages of multicellularity, so that policing of constituent parts was important to prevent selfish elements overwhelming the germline (Michod and Roze, 2001; Michod, 2007). It is also unclear how often social parasites of somatic origin (next section) would evolve in the absence of modern-day immune policing mechanisms that prevent cancers from evolving (Ågren et al., 2019). In superorganisms, their modular structure, a degree of individual control over caste fate, and the ability of workers to lay haploid, unfertilised eggs (in hymenopteran societies) – coupled with polyandry and/or polygyny in some species – creates more potential for conflict and hence worker selfishness (Beekman and Oldroyd, 2019 and references therein). Worker reproduction is usually inhibited by the presence of a reproductive queen that emits a pheromone signaling her fertility. These conserved signals suppress the activation of worker ovaries and so act as a form of “policing” (Van Oystaeyen et al., 2014). Reproductive workers are rare when fertile queens are present; for example, less than 1% of honeybee workers lay eggs (Bourke, 2019 and references therein). Reproducing workers have been compared to cancerous, somatic cell lineages in multicellular organisms, which also selfishly replicate at catastrophic cost to the organism (Tsuji and Dobata, 2011; Teseo et al., 2013). In multicellular organisms, constant immunosurveillance by the immune system identifies and eliminates mutant cells before they develop into cancers via tumor-specific antigens present on malignant cells (Dunn et al., 2002; Pradeu, 2013; Corthay, 2014; Feng et al., 2018). In superorganisms, the main form of policing is workers seeking out and eating the eggs of other workers, which they distinguish from queen-laid eggs by specific chemical odors (Ratnieks and Visscher, 1989). In clonal raider ants, where individuals are genetically identical, all ants reproduce during specific phases of the colony’s lifecycle. However, some ants continue to reproduce uncontrollably outside of these phase, as they fail to respond to regulating signals that control reproductive synchrony in the colony (Teseo et al., 2013). These aberrantly replicating ants are detected through divergent cuticular hydrocarbons (CHCs) and killed by nestmates, similar to the organismal immune system detecting malignant cells via cancer-specific antigens (Teseo et al., 2013; Feng et al., 2018). Mutations that cause ants to behave selfishly have also emerged in this species: by producing more “germline-like” reproductive individuals, mutant lines can monopolize reproduction in chimeric colonies and create an opportunity for the evolution of social parasites (Teseo et al., 2014); a similar result has been found in multicellular cooperation, suggesting that mechanisms to supress the evolution of cheaters is paramount to social stability (Teseo et al., 2013; Bastiaans et al., 2016; Ågren et al., 2019). The removal of dead, non-infectious insects has parallels with the removal of dead, damaged, or dangerous cells in a body, which can also be considered a form of policing (Pradeu, 2013). Apoptotic cells not removed by phagocytes release noxious chemicals that cause tissue inflammation (Nagata and Tanaka, 2017), whilst dead insects left in the colony reduce worker and larvae survival (Diez et al., 2014). Although historically studied from a conflict resolution perspective, policing is clearly a general mechanism to prevent constituent evolution and conflict of fitness within superorganisms, which has direct analogs to the immune policing that prevents intercellular conflicts in the evolution of multicellular organisms (Bourke, 2011; Pradeu, 2013; Ågren et al., 2019). In both cases, policing by the immune system ensures the cooperation of constituent parts and hence maximal (super)organism fitness (Bourke, 2011). Accordingly, we consider actively performed derived traits as part of superorganism immunity (e.g., worker policing), whilst traits that reduce conflict by default are not (e.g., a single-queen bottleneck). This is consistent with the suppression of tumors in multicellular organisms being part of organismal immunity, whilst unitary inheritance (single zygote-bottleneck) is not (Bourke, 2011 and references therein).
Immunological Uniqueness of the Superorganism
It is essential that biological individuals establish a “boundary” within which cooperation between constituents occurs so that the benefits of cooperation circulate between kin (Queller, 2000; West et al., 2015). Immune systems delimit these boundaries in multicellular organisms by deciding what is accepted and rejected as part of the organism (Pradeu, 2013) and allorecognition systems seem to pre-date the evolution of obligate multicellularity (West et al., 2007 and references therein). It is through this process that the immune system maintains the unique identity of the organism across its life, despite it continually changing through growth, soma replacement and aging (Pradeu, 2012). Superorganisms establish their identity through chemical signatures that permit similar “self” recognition (Lenoir et al., 1999). Each insect produces colony-specific, long-chain CHCs on the surface of its body, which are mixed between individuals to create a uniform colony odor. This odor changes over time depending on the nest environment and the food consumed so that it is truly unique to each colony (Lenoir et al., 1999). Like the multicellular immune system that learns to recognize itself early during embryonic development, individual insects are thought to learn their colony odor early in life, by developing an internal representation of the odor, known as a template (Lenoir et al., 1999; Bos and D’Ettorre, 2012). This template can be acquired during the larval stage but seems to become fixed during a time-sensitive window, shortly after adult emergence. The template can still be updated though as colony odor gradually changes over time. Workers discriminate self from nonself by comparing the odor of individuals they meet with their stored template; this results in cooperation when they match and aggression when they differ (Lenoir et al., 1999; Bos and D’Ettorre, 2012). Although it is possible to artificially fuse colonies and replace queens (e.g., during beekeeping), this requires a period of forced habituation, so that odors can presumably become mixed or the template is updated. Under natural conditions, colony fusion is prevented by guard insects that decide who enters the colony and the identification of intruders by all workers within the nest (Lenoir et al., 1999; Bourke, 2011). The “boundaries” of the superorganism are hence established through this odor-based recognition or colony surveillance system (Bourke, 2011).
Although colony fusion events are relatively rare in superorganisms owing to their effective self-nonself recognition system (Lenoir et al., 1999; Kronauer et al., 2010), colony identity and stability is at risk from interspecific social parasitism (Teseo et al., 2014), caused by “soma” and “germline” parasites. In the cape honeybee, for example, a strain of parasitic workers has evolved that transmits horizontally between colonies of a closely related subspecies to lay female eggs via thelytokous parthenogenesis. They give rise to more parasitic workers (Martin et al., 2002) and too many can cause colony collapse. This is remarkably similar to transmissible cancers in organisms, such as the facial tumors of Tasmanian devils (Tsuji and Dobata, 2011; Teseo et al., 2013). In both cases, these parasites are an asexual lineage of somatic origin that has broken free from their natal (super)organism to infect other (super)organisms (Bourke, 2011). Although our understanding of how somatic parasites evade the immune system is still developing, the high virulence of such diseases places a strong selection pressure on hosts to rapidly adapt (Epstein et al., 2016). We predict that workers evolve stronger discrimination abilities in populations where parasites are present, as well as rapid behavioral responses to kill parasites before they infect the colony. Germline parasites are queens that invade colonies of closely related species. Germline parasites either take up residence alongside the true queen or kill her to monopolize reproduction. They then either replace the colony soma with their own offspring or rely on the hosts’ leftover workforce to raise their sexual brood (Brandt et al., 2005a). Germline parasites that hijack host reproduction are not, as far we are aware, known in metazoan organisms, but exist in colonial organisms (Cremer and Sixt, 2009 and references therein). Increased self-nonself discrimination, enhanced defensive behaviors, and the evacuation of the host queen are all mechanisms that colonies utilize to prevent germline infections. It is believed most germline parasites are successfully killed when trying to invade a colony (Brandt et al., 2005a).
Immune Elimination of Pathogens and Parasites
Protection against microbial pathogens has been reviewed extensively elsewhere (Cremer et al., 2007, 2018; Cremer and Sixt, 2009; Wilson-Rich et al., 2009; Evans and Spivak, 2010; Rosengaus et al., 2011; Cremer, 2019), but to summarize the key findings, we see the evolution of colony-level resistance and possibly tolerance mechanisms against microbial diseases in social insects. Resistance encompasses all traits that limit the probability of infection, as well those that reduce pathogen load and lead to pathogen clearance. For example, this includes infection avoidance (Tranter et al., 2015; Pereira and Detrain, 2020), grooming (Hughes et al., 2002; Reber et al., 2011; Tragust et al., 2013a, b), the use of antimicrobials (Stow et al., 2007; Tragust et al., 2013a; Pull et al., 2018b), and reorganization of social networks (Stroeymeyt et al., 2018). Resistance mechanisms affect pathogen fitness and so can select for higher pathogen virulence over time (Cremer et al., 2018). Tolerance is the ability of organisms to cope with the damage caused by a pathogen, rather than targeting the pathogen itself. Consequently, tolerance mechanisms do not reduce pathogen load, hence relaxing selection on pathogen virulence (Råberg et al., 2009; Kutzer and Armitage, 2016). Although rarely studied, there is some evidence that colonies can tolerate the impact of infected workers on colony fitness (Scharf et al., 2012). Indeed, recent work has shown that “lazy” workers specializing on inactivity act as a reserve for when the rest of the worker force is compromised (Charbonneau et al., 2017). This could be a faster mechanism of “soma” replacement than raising new workers from eggs (Cremer et al., 2018). Whether a colony opts for resistance or tolerance will depend on pathogen–host ecology and life history. Highly virulent pathogens should generally always elicit resistance, though annual societies may opt for tolerance over costly resistance, where possible, to maximize reproduction over their comparatively short colony lifespans. Schmid-Hempel (1998) and Boomsma et al. (2005) give extensive summaries on how host life history affects social insect pathogen assemblages and disease protection mechanisms.
Social insect colonies are infested with a startling diversity of other organisms, which range from benign, non-specific associations, to extremely host-specific, co-evolved parasites (Schmid-Hempel, 1998). Although some parasites cause considerable damage to colony health when they act as vectors of other diseases or prevent queen reproduction, they generally seem to have low impacts. Like larger intercellular parasites of animals, which often exist “outside” of the body (e.g., worms in the digestive tract, lice in feathers), these larger colony parasites may be harder to remove because they are not susceptible to many of the superorganism’s immunity defenses. Indeed, many social insect parasites have morphological adaptations to protect them from attack and/or develop chemical profiles that closely match their host, making them undetectable (Schmid-Hempel, 1998). Consequently, many parasites, especially those that have low levels of virulence, are likely to be tolerated. However, encapsulation of parasites (Neumann et al., 2001) and, in heavy infestations, nest abandonment are more drastic options (Cremer et al., 2007). In general, although some systems are well studied (e.g., the Varroa mite; Rosenkranz et al., 2010), less is known about how social insects cope with macro parasites, and this remains an area for exciting future research.
Immunological Cognitive Networks
Organismal cellular immunity is an example of a living cognitive network (Piñero and Solé, 2019). Living cognitive networks are defined by the ability to process information (carry out computations) and draw on past events (possess a form of memory) in order to optimize group-level decision-making. Although the brain is a classic example of a cognitive network that is neuronaly based, this is not a requirement for cognitive network formation in general. We propose that, like the organismal immune system, superorganism immunity functions as an aneural “liquid brain,” where, unlike neuronaly based “solid” brains, the nodes of the cognitive network (immune cells or workers) have no fixed physical location. As in traditional solid brains, interactions based on simple rules between individuals can lead to complex emergent cognitive outputs at the superorganism-level, which are not inherently known to the individuals that make up the network (Couzin and Franks, 2003; Piñero and Solé, 2019), i.e., computation by distributed processing (Gordon, 2016). Collective cognition in both social insect and immune networks share additional key traits aside from their liquid-like nature. The first is somatic division of labor, which is epitomized by the different task-performing groups of social insects and the diverse effector cells found in the immune system. The second trait relates to the similarities in the algorithms that describe the search dynamics of social insects and immune cells. Recent work has highlighted how both adopt a variety of directional as well as random search strategies dependent on the environment and availability of stimuli (Moses et al., 2019). Both rely on frequent contacts between nodes (individuals/cells) as well as on physical structures, such as nest tunnels or vasculature, for guiding movements. A key unifying property is that “there is no one best search strategy that can be used for all search problems […]; instead searchers change how they move and interact with each other and the physical environment in response to specific search problems in specific environments.” (Moses et al., 2019). A search problem common to both types of network is the existence of a trade-off between search speed and accuracy, with optimal algorithms depending in both cases on the spatial layout and temporal stability of targets in the environment. With respect to random searches, studies combining modeling and empirical approaches have revealed important similarities in the random search strategies adopted by ants and T cells (Fricke et al., 2016), though it is unknown if workers utilize such search strategies to conduct colony-level immunosurveillance (Table 1). These findings reveal that fundamental shared tasks between individual and social immune systems (such as the differentiation between self/non-self and the treatment of invading microorganisms) may be regulated by convergent mechanisms of collective action.
In summary, there is compelling evidence that superorganisms have evolved an immune system that contributes significantly to their unique individuality. Although more comparative data are needed, it may even be possible to determine when insect societies have reached key milestones in superorganism evolution by examining the status of their colony-level immune system development. Hence, as discussed in more detail in sections to come, we suggest that, as with the evolution of multicellularity, immune system evolution could be an important criterion for determining when key steps along the road to superorganismality have been taken.
The Role of Immunity in the Major Transition to Superorganismality
In this section, we examine how disease and selection for immunity may have shaped the transition to superorganismality (Figure 2). Major evolutionary transitions can be broken down into three steps, as defined by Bourke (2011): (1) group formation concerns the genetic and ecological factors that initially favor social life, (2) group maintenance prevents exploitation of the social group (e.g., from selfish and parasitic elements), and (3) group transformation is the development of the group into a complex, integrated biological individual. Although group formation and maintenance possibly overlap (West et al., 2015), we consider them separately in order to partition traits that promote group-living from post-group formation adaptations that evolved specifically to protect the new individual. Following Bourke, we use the broad term “group” to describe these processes, though only groups formed through direct co-ancestry (e.g., aggregations of clonal cells or families with retained offspring) can potentially undergo a major transition in individuality (Boomsma and Gawne, 2018 and references therein).
