- 1Shanghai Minhang High School, Shanghai, China
- 2Plant Gene Expression Center, USDA-ARS, Albany, CA, United States
- 3Department of Plant and Microbial Biology, University of California, Berkeley, Berkeley, CA, United States
- 4Department of Soil, Water and Environmental Science, University of Arizona, Tucson, AZ, United States
- 5Key Laboratory of Genetics and Germplasm Innovation of Tropical Special Forest Trees and Ornamental Plants, Ministry of Education, College of Forestry, Hainan University, Haikou, China
- 6Key Laboratory for Earth Surface Processes of the Ministry of Education, Department of Ecology, College of Urban and Environmental Sciences, Peking University, Beijing, China
- 7Key Laboratory of Vegetation Restoration and Management of Degraded Ecosystems, South China Botanical Garden, Chinese Academy of Sciences, Guangzhou, China
- 8Guangdong Provincial Key Laboratory of Applied Botany, South China Botanical Garden, Chinese Academy of Sciences, Guangzhou, China
- 9Center for Plant Ecology, Core Botanical Garden, Chinese Academy of Sciences, Guangzhou, China
Reproductive phenology is sensitive to climatic changes and is associated with species functional types, distribution ranges, and their corresponding climatic niches. Phylogenetic niche conservatism in reproductive phenology also constrains its diversity and the distribution of species. Therefore, we assessed the effects of photosynthetic pathway, life history, phylogeny, and climatic niche on reproductive phenology. For 190 Poaceae species in subtropical China, we compiled data on flowering onset and reproductive period, functional type (photosynthetic pathway and life history), and 18 climatic variables across the species’ global distributions and used phylogenetic models to determine associations. We found strong phylogenetic signals in flowering onset but not in reproductive period. Photosynthetic pathway and life history have significant interactive effects on both flowering onset and reproductive period, such that C3 annual grasses flowered the earliest and had the longest reproductive period. We found that species with wider climatic niches would flower earlier and have longer reproductive periods. Specifically, species that experience wider ranges of mean annual precipitation and coldest-month temperatures would flower earlier, and species with higher mean annual temperature and wider ranges of wettest-quarter precipitation have a longer reproductive period. This study finds that the diversity of reproductive phenology among subtropical grasses is constrained by evolution and climatic niche and that photosynthetic pathway and life history have an interactive effect on the timing and the duration of reproduction.
Introduction
Reproductive phenology is key to the success of individual plant reproduction and population persistence (Rathcke and Lacey, 1985; Elzinga et al., 2007; Craine et al., 2012a). Differences in reproductive phenology among co-occurring species minimize direct competition and are often important in maintaining high species diversity in communities (Craine et al., 2012b). However, the reproductive phenology of species is not fixed and may be sensitive to changes in climate (Anderson et al., 2012). Flowering onset and duration, for example, are determined by a network of signals including day length (Colasanti and Coneva, 2009), light quality (Cerdán and Chory, 2003), temperature (Fitter and Fitter, 2002; Colasanti and Coneva, 2009), atmospheric CO2 concentration (Springer and Ward, 2007), and drought (Franks, 2011). In temperate grasses, vernalization, or a prolonged exposure to cold temperature, is required to induce the expression of the FLOWERING LOCUS T (FT) gene, a key gene in flower development (Corbesier et al., 2007; Tamaki et al., 2007; Lv et al., 2014). Besides environmental factors, recent studies have found that the reproductive phenology of species is also influenced by life history, photosynthetic pathway, and phylogeny (Davies et al., 2013; Du et al., 2015; Cortés-Flores et al., 2017; Munson and Long, 2017). Despite these various findings, there has not yet been a systematic study on how reproductive phenology is shaped by plant functional types, phylogeny, and climatic niches.
As the fifth largest plant family comprising ∼12,000 species, grasses (members of the Poaceae family) are an essential food source for many species, including our own, and thus any potential phenological shifts under climatic change might have huge ecological and economic consequences (Munson and Long, 2017). Phylogenetic niche conservatism (PNC) is the tendency of closely related species to share similar niches and traits (Wiens, 2004; Wiens and Graham, 2005) and has been found in flowering onset in many species from different families (Davies et al., 2013; Du et al., 2015; Li et al., 2016). Although the phenology of grasses has been studied in a small number of crops and dominant grassland species (Craine et al., 2012a; Li et al., 2016), studies that encompass the great diversity of grass species are still lacking. This may be partly due to difficulties of long-term phenological surveys on many species or distinctions between flower and fruit stages for grasses in the field (Primack and Gallinat, 2017). However, since PNC in flowering phenology has been widely found and a number of grass morphological and physiological traits show PNC (Liu et al., 2012; Liu and Osborne, 2015), we also expect to find PNC in the reproductive phenology of grasses.
Divergent structural and physiological traits among C3 and C4 grasses (Edwards et al., 2010; Sage et al., 2012; Taylor et al., 2014; Lundgren et al., 2019) may lead to differences in reproductive phenology. In grasses, C4 species have higher photosynthetic rates, greater water use efficiencies, and faster growth under warm and dry conditions than their C3 counterparts (Ehleringer, 1978; Way et al., 2014; Atkinson et al., 2016). Therefore, where C3 and C4 grasses coexist, C3 grasses might flower earlier to gain an initial reproductive advantage before intense competition with C4 grasses for space and other resources begins. In addition, the reproductive duration of C3 grasses might be longer than that of C4 grasses because C4 grasses are adapted to warmer regions where the warm climate delays the flowering onset and shortens the duration (Sherry et al., 2011). Using herbarium records of 16 grass species, a recent study found that C3 grasses indeed start to flower earlier than C4 grasses (Munson and Long, 2017). However, whether this pattern in reproductive phenology between C3 and C4 grasses is widespread has not been tested.
Life history may be another important trait in determining phenology due to fundamental differences in resource allocation strategies across life history functional types (Lundgren and Des Marais, 2020). While annuals tend to flower once in a single growing season and die, biennials and triennials flower in their 2nd and 3rd years, respectively. In contrast, perennials persist over multiple growing seasons but may have multiple flowering periods per year (iteroparity) or flower once over multiple years (semelparity; Lundgren and Des Marais, 2020). Therefore, to ensure that their sole reproductive effort is successful and to limit competition with established perennials, annuals may flower earlier and longer than perennials. Munson and Long (2017) found that annual grasses flowered earlier than perennial grasses in a sample of 16 species, but only in regions with a higher mean annual temperature. Given that, across subtropical grasses, life history influences the diversity of several functional traits (Liu et al., 2019b), we expected that reproductive phenology would also differ between annual and perennial grasses. Therefore, as multiple environmental and functional factors will affect the reproductive phenology of grasses, more species across multiple ecosystems need to be tested to determine whether photosynthetic pathway and life history have interactive effects on grass reproductive phenology and whether the patterns depend on phylogenetic background.
Climatic niches depict where species occur over space and time and reflect their adaptive strategies (Soberón, 2007; MacLean and Beissinger, 2017). Across species, variation in climatic niche is closely related to variation in functional traits (Thuiller et al., 2004). Species with wide geographic ranges are expected to have higher adaptability and are more able to change their traits to better fit their environment than species with narrow ranges (Lavergne et al., 2004; Slatyer et al., 2013). In terms of reproductive phenology, species with larger ranges are predicted to be more able to alter the timing of reproduction in response to changes in the local environments than narrow-ranging species. For example, flowering onset is likely to be related to climatic conditions, especially temperature (Fitter and Fitter, 2002). Therefore, in climate change scenarios, knowledge of the relationships between reproductive phenology and their climatic niches is urgently needed for predicting the survival and the distribution of species.
Previous phylogenetic studies on flowering phenology have included species across multiple families (Munguía-Rosas et al., 2011; Du et al., 2015), but few have focused on many species within one family, which can assess phylogenetic effects at a more detailed scale (Staggemeier et al., 2010). Furthermore, tropical, and subtropical regions contain over half of the herbaceous species in Poaceae (except Bambusoideae and Pooideae; Watson et al., 1992; Osborne et al., 2014). Grass species in subtropical China are diverse in life history and photosynthetic pathway and have been well surveyed and studied, providing an ideal basis for further study (Liu et al., 2019b). Here we use data on reproductive phenology, photosynthetic pathway, life history, phylogeny, and global climatic niche for 190 subtropical grasses in order to test the following hypotheses while controlling for phylogenetic effects: (1) There are strong phylogenetic signals in two reproductive phenological traits (flowering onset and reproductive period); (2) C3 grasses flower earlier and have longer reproductive periods than C4 grasses, and similarly, annuals flower earlier and have longer reproductive periods than perennials. Photosynthetic pathway and life history may have interactive effects on flowering onset and reproductive period; and (3) Species with wider global climatic niches might flower earlier and have longer reproductive periods, with some key climatic niche variables playing more important roles than others, for example, coldest-month temperature may be more important than mean annual temperature.
