- 1Division of Sustainable Energy and Environmental Engineering, Graduate School of Engineering, Osaka University, Suita, Japan
- 2Graduate School of Agriculture, Hokkaido University, Sapporo, Japan
- 3Field Science Center for Northern Biosphere, Hokkaido University, Sapporo, Japan
- 4Akkeshi Marine Station, Field Science Center for Northern Biosphere, Hokkaido University, Akkeshi, Japan
- 5Research Faculty of Agriculture, Hokkaido University, Sapporo, Japan
- 6Department of Ecosystem Studies, The University of Tokyo, Bunkyo-ku, Japan
- 7Institute for Global Environmental Strategies, Hayama, Japan
The introduction of renewable energy (RE) is essential for building a sustainable society. However, RE can cause conflicts between energy production and biodiversity conservation. This study conducted a scenario analysis to evaluate potential conflicts in the nexuses between energy and biodiversity for the Bekambeushi River watershed located in northeastern Japan. The increasing rate of pastureland abandonment resulting from a declining farmer population is a source of great uncertainty in this area. Two alternative sources of RE were selected to utilize these abandoned pasturelands, each taking a unique approach to meet targets stipulated by regional energy plans, thereby producing different ecological consequences at the landscape scale. Thirty-one RE introduction options were simulated, comprising a range of pastureland abandonment expansion speeds and ratios of solar photovoltaic (PV) plant installation to biomass energy use. These were superimposed using two IPCC representative concentration pathway (RCP) scenarios, 2.6 and 8.5, resulting in 62 scenarios that were summarized as three groups based on the RE supply–demand balance and the ecological impacts. The LANDIS-II model was used to simulate these scenarios from 2016 to 2100. The results indicate that both the rate of pastureland abandonment and the ratio of the two RE sources had a large impact on changes in tree species diversity and the habitat suitability of raptors. Abandoned pastureland converted to tree biomass energy production shifted to pioneer species-dominated forest. The plant species composition of transitional forests varied between the climate scenarios. The higher temperature of the RCP 8.5 scenario toward 2100 prevented the establishment of Betula platyphylla and altered tree species diversity and the habitat suitability of Ketupa blakistoni blakistoni. Biomass energy utilization produced less energy than the demand but increased the three ecological indicators. Solar PV systems provided more energy than the regional demand, but the tree diversity and habitat suitability indices for two raptors declined. However, an appropriate mixture of the two RE sources satisfied the regional energy demand and maintained ecological conditions. Our results suggest that land–energy planning should consider energy–biodiversity nexuses to strike a balance between decarbonization and biodiversity conservation.
Introduction
Building a decarbonized society has become a key global concern (UNFCCC, 2015). Currently, 186 parties have submitted a nationally determined contribution (NDC) aimed at limiting global warming to 1.5–2°C (UNFCCC, 2019). Japan’s NDC pledged to reduce CO2 emissions by 80% by 2050 (Cabinet Office, Government of Japan, 2016). In Japan, 86.3% of greenhouse gas emissions in 2016 were CO2 from the energy sector (Ministry of the Environment, Japan [MOE], 2019a), and the government is planning to shift to a decarbonized energy supply system (Ministry of the Environment, Japan [MOE], 2018, 2019b). However, the Special Report on Global Warming of 1.5°C (IPCC, 2018) suggested that the emission pathway reflecting the current NDCs will raise the global mean temperature by 3°C. Therefore, against the current situation, transformative changes in social systems are required to build a sustainable society (IPCC, 2018; IPBES, 2019).
Promoting the introduction of renewable energy (RE) plays a key role in reducing greenhouse gas emissions (IPCC, 2014), but its introduction could impose a burden on ecosystems, such as land use change during construction and maintenance during operation (Abbasi and Abbasi, 2000; Field et al., 2008; Gasparatos et al., 2017; Gibson et al., 2017). The IPBES also indicated the need for careful consideration of ecosystems when introducing RE (IPBES, 2019). The introduction of RE is related to the UN’s Sustainable Development Goals (SDGs): Goal 7: Affordable and clean energy, Goal 13: Climate action, and Goal 15: Life on land (United Nations, 2015). Therefore, toward developing a post-2020 biodiversity framework, RE utilization constructed in harmony with nature is essential for building a sustainable society.