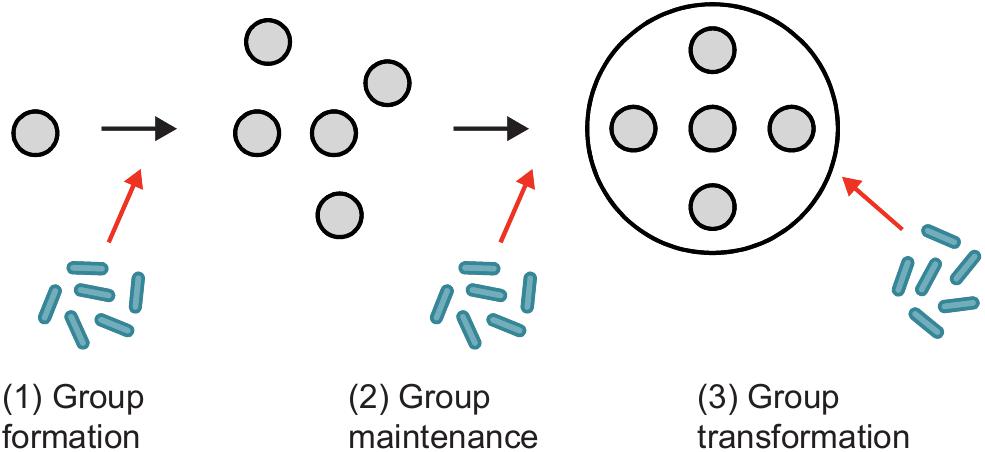
Figure 2. Disease and the emergence of a major transition. (1) Disease causing agents have the potential to both hinder and promote the formation of a social group. For example, an increase in the risk of disease due to the close proximity of relatives may select against group formation, whilst additive effects of individual immunity and cooperative disease defense may positively select for sociality. (2) Once a group has formed, the aforementioned increase in the cost of disease may outweigh the benefits afforded by sociality. Consequently, pre-existing individual-level disease defenses should be co-opted and elaborated to maintain group integrity instead. Additional disease defenses that function specifically to protect the group are therefore predicted to evolve, such as self/non-self recognition and an ability to deal with infected group members. (3) Group transformation into a cohesive, complex biological individual. Increases in complexity (e.g., number of cell or worker types) and size both encourage and require a corresponding increase in immune defenses, as larger, more complex biological individuals are expected to encounter a greater diversity and number of disease-causing agents. (Figure inspired by West et al., 2015).
Group Formation
Formation of a cooperating group is the first step towards a major transition (Bourke, 2011; West et al., 2015). Two conditions favor the evolution of cooperation: ecological and efficiency benefits that encourage cooperation and a mechanism to direct those benefits back to the actor or its relatives (West et al., 2015). The latter is achieved through kin selection where helping relatives ensures shared genes are passed on to the next generation. Benefits promoting cooperation are numerous and well-studied, but the role of disease has largely been neglected (but examples include, Rosengaus et al., 2013; Cole and Rosengaus, 2019; Nuotclà et al., 2019). Although disease has long been considered an antagonist that hinders group formation (Freeland, 1976; Hamilton, 1987), the view that group-living increases infection risk is over-simplistic; studies searching for a correlation between group living and disease risk have produced mixed results (Wilson et al., 2003; Nunn et al., 2015; Ezenwa et al., 2016). On the one hand, this may be due to the evolution of derived traits in social animals that compensate for an increased infection risk (Ezenwa et al., 2016), but on the other, there are likely innate benefits to group-living that reduce the impact of infectious disease. For colonies of cells and insects, the additive effects of individual defenses, i.e., autonomous immunity in cells and individual immunity in insects, may reduce the overall risk of infection in the group and so foster cooperation (e.g., percolation within a heterogenous network; Sander et al., 2002). For example, the individual use of antimicrobial substances by cells and insects in the same space could create a barrier against disease transmission in their nests. Indeed, many solitary and subsocial insects have been shown to use antimicrobial substances, which, in family groups, have important impacts on offspring growth and development (Cotter et al., 2013; Diehl et al., 2015; Meunier, 2015). When close kin live together with little competition, there is no disadvantage to providing direct aid to others; for example, grooming can easily be directed to others instead of oneself. Group-living may also provide indirect benefits that aid recovery from infections, since resource acquisition and other tasks that trade-off with immunity are shared, allowing sick individuals to invest resources into clearing and recovering from infection (Hart, 1990; Ezenwa et al., 2016). Additionally, this may confer improved tolerance to infections where the impact it has on the host is ameliorated, e.g., through increased food consumption (Almberg et al., 2015), leading eventually to reduced pathogen virulence. Pathogen evolution and diversity could be altered by simple social behaviors, such as grooming, leading to disrupted within-host pathogen dynamics during coinfections that result in altered pathogen communities and virulence (Milutinović et al., 2020). This could select for group-living if it positively affects host fitness. Much like during the evolution of the individual immune system, many of the building blocks of superorganism immunity (e.g., hygienic behaviors, external immune activity, etc.) are widespread in non-social insect lineages (Bulmer et al., 2012; Otti et al., 2014; Meunier, 2015). Therefore, initially simple, cooperative behaviors such as grooming, combined with means to deploy disinfectants into the external environment, could both protect the group and encourage further cooperation (Esparza-Mora et al., 2020).
Group Maintenance
Once a group has formed, mechanisms to maintain cooperation should evolve that prevent social cheaters, pathogens and parasites from driving newly emerged groups to extinction (Godfrey-Smith and Goodnight, 2013). Social cheaters harm the group by using its resources but not contributing back. True parasites and pathogens are more likely to spread in groups of relatives, since there is no to little genetic diversity in a colony of clonal cells or insect relatives (Hamilton, 1987). Moreover, in groups of mutually dependent individuals, be they cells or insects, a single infected individual is a hazard to all others: cells and insects that have lost their totipotency cannot simply disperse and reproduce elsewhere when infection breaks out (they are “all in the same boat”; Ågren et al., 2019), even within the more modular insect colonies (Chouvenc and Su, 2012; Loreto and Hughes, 2016). Hence, at this stage, selection should produce defenses that evolve specifically to promote the health of the group, via inclusive fitness benefits, as well as ways to deal with lethal infections. This requires an ability to detect social cheaters and diseases, as discussed above. Recall that cancerous cells possess specific antigens that guide their immune elimination (Urban and Schreiber, 1992; Feng et al., 2018), worker laid eggs an odor that differs to queen laid eggs (Ratnieks and Visscher, 1989), and aberrantly reproducing workers in clonal raider ants have divergent CHC profiles (Teseo et al., 2013). Diseased insects also smell differently to their nestmates and so can be actively eliminated from the colony (Richard et al., 2008; Swanson et al., 2009; Baracchi et al., 2012; Pull et al., 2018b). There is also evidence for the mechanical “pushing out” of cancerous cells clumps from proliferative tissue (Hogan et al., 2009; Ågren et al., 2019). At this stage chemical communication is most likely to be based on simple cues triggered by illness/damage, but, because groups are composed of kin, the use of signals that are actively broadcast may also evolve in more complex groups (Cremer et al., 2018). Signaling of intracellular infections is also paramount in the elimination of compromised cells in a body (Grimsley and Ravichandran, 2003; Ravichandran, 2010; Feng et al., 2018). Moreover, at this step, immune defenses help to delineate the group, i.e., determine what is self and what is non-self, a feature that also emerged very early during the evolutionary origins of animal multicellularity (Müller et al., 1999; Pradeu, 2012). Over time, the accumulation of immunity adaptations that police social cheaters, maintain group identity and prevent infection, led to the formation of stable groups beginning to show organism-like design.
Group Transformation Into a Biological Individual
In rare instances, some stable, cooperating groups developed permanent separation of helper and reproductive roles and underwent a major transition, forming a new kind of biological individual. In many multicellular organisms (such as higher metazoans), the soma component loses its totipotency completely during this transition, and soma and germline roles become irreversibly independent. However, a fully sequestered germline is not generally required for major transitions in fraternal organism evolution (Queller, 2000), as evidenced by its absence in plants and early branching metazoan phyla (Extavour, 2007; Radzvilavicius et al., 2016; Fierro-Constaín et al., 2017). In contrast, group-adapted immune systems are conserved and taxonomically widespread, so may be a more useful criterion for defining major transitions in biological individuality (Pradeu, 2013). This is not to denigrate the importance of germ-soma segregation during the evolution of (metazoan) complexity, where it is clearly correlated with greater individual size and complexity, but we hypothesize that this emerges in parallel to or even after the foundations of a group-level immune system have been established. In metazoan immunity, evidence of a core ancestral immune system is well described (see next section), yet effector cell and immune pathway diversity varies greatly between animal phyla, with mechanisms of adaptive immunity appearing to be restricted to arguably more complex animal lineages (Müller et al., 2018). A comprehensive quantitative comparison of immune system and organismal complexity (as defined by cell-type, tissue diversity and degree of germ-soma segregation) has to our knowledge not been conducted in Metazoa, though, generally, larger organisms have more cell types (Bonner, 2004; Strassmann and Queller, 2007) and a fully sequestered germline. Hence, we predict that gradients of animal complexity, size and immune specialization should be positively correlated with each other. A gradient of complexity is also apparent among social insects (based on colony size and the number of different worker types; Bonner, 1993; Strassmann and Queller, 2007) including species whose colonies have ostensibly passed an evolutionary point of no return in individuality (Ferguson-Gow et al., 2014; Bourke, 2019). With an increase in size and complexity the probability of infection is likely to rise, and available data on parasite richness suggest this relationship holds true (Schmid-Hempel, 1998). A higher density of hosts with more intricate interactions facilitates greater disease transmission in larger colonies; additionally, they are a larger target with more niches for parasites to exploit. For example, different parasite taxa preferentially select small or larger worker castes within a colony as hosts (Schmid-Hempel, 1998). In the fungus-growing ants (higher attines), larger workers that are morphologically specialized for leaf-cutting have small metapleural glands relative to their size and more porous infrabuccal filters than smaller workers, rendering them more susceptible to parasites (Hughes et al., 2002, 2008b). Furthermore, higher levels of foraging and nest expansion in large colonies should increase pathogen exposure and transmission. Consequently, escalating colony size, complexity, and integration will require a corresponding scaling in immunity. Comparative studies between species demonstrate an increase in the strength of antimicrobials used by bees that correlates which correlates with colony size and complexity (Stow et al., 2007). Within species, denser and/or larger colonies produces higher immunocompetence in workers (Ruiz-Gonzalez et al., 2009; Armitage and Boomsma, 2010) and larger colonies have increased survival when exposed to pathogens compared to small ones (Leclerc and Detrain, 2018). Since larger colonies have more workers, exhibit advanced self-organization and increased task specialization, immunity itself should also become more integrated and complex with colony size. With more workers some colony members may be able to specialize on immunity roles (e.g., waste management; Eyer et al., 2013) and novel disease-related tasks can evolve, such as colony-level medication with substances collected from the environment (Chapuisat et al., 2007; Simone-Finstrom and Spivak, 2012; Bos et al., 2015); such behavior is less likely to evolve in smaller societies as the limited number of workers should prioritize essential functions such as food collection. As well as the active elimination of sick individuals by nestmates, self-removal mechanisms should also occur: cellular apoptosis (Michod and Roze, 2001) and social apoptosis (“self-removal”; Rueppell, 2004; Heinze and Walter, 2010; Rueppell et al., 2010; Bos et al., 2012; Page et al., 2016; Leclerc and Detrain, 2017) allow individuals to isolate themselves, thereby protecting their kin (Ugelvig and Cremer, 2007; Stroeymeyt et al., 2018). The causal relationship between the emergence of these advanced traits and superorganism complexity is unclear, and we are open to the view that selection on social immune traits due to ecological pressure could have also facilitated subsequent increases in complexity, rather than vice versa. Although an entirely open area of research, we expect large, more cohesive colonies to have efficient, rapid responses to disease, greater worker specialization for dealing with disease, and an increased protection of their highly specialized but more vulnerable queens, compared to smaller, less complex species.
Evolution of Superorganism Immunity
Several interesting and open questions arise from an immunological perspective of the major evolutionary transitions: do immune systems evolve before a full transition to individuality? Is it possible to undergo a major evolutionary transition without some form of immunity? In the transition to multicellularity some form of immunity probably evolved before biological individuality became fixed and pre-adaptions existed that facilitated immune system evolution. Given their strong, evolutionary convergences (Cremer and Sixt, 2009), organismal and superorganismal immune system evolution likely followed similar patterns.
In organismal immunity, many of the building blocks that make up antimicrobial defense mechanisms can be traced back to progenitor elements found in simpler ancestors. A classic example of this is the RNAi pathway, a conserved intracellular defense system of eukaryotes, which consists of an evolutionarily agglomeration of components derived from diverse prokaryotic ancestors (Shabalina and Koonin, 2008). The co-option and reuse of the same, probably ancient, protein domains appear widespread in the evolution of eukaryotic innate immunity (Richter and Levin, 2019). Importantly, the emergence of novel forms of immunity occurred concomitantly with the major transitions in eukaryotic complexity, and many of these immune functions have been retained ever since. For example, in animals, the Toll-like receptor (TLR) signaling pathway is a key host immune receptor cascade involved in vertebrate innate immunity (Akira, 2003). But fully functioning Toll/TLR signaling pathways are also found in distantly related animal phyla such as Drosophila (Lemaitre et al., 1996) and non-bilaterian animals such as Porifera and Cnidaria (Wiens et al., 2006; Hemmrich et al., 2007; Franzenburg et al., 2012). This demonstrates that an immune role for TLR-signaling evolved early in animal evolution and has been conserved across Metazoa ever since. Effector immune cells are also present from sponges through to mammals and are essential for innate immunity (Buchmann, 2014). Highly conserved cysteine-rich scavenger receptors, as well as G-protein coupled receptor genes, are found across the metazoans, which facilitate effector cell adhesion, self/non self-recognition, phagocytosis and melanization (Müller et al., 1999; Dzik, 2010; Pita et al., 2018). The importance of effector cells in the evolution of multicellular life is highlighted by the slime mould Dictyostelium, a facultatively multicellular organism possessing sentinel cells that engulf bacteria and sequester toxins (Chen et al., 2007). Some sentinel cell and phagocytic functions in Dictyostelium (Chen et al., 2007) involve a TIR-domain containing protein, as well as other signal transducers and activators of transcription that have gene homologs in animals (Dunn et al., 2018), again hinting that these may have evolved early on in multicellular evolution.