Materials and Methods
Species Sampling and Reproductive Phenology
Subtropical China has a typical East Asian monsoon climate. Its central region, Guangdong Province, has a mean annual temperature of 21.2°C (13.6°C in January and 28.9°C in July) and a mean annual precipitation of ∼1,700 mm, of which 80% occurs in the wet season from April to September.
There are 316 herbaceous Poaceae species in the native flora of subtropical China, according to the “Flora of Guangdong” (South China Botanical Garden, 2009). Here we only considered herbaceous grasses and so excluded the 153 species of woody bamboos in this region to keep the same life form. We assigned photosynthetic pathway (C3 or C4 species) for each species based on published literature (Grass Phylogeny Working Group, 2012; Osborne et al., 2014), as well as life history (annuals or perennials) and reproductive phenology from the flora for 290 species (South China Botanical Garden, 2009). Of these species, 9.5% are C3 annuals, 14.9% are C3 perennials, 26.6% are C4-annuals, and 49.8% are C4 perennials. Furthermore, after matching species with global occurrence and phylogenetic information, we had a final sample of 190 species, which constituted 60.1% of the total native Poaceae species in subtropical China. This sample also well represented the floral pool, with 10.0% C3 annuals, 12.1% C3 perennials, 27.9% C4 annuals, and 50.0% C4 perennials (Supplementary Table S1).
Following previous studies on phenology using monthly data (Hart et al., 2016), we assigned the initial flowering month (e.g., May is recorded as 5) as “flowering onset” and calculated “reproductive period” as the difference between “flowering onset” and flowering and fruiting end month. For the 11 species whose reproductive period ends in the following year, we calculated the period directly (e.g., for Heteropogon triticeus, which flowers and fruits from October until March, the flowering onset is 10 and the reproductive period is 5). This method not only enabled the correct calculation of reproductive period but also avoided the circular problem of underestimating phylogenetic signals in plant phenology (Staggemeier et al., 2019).
Climatic Data and Climatic Niche Calculation
For climatic data, we obtained geo-referenced locality data for each species from the Global Biodiversity Information Facility1, using the R statistical computing environment v. 3.5.1 (R Core Team, 2018), and the occ_download function in the rgbif package (Chamberlain et al., 2014). We carefully checked each species’ occurrence data to ensure that the database is representative of the distributions of the grasses (Supplementary Figure S1). Next, we extracted the climatic data for all occurrence points from the WorldClim database (Hijmans et al., 2005), using the function extract in the R package raster (Hijmans et al., 2015). The WorldClim dataset has climatic data at 1-km2 resolution for 1950–2000. We included nine climatic variables to calculate climatic niches: mean annual temperature (MAT, bio1), temperature seasonality (Ts, bio4, standard deviation across 12 monthly temperature values), maximum temperature of the warmest month (Tmax, bio5), minimum temperature of the coldest month (Tmin, bio6), mean annual precipitation (MAP, bio12), precipitation seasonality (Ps, bio15, coefficient of variation across 12 monthly precipitation values), precipitation of the wettest and the driest quarters (Pmax and Pmin, bio16, and bio17, respectively), and mean annual solar radiation (sr). We did not use precipitation of the wettest and the driest months because the monthly precipitation values have high inter-annual fluctuations, and thus the precipitation values based on quarters are more biologically meaningful for plants. Across the 190 species, the sample size of occurrence data per species ranged from 1 to 170,647 (mean = 2,612).
We estimated climatic niches for each species using climatic variable values extracted from localities across its geographic range (Quintero and Wiens, 2013). The mean and range (maximum – minimum) of the nine climatic variables were calculated for each species, using mean, max, and min functions in R, giving a total of 18 indices (9 × 2) to define the species’ climatic niches (Supplementary Table S1). The indices related to temperature, precipitation, and solar radiation can set the species’ climatic range limits and potential climatic tolerances.
Phylogenetic Tree
A phylogenetic tree of the 190 study species was constructed by integrating published phylogenies based on chloroplast genes (Supplementary Figure S2). We first extracted 146 species from a Poaceae super tree ∼3,000 species (Edwards et al., 2010) after checking synonymies based on the GrassBase-Synonymy database2. We then searched published phylogenies for different genera and identified 27 more species as congeners in the super tree. Finally, we assembled the last 17 species as polytomies into the 190-species tree using the function bind.tree in the R package ape (Paradis et al., 2004). When we focused on phylogenetic relationships rather than the exact divergence times among species, polytomies and missing branch length information only had negligible impacts on the phylogenetic signals calculated using phylogenetic generalized least square (PGLS; Münkemüller et al., 2012). Therefore, we transformed the branch lengths of the phylogenetic tree using Grafen’s method to avoid the effects of setting dichotomies/polytomies (Grafen, 1989).
Data Analysis
To determine if there are phylogenetic signals in reproductive phenology traits, we estimated Pagel’s λ. This indicates the degree to which residual trait variation shows similarity based on phylogenetic relationships across species (Pagel, 1999). The λ values vary between 0 and 1 in which, λ = 0 indicates no phylogenetic signal and thus this trait is independent of phylogeny. In contrast, λ = 1 implies that the distribution of trait values across the phylogeny is as expected under the Brownian model of trait evolution (i.e., trait variation completely depends on phylogeny). We estimated λ for both flowering onset and reproductive period using PGLS models, using the function pgls in the R package caper (Orme and Freckleton, 2018).
To establish how reproductive phenology differed with photosynthetic type (PT) and life history (AP, annuals and perennials) and whether interactive effects existed between PT and AP, we also constructed PGLS models. PGLS performs well irrespective of the intensity of the phylogenetic signal, which is ideal for comparisons across large numbers of traits differing in their associations with phylogeny (Revell, 2010). We compared four alternative models (y ∼ PT × AP, y ∼ PT + AP, y ∼ PT, and y ∼ AP), where the response variable was either flowering onset or reproductive period, and selected the best-fitted model that had the lowest Akaike information criterion (AIC) scores (Anderson, 2007).
How reproductive phenology related to the 18 climatic niches (nine means and ranges of MAT, Ts, Tmax, Tmin, MAP, Ps, Pmax, Pmin, and sr) was analyzed using stepwise regression based on PGLS. As many factors in a stepwise regression will give redundant factors in the selected best model, we constructed four potential models and compared them. We first built a full model (M1, with all climatic variable means and ranges as factors), from which we obtained its best-fitted model (M1best) based on stepwise regressions by using function step in R. Next, we built a mean-value-based model (using the nine mean variables as factors) and obtained its corresponding best-fitted model M2. Similarly, a range-value-based model (nine range variables as factors) produced its corresponding best-fitted model M3. Then, we combined significant factors from M1best, M2, and M3 to construct a more comprehensive model (M4). The interactive factor PT × AP was added in M1 to M4 according to the results of the abovementioned analysis. We did not add interactive factors among climatic niches because similar previous studies had already tested and treated them as additive factors (Atkin et al., 2015). We then compared the four models to find the best-fitted model based on AIC scores and reported percentages of variation explained by each factor. Finally, we analyzed the relationships between significant climatic factors and reproductive phenology traits using standardized major axis (SMA) analysis as well as PGLS to determine the role that phylogeny plays on these relationships. In order to test the interactive effects (PT × AP) on the relationships, we ran SMAs for all data and the four PT-AP groups (C3 annuals, C3 perennials, C4 annuals, and C4-perennials) separately. SMA analysis was performed using sma function in the R package smatr (Warton et al., 2012).
Results
Phylogenetic Signal in Reproductive Phenology Traits
Flowering onset showed a significant phylogenetic signal (λ = 0.50, P < 0.001 for λ = 0 or 1), whereas reproductive period had no phylogenetic signal (λ = 0.00 with P = 1.000 for λ = 0; Table 1A).