Three conclusions emerge from research on organismal immune system evolution that might provide useful insights for studying the evolution of immunity during the major transition to superorganismality: (i) New forms of immunity are constructed from diverse and often unrelated building blocks found in simpler ancestors; (ii) Progenitor building blocks are repeatedly co-opted into immune roles in independent evolutionary lineages; and (iii) New forms of immunity are highly conserved following a major transition, although modifications and additions to core immune processes are widespread. Although the study of disease defense in group-living insects is an important and fascinating area of research in its own right (Cotter and Kilner, 2010; Van Meyel et al., 2018), studies on subsocial and family based cooperative breeders – the most likely ancestors of superorganisms (Linksvayer and Wade, 2005; Nalepa, 2015; Boomsma and Gawne, 2018; Cole and Rosengaus, 2019) – could prove especially useful comparative models for exploring the evolution of social immune systems (e.g., Nuotclà et al., 2019). Indeed, many solitary, familial and aggregative insects possess behavioral and physiological adaptations, such as pathogen avoidance, grooming and the production of antimicrobials (de Roode and Lefèvre, 2012; Meunier, 2015). How these traits become co-opted and modified with the transition to superorganismality, so that they provide colony-level immunity instead of individual protection, is unknown. However, many of the adaptations that we believe are necessary for a social immune system (Table 1) seem to be missing in most non-superorganisms (Meunier, 2015), appearing only in advanced taxa “close” to the superorganismal threshold. Whether this is a true reflection or sampling bias remains to be seen but suggests that novel evolutionary innovations are also important. Although we lack sufficient data to make firm conclusions about the evolution of immunity in social insects, we can speculate, broadly, how superorganism immunity likely emerged concomitantly with the evolution of superorganisms, from their family based ancestors (Figure 3).
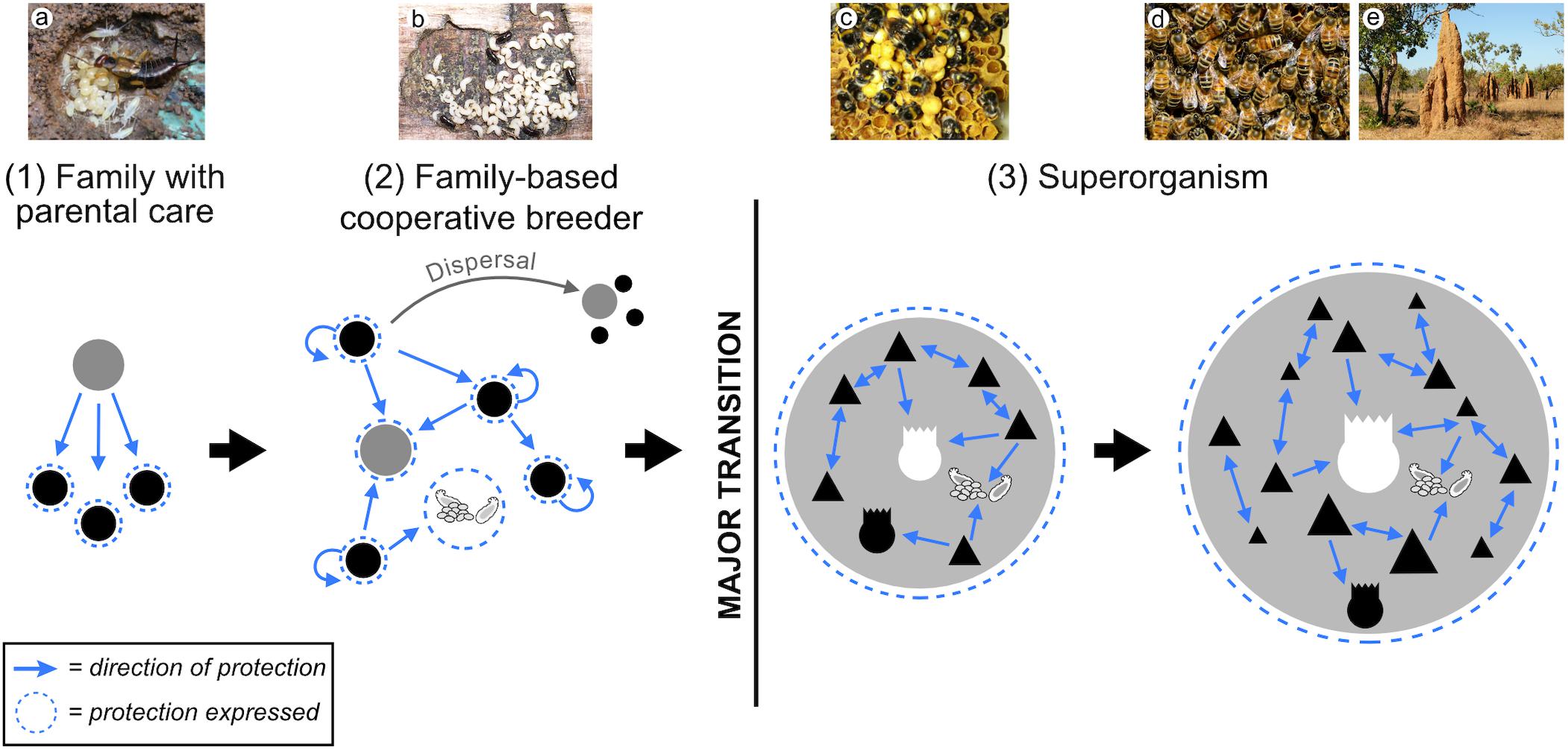
Figure 3. The evolution of superorganism immunity. Superorganisms evolve from subsocial and family based cooperatively breeding ancestors with full-sibling helpers, termed the “subsocial route” to sociality. (1) In insect families [e.g., earwig mother and offspring (a)], extended parental care is primarily administered from parent to offspring to increase the chances of offspring survival and thus parent reproductive success. Both solitary and subsocial insects express many behaviors that appear to have acted as the building blocks upon which social immune systems later evolved. (2) In family based cooperative breeders, some totipotent offspring are retained as helpers at the nest to raise additional siblings. There is considerable variation in insect cooperatively breeding systems, with helpers in some species gaining most of their fitness through independent reproduction, whereas others derive most, if not all, fitness indirectly. Consequently, the evolution of incipient social immune systems in more advanced societies may be observed (i.e., the single piece nesting lower termites). However, in less complex societies where most/all individuals readily disperse [e.g., Xyleborinus ambrosia beetles (b)], social disease defenses are predicted to be less advanced. Hence, we expect social disease protection of individuals, but not a true social immune system. (3) In contrast, obligate worker altruism and the subsequent “transfer of fitness” to the colony-level selects for the evolution of a true immune system in superorganisms. This superorganism immunity is a higher-level adaptation that maximizes superorganism fitness. We predict significant variation in immune system protection that reflects the degree of “individuation” found among superorganisms, i.e., from less advanced [e.g., bumblebees (c)] to more advanced linages [e.g., honeybees (d) and fungus-growing termites (e)]. Photos by: (a) Tom Oates, 2010, available at Wikimedia Commons (CC BY-SA 3.0: https://creativecommons.org/licenses/by-sa/3.0/deed.en), (b) Peter Biedermann, (c) Victoria Blanchard, (d,e) Pixabay.com.
Families With Parental Care
Families are temporary associations between parent and offspring, where either one or both parents provide care to young that disperse once independent (Clutton-Brock, 1991). All individuals are totipotent and the group dissolves once parental care is no longer required. In insects, families with parental care are often termed subsocial to highlight their incipient role in social evolution (Wilson, 1971). Family life with parental care is the first step towards the evolution of complex sociality across the animal kingdom (Hughes et al., 2008a; Chak et al., 2017; Downing et al., 2020). Lifetime monogamy between parents generates maximal sibling relatedness (Boomsma, 2009; Downing et al., 2016, 2020), whilst parental care creates an avenue for helpers to evolve, by putting off dispersal and providing sibling care instead (Holman, 2014).
Parental care seems to have also played an incipient role in the evolution of social immune systems. Based on extant examples, we expect the subsocial ancestors of superorganisms to have already possessed personal immunity (physiological and behavioral defenses) and exhibited at least some form of parental behavior (either direct brood rearing or nest defense), including extended disease protection of offspring (Linksvayer and Wade, 2005; Trumbo, 2012; Nalepa, 2015; Cole and Rosengaus, 2019). One hypothesis, backed by transcriptomic data, is that an earlier expression of parental care genes in retained offspring provided the substrate upon which helper behavior in societies evolved (Linksvayer and Wade, 2005; Rehan et al., 2014). Both ant queens and termite pairs undertake a variety of behavioral disease defenses during colony foundation that are later performed by workers (Chouvenc et al., 2011; Pull and Cremer, 2017; Cole and Rosengaus, 2019), but whether this is evidence that social disease defenses emerge from parental care requires elucidation.
In many Hemiptera, the only parental care provided by mothers is the guarding of eggs from parasitoids, with mothers immediately abandoning their young when they hatch (Wilson, 1971). More widely, preventing microbial or parasitic infection of eggs and young is observed in many taxa (Trumbo, 2012; Cotter et al., 2013). Constructing nests in which to lay eggs and raise young is common and often takes place in microbialy rich soil or wood (Trumbo, 2012). Such behavior imposes a need on parents to evolve antimicrobial defenses that keep the nest environment sanitary; consequently, the use of exocrine gland compounds, antimicrobial faeces and the segregation of potentially harmful waste are commonly observed (Meunier, 2015). Moreover, when food is provided to developing brood it also requires processing so that it does not become a source of contagion, and to prevent microorganisms outcompeting the insect’s young. This has been well studied in burying beetles and beewolfs, where parents use chemicals and/or form permanent symbiosis with antibiotic-producing microbes, to manage microbial communities on food and prevent spoilage (Kaltenpoth et al., 2005; Rozen et al., 2008; Herzner et al., 2011; Rosengaus et al., 2013; Shukla et al., 2018).
As a consequence of parental care, many pre-adaptations, such as grooming, antimicrobial secretions, the formation of stable microbial symbioses, and rudimentary nest structures existed that could have facilitated transitions in insect social complexity, by limiting the impact of disease in prototypical colonies (Figure 4). Moreover, when independent reproduction is risky (Kennedy et al., 2018), the enhanced protection provided by extended parental care may have acted as an additional incentive for offspring to stay with their parents instead, where they can gain both indirect fitness through raising siblings and potentially direct fitness through nest inheritance (Downing et al., 2018; Cole and Rosengaus, 2019). When this switch occurs, family life evolves beyond simple parent-offspring associations, to cooperatively breeding families.
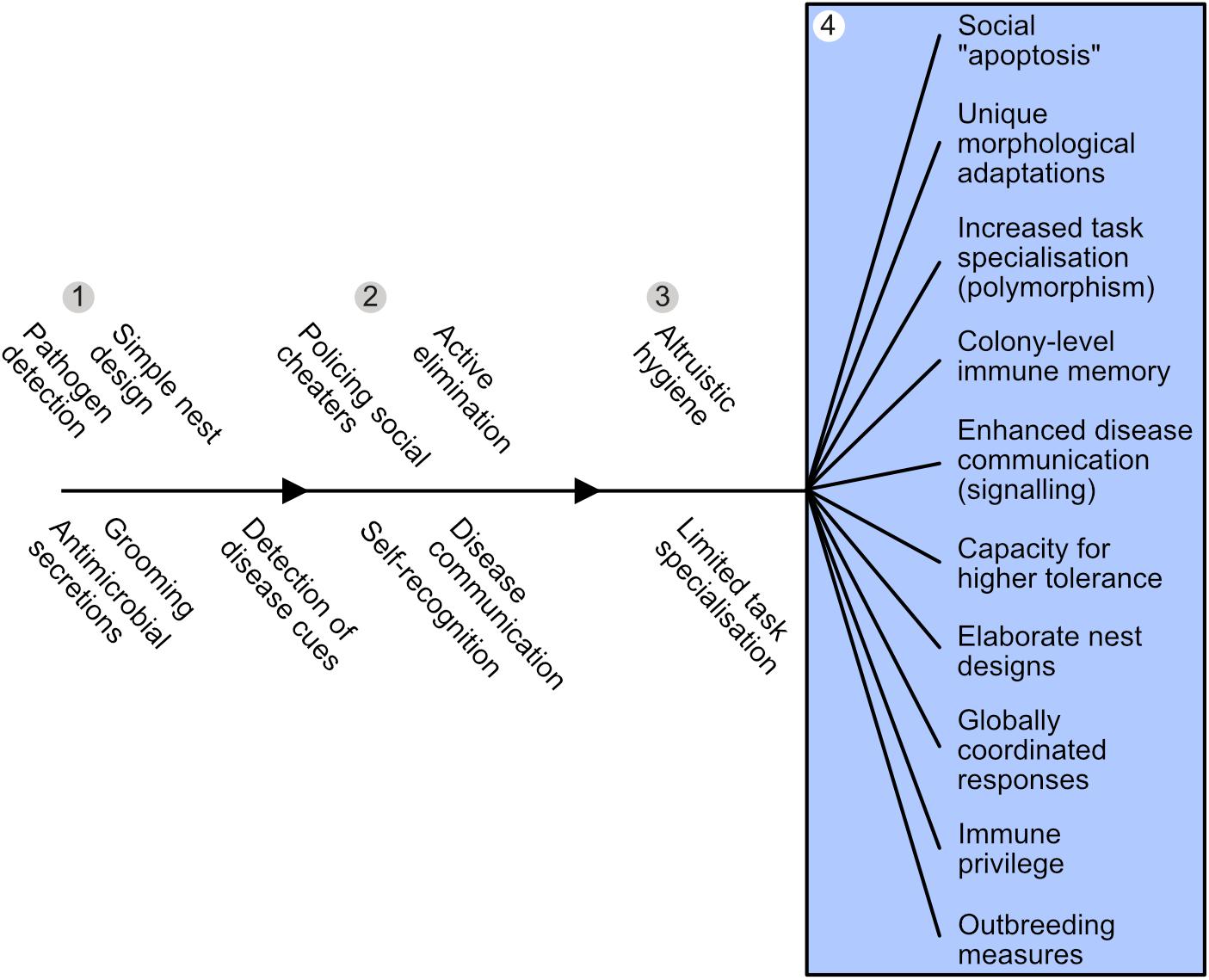
Figure 4. Emergence and diversification of superorganism immunity. Adaptations that may have evolved prior to – and thus enabled – the transition to superorganismality are listed in the order we hypothesize they might appear, as an insect family approaches superorganismality. Colony-level immunity undergoes a systemic diversification and specialization to become a social immune system in superorganisms (blue box) that is functionally equivalent to an individual immune system. (1) Numerous pre-adaptions present in subsocial ancestors, such as pathogen detection, grooming and antimicrobial use, may have facilitated initial insect cooperation. (2) As insect family groups become larger and more interdependent, adaptations are predicted to evolve to counter the increased risk of disease inherent to kin-structured groups living in close social proximity, i.e., detection and/or elimination of sick family members (e.g., either avoidance or cannibalism/exclusion from nest). Additionally, mechanisms to police social cheaters and allorecognition should emerge to maintain social stability. (3) The evolution of altruistic hygiene, where helpers risk survival to augment the fitness of siblings is predicted in species where worker individuals accrue a large but not total proportion of fitness indirectly. Specializations for disease-related tasks are predicted and an incipient social immune system emerges. (4) Where reproductive-worker roles become effectively obligate, social immune systems with corresponding levels of diversification, specialization and intricacy are predicted to emerge (blue box).
Family Based Co-operative Breeders
Cooperative breeders are social groups that exhibit alloparental care. Some individuals help raise offspring that are not their own but, importantly, retain the ability to reproduce in the future (Crespi and Yanega, 1995; Clutton-Brock, 2002). Only family based groups that originate through offspring retention express complex sociality with reproductive division of labor; this is apparent in cooperatively breeding insects (Hughes et al., 2008a; Boomsma, 2009; Boomsma and Gawne, 2018), crustaceans (Chak et al., 2017), birds (Downing et al., 2020), and mammals (Jarvis, 1981; Lukas and Clutton-Brock, 2013). Family based cooperative breeders are the most likely pre-cursors of superorganisms (Boomsma and Gawne, 2018), and have hence also been termed facultatively eusocial (Crespi and Yanega, 1995; Boomsma and Gawne, 2018). Insect examples include polistine wasps, the gall-forming thrips and aphids and ambrosia beetles (Choe and Crespi, 1997; Boomsma and Gawne, 2018).
In these family based cooperative breeders, hygienic measures that are otherwise performed as part of parental care can now be used instead as part of sib-care. Due to pre-existing adaptations from parental care (previous section), each individual is already equipped with behaviors and morphological structures that can provide cooperative disease care within their natal nest, which may result in enhanced disease protection of the family group (Nuotclà et al., 2019). For example, helpers grooming the brood and one another, social microbial management, proactive nest hygiene and the production of antimicrobial substances are frequently found (Benton and Foster, 1992; Turnbull et al., 2012; Biedermann and Rohlfs, 2017; Nuotclà et al., 2019). Within the cooperative breeders, we find a gradient of social complexity, with the social aphids and thrips and their physically differentiated soldier castes, sitting close to the superorganismal border (Boomsma and Gawne, 2018). Closer still are the many one-piece/wood-dwelling lower termites, such as Zootermopsis. In such species, colony life is obligate and perennial, queens are moderately specialized for reproduction and pre-soldier instars can become reproductive (Myles, 1986), although this capacity is lost following the final soldier moult (Thorne, 1997). The helpers (“false-workers”) behave altruistically but are totipotent and, aside from individuals destined to become soldiers, can independently reproduce under the right colony conditions (either by dispersing or through nest inheritance; Shellman-Reeve, 1997; Myles, 1999; Korb et al., 2012; Boomsma and Gawne, 2018). Their colonies are also small, have simple nest architecture, and helpers lack any clear task specialization (Rosengaus and Traniello, 1993). Although social disease defenses are clearly documented in other cooperative breeders (Benton and Foster, 1992; Turnbull et al., 2012; Nuotclà et al., 2019), decades of work by Rosengaus (Rosengaus et al., 2011 and references therein) shows that collective immune defenses are very well-developed in Zootermopsis: for example, grooming is effective against highly virulent pathogens (Rosengaus et al., 1998), they inform nestmates of the presence of pathogens (Rosengaus et al., 1999) and they produce effective antimicrobials (Rosengaus et al., 2004).
It is probably no coincidence that Zootermopsis and one-piece nesting termite species (Calleri et al., 2010), which seem to have progressed some way towards superorganismality due to the presence of a permanent and irreversible physical soldier caste, have considerable cooperative disease defenses. This suggests that collective immune defenses evolve before – and probably thus enable – transitions to superorganismality. We believe there are several reasons for this: when offspring are retained at the nest as helpers, the per capita risk of disease increases due to the density of potential hosts and the frequency of social interactions between them (Schmid-Hempel, 1998); nests begin to accumulate larger amounts of dangerous waste; and, chiefly, since family based groups are by definition closely related, they are more susceptible to the same pathogens, which is especially true in clonal and inbred species (Hamilton, 1987; Chapman et al., 2000; Abbot et al., 2001). When the presence of helpers reduces the cost of disease for parents, even initially small effects on parental fitness could select for further social immune elaborations in retained offspring (Holman, 2014). This positive feedback on fitness might then strengthen selection for reproductive division of labor, as disease-related tasks are inherently risky (Cooper and West, 2018); indeed, specific adaptations against disease seem to be more apparent in physical castes that are sterile (Benton and Foster, 1992; Turnbull et al., 2012). Division of labor may also free reproducers of some of the costs of immune investment – possibly improving fecundity – whilst increasing their dependency on the helpers for immune protection. The more advanced a cooperatively breeding society becomes (i.e., larger with more division of labor; Cooper and West, 2018), the greater the interdependency between reproductive and helpers will be, and the likelihood that a social immune system thus evolves as part of the process of individuation leading to superorganismality. Immunity-driven changes in reproducer-helper dependency may even accelerate this transition (based on positive feedback; Holman, 2014).
Superorganisms
Superorganismal adaptations can only evolve when within-colony selection of constituents is negligible (Gardner and Grafen, 2009). In superorganisms, within-colony selection is negated by strict lifetime monogamy of colony founders, resulting in maximal sibling relatedness equal to that of parent and offspring, and genetic bottlenecking during colony foundation (Gardner and Grafen, 2009; Boomsma and Gawne, 2018). However, recall that policing mechanisms are still necessary to mitigate lower-level constituent evolution (Ågren et al., 2019). During the process of individualization, there is a “transfer of fitness” from the lower-level constituents to the new, higher-level entity, so that it becomes the fitness-maximizing biological individual. When this process is complete, such that queen and worker roles become totally interdependent, workers become as irrevocably committed to their natal colony as a somatic cell is to a human body (Boomsma and Gawne, 2018). This eliminates conflict over the performance of somatic tasks by workers, since it is in the interest of all colony members to grow the colony, find food, maintain nest homeostasis and prevent disease (Boomsma and Gawne, 2018). Moreover, it is likely that queens in mature colonies become heavily dependent on the workers to keep them healthy due to extreme adaptations for reproduction (e.g., physogastry in termite queens prevents self-grooming).
Unlike a totipotent helper, superorganism workers are free to evolve specializations that solely enhance the fitness of the superorganism, as they are unrestricted by potential costs to independent reproduction (Gardner, 2013). For example, physical worker castes that are morphologically specialized for certain tasks only evolve in Hymenoptera once superorganismality is achieved (Beekman and Oldroyd, 2019). Superorganismality subsequently selects for “better” workers (and queens) with specific adaptations for superorganism immunity, such as the potential evolution of “social apoptosis” (support for which is seen in: Heinze and Walter, 2010; Bos et al., 2012; Page et al., 2016; Leclerc and Detrain, 2017; Pull et al., 2018b), disease-reducing morphological adaptations (Stow et al., 2007; Morgan, 2008), physical task specialization (Hughes et al., 2010), sophisticated disease communication (Richard et al., 2008; Baracchi et al., 2012; Hernández López et al., 2017; Pull et al., 2018b), and globally coordinated responses to infection (Hart and Brown, 2002; Stroeymeyt et al., 2018), among multiple possible traits (Figure 4). In other words, disease defense in superorganisms can undergo a complete developmental overhaul, to become as specialized, diversified and integrated as necessary, according to a superorganism’s life history and pathogen pressure (Boomsma et al., 2005; Boomsma and Gawne, 2018; Cremer et al., 2018). This advanced, derived form of immune protection – or superorganism immunity – hence constitutes a colony-level immune system that exists to provide systemic colony protection, resulting in maximal superorganism fitness.
Once obligate queen-worker roles evolve, measures such as polyandry can also develop that increase within-colony genetic heterozygosity. Not only does polyandry decrease a colony’s disease susceptibility (Tarpy, 2003; Hughes et al., 2004; Seeley and Tarpy, 2007) and make it harder for pathogens to adapt to their hosts (Hughes et al., 2004), it is also likely that increased colony heterozygosity improves the efficacy of other traits. For example, it has been shown that tramp ant colonies with higher heterozygosity have improved pathogen removal abilities than colonies with lower heterozygosity (due to experimental inbreeding; Ugelvig et al., 2010; but see also: Reber et al., 2008) and that polyandrous leaf cutting ants have larger metapleural glands than monogamous species (Hughes et al., 2008b). Polyandry also enhances task specialization: in desert ants, task specialization including waste management is partly controlled by patriline (Eyer et al., 2013) and recent work on altruistic rescue behavior reveals that such specialization is both heritable and genetically determined, with some patrilines being more likely to act as “first responders” (Andras et al., 2020). Consequently, polyandry – which can only evolve in superorganismal colonies (Hughes et al., 2008a; Boomsma, 2009; Beekman and Oldroyd, 2019) – may further increase the effectiveness and complexity of superorganism immunity.
Future Directions
The idea we have endeavored to advance in this review, that superorganisms have evolved an immune system and that immunity enables major transitions, are hypotheses that require rigorous testing. Of particular importance is determining when a social immune system has evolved and how this relates to the transition to superorganismality; that is, does immunity evolve before, with or after this transition? Whilst it is likely that many ant, bee and termite species do indeed have a social immune system, a major difficulty is that, like the metazoan immune system, it also represents a distributed “organ”: it is an incredibly diverse and diffuse self-organized set of adaptations that emerges from local interactions between individuals with their nestmates, the brood, reproductives, nest architecture and the parasites/pathogens themselves, in addition to the actions of contaminated and sick nestmates. This makes it difficult to pin down exactly when an immune system has emerged, since it needs to be studied holistically and should be backed up with comparative analyses across species. Despite these challenges, we are optimistic that a set of criteria can be established for determining when colony-level immunity has evolved (Table 1). We hope that by highlighting the general importance of immunity in the major transitions (Pradeu, 2013), it can be used alongside other important criteria such as queen-worker differentiation (Wheeler, 1911; Boomsma and Gawne, 2018), to determine which species are superorganisms.
We currently lack good data showing that superorganism immune systems affect colony fitness and are heritable (Cremer et al., 2018). Specific behaviors have been shown to have an inherited genetic component (Rothenbuhler, 1964; Eyer et al., 2013), but studies are still needed that show social immune systems are shaped by natural selection. We can theorize that colonies with enhanced superorganism immunity should exhibit lower worker and brood mortality rates (Diez et al., 2014); higher levels of worker productivity, because activity and lifespans of healthy workers will not be affected by immunity trade-offs or pathogen-induced cognitive impairment (Moret and Schmid-Hempel, 2000; Gomez-moracho et al., 2017); fair better in intra- and interspecific competition for resources; and have well provisioned healthy queens that lay more eggs. A relatively unexplored aspect of colony immunity is the cost it incurs. For example, increased levels of grooming and the production of expensive glandular secretions will likely entail productivity and metabolic costs that trade-off with other colony-level tasks. Nest disinfection with caustic chemicals that harm nestmates (Poulsen et al., 2002; Theis et al., 2015; Pull et al., 2018a) and excessive use of destructive hygienic behaviors, such as cannibalism (Davis et al., 2018), could represent forms of superorganism autoimmunity. At a macroevolutionary scale, the presence of a sting for example in ants is costly, and precludes the evolution of other traits, such as larger colony size and defensive spines (Blanchard and Moreau, 2017). Consequently, just as immune systems trade-off with other fitness determining traits in organisms, we expect colony-level immunity to cause life history trade-offs at the level of the superorganism.
A major transitions perspective has helped in our understanding of the ultimate explanations for the evolution of superorganism immunity (Bourke, 2011; Cremer et al., 2018). But the recent suggestion that the evolution of biological individuality begins at the switch to superorganismality (Bourke, 2019) opens up a new line of research that is so far relatively unexplored; namely, that increases in colony complexity (measured as colony size and number of worker types; Bonner, 1993, 2004; Strassmann and Queller, 2007; Ferguson-Gow et al., 2014) will have strong influences on the evolution of colony-level immunity, or vice-versa (Burchill and Moreau, 2016). We would even suggest, based on work in single-piece nesting lower termites (Rosengaus et al., 2011), that the process of individuation begins before a major transition occurs. This is because an incipient immune system of some form is likely necessary to enable a major transition and so probably evolves during the group maintenance stage as it transitions into a biological individual, along with other adaptations that accrue to benefit the survival of the group. Still, we expect that once a major transition has occurred, superorganism immunity can undergo expansive evolutionary innovation and diversification, as workers become as specialized as necessary for the task of immunity (Figure 4; Gardner, 2013; Boomsma and Gawne, 2018). This may in part explain informal observations that some superorganismal taxa, such as the small, relatively simple societies of bumblebees, appear still to rely more on individual immunity than their colony-level immunity compared to the more cohesive, larger colonies of honeybees, i.e., honeybees have undergone greater individuation (Bourke, 2019). Rigorous testing and comparative analyses across species are, therefore, needed to examine whether an immunity–complexity correlation exists, and such research will undoubtedly uncover important relationships about the direction of the causes and consequences of immunological complexity, which may also help to explain patterns of complexity observed in the multicellular domain.