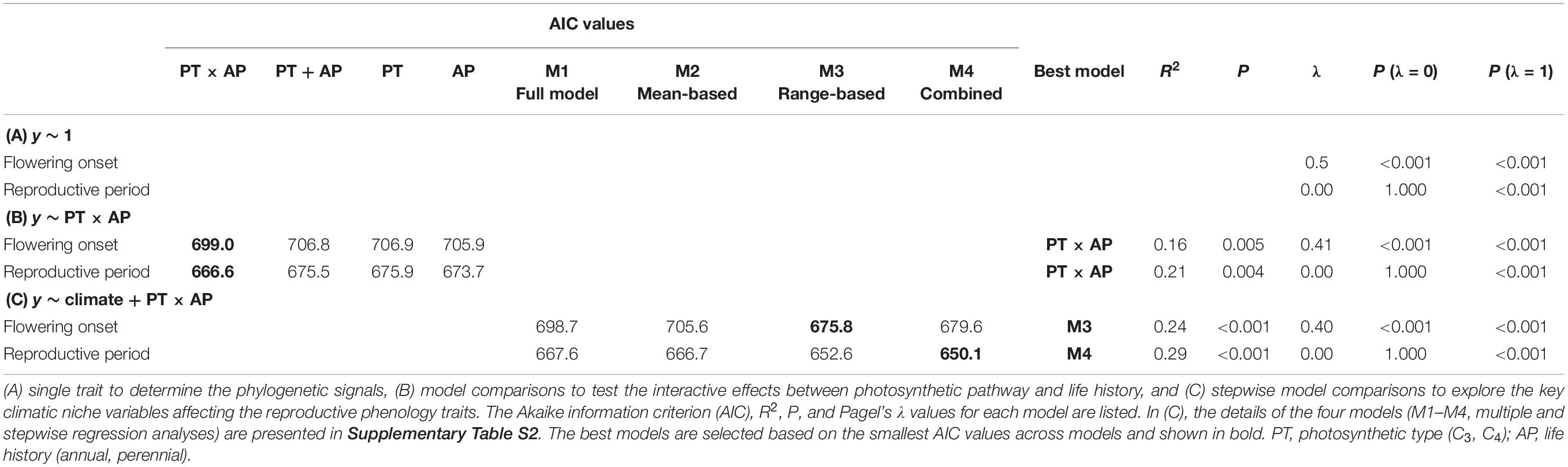
Table 1. Phylogenetic generalized least square models on the reproductive phenology traits of 190 grass species.
Reproductive Phenology and Climatic Niches Among Photosynthetic and Life History Types
The 190 grass species belonged to five C3 and four C4 independent lineages across the phylogenetic tree, while annual and perennial species were dispersed randomly across the tree (Supplementary Figure S2). C3 annual grasses started to flower one month earlier than C3 perennials, C4 annuals, and C4 perennials (Figure 1A). C3 annuals also showed the longest reproductive period and C3 perennials the shortest (Figure 1B). Further PGLS model comparisons showed that photosynthetic pathway (PT) and life history type (AP) had significant interactive effects on both flowering onset and reproductive period. y ∼ PT × AP was selected as the best-fitted model (Table 1B). The interaction PT × AP explained 70.1 and 71.1% of total variation of flowering onset and reproductive period, respectively, far more than single factors PT and AP explained (Table 2A).
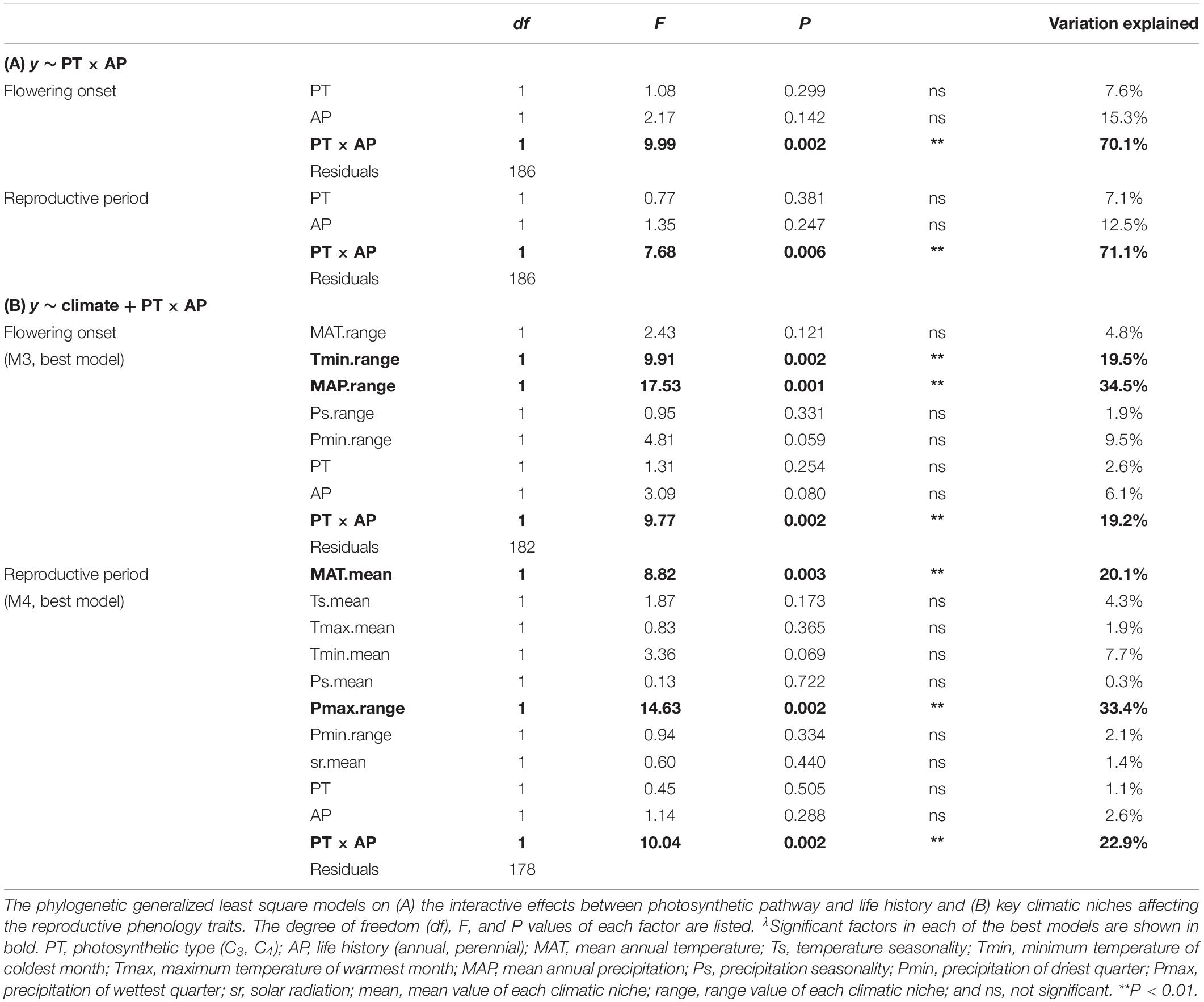
Table 2. Factors and their explanatory powers in each of the best-fitting models on the reproductive phenology traits of 190 grass species.