Another important aspect is understanding superorganism immunity evolution. One approach to this question is to examine organisms that most likely resemble the family based ancestors of modern day social insects (Meunier, 2015). Burying beetles with extensive parental care are now well-established models of cooperation and conflict in families (Scott, 1998), and, more recently, earwig family life has become another promising model system to study parental care in insects (Diehl et al., 2015). Both taxa use antimicrobial chemicals to control and shape the microbial community that surrounds developing offspring (Hoback et al., 2004; Gasch et al., 2013), with interesting trade-offs between individual immunity and how much care they can invest into to their young (Cotter et al., 2013; Diehl et al., 2015). Another promising but understudied system are the wood-eating cockroaches of the genus Cryptocercus. Phylogenetic approaches group these insects together in a clade with termites, indicating the latter likely evolved from subsocial wood eating cockroach ancestors (Lo et al., 2000). Indeed, Cryptocercus exhibit extended parental care and have faeces with antifungal properties; it has been suggested that these and similar pre-adaptations were factors encouraging the evolution of sociality in termites (Rosengaus et al., 2013; Nalepa, 2015). Studying family based cooperative breeders, such as ambrosia beetles (Nuotclà et al., 2019), will undoubtedly shed light on the role of relatedness, cooperation and conflict in the evolution of social disease defenses. In most of these models, the whole lifecycle can be observed in the laboratory, offspring relatedness may be manipulated through cross fostering and in- or outbreeding of parents, and selection experiments with pathogens are also possible. Moreover, studying social disease defense will help identify the adaptations that served as pre-cursors to superorganism immunity and facilitated superorganism evolution. Such an approach could also be used to determine the criteria used to define major transitions in biological individuality, which has represented a source of controversy in recent years (Godfrey-Smith and Goodnight, 2013; Boomsma and Gawne, 2018). For example, is a strict segregation between germ and soma really necessary for explaining a major transition, or could immune system evolution be better placed to define this boundary (Pradeu, 2013)? Patterns of immune system and germ-soma evolution in Metazoa suggest that the former holds greater explanatory power. This question could be addressed comparatively in the termites, where species have either helpers that almost all eventually try to breed, species where ≤1% of true workers become reproductive, and species with total worker sterility (Shellman-Reeve, 1997). Additionally, do the social disease defenses of cooperative breeders such as ambrosia beetles, thrips and aphids constitute crude collective immunity, comparable to the crude immunity of facultatively multicellular organisms (Chen et al., 2007)? By taking such a broad, comparative approach, we might be able to answer general questions about immune system evolution that are difficult or impossible to address using multicellular models alone.
Conclusion
In the years since Hamilton’s original work identifying disease as a major constraint on the evolution of insect sociality (Hamilton, 1987), our view of how social insects overcome the problem of disease has shifted dramatically, from a focus on genetic resistance to a well-developed, comprehensive understanding of the role of disease defense in social insect evolution. By viewing immunity through the lens of the major transitions, and unifying it with the recent resurrection of Wheeler’s original superorganism concept (Wheeler, 1911; Boomsma and Gawne, 2018), there is growing evidence that social insects have evolved an immune system that is convergent with the individual immune system of multicellular organisms. Since immune systems are crucial to the evolution of individuality and provide a level of protection that goes beyond mere infectious disease defense (Pradeu, 2012, 2013) – the major focus of social immunity research – we suggest the use of the term superorganism immunity to describe the protection arising from a fully functioning (social) immune system (Aanen, 2018). We hope that future research on social disease defenses in family based groups can begin to reveal how disease selection pressures initially promote group living, and how, in cooperatively breeding species, these behaviors maintain sociality once a stable group has formed; both of which are necessary steps for a major transition in superorganismality to occur. Importantly, such research would help provide us with fundamental insights into how selection acting on groups of cooperating relatives produces complex, higher-level adaptations, such as immunity in (super)organisms. Researchers have only just begun to scratch the surface of the evolution and ecology of superorganism immunity in recent years, and we hope that this review helps to stimulate further work in this burgeoning field.
Author Contributions
Both authors contributed to the original idea, graphs, writing, researching, and editing of the manuscript.
Funding
DM is supported by the Deutsche Forschungsgemeinschaft (DFG), grant number MC 436/5-1.
Conflict of Interest
The authors declare that the research was conducted in the absence of any commercial or financial relationships that could be construed as a potential conflict of interest.
Acknowledgments
We would like to thank Sylvia Cremer and Barbara Milutinović for valuable discussion and comments on the manuscript, as well as Sheena Cotter and Patrick Kennedy, for their constructive and helpful feedback that greatly improved this review. We acknowledge the support given by the Open Access Publication Initiative of Freie Universität Berlin.
References
Aanen, D. K. (2018). Social immunity: the disposable individual. Curr. Biol. 28, R322–R324. doi: 10.1016/j.cub.2018.02.050
Abbot, P., Withgott, J. H., and Moran, N. A. (2001). Genetic conflict and conditional altruism in social aphid colonies. Proc. Natl. Acad. Sci. U.S.A. 98, 12068–12071. doi: 10.1073/pnas.201212698
Ågren, J. A., Davies, N. G., and Foster, K. R. (2019). Enforcement is central to the evolution of cooperation. Nat. Ecol. Evol. 3, 1018–1029. doi: 10.1038/s41559-019-0907-1
Akira, S. (2003). Toll-like receptor signaling. J. Biol. Chem. 278, 38105–38108. doi: 10.1074/jbc.R300028200
Almberg, E. S., Cross, P. C., Dobson, A. P., Smith, D. W., Metz, M. C., Stahler, D. R., et al. (2015). Social living mitigates the costs of a chronic illness in a cooperative carnivore. Ecol. Lett. 18, 660–667. doi: 10.1111/ele.12444
Andras, J. P., Hollis, K. L., Carter, K. A., Couldwell, G., and Nowbahari, E. (2020). Analysis of ants’ rescue behavior reveals heritable specialization for first responders. J. Exp. Biol. 223:jeb212530. doi: 10.1242/jeb.212530
Armitage, S. A. O., and Boomsma, J. J. (2010). The effects of age and social interactions on innate immunity in a leaf-cutting ant. J. Insect Physiol. 56, 780–787. doi: 10.1016/j.jinsphys.2010.01.009
Baer, B., and Schmid-Hempel, P. (1999). Experimental variation in polyandry affects parasite loads and fitness in a bumble-bee. Nature 397, 151–154. doi: 10.1038/16451
Baracchi, D., and Cini, A. (2014). A socio-spatial combined approach confirms a highly compartmentalised structure in honeybees. Ethology 120, 1167–1176. doi: 10.1111/eth.12290
Baracchi, D., Fadda, A., and Turillazzi, S. (2012). Evidence for antiseptic behaviour towards sick adult bees in honey bee colonies. J. Insect Physiol. 58, 1589–1596. doi: 10.1016/j.jinsphys.2012.09.014
Bastiaans, E., Debets, A. J. M., and Aanen, D. K. (2016). Experimental evolution reveals that high relatedness protects multicellular cooperation from cheaters. Nat. Commun. 7, 1–10. doi: 10.1038/ncomms11435
Beekman, M., and Oldroyd, B. P. (2019). Conflict and major transitions — why we need true queens. Curr. Opin. Insect Sci. 34, 73–79. doi: 10.1016/j.cois.2019.03.009
Benton, T. G., and Foster, W. A. (1992). Altruistic housekeeping in a social aphid. Proc. R. Soc. B 247, 199–202. doi: 10.1098/rspb.1992.0029
Beros, S., Jongepier, E., Hagemeier, F., and Foitzik, S. (2015). The parasite’s long arm: a tapeworm parasite induces behavioural changes in uninfected group members of its social host. Proc. R. Soc. B 282:20151473. doi: 10.1098/rspb.2015.1473
Biedermann, P. H., and Rohlfs, M. (2017). Evolutionary feedbacks between insect sociality and microbial management. Curr. Opin. Insect Sci. 22, 92–100. doi: 10.1016/j.cois.2017.06.003
Blanchard, B. D., and Moreau, C. S. (2017). Defensive traits exhibit an evolutionary trade-off and drive diversification in ants. Evolution 71, 315–328. doi: 10.1111/evo.13117
Bonner, J. T. (2004). Perspective: the size-complexity rule. Evolution 58, 1883–1890. doi: 10.1111/j.0014-3820.2004.tb00476.x
Boomsma, J. J. (2009). Lifetime monogamy and the evolution of eusociality. Philos. Trans. R. Soc. B 364, 3191–3207. doi: 10.1098/rstb.2009.0101
Boomsma, J. J., and Gawne, R. (2018). Superorganismality and caste differentiation as points of no return: how the major evolutionary transitions were lost in translation. Biol. Rev. 93, 28–54. doi: 10.1111/brv.12330
Boomsma, J. J., and Ratnieks, F. L. W. (1996). Paternity in eusocial Hymenoptera. Philos. Trans. R. Soc. B 351, 947–975. doi: 10.1098/rstb.1996.0087
Boomsma, J. J., Schmid-Hempel, P., and Hughes, W. O. H. (2005). “Life histories and parasite pressure across the major groups of social insects,” in Insect Evolutionary Ecology, eds M. D. E. Fellowes, G. Holloway, and J. Rolff (Wallingford: CABI Publishing), 139–176. doi: 10.1079/9780851998121.0139
Bos, N., and D’Ettorre, P. (2012). Recognition of social identity in ants. Front. Psychol. 3:83. doi: 10.3389/fpsyg.2012.00083
Bos, N., Lefèvre, T., Jensen, A. B., and D’Ettorre, P. (2012). Sick ants become unsociable. J. Evol. Biol. 25, 342–351. doi: 10.1111/j.1420-9101.2011.02425.x
Bos, N., Sundström, L., Fuchs, S., and Freitak, D. (2015). Ants medicate to fight disease. Evolution 69, 2979–2984. doi: 10.1111/evo.12752
Bot, A. N. M., Ortius-Lechner, D., Finster, K., Maile, R., and Boomsma, J. J. (2002). Variable sensitivity of fungi and bacteria to compounds produced by the metapleural glands of leaf-cutting ants. Insectes Soc. 49, 363–370. doi: 10.1007/PL00012660
Bourke, A. F. (2019). Inclusive fitness and the major transitions in evolution. Curr. Opin. Insect Sci. 34, 61–67. doi: 10.1016/j.cois.2019.03.008
Brandt, M., Foitzik, S., Fischer-Blass, B., and Heinze, J. (2005a). The coevolutionary dynamics of obligate ant social parasite systems – between prudence and antagonism. Biol. Rev. 80, 251–267. doi: 10.1017/S1464793104006669
Brandt, M., Heinze, J., Schmitt, T., and Foitzik, S. (2005b). A chemical level in the coevolutionary arms race between an ant social parasite and its hosts. J. Evol. Biol. 18, 576–586. doi: 10.1111/j.1420-9101.2004.00867.x
Buchmann, K. (2014). Evolution of innate immunity: clues from invertebrates via fish to mammals. Front. Immunol. 5:459. doi: 10.3389/fimmu.2014.00459
Bulmer, M. S., Denier, D., Velenovsky, J., and Hamilton, C. (2012). A common antifungal defense strategy in Cryptocercus woodroaches and termites. Insectes Soc. 59, 469–478. doi: 10.1007/s00040-012-0241-y
Burchill, A. T., and Moreau, C. S. (2016). Colony size evolution in ants: macroevolutionary trends. Insectes Soc. 63, 291–298. doi: 10.1007/s00040-016-0465-3
Calleri, D. V. II, McGrail Reid, E., Rosengaus, R. B., Vargo, E. L., and Traniello, J. F. (2006). Inbreeding and disease resistance in a social insect: effects of heterozygosity on immunocompetence in the termite Zootermopsis angusticollis. Proc. R. Soc. B 273, 2633–2640. doi: 10.1098/rspb.2006.3622
Calleri, D. V. II, Rosengaus, R. B., and Traniello, J. F. (2010). Disease resistance in the drywood termite, Incisitermes schwarzi: does nesting ecology affect immunocompetence? J. Insect Sci. 10:44. doi: 10.1673/031.010.4401
Calleri, D. V. II, Rosengaus, R. B., and Traniello, J. F. A. (2005). Disease and colony foundation in the dampwood termite Zootermopsis angusticollis: the survival advantage of nestmate pairs. Naturwissenschaften 92, 300–304. doi: 10.1007/s00114-005-0630-4
Chak, S. T. C., Duffy, J. E., Hultgren, K. M., and Rubenstein, D. R. (2017). Evolutionary transitions towards eusociality in snapping shrimps. Nat. Ecol. Evol. 1:0096. doi: 10.1038/s41559-017-0096
Chapman, T. W., Crespi, B. J., Kranz, B. D., and Schwarz, M. P. (2000). High relatedness and inbreeding at the origin of eusociality in gall-inducing thrips. Proc. Natl. Acad. Sci. U.S.A. 97, 1648–1650. doi: 10.1073/pnas.020510097
Chapuisat, M., Oppliger, A., Magliano, P., and Christe, P. (2007). Wood ants use resin to protect themselves against pathogens. Proc. R. Soc. B 274, 2013–2017. doi: 10.1098/rspb.2007.0531
Charbonneau, D., Sasaki, T., and Dornhaus, A. (2017). Who needs ‘lazy’ workers? Inactive workers act as a ‘reserve’ labor force replacing active workers, but inactive workers are not replaced when they are removed. PLoS One 12:e0184074. doi: 10.1371/journal.pone.0184074
Chen, G., Zhuchenko, O., and Kuspa, A. (2007). Immune-like phagocyte activity in the social amoeba. Science 317, 678–681. doi: 10.1126/science.1143991
Choe, J. C., and Crespi, B. J. (1997). The Evolution of Social Behaviour in Insects and Arachnids. Cambridge: Cambridge University Press.
Chouvenc, T., Efstathion, C. A., Elliott, M. L., and Su, N.-Y. (2013). Extended disease resistance emerging from the faecal nest of a subterranean termite. Proc. R. Soc. B 280:20131885. doi: 10.1098/rspb.2013.1885
Chouvenc, T., Robert, A., Sémon, E., and Bordereau, C. (2011). Burial behaviour by dealates of the termite Pseudacanthotermes spiniger (Termitidae, Macrotermitinae) induced by chemical signals from termite corpses. Insectes Soc. 59, 119–125. doi: 10.1007/s00040-011-0197-3
Chouvenc, T., and Su, N.-Y. (2012). When subterranean termites challenge the rules of fungal epizootics. PLoS One 7:e34484. doi: 10.1371/journal.pone.0034484
Clarke, E., and Okasha, S. (2013). “Species and organisms: what are the problems,” in From Groups to Individuals: Evolution and Emerging Individuality, eds F. Bouchard and P. Huneman (Cambridge, MA: MIT Press), 55–76.
Clutton-Brock, T. (2002). Breeding together: kin selection and mutualism in cooperative vertebrates. Science 296, 69–72. doi: 10.1126/science.296.5565.69
Clutton-Brock, T. H. (1991). The Evolution of Parental Care. Princeton, NJ: Princeton University Press.
Cole, E. L., and Rosengaus, R. B. (2019). Pathogenic dynamics during colony ontogeny reinforce potential drivers of termite eusociality: mate assistance and biparental care. Front. Ecol. Evol. 7:473. doi: 10.3389/fevo.2019.00473
Cooper, G. A., and West, S. A. (2018). Division of labour and the evolution of extreme specialization. Nat. Ecol. Evol. 2, 1161–1167. doi: 10.1038/s41559-018-0564-9
Corthay, A. (2014). Does the immune system naturally protect against cancer? Front. Immunol. 5:197. doi: 10.3389/fimmu.2014.00197
Cotter, S. C., and Kilner, R. M. (2010). Personal immunity versus social immunity. Behav. Ecol. 21, 663–668. doi: 10.1093/beheco/arq070
Cotter, S. C., Littlefair, J. E., Grantham, P. J., and Kilner, R. M. (2013). A direct physiological trade-off between personal and social immunity. J. Anim. Ecol. 82, 846–853. doi: 10.1111/1365-2656.12047
Couvillon, M. J., Segers, F. H. I. D., Cooper-Bowman, R., Truslove, G., Nascimento, D. L., Nascimento, F. S., et al. (2013). Context affects nestmate recognition errors in honey bees and stingless bees. J. Exp. Biol. 216, 3055–3061. doi: 10.1242/jeb.085324
Couzin, I. D., and Franks, N. R. (2003). Self-organized lane formation and optimized traffic flow in army ants. Proc. R. Soc. B 270, 139–146. doi: 10.1098/rspb.2002.2210
Cremer, S. (2019). Social immunity in insects. Curr. Biol. 29, R458–R463. doi: 10.1016/j.cub.2019.03.035
Cremer, S., Armitage, S. A. O., and Schmid-Hempel, P. (2007). Social immunity. Curr. Biol. 17, 693–702. doi: 10.1016/j.cub.2007.06.008
Cremer, S., Pull, C. D., and Fürst, M. A. (2018). Social immunity: emergence and evolution of colony-level disease protection. Annu. Rev. Entomol. 63, 105–123. doi: 10.1146/annurev-ento-020117-043110
Cremer, S., and Sixt, M. (2009). Analogies in the evolution of individual and social immunity. Philos. Trans. R. Soc. B 364, 129–142. doi: 10.1098/rstb.2008.0166
Crespi, B. J., and Yanega, D. (1995). The definition of eusociality. Behav. Ecol. 6, 109–115. doi: 10.1093/beheco/6.1.109
da Silva, I. B., Haifig, I., Vargo, E. L., Casarin, F. E., da Mota, M. L., Lima, J. T., et al. (2019). Ergatoid reproductives in the Neotropical termite Nasutitermes aquilinus (Holmgren) (Blattaria: Isoptera: Termitidae): developmental origin, fecundity, and genetics. Insect Sci. doi: 10.1111/1744-7917.12727 [Epub ahead of print].
Dannenberg, L. C., and Seaver, E. C. (2018). Regeneration of the germline in the annelid Capitella teleta. Dev. Biol. 440, 74–87. doi: 10.1016/j.ydbio.2018.05.004
Davis, H. E., Meconcelli, S., Radek, R., and McMahon, D. P. (2018). Termites shape their collective behavioural response based on stage of infection. Sci. Rep. 8, 14433. doi: 10.1038/s41598-018-32721-7
De Mulder, K., Kuales, G., Pfister, D., Willems, M., Egger, B., Salvenmoser, W., et al. (2009). Characterization of the stem cell system of the acoel Isodiametra pulchra. BMC Dev. Biol. 9:69. doi: 10.1186/1471-213X-9-69
de Roode, J. C., and Lefèvre, T. (2012). Behavioral immunity in insects. Insects 3, 789–820. doi: 10.3390/insects3030789
Diehl, J. M., Körner, M., Pietsch, M., and Meunier, J. (2015). Feces production as a form of social immunity in an insect with facultative maternal care. BMC Evol. Biol. 15:40. doi: 10.1186/s12862-015-0330-4
Diez, L., Lejeune, P., and Detrain, C. (2014). Keep the nest clean: survival advantages of corpse removal in ants. Biol. Lett. 10:20140306. doi: 10.1098/rsbl.2014.0306
Downing, P. A., Cornwallis, C. K., and Griffin, A. S. (2016). How to make a sterile helper. BioEssays 39:e201600136. doi: 10.1002/bies.201600136
Downing, P. A., Griffin, A. S., and Cornwallis, C. K. (2018). Sex differences in helping effort reveal the effect of future reproduction on cooperative behaviour in birds. Proc. R. Soc. B 285:20181164. doi: 10.1098/rspb.2018.1164
Downing, P. A., Griffin, A. S., and Cornwallis, C. K. (2020). Group formation and the evolutionary pathway to complex sociality in birds. Nat. Ecol. Evol. 4, 479–486. doi: 10.1038/s41559-020-1113-x
Dunn, G. P., Bruce, A. T., Ikeda, H., Old, L. J., and Schreiber, R. D. (2002). Cancer immunoediting: from immunosurveillance to tumor escape. Nat. Immunol. 3, 991–998. doi: 10.1038/ni1102-991
Dunn, J. D., Bosmani, C., Barisch, C., Raykov, L., Lefrançois, L. H., Cardenal-Muñoz, E., et al. (2018). Eat prey, live: Dictyostelium discoideum as a model for cell-autonomous defenses. Front. Immunol. 8:1906. doi: 10.3389/fimmu.2017.01906
Dzik, J. M. (2010). The ancestry and cumulative evolution of immune reactions. Acta Biochim. Pol. 57, 443–466. doi: 10.18388/abp.2010_2431
Epstein, B., Jones, M., Hamede, R., Hendricks, S., McCallum, H., Murchison, E. P., et al. (2016). Rapid evolutionary response to a transmissible cancer in Tasmanian devils. Nat. Commun. 7:12684. doi: 10.1038/ncomms12684
Esparza-Mora, M. A., Davis, H. E., Meconcelli, S., Plarre, R., and McMahon, D. P. (2020). Inhibition of a secreted immune molecule interferes with termite social immunity. Front. Ecol. Evol. 8:75. doi: 10.3389/fevo.2020.00075
Evans, J. D., and Spivak, M. (2010). Socialized medicine: individual and communal disease barriers in honey bees. J. Invertebr. Pathol. 103, S62–S72. doi: 10.1016/j.jip.2009.06.019
Extavour, C. G. M. (2007). Evolution of the bilaterian germ line: lineage origin and modulation of specification mechanisms. Integr. Comp. Biol. 47, 770–785. doi: 10.1093/icb/icm027
Eyer, P.-A., Freyer, J., and Aron, S. (2013). Genetic polyethism in the polyandrous desert ant Cataglyphis cursor. Behav. Ecol. 24, 144–151. doi: 10.1093/beheco/ars146
Ezenwa, V. O., Ghai, R. R., McKay, A. F., and Williams, A. E. (2016). Group living and pathogen infection revisited. Curr. Opin. Behav. Sci. 12, 66–72. doi: 10.1016/j.cobeha.2016.09.006
Feng, M., Marjon, K. D., Zhu, F., Weissman-Tsukamoto, R., Levett, A., Sullivan, K., et al. (2018). Programmed cell removal by calreticulin in tissue homeostasis and cancer. Nat. Commun. 9, 1–15. doi: 10.1038/s41467-018-05211-7
Ferguson-Gow, H., Sumner, S., Bourke, A. F. G., and Jones, K. E. (2014). Colony size predicts division of labour in attine ants. Proc. R. Soc. B 281, 20141411. doi: 10.1098/rspb.2014.1411
Fields, C., and Levin, M. (2018). Are planaria individuals? What regenerative biology is telling us about the nature of multicellularity. Evol. Biol. 45, 237–247. doi: 10.1007/s11692-018-9448-9
Fierro-Constaín, L., Schenkelaars, Q., Gazave, E., Haguenauer, A., Rocher, C., Ereskovsky, A., et al. (2017). The conservation of the germline multipotency program, from sponges to vertebrates: a stepping stone to understanding the somatic and germline origins. Genome Biol. Evol. 9:evw289. doi: 10.1093/gbe/evw289
Franzenburg, S., Fraune, S., Kunzel, S., Baines, J. F., Domazet-Loso, T., and Bosch, T. C. G. (2012). MyD88-deficient Hydra reveal an ancient function of TLR signaling in sensing bacterial colonizers. Proc. Natl. Acad. Sci. U.S.A. 109, 19374–19379. doi: 10.1073/pnas.1213110109
Fricke, G. M., Letendre, K. A., Moses, M. E., and Cannon, J. L. (2016). Persistence and adaptation in immunity: T cells balance the extent and thoroughness of search. PLoS Comput. Biol. 12:e1004818. doi: 10.1371/journal.pcbi.1004818
Funayama, N. (2010). The stem cell system in demosponges: insights into the origin of somatic stem cells. Dev. Growth Differ. 52, 1–14. doi: 10.1111/j.1440-169X.2009.01162.x
Gardner, A. (2013). “Adaptation of Individuals and Groups,” in Groups to Individuals: Evolution and Emerging Individuality, eds F. Bouchard and P. Huneman (Cambridge, MA: The MIT Press), 99–116. doi: 10.7551/mitpress/8921.003.0010
Gardner, A., and Grafen, A. (2009). Capturing the superorganism: a formal theory of group adaptation. J. Evol. Biol. 22, 659–671. doi: 10.1111/j.1420-9101.2008.01681.x
Gasch, T., Schott, M., Wehrenfennig, C., Düring, R.-A., and Vilcinskas, A. (2013). Multifunctional weaponry: the chemical defenses of earwigs. J. Insect Physiol. 59, 1186–1193. doi: 10.1016/j.jinsphys.2013.09.006
Godfrey-Smith, P., and Goodnight, C. J. (2013). From Groups to Individuals: Evolution and Emerging Individuality. Cambridge, MA: MIT Press.
Gomez-moracho, T., Heeb, P., and Lihoreau, M. (2017). Effects of parasites and pathogens on bee cognition. Ecol. Entomol. 42, 51–64. doi: 10.1111/een.12434
Gordon, D. M. (2016). From division of labor to the collective behavior of social insects. Behav. Ecol. Sociobiol. 70, 1101–1108. doi: 10.1007/s00265-015-2045-3
Grimsley, C., and Ravichandran, K. S. (2003). Cues for apoptotic cell engulfment: eat-me, don’t eat-me and come-get-me signals. Trends Cell Biol. 13, 648–656. doi: 10.1016/j.tcb.2003.10.004
Hamilton, W. D. (1987). “Kinship, recognition, disease, and intelligence: constraints of social evolution,” in Animal Societies: Theories and Facts, eds Y. Ito, J. Brown, and J. Kikkawa (Tokyo: Japan Science Society Press), 81–102.
Hart, A. G., and Brown, M. J. F. (2002). A colony-level response to disease control in a leaf-cutting ant. Naturwissenschaften 89, 275–277. doi: 10.1007/s00114-002-0316-0
Hart, B. L. (1990). Behavioral adaptations to pathogens and parasites: five strategies. Neurosci. Biobehav. Rev. 14, 273–294. doi: 10.1016/S0149-7634(05)80038-7
Heinze, J., and Walter, B. (2010). Moribund ants leave their nests to die in social isolation. Curr. Biol. 20, 249–252. doi: 10.1016/j.cub.2009.12.031
Hemmrich, G., Miller, D. J., and Bosch, T. C. G. (2007). The evolution of immunity: a low-life perspective. Trends Immunol. 28, 449–454. doi: 10.1016/j.it.2007.08.003
Hernández López, J., Riessberger-Gallé, U., Crailsheim, K., and Schuehly, W. (2017). Cuticular hydrocarbon cues of immune-challenged workers elicit immune activation in honeybee queens. Mol. Ecol. 26, 3062–3073. doi: 10.1111/mec.14086
Herzner, G., Engl, T., and Strohm, E. (2011). Cryptic combat against competing microbes is a costly component of parental care in a digger wasp. Anim. Behav. 82, 321–328. doi: 10.1016/j.anbehav.2011.05.006
Hoback, W. W., Bishop, A. A., Kroemer, J., Scalzitti, J., and Shaffer, J. J. (2004). Differences among antimicrobial properties of carrion beetle secretions reflect phylogeny and ecology. J. Chem. Ecol. 30, 719–729. doi: 10.1023/B:JOEC.0000028427.53141.41
Hoffmann, K., and Korb, J. (2011). Is there conflict over direct reproduction in lower termite colonies? Anim. Behav. 81, 265–274. doi: 10.1016/j.anbehav.2010.10.017
Hofmeyr, S., and Forrest, S. (1999). Immunity by design: an artificial immune system. Proc. 1st Annu. Conf. Genet. Evol. Comput. 2, 1289–1296.