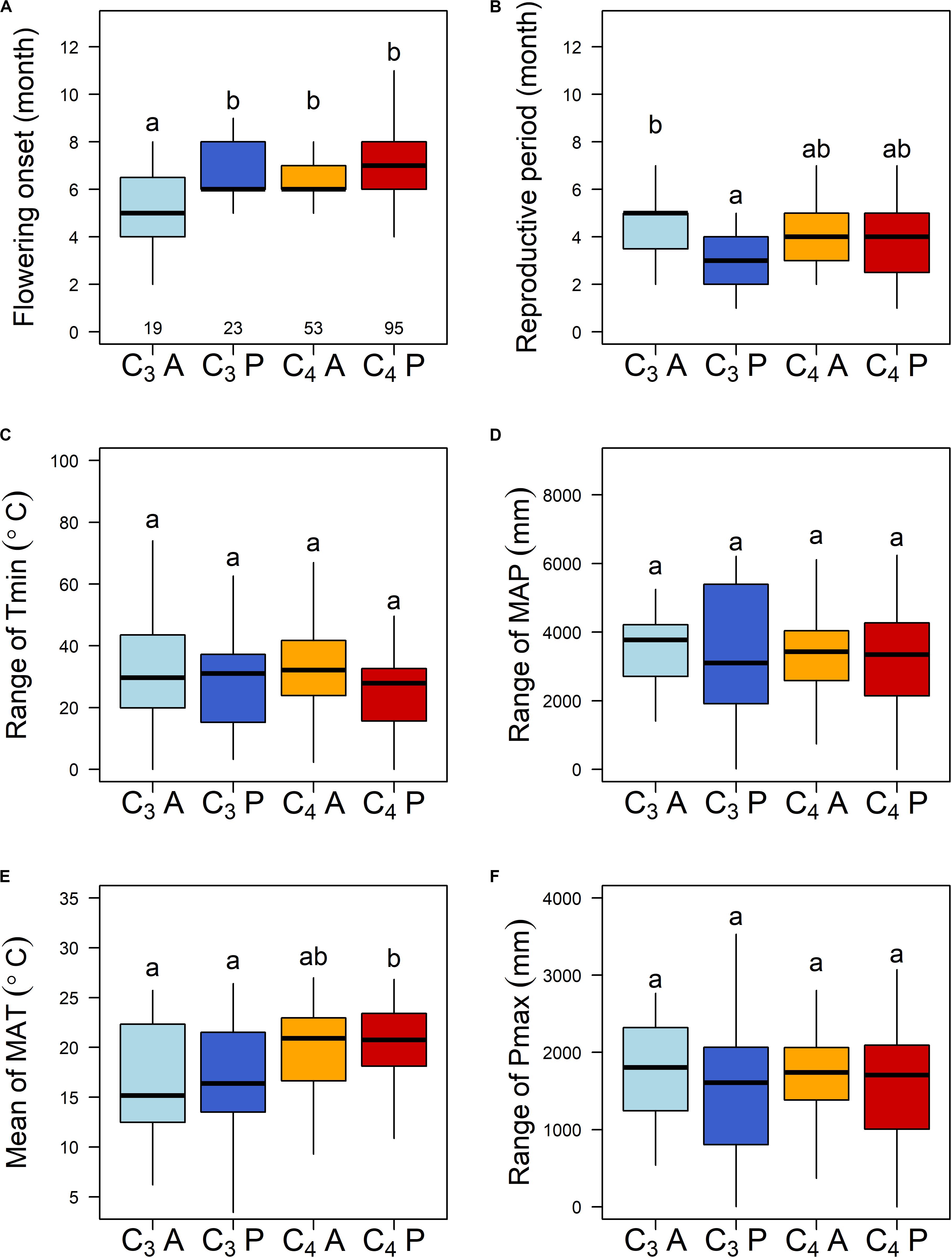
Figure 1. (A,B) Reproductive phenology traits and key climatic niches of different photosynthetic pathways and life history types of the 190 subtropical grasses. C3A, C3 annuals; C3P, C3 perennials; C4A, C4 annuals; and C4P, C4 perennials. The sample sizes for each group are 19, 23, 53, and 95, respectively. Different letters indicate statistically significant differences. (C–F) Mean and range are the mean and the range values across localities of each species. Tmin, minimum temperature of the coldest month; MAP, mean annual precipitation; MAT, mean annual temperature; and Pmax, precipitation of the wettest quarter.
For climatic niches, C3 annuals, and C3 perennials occurred in localities with the lowest MAT, while C4 perennials occurred in the highest, based on multiple comparisons (Figure 1E). The other three key climatic niche variables (Tmin range, MAP range, and Pmax range) did not differ among the four groups (Figures 1C,D,F).
Relationships Between Reproductive Phenology Traits and Climatic Niches
Phylogenetic generalized least square stepwise model comparison on the relationships between flowering onset and climatic niches selected the range-based model (M3) as the one with the best fit (Table 1C). Further analyses using this model found that, among multiple factors, Tmin range, MAP range, and PT × AP were the three significant factors that explained 19.5, 34.5, and 19.2% of the total variation in flowering onset, respectively (Table 2B). Separate bivariate relationships showed that flowering onset was significantly earlier with both increasing Tmin range and MAP range (Figures 2A,B). However, the relationships were driven by C4 perennials because the models on the other three groups were not significant (Figures 2A,B and Supplementary Table S3A).
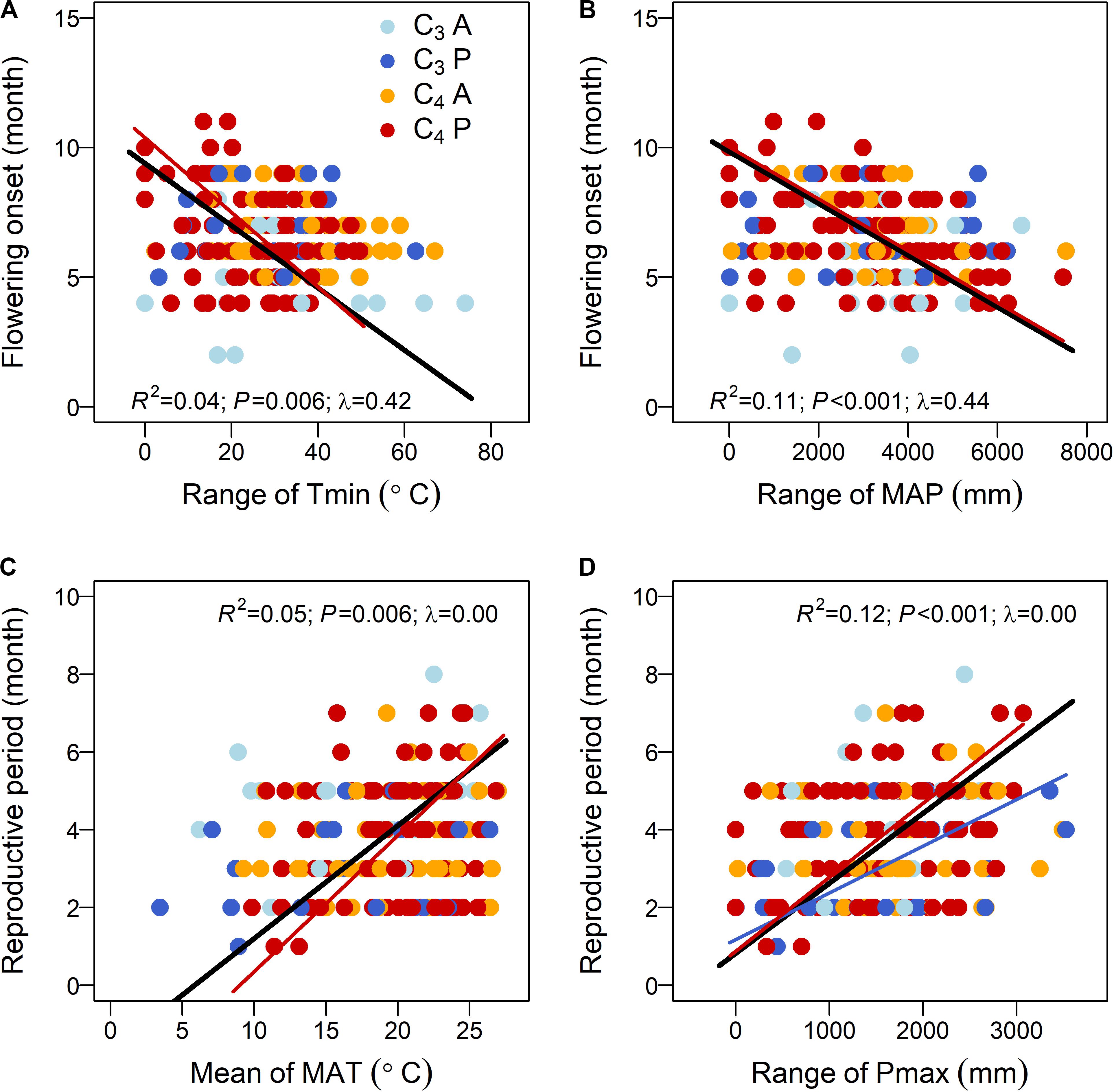
Figure 2. Relationships between (A,B) flowering onset and (C,D) reproductive period and key climatic niche variables of the 190 subtropical grasses. Points are colored by photosynthetic pathway and life history (C3A, C3 annuals—blue; C3P, C3 perennials—dark blue; C4A, C4 annuals—orange; and C4P, C4 perennials—red). The models are tested for all data and four groups separately, but only the lines of significant models are plotted. The R2, P, and Pagel’s λ values for the model of all data are listed, while the coefficients for the other models are presented in Supplementary Table S3. The four key climatic niche variables are significant factors of the best-fitted models in Table 2.
For the relationship between reproductive period and climatic niche, the best-fitted model (M4) included both mean and range values of climatic niche variables (Table 1C). Specifically, the significant factors of the best-fitted model were MAT mean, Pmax range, and PT × AP, which explained 20.1, 33.4, and 22.9% of the total variation of reproductive period, respectively (Table 2B). Bivariate relationships exhibited that reproductive period was significantly longer with increasing MAT mean and Pmax range (Figures 2C,D). The relationship based on MAT mean was driven by C4 perennials, while the relationship of Pmax range was significant for both C3 and C4 perennials (Figures 2C,D and Supplementary Table S3B).
Discussion
In this study, strong phylogenetic signals in flowering onset, but not reproductive period of grasses indicate the importance of phylogeny in comparative studies on phenology. Significant interactive effects between photosynthetic pathway and life history on reproductive phenology reflect different adaptive strategies. Climatic niche affects reproductive phenology, with species with wider climatic niches flowering earlier and having a longer reproductive period than those with narrower climatic niches.
Phylogenetic Signals in Reproductive Phenology Traits
The high phylogenetic dependence of flowering onset of grasses (λ = 0.50) was consistent with previous studies that strong phylogenetic signals existed in flowering time across 19,631 species in China (Du et al., 2015) and in flowering onset of 62 meadow species (Li et al., 2016). Generally, it was challenging to detect phylogenetic signals within one family due to stronger PNC across families than within families (Losos, 2008), but our data illustrated clear phylogenetic patterns in reproductive phenology within the Poaceae family. Our results also suggested that during the adaptation and evolution processes of grasses, flowering onset was a key trait under stabilizing selection and was therefore phylogenetically conserved (Donoghue, 2008; Anderson et al., 2012), compared with reproductive period. A potential reason was that flowering induction and onset in response to environmental stimuli, such as vernalization, is an elaborate process that involves many molecular regulations and resource investments (Lv et al., 2014; Xu et al., 2019), which tended to be fixed once evolved as an economical strategy. Reproductive period, on the other hand, can be more labile and vary with environmental changes (de Xavier et al., 2019). Environmental stresses that may occur during the reproductive period means that growth and resource allocation to reproduction needs to be more flexible than just initiating flowering in order to ensure survival.
Furthermore, the phylogenetic signals in flowering onset indicated that non-phylogenetic methods were not appropriate due to statistical independence (Revell, 2010), as all the effects of life history, photosynthetic pathway, and climatic niches on flowering onset were significantly related to phylogeny (Table 1). Therefore, we suggest that phylogenetic relationships should be considered when searching for ecological drivers of reproductive phenology in comparative analyses (Davies et al., 2013; Li et al., 2016).
Reproductive Phenology Traits Among Photosynthetic and Life History Types Reflect Their Adaptive Strategies
Due to the interactive effect between photosynthetic pathway (PT) and life history (AP), only C3 annual grasses, not C3 perennials, initiated flowering earlier than C4 grasses (Figure 1A). This result agreed with previous findings that C3 annual grasses started flowering the earliest in western United States (Munson and Long, 2017). C3 grasses may flower earlier and reproduce longer to compensate for growth disadvantages in competing for space and resources with C4 grasses (Atkinson et al., 2016). However, in our data, C3 grasses did flower earlier than C4 grasses, but only within annual species. Most C4 grasses, which were late flowering regardless of life history, are in tropical/subtropical areas where the evolution of responses to cold/day length in early spring is not as necessary as for the C3 annuals inhabiting temperate regions (Edwards et al., 2010). Our results also agreed with previous findings that annuals flower earlier than congeneric perennials in a long-term observational study of 385 plants (Fitter and Fitter, 2002). Thus, the interactive effect (PT × AP) likely arose from perennials because they had more flexibility in flowering onset and reproductive period during multiple growing seasons (Lundgren and Des Marais, 2020).
Our hypothesis that reproductive period would be associated with either PT or AP was not supported due to the interactive effect (PT × AP), with C3 annuals and C3 perennials showing the longest and the shortest reproductive period, respectively (Figure 1B). One reason for the similar reproductive period between C3 and C4 grasses might be their relatively narrow local climatic ranges in the study region. The local winter temperature (Dec–Mar) was 5–15°C, while vernalization requires 0–5°C for 15–30 days. Therefore, some C3 grasses originally from temperate regions (Figure 1E) may have lost their coldness stimulation and initiated flowering just as late as C4 grasses (Munson and Long, 2017), diminishing the differences between the two. We did not find any literature that directly compared the reproductive period of C3 and C4 plants, so it is possible that C3 and C4 grasses have similar reproductive lengths. Our observation on C3 wheat and C4 corn, which both have a reproductive period of 1 to 2 months, supports this. Another possible reason for a longer reproductive period in annuals than in perennials within C3 species only is that the vegetative growth and the reproduction of annuals occur simultaneously. This might prolong the reproductive period in annuals, while the two stages are more distinct and flexible in perennials (Albani and Coupland, 2010). A longer reproductive period of annuals was consistent with the findings from 302 C3 tropical tree species (Bawa et al., 2003).
Early flowering is an important strategy to occupy the cold early spring niches, particularly in temperate species (Munguía-Rosas et al., 2011). With a mean winter temperature of 5–15°C, the subtropical species studied here have rarely experienced a cold early spring; in contrast, the mean temperature of cold winter was at least below 5°C in the Western United States (Munson and Long, 2017). Thus, the early flowering of C3 annuals should be an inherited trait. Indeed six out of nine C3 annual species that flowered earlier than May were from the subfamily Pooideae. Members of this group are reported to flower earlier than those from other Poaceae subfamilies (Liu et al., 2019a) and are mainly distributed in temperate regions (Edwards and Smith, 2010) and acclimated to cold stress (Schubert et al., 2019). Therefore, our results reflected different ecological adaptation strategies of different photosynthetic pathways, life histories, and phylogenetic origins in grass species. Consistent with the patterns that we previously found in morphological and physiological traits (Liu et al., 2019b), life history was more important than photosynthetic pathway in explaining differences in both flowering onset and reproductive period among subtropical grasses.
The Four Climatic Niches Affected Reproductive Phenology After Considering Phylogeny
Our hypothesis that species with wider global climatic niches might flower earlier and have a longer reproductive period, based on geographical range theories (Lavergne et al., 2004; Thuiller et al., 2004), was supported by four key climatic niche variables. Specifically, species with wider ranges of Tmin and MAP flowered earlier, and species with higher mean MAT and a wider range of Pmax had longer reproductive periods. These relationships were mainly driven by C4 perennials (Figure 2). After accounting for phylogenetic relationships, the effect size of models based on climatic niches was small (even for the best multiple-factor models, R2 ranged from 0.16 to 0.29; Table 1). However, the four significant climatic niches still have important ecological implications.
The minimum temperature of the coldest month (Tmin) was a better predictor of flowering onset than other temperature variables, especially in representing the breadth of temperature niche (i.e., range). Clearly, Tmin could capture the coldness in early spring for flowering induction better than MAT. Previous studies have reported that grass species flowered earlier under lower temperatures (Evans, 1971; Fitter and Fitter, 2002; Sherry et al., 2011); however, we did not find this using our phylogenetic models. A possible reason for the contrasting results is that previous studies have matched phenological records with local temperatures, but our data were sampled across each species’ global distribution (climatic niches). This partially explained the small effect sizes of all the PGLS models and revealed that, compared with local temperature as the physiological driver, the range of Tmin was the ecological driver of flowering onset.