Hogan, C., Dupré-Crochet, S., Norman, M., Kajita, M., Zimmermann, C., Pelling, A. E., et al. (2009). Characterization of the interface between normal and transformed epithelial cells. Nat. Cell Biol. 11, 460–467. doi: 10.1038/ncb1853
Holman, L. (2014). Conditional helping and evolutionary transitions to eusociality and cooperative breeding. Behav. Ecol. 25, 1173–1182. doi: 10.1093/beheco/aru100
Horvath, P., and Barrangou, R. (2010). CRISPR/Cas, the immune system of Bacteria and Archaea. Science 327, 167–170. doi: 10.1126/science.1179555
Hughes, W. O. H., Boomsma, J. J., Url, S., and Hughes, W. H. (2004). Genetic diversity and disease resistance in leaf-cutting ant societies. Evolution 58, 1251–1260. doi: 10.1111/j.0014-3820.2004.tb01704.x
Hughes, W. O. H., Bot, A. N. M., and Boomsma, J. J. (2010). Caste-specific expression of genetic variation in the size of antibiotic-producing glands of leaf-cutting ants. Proc. R. Soc. B 277, 609–615. doi: 10.1098/rspb.2009.1415
Hughes, W. O. H., Oldroyd, B. P., Beekman, M., and Ratnieks, F. L. W. (2008a). Ancestral monogamy shows kin selection is key to the evolution of eusociality. Science 320, 1213–1216. doi: 10.1126/science.1156108
Hughes, W. O. H., Pagliarini, R., Madsen, H. B., Dijkstra, M. B., and Boomsma, J. J. (2008b). Antimicrobial defense shows an abrupt evolutionary transition in the fungus-growing ants. Evolution 62, 1252–1257. doi: 10.1111/j.1558-5646.2008.00347.x
Hughes, W. O. H. H., Eilenberg, J., and Boomsma, J. J. (2002). Trade-offs in group living: transmission and disease resistance in leaf-cutting ants. Proc. R. Soc. B 269, 1811–1819. doi: 10.1098/rspb.2002.2113
Jarvis, J. (1981). Eusociality in a mammal: cooperative breeding in naked mole-rat colonies. Science 212, 571–573. doi: 10.1126/science.7209555
Kaltenpoth, M., Göttler, W., Herzner, G., and Strohm, E. (2005). Symbiotic bacteria protect wasp larvae from fungal infestation. Curr. Biol. 15, 475–479. doi: 10.1016/j.cub.2004.12.084
Kennedy, P., Higginson, A. D., Radford, A. N., and Sumner, S. (2018). Altruism in a volatile world. Nature 555, 359–362. doi: 10.1038/nature25965
Konrad, M., Pull, C. D., Metzler, S., Seif, K., Naderlinger, E., Grasse, A. V., et al. (2018). Ants avoid superinfections by performing risk-adjusted sanitary care. Proc. Natl. Acad. Sci. U.S.A. 115, 2782–2787. doi: 10.1073/pnas.1713501115
Korb, J. (2006). Limited food induces nepotism in drywood termites. Biol. Lett. 2, 364–366. doi: 10.1098/rsbl.2006.0497
Korb, J. (2007). Workers of a drywood termite do not work. Front. Zool. 4:7. doi: 10.1186/1742-9994-4-7
Korb, J., Buschmann, M., Schafberg, S., Liebig, J., and Bagnères, A. G. (2012). Brood care and social evolution in termites. Proc. R. Soc. B 279, 2662–2671. doi: 10.1098/rspb.2011.2639
Korb, J., and Roux, E. A. (2012). Why join a neighbour: fitness consequences of colony fusions in termites. J. Evol. Biol. 25, 2161–2170. doi: 10.1111/j.1420-9101.2012.02617.x
Korb, J., and Schmidinger, S. (2004). Help or disperse? Cooperation in termites influenced by food conditions. Behav. Ecol. Sociobiol. 56, 89–95. doi: 10.1007/s00265-004-0757-x
Korb, J., and Thorne, B. (2017). “Sociality in termites,” in Comparative Social Evolution, eds D. R. Rubenstein and P. Abbot (Cambridge: Cambridge University Press), 124–153. doi: 10.1017/9781107338319.006
Kronauer, D. J. C., Schöning, C., D’Ettorre, P., and Boomsma, J. J. (2010). Colony fusion and worker reproduction after queen loss in army ants. Proc. R. Soc. B 277, 755–763. doi: 10.1098/rspb.2009.1591
Kutzer, M. A. M., and Armitage, S. A. O. (2016). Maximising fitness in the face of parasites: a review of host tolerance. Zoology 119, 281–289. doi: 10.1016/j.zool.2016.05.011
Leclerc, J. B., and Detrain, C. (2017). Loss of attraction for social cues leads to fungal-infected Myrmica rubra ants withdrawing from the nest. Anim. Behav. 129, 133–141. doi: 10.1016/j.anbehav.2017.05.002
Leclerc, J. B., and Detrain, C. (2018). Impact of colony size on survival and sanitary strategies in fungus-infected ant colonies. Behav. Ecol. Sociobiol. 72:3. doi: 10.1007/s00265-017-2415-0
Lemaitre, B., Nicolas, E., Michaut, L., Reichhart, J.-M., and Hoffmann, J. A. (1996). The dorsoventral regulatory gene cassette spätzle/Toll/cactus controls the potent antifungal response in Drosophila adults. Cell 86, 973–983. doi: 10.1016/S0092-8674(00)80172-5
Lenoir, A., Fresneau, D., Errard, C., and Hefetz, A. (1999). “Individuality and colonial identity in ants: the emergence of the social representation concept,” in Information Processing in Social Insects, eds C. Detrain, J. L. Deneubourg, and J. Pasteels (Basel: Birkhäuser Verlag), 219–237. doi: 10.1007/978-3-0348-8739-7_12
Linksvayer, T. A., and Wade, M. J. (2005). The evolutionary origin and elaboration of sociality. Q. Rev. Biol. 80, 317–336. doi: 10.1086/432266
Lo, N., Tokuda, G., Watanabe, H., Rose, H., Slaytor, M., Maekawa, K., et al. (2000). Evidence from multiple gene seqeunces indicates that termites evolved from wood-feeding cockroaches. Curr. Biol. 10, 801–804. doi: 10.1016/S0960-9822(00)00561-3
Loreto, R. G., and Hughes, D. P. (2016). Disease in the society: infectious cadavers result in collapse of ant sub-colonies. PLoS One 11:e0160820. doi: 10.1371/journal.pone.0160820
Lukas, D., and Clutton-Brock, T. H. (2013). The evolution of social monogamy in mammals. Science 341, 526–530. doi: 10.1126/science.1238677
Martin, S. J., Beekman, M., Wossler, T. C., and Ratnieks, F. L. W. (2002). Parasitic cape honeybee workers, Apis mellifera capensis, evade policing. Nature 415, 163–165. doi: 10.1038/415163a
Mersch, D. P., Crespi, A., and Keller, L. (2013). Tracking individuals shows spatial fidelity is a key regulator of ant social organization. Science 340, 1090–1093. doi: 10.1126/science.1234316
Meunier, J. (2015). Social immunity and the evolution of group living in insects. Philos. Trans. R. Soc. B 370, 20140102. doi: 10.1098/rstb.2014.0102
Michod, R. E. (2007). Evolution of individuality during the transition from unicellular to multicellular life. Light Evol. 1, 129–143. doi: 10.17226/11790
Michod, R. E., and Roze, D. (2001). Cooperation and conflict in the evolution of multicellularity. Heredity (Edinb). 86, 1–7. doi: 10.1046/j.1365-2540.2001.00808.x
Milutinović, B., Stock, M., Grasse, A. V., Naderlinger, E., Hilbe, C., and Cremer, S. (2020). Social immunity modulates competition between coinfecting pathogens. Ecol. Lett. 23, 565–574. doi: 10.1111/ele.13458
Moret, Y., and Schmid-Hempel, P. (2000). Survival for immunity: the price of immune system activation for bumblebee workers. Science 290, 1166–1168. doi: 10.1126/science.290.5494.1166
Morgan, E. D. (2008). Chemical sorcery for sociality: exocrine secretions of ants (Hymenoptera: Formicidae). Myrmecol. News 11, 79–90.
Moses, M. E., Cannon, J. L., Gordon, D. M., and Forrest, S. (2019). Distributed adaptive search in T Cells: lessons from ants. Front. Immunol. 10:1357. doi: 10.3389/fimmu.2019.01357
Müller, V., de Boer, R. J., Bonhoeffer, S., and Szathmáry, E. (2018). An evolutionary perspective on the systems of adaptive immunity. Biol. Rev. 93, 505–528. doi: 10.1111/brv.12355
Müller, W. E. G., Koziol, C., Müller, I. M., and Wiens, M. (1999). Towards an understanding of the molecular basis of immune responses in sponges: the marine demosponge Geodia cydonium as a model. Microsc. Res. Tech. 44, 219–236. doi: 10.1002/(sici)1097-0029(19990215)44:4<219::aid-jemt3>3.0.co;2-7
Myles, T. G. (1986). Reproductive soldiers in the Termopsidae (Isoptera). Pan-Pac. Entomol. 62, 293–299.
Myles, T. G. (1999). Review of secondary reproduction in termites (Insecta: Isoptera) with comments on its role in termite ecology and social evolution. Sociobiology 33, 1–43.
Nagata, S., and Tanaka, M. (2017). Programmed cell death and the immune system. Nat. Rev. Immunol. 17, 333–340. doi: 10.1038/nri.2016.153
Nalepa, C. A. (2015). Origin of termite eusociality: trophallaxis integrates the social, nutritional, and microbial environments. Ecol. Entomol. 40, 323–335. doi: 10.1111/een.12197
Natsopoulou, M. E., McMahon, D. P., and Paxton, R. J. (2016). Parasites modulate within-colony activity and accelerate the temporal polyethism schedule of a social insect, the honey bee. Behav. Ecol. Sociobiol. 70, 1019–1031. doi: 10.1007/s00265-015-2019-5
Neumann, P., Pirk, C., Hepburn, H., Solbrig, A., Ratnieks, F., Elzen, P., et al. (2001). Social encapsulation of beetle parasites by Cape honeybee colonies (Apis mellifera capensis Esch.). Naturwissenschaften 88, 214–216. doi: 10.1007/s001140100224
Nishimiya-Fujisawa, C., and Kobayashi, S. (2012). Germline stem cells and sex determination in Hydra. Int. J. Dev. Biol. 56, 499–508. doi: 10.1387/ijdb.123509cf
Nunn, C. L., Jordan, F., McCabe, C. M., Verdolin, J. L., and Fewell, J. H. (2015). Infectious disease and group size: more than just a numbers game. Philos. Trans. R. Soc. B 370:20140111. doi: 10.1098/rstb.2014.0111
Nuotclà, J. A., Biedermann, P. H. W., and Taborsky, M. (2019). Pathogen defence is a potential driver of social evolution in ambrosia beetles. Proc. R. Soc. B 286:20192332. doi: 10.1098/rspb.2019.2332
Otti, O., Tragust, S., and Feldhaar, H. (2014). Unifying external and internal immune defences. Trends Ecol. Evol. 29, 625–634. doi: 10.1016/j.tree.2014.09.002
Page, P., Lin, Z., Buawangpong, N., Zheng, H., Hu, F., Neumann, P., et al. (2016). Social apoptosis in honey bee superorganisms. Sci. Rep. 6:27210. doi: 10.1038/srep27210
Pereira, H., and Detrain, C. (2020). Pathogen avoidance and prey discrimination in ants. R. Soc. Open Sci. 7:191705. doi: 10.1098/rsos.191705
Pie, M. R., Rosengaus, R. B., and Traniello, J. F. A. (2004). Nest architecture, activity pattern, worker density and the dynamics of disease transmission in social insects. J. Theor. Biol. 226, 45–51. doi: 10.1016/j.jtbi.2003.08.002
Piñero, J., and Solé, R. (2019). Statistical physics of liquid brains. Philos. Trans. R. Soc. B 374:20180376. doi: 10.1098/rstb.2018.0376
Pita, L., Hoeppner, M. P., Ribes, M., and Hentschel, U. (2018). Differential expression of immune receptors in two marine sponges upon exposure to microbial-associated molecular patterns. Sci. Rep. 8:16081. doi: 10.1038/s41598-018-34330-w
Poulsen, M., Bot, A., Nielsen, M., and Boomsma, J. (2002). Experimental evidence for the costs and hygienic significance of the antibiotic metapleural gland secretion in leaf-cutting ants. Behav. Ecol. Sociobiol. 52, 151–157. doi: 10.1007/s00265-002-0489-8
Pradeu, T. (2012). The Limits of the Self: Immunology and Biological Identity. Oxord: Oxford University Press.
Pradeu, T. (2013). “Immunity and the emergence of individuality,” in From Groups to Individuals: Evolution and Emerging Individuality, eds F. Bouchard and P. Huneman (Cambridge, MA: MIT Press), 77–96.
Pull, C. D., and Cremer, S. (2017). Co-founding ant queens prevent disease by performing prophylactic undertaking behaviour. BMC Evol. Biol. 17:219. doi: 10.1186/s12862-017-1062-4
Pull, C. D., Metzler, S., Naderlinger, E., and Cremer, S. (2018a). Protection against the lethal side effects of social immunity in ants. Curr. Biol. 28, R1139–R1140. doi: 10.1016/j.cub.2018.08.063
Pull, C. D., Ugelvig, L. V., Wiesenhofer, F., Grasse, A. V., Tragust, S., Schmitt, T., et al. (2018b). Destructive disinfection of infected brood prevents systemic disease spread in ant colonies. eLife 7, 1–29. doi: 10.7554/eLife.32073
Queller, D. C. (2000). Relatedness and the fraternal major transitions. Philos. Trans. R. Soc. B 355, 1647–1655. doi: 10.1098/rstb.2000.0727
Queller, D. C., and Strassmann, J. E. (2009). Beyond society: the evolution of organismality. Philos. Trans. R. Soc. B 364, 3143–3155. doi: 10.1098/rstb.2009.0095
Quevillon, L. E., Hanks, E. M., Bansal, S., and Hughes, D. P. (2015). Social, spatial, and temporal organization in a complex insect society. Sci. Rep. 5:13393. doi: 10.1038/srep13393
Råberg, L., Graham, A. L., and Read, A. F. (2009). Decomposing health: tolerance and resistance to parasites in animals. Philos. Trans. R. Soc. B 364, 37–49. doi: 10.1098/rstb.2008.0184
Radzvilavicius, A. L., Hadjivasiliou, Z., Pomiankowski, A., and Lane, N. (2016). Selection for mitochondrial quality drives evolution of the germline. PLoS Biol. 14:e2000410. doi: 10.1371/journal.pbio.2000410
Ratnieks, F. L. W., and Visscher, P. K. (1989). Worker policing in the honeybee. Nature 342, 796–797. doi: 10.1038/342796a0
Ravichandran, K. S. (2010). Find-me and eat-me signals in apoptotic cell clearance: progress and conundrums. J. Exp. Med. 207, 1807–1817. doi: 10.1084/jem.20101157
Reber, A., Castella, G., Christe, P., and Chapuisat, M. (2008). Experimentally increased group diversity improves disease resistance in an ant species. Ecol. Lett. 11, 682–689. doi: 10.1111/j.1461-0248.2008.01177.x
Reber, A., Purcell, J., Buechel, S. D., Buri, P., and Chapuisat, M. (2011). The expression and impact of antifungal grooming in ants. J. Evol. Biol. 24, 954–964. doi: 10.1111/j.1420-9101.2011.02230.x
Rehan, S. M., Berens, A. J., and Toth, A. L. (2014). At the brink of eusociality: transcriptomic correlates of worker behaviour in a small carpenter bee. BMC Evol. Biol. 14:6. doi: 10.1186/s12862-014-0260-6
Richard, F.-J., Aubert, A., and Grozinger, C. (2008). Modulation of social interactions by immune stimulation in honey bee, Apis mellifera, workers. BMC Biol. 6:50. doi: 10.1186/1741-7007-6-50
Richter, D. J., and Levin, T. C. (2019). The origin and evolution of cell-intrinsic antibacterial defenses in eukaryotes. Curr. Opin. Genet. Dev. 58, 111–122. doi: 10.1016/j.gde.2019.09.002
Rink, J. C. (2013). Stem cell systems and regeneration in planaria. Dev. Genes Evol. 223, 67–84. doi: 10.1007/s00427-012-0426-4
Roisin, Y. (1990). Queen replacement in the termite Microcerotermes papuanus. Entomol. Exp. Appl. 56, 83–90. doi: 10.1111/j.1570-7458.1990.tb01383.x
Roisin, Y. (2000). “Diversity and evolution of caste patterns,” in Termites: Evolution, Sociality, Symbioses, Ecology, eds T. Abe, D. E. Bignell, and M. Higashi (Dordrecht: Springer), 95–119.