Species spanning a wider range of precipitation tended to flower earlier. When species undergo drought, their flowering onset tends to be delayed (Craine et al., 2012a). Species of high-precipitation regions (>2,000 mm, subtropical and tropical areas; Figure 1D) tended to extend their reproductive period by flowering earlier and ending later. Therefore, such a flowering strategy could enhance species fitness and was an evolutionary trend based on historical phenological records (Munguía-Rosas et al., 2011; Anderson et al., 2012).
Mean temperature, but not temperature range, affected the reproductive period of grasses. Mean MAT is associated with the accumulation of temperature over time and is therefore important to plant growth and reproduction (Sherry et al., 2011). For example, compared with temperate grasses with a growth season of 6 months or less, subtropical grasses could grow for a whole year. Mean MAT was also the only factor that differed between C3 and C4 species (Munson and Long, 2017; Figure 1E).
Species experiencing a wider range of precipitation tended to have a longer reproductive period. This seemingly contrasts previous findings that reproductive phenology was more sensitive to drought rather than high-level precipitation (Franks, 2011; Craine et al., 2012a), but the drier or the wetter the localities a species occupied, the higher the range of Pmax is. The longer reproductive period is a way to increase the fitness of species with sufficient water resources (Figures 2C,D).
Finally, PNC in flowering onset and its relationships with climatic niche indicated that, when climate changes, a temporal shift in flowering onset was predictable and similar within the same lineage (Staggemeier et al., 2010). Meanwhile, no PNC in the relationships between reproductive period and climatic niches may mean that this aspect of reproductive phenology is less sensitive to climatic change. Although many previous studies had reported PNC in flowering phenology (Lessard-Therrien et al., 2014; Du et al., 2015; Li et al., 2016), few had done phylogenetic analyses on their relationships with climatic variables. For example, Li et al. (2016) tested phylogenetic signals in both phenological and climatic data but did not use phylogeny when examining their relationships. Thus, phylogeny was needed to interpret how reproductive phenology was related with climatic variables.
We acknowledged certain caveats about our analyses. Although we found distinct patterns among groups, more accurate flowering onset and reproductive period data (accurate to date and long-term records) would be favored for future studies. The relatively coarse classification of life history (only the two extreme life histories were considered) and the climatic niches calculated for very widely distributed species might also impede the detection of the key factors in affecting phenology. Sampling species in one family and one region might limit the generality of our results. However, phylogenetic tests within one family could be more reliable than across more distantly related families (Staggemeier et al., 2010). Given that both genetic differences and phenotypic plasticity account for plant responses to climate change (Münzbergová et al., 2017; Malanson et al., 2019), plasticity in reproductive phenology might obscure our results. However, we focused on global climatic niches, which were more stable than local climates (Petitpierre et al., 2012). Furthermore, phenological data from one region could avoid phenotypic plasticity in that measured across larger scales (although it was nearly impossible to measure the phenology of many species in all their global localities), where great plasticity in plant traits occurs (Hoffman et al., 2020). Thus, our trait variation reflected intrinsic phylogenetic differences here (Liu et al., 2015), but phenotypic plasticity should also be considered in future studies trying to assess the effects of climate change (De Kort et al., 2020). Overall, we propose that climatic niche and phylogeny constraints should be considered simultaneously in studying the reproductive phenology of subtropical grasses.
Conclusion
In this study, we integrated the reproductive phenology, global-scale climatic niches, and phylogeny of 190 subtropical Poaceae species. We found strong phylogenetic signals in flowering onset but not in reproductive period, which emphasized the importance of phylogeny in comparative studies of reproductive phenology. There were significant interactive effects between photosynthetic pathway and life history on both flowering onset and reproductive period, such that C3 annual grasses flowered the earliest and had the longest reproductive period. We found four key climatic niche factors (ranges of Tmin, MAP, and Pmax and mean of MAT) associated with reproductive phenology through phylogenetic multiple stepwise regressions and demonstrated that species with wider climatic niches would flower earlier and have longer reproductive periods. Our results suggested that future studies on the role of climate on grass reproductive phenology should consider their evolutionary relationships.
Data Availability Statement
All datasets generated for this study are included in the article/Supplementary Material.
Author Contributions
HL and YC designed the study. HL collected the data. HL, KL, JW, LQ, YM, and RZ performed the analyses and drafted the first manuscript. All the authors contributed substantially to the revisions.
Funding
This work was supported by the National Natural Science Foundation of China (31670411), the Pearl River S&T Nova Program of Guangzhou (201806010083), and the Youth Innovation Promotion Association of the Chinese Academy of Sciences (2019339).
Conflict of Interest
The authors declare that the research was conducted in the absence of any commercial or financial relationships that could be construed as a potential conflict of interest.
Supplementary Material
The Supplementary Material for this article can be found online at: https://www.frontiersin.org/articles/10.3389/fevo.2020.00181/full#supplementary-material
FIGURE S1 | Global distribution localities of 190 subtropical grasses.
FIGURE S2 | A phylogenetic tree of 190 subtropical grasses, with corresponding information on photosynthetic pathway, life history, and reproductive phenology.
TABLE S1 | Full dataset in this study, with definitions and descriptions for each index.
TABLE S2 | Phylogenetic multiple stepwise regression analyses on flowering onset and reproductive period based on 18 climatic niche variables, photosynthetic pathway, and life history.
Footnotes
References
Albani, M. C., and Coupland, G. (2010). Comparative analysis of flowering in annual and perennial plants. Curr. Top. Dev. Biol. 91, 323–348. doi: 10.1016/S0070-2153(10)91011-9
Anderson, D. R. (2007). Model Based Inference in The Life Sciences: A Primer on Evidence. New York, NY: Springer.
Anderson, J. T., Inouye, D. W., McKinney, A. M., Colautti, R. I., and Mitchell-Olds, T. (2012). Phenotypic plasticity and adaptive evolution contribute to advancing flowering phenology in response to climate change. Proc. R. Soc. B Biol. Sci. 279, 3843–3852. doi: 10.1098/rspb.2012.1051
Atkin, O. K., Bloomfield, K. J., Reich, P. B., Tjoelker, M. G., Asner, G. P., Bonal, D., et al. (2015). Global variability in leaf respiration in relation to climate, plant functional types and leaf traits. New Phytol. 206, 614–636. doi: 10.1111/nph.13253
Atkinson, R. R. L., Mockford, E. J., Bennett, C., Christin, P. A., Spriggs, E. L., Freckleton, R. P., et al. (2016). C4 photosynthesis boosts growth by altering physiology, allocation and size. Nat. Plants 2, 1–5. doi: 10.1038/NPLANTS.2016.38
Bawa, K. S., Kang, H., and Grayum, M. H. (2003). Relationships among time, frequency, and duration of flowering in tropical rain forest trees. Am. J. Bot. 90, 877–887. doi: 10.3732/ajb.90.6.877
Cerdán, P. D., and Chory, J. (2003). Regulation of flowering time by light quality. Nature 423, 881–885. doi: 10.1038/nature01636
Chamberlain, S., Ram, K., Barve, V., and Mcglinn, D. (2014). rgbif: Interface to the Global Biodiversity Information Facility API. R Packag. version 0. 7. 7.