Rosengaus, R. B., Jordan, C., Lefebvre, M. L., and Traniello, J. F. A. (1999). Pathogen alarm behavior in a termite: a new form of communication in social insects. Naturwissenschaften 86, 544–548. doi: 10.1007/s001140050672
Rosengaus, R. B., Maxmen, A. B., Coates, L. E., and Traniello, J. F. A. (1998). Disease resistance: A benefit of sociality in the dampwood termite Zootermopsis angusticollis (Isoptera: Termopsidae). Behav. Ecol. Sociobiol. 44, 125–134. doi: 10.1007/s002650050523
Rosengaus, R. B., Mead, K., Du Comb, W. S., Benson, R. W., and Godoy, V. G. (2013). Nest sanitation through defecation: antifungal properties of wood cockroach feces. Naturwissenschaften 100, 1051–1059. doi: 10.1007/s00114-013-1110-x
Rosengaus, R. B., and Traniello, J. F. A. (1993). Temporal polyethism in incipient colonies of the primitive termite Zootermopsis angusticollis: a single multiage caste. J. Insect Behav. 6, 237–252. doi: 10.1007/BF01051507
Rosengaus, R. B., Traniello, J. F. A., and Bulmer, M. S. (2011). “Ecology, behavior and evolution of disease resistance in termites,” in Biology of Termites: A Modern Synthesis, eds D. E. Bignell, Y. Roisin, and N. Lo (New York: Springer), 165–191. doi: 10.1007/978-90-481-3977-4_7
Rosengaus, R. B., Traniello, J. F. A., Lefebvre, M. L., and Maxmen, A. B. (2004). Fungistatic activity of the sternal gland secretion of the dampwood termite Zootermopsis angusticollis. Insectes Soc. 51, 259–264. doi: 10.1007/s00040-004-0749-x
Rosenkranz, P., Aumeier, P., and Ziegelmann, B. (2010). Biology and control of Varroa destructor. J. Invertebr. Pathol. 103, S96–S119. doi: 10.1016/j.jip.2009.07.016
Rothenbuhler, W. C. (1964). Behavior genetics of nest cleaning in honey bees. IV. Responses of F1 and backcross generations to disease-killed brood. Am. Zool. 4, 111–123. doi: 10.1093/icb/4.2.111
Rozen, D. E., Engelmoer, D. J. P., and Smiseth, P. T. (2008). Antimicrobial strategies in burying beetles breeding on carrion. Proc. Natl. Acad. Sci. U.S.A. 105, 17890–17895. doi: 10.1073/pnas.0805403105
Rueppell, O. (2004). From Genes to Societies. Sci. Aging Knowl. Environ. 2004:e5. doi: 10.1126/sageke.2004.5.pe5
Rueppell, O., Hayworth, M. K., and Ross, N. P. (2010). Altruistic self-removal of health-compromised honey bee workers from their hive. J. Evol. Biol. 23, 1538–1546. doi: 10.1111/j.1420-9101.2010.02022.x
Ruiz-Gonzalez, M. X., Moret, Y., and Brown, M. J. F. (2009). Rapid induction of immune density-dependent prophylaxis in adult social insects. Biol. Lett. 5, 781–783. doi: 10.1098/rsbl.2009.0505
Sander, L. M., Warren, C. P., Sokolov, I. M., Simon, C., and Koopman, J. (2002). Percolation on heterogeneous networks as a model for epidemics. Math. Biosci. 180, 293–305. doi: 10.1016/S0025-5564(02)00117-7
Scharf, I., Modlmeier, A. P., Beros, S., and Foitzik, S. (2012). Ant societies buffer individual-level effects of parasite infections. Am. Nat. 180, 671–683. doi: 10.1086/667894
Schmid-Hempel, P., and Crozier, R. H. (1999). Polyandry versus polygyny versus parasites. Philos. Trans. R. Soc. B 354, 507–515. doi: 10.1098/rstb.1999.0401
Scott, M. P. (1998). The ecology and behavior of burying beetles. Annu. Rev. Entomol. 43, 595–618. doi: 10.1146/annurev.ento.43.1.595
Seeley, T., and Tarpy, D. (2007). Queen promiscuity lowers disease within honeybee colonies. Proc. R. Soc. B 274, 67–72. doi: 10.1098/rspb.2006.3702
Shabalina, S., and Koonin, E. (2008). Origins and evolution of eukaryotic RNA interference. Trends Ecol. Evol. 23, 578–587. doi: 10.1016/j.tree.2008.06.005
Shellman-Reeve, J. S. (1997). “The spectrum of eusociality in termites,” in The Evolution of Social Behavior in Insects and Arachnids, eds J. C. Choe and B. J. Crespi (New York, NY: Cambridge University Press), 52–93. doi: 10.1017/cbo9780511721953.005
Shukla, S. P., Plata, C., Reichelt, M., Steiger, S., Heckel, D. G., Kaltenpoth, M., et al. (2018). Microbiome-assisted carrion preservation aids larval development in a burying beetle. Proc. Natl. Acad. Sci. U.S.A. 115, 11274–11279. doi: 10.1073/pnas.1812808115
Simone-Finstrom, M. D., and Spivak, M. (2012). Increased resin collection after parasite challenge: a case of self-medication in honey bees? PLoS One 7:e034601. doi: 10.1371/journal.pone.0034601
Smith, J. M., and Szathmary, E. (1997). The Major Transitions in Evolution. Oxford: Oxford University Press.
Solana, J. (2013). Closing the circle of germline and stem cells: the primordial stem cell hypothesis. Evodevo 4:2. doi: 10.1186/2041-9139-4-2
Stow, A., Briscoe, D., Gillings, M., Holley, M., Smith, S., Leys, R., et al. (2007). Antimicrobial defences increase with sociality in bees. Biol. Lett. 3, 422–424. doi: 10.1098/rsbl.2007.0178
Strassmann, J. E., and Queller, D. C. (2007). Insect societies as divided organisms: the complexities of purpose and cross-purpose. Proc. Natl. Acad. Sci. U.S.A. 104(Supp.l), 8619–8626. doi: 10.1073/pnas.0701285104
Stroeymeyt, N., Casillas-Pérez, B., and Cremer, S. (2014). Organisational immunity in social insects. Curr. Opin. Insect Sci. 5, 1–15. doi: 10.1016/j.cois.2014.09.001
Stroeymeyt, N., Grasse, A. V., Crespi, A., Mersch, D. P., Cremer, S., and Keller, L. (2018). Social network plasticity decreases disease transmission in a eusocial insect. Science 362, 941–945. doi: 10.1126/science.aat4793
Swanson, J. A. I., Torto, B., Kells, S. A., Mesce, K. A., Tumlinson, J. H., and Spivak, M. (2009). Odorants that induce hygienic behavior in honeybees: identification of volatile compounds in chalkbrood-infected honeybee larvae. J. Chem. Ecol. 35, 1108–1116. doi: 10.1007/s10886-009-9683-8
Tarpy, D. D. R. (2003). Genetic diversity within honeybee colonies prevents severe infections and promotes colony growth. Proc. R. Soc. B 270, 99–103. doi: 10.1098/rspb.2002.2199
Teseo, S., Châline, N., Jaisson, P., and Kronauer, D. J. C. (2014). Epistasis between adults and larvae underlies caste fate and fitness in a clonal ant. Nat. Commun. 5:3363. doi: 10.1038/ncomms4363
Teseo, S., Kronauer, D. J. C., Jaisson, P., and Châline, N. (2013). Enforcement of reproductive synchrony via policing in a clonal ant. Curr. Biol. 23, 328–332. doi: 10.1016/j.cub.2013.01.011
Theis, F. J., Ugelvig, L. V., Marr, C., and Cremer, S. (2015). Opposing effects of allogrooming on disease transmission in ant societies. Philos. Trans. R. Soc. B 370:20140108. doi: 10.1098/rstb.2014.0108
Thorne, B. L. (1997). Evolution of eusociality in termites. Annu. Rev. Ecol. Syst. 28, 27–54. doi: 10.1146/annurev.ecolsys.28.1.27
Tragust, S., Mitteregger, B., Barone, V., Konrad, M., Ugelvig, L. V., and Cremer, S. (2013a). Ants disinfect fungus-exposed brood by oral uptake and spread of their poison. Curr. Biol. 23, 76–82. doi: 10.1016/j.cub.2012.11.034
Tragust, S., Ugelvig, L. V., Chapuisat, M., Heinze, J., and Cremer, S. (2013b). Pupal cocoons affect sanitary brood care and limit fungal infections in ant colonies. BMC Evol. Biol. 13:225. doi: 10.1186/1471-2148-13-225
Tranter, C., LeFevre, L., Evison, S. E. F., and Hughes, W. O. H. (2015). Threat detection: contextual recognition and response to parasites by ants. Behav. Ecol. 26, 396–405. doi: 10.1093/beheco/aru203
Trumbo, S. T. (2012). “Patterns of parental care in invertebrates,” in The Evolution of Parental Care, eds N. J. Royle and P. T. Smiseth (Oxford: Oxford University Press), 81–100. doi: 10.1093/acprof:oso/9780199692576.003.0005
Tsuji, K., and Dobata, S. (2011). Social cancer and the biology of the clonal ant Pristomyrmex punctatus (Hymenoptera: Formicidae). Myrmecological News 15, 91–99.
Turnbull, C., Caravan, H., Chapman, T., Nipperess, D., Dennison, S., Schwarz, M., et al. (2012). Antifungal activity in thrips soldiers suggests a dual role for this caste. Biol. Lett. 8, 526–529. doi: 10.1098/rsbl.2012.0184
Ugelvig, L. V., and Cremer, S. (2007). Social prophylaxis: group interaction promotes collective immunity in ant colonies. Curr. Biol. 17, 1967–1971. doi: 10.1016/j.cub.2007.10.029
Ugelvig, L. V., Kronauer, D. J. C., Schrempf, A., Heinze, J., and Cremer, S. (2010). Rapid anti-pathogen response in ant societies relies on high genetic diversity. Proc. R. Soc. B 277, 2821–2828. doi: 10.1098/rspb.2010.0644
Urban, J. L., and Schreiber, H. (1992). Tumor antigens. Annu. Rev. Immunol. 10, 617–644. doi: 10.1146/annurev.iy.10.040192.003153
Van Meyel, S., Körner, M., and Meunier, J. (2018). Social immunity: why we should study its nature, evolution and functions across all social systems. Curr. Opin. Insect Sci. 28, 1–7. doi: 10.1016/j.cois.2018.03.004
Van Oystaeyen, A., Oliveira, R. C., Holman, L., van Zweden, J. S., Romero, C., Oi, C. A., et al. (2014). Conserved class of queen pheromones stops social insect workers from reproducing. Science 343, 287–290. doi: 10.1126/science.1244899
Walker, T. N., and Hughes, W. O. H. (2009). Adaptive social immunity in leaf-cutting ants. Biol. Lett. 5, 446–448. doi: 10.1098/rsbl.2009.0107
West, S. A., Diggle, S. P., Buckling, A., Gardner, A., and Griffin, A. S. (2007). The social lives of microbes. Annu. Rev. Ecol. Evol. Syst. 38, 53–77. doi: 10.1146/annurev.ecolsys.38.091206.095740
West, S. A., Fisher, R. M., Gardner, A., and Toby Kiers, E. (2015). Major evolutionary transitions in individuality. Proc. Natl. Acad. Sci. U.S.A. 112, 10112–10119. doi: 10.1073/pnas.1421402112
Wheeler, W. M. (1911). The ant-colony as an organism. J. Morphol. 22, 307–325. doi: 10.1002/jmor.1050220206
Wiens, M., Korzhev, M., Perovic-Ottstadt, S., Luthringer, B., Brandt, D., Klein, S., et al. (2006). Toll-like receptors are part of the innate immune defense system of sponges (Demospongiae: Porifera). Mol. Biol. Evol. 24, 792–804. doi: 10.1093/molbev/msl208
Wilson, E. O. (1971). The Insect Societies. Cambridge: The Belknap Press of Harvard Univeristy Press.
Wilson, K., Knell, R., Boots, M., and Koch-Osborne, J. (2003). Group living and investment in immune defence: an interspecific analysis. J. Anim. Ecol. 72, 133–143. doi: 10.1046/j.1365-2656.2003.00680.x
Keywords: superorganism, disease, social evolution, major evolutionary transition, social immunity
Citation: Pull CD and McMahon DP (2020) Superorganism Immunity: A Major Transition in Immune System Evolution. Front. Ecol. Evol. 8:186. doi: 10.3389/fevo.2020.00186
Received: 08 January 2020; Accepted: 25 May 2020;
Published: 24 June 2020.
Edited by:
Heikki Helanterä, University of Oulu, FinlandReviewed by:
Patrick Kennedy, University of Bristol, United KingdomSheena Cotter, University of Lincoln, United Kingdom
Copyright © 2020 Pull and McMahon. This is an open-access article distributed under the terms of the Creative Commons Attribution License (CC BY). The use, distribution or reproduction in other forums is permitted, provided the original author(s) and the copyright owner(s) are credited and that the original publication in this journal is cited, in accordance with accepted academic practice. No use, distribution or reproduction is permitted which does not comply with these terms.
*Correspondence: Christopher D. Pull, Y2hyaXMucHVsbEBnbWFpbC5jb20=; Dino P. McMahon, ZGluby1wZXRlci5tY21haG9uQGJhbS5kZQ==