Colasanti, J., and Coneva, V. (2009). Mechanisms of floral induction in grasses: something borrowed, something new. Plant Physiol. 149, 56–62. doi: 10.1104/pp.108.130500
Corbesier, L., Vincent, C., Jang, S., Fornara, F., Fan, Q., Searle, I., et al. (2007). FT protein movement contributes to long-distance signaling in floral induction of Arabidopsis. Science 316, 1030–1033. doi: 10.1126/science.1141752
Cortés-Flores, J., Hernández-Esquivel, K. B., González-Rodríguez, A., and Ibarra-Manríquez, G. (2017). Flowering phenology, growth forms, and pollination syndromes in tropical dry forest species: influence of phylogeny and abiotic factors. Am. J. Bot. 104, 39–49. doi: 10.3732/ajb.1600305
Craine, J. M., Wolkovich, E. M., Gene Towne, E., and Kembel, S. W. (2012a). Flowering phenology as a functional trait in a tallgrass prairie. New Phytol. 193, 673–682. doi: 10.1111/j.1469-8137.2011.03953.x
Craine, J. M., Wolkovich, E. M., and Towne, E. G. (2012b). The roles of shifting and filtering in generating community-level flowering phenology. Ecography 35, 1033–1038. doi: 10.1111/j.1600-0587.2012.07625.x
Davies, T. J., Wolkovich, E. M., Kraft, N. J. B., Salamin, N., Allen, J. M., Ault, T. R., et al. (2013). Phylogenetic conservatism in plant phenology. J. Ecol. 101, 1520–1530. doi: 10.1111/1365-2745.12154
De Kort, H., Panis, B., Helsen, K., Douzet, R., Janssens, S. B., and Honnay, O. (2020). Pre-adaptation to climate change through topography-driven phenotypic plasticity. J. Ecol. 108, 1465–1474. doi: 10.1111/1365-2745.13365
de Xavier, R. O., Leite, M. B., and da Silva Matos, D. M. (2019). Phenological and reproductive traits and their response to environmental variation differ among native and invasive grasses in a Neotropical savanna. Biol. Invasions 21, 2761–2779. doi: 10.1007/s10530-019-02013-w
Donoghue, M. J. (2008). A phylogenetic perspective on the distribution of plant diversity. Proc. Natl. Acad. Sci. U.S.A. 105, 11549–11555. doi: 10.1073/pnas.0801962105
Du, Y., Mao, L., Queenborough, S. A., Freckleton, R. P., Chen, B., and Ma, K. (2015). Phylogenetic constraints and trait correlates of flowering phenology in the angiosperm flora of China. Glob. Ecol. Biogeogr. 24, 928–938. doi: 10.1111/geb.12303
Edwards, E. J., Osborne, C. P., Stromberg, C. A. E., Smith, S. A., Consortium, C. G., Bond, W. J., et al. (2010). The origins of C4 grasslands: integrating evolutionary and ecosystem science. Science 328, 587–591. doi: 10.1126/science.1177216
Edwards, E. J., and Smith, S. A. (2010). Phylogenetic analyses reveal the shady history of C4 grasses. Proc. Natl. Acad. Sci. U.S.A. 107, 2532–2537. doi: 10.1073/pnas.0909672107
Ehleringer, J. R. (1978). Implications of quantum yield differences on the distributions of C3 and C4 grasses. Oecologia 31, 255–267. doi: 10.1007/BF00346246
Elzinga, J. A., Atlan, A., Biere, A., Gigord, L., Weis, A. E., and Bernasconi, G. (2007). Time after time: flowering phenology and biotic interactions. Trends Ecol. Evol. 22, 432–439. doi: 10.1016/J.TREE.2007.05.006
Evans, L. T. (1971). Flower induction and the florigen concept. Annu. Rev. Plant Physiol. 22, 365–394. doi: 10.1146/annurev.pp.22.060171.002053
Fitter, A. H., and Fitter, R. S. R. (2002). Rapid changes in flowering time in British plants. Science 296, 1689–1691. doi: 10.1126/science.1071617
Franks, S. J. (2011). Plasticity and evolution in drought avoidance and escape in the annual plant Brassica rapa. New Phytol. 190, 249–257. doi: 10.1111/j.1469-8137.2010.03603.x
Grass Phylogeny Working Group (2012). New grass phylogeny resolves deep evolutionary relationships and discovers C4 origins. New Phytol. 193, 304–312. doi: 10.1111/j.1469-8137.2011.03972.x
Hart, R., Georgian, E. M., and Salick, J. (2016). Fast and cheap in the fall: phylogenetic determinants of late flowering phenologies in Himalayan Rhododendron. Am. J. Bot. 103, 198–206. doi: 10.3732/ajb.1500440
Hijmans, R. J., Cameron, S. E., Parra, J. L., Jones, P. G., and Jarvis, A. (2005). Very high resolution interpolated climate surfaces for global land areas. Int. J. Climatol. 25, 1965–1978. doi: 10.1002/joc.1276
Hijmans, R. J., van Etten, J., Sumner, M., Cheng, J., Bevan, A., Bivand, R., et al. (2015). Raster: Geographic Analysis and Modeling. R package version 2.8–19. Available online at: https://cran.r-project.org/web/packages/raster/ (accessed May 30, 2019).
Hoffman, A. M., Bushey, J. A., Ocheltree, T. W., and Smith, M. D. (2020). Genetic and functional variation across regional and local scales is associated with climate in a foundational prairie grass. New Phytol. [Online ahead of print]. doi: 10.1111/nph.16547
Lavergne, S., Thompson, J. D., Garnier, E., and Debussche, M. (2004). The biology and ecology of narrow endemic and widespread plants: a comparative study of trait variation in 20 congeneric pairs. Oikos 107, 505–518. doi: 10.1111/j.0030-1299.2004.13423.x
Lessard-Therrien, M., Davies, T. J., and Bolmgren, K. (2014). A phylogenetic comparative study of flowering phenology along an elevational gradient in the Canadian subarctic. Int. J. Biometeorol. 58, 455–462. doi: 10.1007/s00484-013-0672-9
Li, L., Li, Z., Cadotte, M. W., Jia, P., Chen, G., Jin, L. S., et al. (2016). Phylogenetic conservatism and climate factors shape flowering phenology in alpine meadows. Oecologia 182, 419–428. doi: 10.1007/s00442-016-3666-6
Liu, H., Edwards, E. J., Freckleton, R. P., and Osborne, C. P. (2012). Phylogenetic niche conservatism in C4 grasses. Oecologia 170, 835–845. doi: 10.1007/s00442-012-2337-5
Liu, H., and Osborne, C. P. (2015). Water relations traits of C4 grasses depend on phylogenetic lineage, photosynthetic pathway, and habitat water availability. J. Exp. Bot. 66, 761–773. doi: 10.1093/jxb/eru430
Liu, H., Osborne, C. P., Yin, D., Freckleton, R. P., Jiang, G., and Liu, M. (2019a). Phylogeny and ecological processes influence grass coexistence at different spatial scales within the steppe biome. Oecologia 191, 25–38. doi: 10.1007/s00442-019-04475-0
Liu, H., Taylor, S. H., Xu, Q., Lin, Y., Hou, H., Wu, G., et al. (2019b). Life history is a key factor explaining functional trait diversity among subtropical grasses, and its influence differs between C3 and C4 species. J. Exp. Bot. 70, 1567–1580. doi: 10.1093/jxb/ery462
Liu, H., Xu, Q., He, P., Santiago, L. S., Yang, K., and Ye, Q. (2015). Strong phylogenetic signals and phylogenetic niche conservatism in ecophysiological traits across divergent lineages of Magnoliaceae. Sci. Rep. 5:12246. doi: 10.1038/srep12246
Losos, J. B. (2008). Phylogenetic niche conservatism, phylogenetic signal and the relationship between phylogenetic relatedness and ecological similarity among species. Ecol. Lett. 11, 995–1003. doi: 10.1111/j.1461-0248.2008.01229.x
Lundgren, M. R., and Des Marais, D. L. (2020). Life history variation as a model for understanding trade-offs in plant-environment interactions. Curr. Biol. 30, R180–R189. doi: 10.1016/j.cub.2020.01.003
Lundgren, M. R., Dunning, L. T., Olofsson, J. K., Moreno-Villena, J. J., Bouvier, J. W., Sage, T. L., et al. (2019). C4 anatomy can evolve via a single developmental change. Ecol. Lett. 22, 302–312. doi: 10.1111/ele.13191
Lv, B., Nitcher, R., Han, X., Wang, S., Ni, F., Li, K., et al. (2014). Characterization of Flowering Locus T1 (FT1) gene in Brachypodium and wheat. PLoS One 9:e0094171. doi: 10.1371/journal.pone.0094171
MacLean, S. A., and Beissinger, S. R. (2017). Species’ traits as predictors of range shifts under contemporary climate change: a review and meta-analysis. Glob. Chang. Biol. 23, 4094–4105. doi: 10.1111/gcb.13736
Malanson, G. P., DeRose, R. J., and Bekker, M. F. (2019). Individual variation and ecotypic niches in simulations of the impact of climatic volatility. Ecol. Modell. 411:108782. doi: 10.1016/j.ecolmodel.2019.108782
Munguía-Rosas, M. A., Ollerton, J., Parra-Tabla, V., and De-Nova, J. A. (2011). Meta-analysis of phenotypic selection on flowering phenology suggests that early flowering plants are favoured. Ecol. Lett. 14, 511–521. doi: 10.1111/j.1461-0248.2011.01601.x
Münkemüller, T., Lavergne, S., Bzeznik, B., Dray, S., Jombart, T., Schiffers, K., et al. (2012). How to measure and test phylogenetic signal. Methods Ecol. Evol. 3, 743–756. doi: 10.1111/j.2041-210X.2012.00196.x
Munson, S. M., and Long, A. L. (2017). Climate drives shifts in grass reproductive phenology across the western USA. New Phytol. 213, 1945–1955. doi: 10.1111/nph.14327
Münzbergová, Z., Hadincová, V., Skálová, H., and Vandvik, V. (2017). Genetic differentiation and plasticity interact along temperature and precipitation gradients to determine plant performance under climate change. J. Ecol. 105, 1358–1373. doi: 10.1111/1365-2745.12762
Orme, D., and Freckleton, R. (2018). Caper: Comparative Analysis of Phylogenetics and Evolution in R. R package version 1.0.1. Available online at: https://cran.r-project.org/web/packages/caper/ (accessed May 30, 2019).
Osborne, C. P., Salomaa, A., Kluyver, T. A., Visser, V., Kellogg, E. A., Morrone, O., et al. (2014). A global database of C4 photosynthesis in grasses. New Phytol. 204, 441–446. doi: 10.1111/nph.12942
Pagel, M. (1999). Inferring the historical patterns of biological evolution. Nature 401, 877–884. doi: 10.1038/44766
Paradis, E., Claude, J., and Strimmer, K. (2004). APE: analyses of phylogenetics and evolution in R language. Bioinformatics 20, 289–290. doi: 10.1093/bioinformatics/btg412
Petitpierre, B., Kueffer, C., Broennimann, O., Randin, C., Daehler, C., and Guisan, A. (2012). Climatic niche shifts are rare among terrestrial plant invaders. Science 335, 1344–1348. doi: 10.1126/science.1215933
Primack, R. B., and Gallinat, A. S. (2017). Insights into grass phenology from herbarium specimens. New Phytol. 213, 1567–1568. doi: 10.1111/nph.14439
Quintero, I., and Wiens, J. J. (2013). What determines the climatic niche width of species? The role of spatial and temporal climatic variation in three vertebrate clades. Glob. Ecol. Biogeogr. 22, 422–432. doi: 10.1111/geb.12001
R Core Team (2018). R: A Language and Environment for Statistical Computing. Vienna: R Foundation for Statistical Computing.
Rathcke, B., and Lacey, E. P. (1985). Phenological patterns of terrestrial plants. Annu. Rev. Ecol. Syst. 16, 179–214. doi: 10.1146/annurev.es.16.110185.001143
Revell, L. J. (2010). Phylogenetic signal and linear regression on species data. Methods Ecol. Evol. 1, 319–329. doi: 10.1111/j.2041-210X.2010.00044.x
Sage, R. F., Sage, T. L., and Kocacinar, F. (2012). Photorespiration and the evolution of C4 photosynthesis. Annu. Rev. Plant Biol. 63, 19–47. doi: 10.1146/annurev-arplant-042811-105511
Schubert, M., Grønvold, L., Sandve, S. R., Hvidsten, T. R., and Fjellheim, S. (2019). Evolution of cold acclimation and its role in niche transition in the temperate grass subfamily Pooideae. Plant Physiol. 180, 404–419. doi: 10.1104/pp.18.01448
Sherry, R. A., Zhou, X., Gu, S., Arnone, J. A., Johnson, D. W., Schimel, D. S., et al. (2011). Changes in duration of reproductive phases and lagged phenological response to experimental climate warming. Plant Ecol. Divers. 4, 23–35. doi: 10.1080/17550874.2011.557669
Slatyer, R. A., Hirst, M., and Sexton, J. P. (2013). Niche breadth predicts geographical range size: a general ecological pattern. Ecol. Lett. 16, 1104–1114. doi: 10.1111/ele.12140
Soberón, J. (2007). Grinnellian and Eltonian niches and geographic distributions of species. Ecol. Lett. 10, 1115–1123. doi: 10.1111/j.1461-0248.2007.01107.x
South China Botanical Garden (2009). Flora of Guangdong (In Chinese). Guangzhou: Guangdong Science and Technology Press.
Springer, C. J., and Ward, J. K. (2007). Flowering time and elevated atmospheric CO 2. New Phytol. 176, 243–255. doi: 10.1111/j.1469-8137.2007.02196.x
Staggemeier, V. G., Camargo, M. G. G., Diniz-Filho, J. A. F., Freckleton, R., Jardim, L., and Morellato, L. P. C. (2019). The circular nature of recurrent life cycle events: a test comparing tropical and temperate phenology. J. Ecol. 108, 393–404. doi: 10.1111/1365-2745.13266
Staggemeier, V. G., Diniz-Filho, J. A. F., and Morellato, L. P. C. (2010). The shared influence of phylogeny and ecology on the reproductive patterns of Myrteae (Myrtaceae). J. Ecol. 98, 1409–1421. doi: 10.1111/j.1365-2745.2010.01717.x
Tamaki, S., Matsuo, S., Hann, L. W., Yokoi, S., and Shimamoto, K. (2007). Hd3a protein is a mobile flowering signal in rice. Science 316, 1033–1036. doi: 10.1126/science.1141753
Taylor, S. H., Ripley, B. S., Martin, T., De-Wet, L. A., Woodward, F. I., and Osborne, C. P. (2014). Physiological advantages of C4 grasses in the field: a comparative experiment demonstrating the importance of drought. Glob. Chang. Biol. 20, 1992–2003. doi: 10.1111/gcb.12498
Thuiller, W., Lavorel, S., Midgley, G., Lavergne, S., and Rebelo, T. (2004). Relating plant traits and species distributions along bioclimatic gradients for 88 Leucadendron taxa. Ecology 85, 1688–1699. doi: 10.1890/03-0148
Warton, D. I., Duursma, R. A., Falster, D. S., and Taskinen, S. (2012). smatr 3-an R package for estimation and inference about allometric lines. Methods Ecol. Evol. 2, 257–259. doi: 10.1111/j.2041-210x.2011.00153.x
Watson, L., Macfarlane, T. D., and Dallwitz, M. J. (1992). The Grass Genera of The World. Available online at: http://delta-intkey.com/ (accessed March 30, 2019).
Way, D. A., Katul, G. G., Manzoni, S., and Vico, G. (2014). Increasing water use efficiency along the C3 to C4 evolutionary pathway: a stomatal optimization perspective. J. Exp. Bot. 65, 3683–3693. doi: 10.1093/jxb/eru205
Wiens, J. J. (2004). Speciation and ecology revisited: phylogenetic niche conservatism and the origin of species. Evolution 58, 193–197. doi: 10.1554/03-447
Wiens, J. J., and Graham, C. H. (2005). Niche conservatism: integrating evolution, ecology, and conservation biology. Annu. Rev. Ecol. Evol. Syst. 36, 519–539. doi: 10.1146/annurev.ecolsys.36.102803.095431
Keywords: diversity, flowering phenology, geographic distribution, climatic niche, phylogenetic niche conservatism, life history, photosynthetic pathway
Citation: Li K, Wang J, Qiao L, Zheng R, Ma Y, Chen Y, Hou X, Du Y, Gao J and Liu H (2020) Diversity of Reproductive Phenology Among Subtropical Grasses Is Constrained by Evolution and Climatic Niche. Front. Ecol. Evol. 8:181. doi: 10.3389/fevo.2020.00181
Received: 25 December 2019; Accepted: 22 May 2020;
Published: 30 June 2020.
Edited by:
George P. Malanson, The University of Iowa, United StatesReviewed by:
Rafael De Oliveira Xavier, University of São Paulo, BrazilDavid R. Butler, Texas State University, United States
Copyright © 2020 Li, Wang, Qiao, Zheng, Ma, Chen, Hou, Du, Gao and Liu. This is an open-access article distributed under the terms of the Creative Commons Attribution License (CC BY). The use, distribution or reproduction in other forums is permitted, provided the original author(s) and the copyright owner(s) are credited and that the original publication in this journal is cited, in accordance with accepted academic practice. No use, distribution or reproduction is permitted which does not comply with these terms.
*Correspondence: Hui Liu, aHVpLmxpdUBzY2JnLmFjLmNu