Previous global-scale studies have demonstrated trade-offs between biodiversity conservation and climate change mitigation, including the introduction of RE (de Vries et al., 2007; Santangeli et al., 2016a; Palomo et al., 2019). Santangeli et al. (2016b) identified the overlap of biodiversity conservation areas with high potential for crop, solar photovoltaic (PV), and wind energy production to identify areas that have a low impact on biodiversity. Harper et al. (2018) pointed out that the land use conversions of carbon-rich forests for energy crop production to mitigate climate change would reduce the total amount of carbon fixation by coupling an integrated assessment model (IAM) and a dynamic global vegetation model or DGVM. Ohashi et al. (2019) predicted changes in the habitats of 8,428 species using a species distribution model based on future land use under Shared Socioeconomic Pathways (SSPs) scenarios reflecting climate change mitigation measures calculated using an IAM and stated that habitat reduction due to climate change is greater than land use conversion under mitigation measures.
Local-scale studies have also indicated potential conflicts between the introduction of RE and biodiversity (Kienast et al., 2017). Tarr et al. (2017) calculated the future habitat suitability of 16 wildlife species under biomass energy harvesting scenarios using a spatially explicit state-and-transition model. Tarr et al. (2017) stated that bioenergy policies will cause trade-offs between species that require different ecological niches. Hori et al. (2016) and Hori et al. (2019) optimized the RE mix at a local scale by focusing on solar, hydro, wind, geothermal, and biomass resources and visualized trade-offs six indicators: the proportion of developed RE, economic balance between initial installation costs and returns from RE production, decrease in CO2 emissions, circulation rate of biomass resource in a region, RE diversity, and area of potentially impacted ecosystem. Moore-O’Leary et al. (2017) described relationships between land, energy, and ecosystems as nexuses that invoke such trade-offs. The types and amount of RE sources are spatially distributed heterogeneously (Pogson et al., 2013), and the environmental impacts of RE utilization differ among RE sources (Abbasi and Abbasi, 2000; Gibson et al., 2017). Therefore, climate change mitigation plans need to be developed considering the nexuses between local RE resources and biodiversity conservation.
Quantitative models can effectively evaluate the impact of RE on ecosystems (Laranjeiro et al., 2018; Raoux et al., 2018). Future climate change will also affect local ecosystem conditions and alter the supply potentials of various ecosystem services (Cantarello et al., 2017). Most studies dealing with conflicts between the introduction of RE and biodiversity have only focused on changes in land use and land cover classes as state spaces of the Markov process (Costanza et al., 2015; Duden et al., 2017; Tarr et al., 2017). However, process-based models that simulate the climate change response of vegetation will provide robust and informative scientific suggestions (Gustafson et al., 2015; Keane et al., 2015) for maintaining future energy–biodiversity nexuses to local stakeholders.
A FLM is a process-based dynamic simulation model of vegetation succession at a landscape scale under future climate change (Xi et al., 2009; Shifley et al., 2017). Previous studies have applied FLMs to quantify the effects of human and natural disturbances, such as vegetation change caused by economic growth (Ward et al., 2005; Thompson et al., 2016; Duveneck and Thompson, 2019) and from a shrinking society (Haga et al., 2018; Sil et al., 2019). FLMs have also been used to simulate the impacts of aboveground and deadwood biomass harvesting for fuels on ecosystem services and the quality of wildlife habitats (see, for example, Creutzburg et al., 2016; Thompson et al., 2016; Hof et al., 2018).
Future social scenario narratives in the fields of both climate change and biodiversity and ecosystem services do not specify ecosystem management activities under decarbonization requirements at local scales (Millennium Ecosystem Assessment [MA], 2005; O’Neill et al., 2014). Therefore, the purpose of this study was to explore future scenarios that strike a balance between the introduction of RE and biodiversity conservation under changing climate and societal conditions using a process-based forest landscape model (FLM). The specific objectives were to (1) simulate ecosystem impacts and expected energy supply under mixed RE energy installation scenarios, (2) identify the RE–biodiversity nexuses in a local basin, and (3) explore the scenarios to meet both RE utilization and biodiversity conservation.
Materials and Methods
We simulated vegetation succession under different land use scenarios and evaluated energy supply potential, ecosystem services, and ecological impacts using an FLM.
Study Area Description
The Bekambeushi River watershed in northeastern Japan, where RE introduction plans, such as woody biomass (Akkeshi town, 2018a) and a solar PV plant (Nikkei XTECH, 2018), are underway, was selected as the case study area (Figure 1A). The total area of the watershed is 700 km2, with a small difference in elevation (maximum elevation = 141 m) (Figure 1B; Geosptial Information Authority of Japan [GSI], 2019). The forest and pastureland soils consist of Andosols (Obara et al., 2016). The current monthly mean air temperature ranges from −8 to 20°C and annual precipitation is 1200 mm (Esgf-CoG, 2017). In particular, changes in temperature are concerned, with the mean air temperature increase 1.4–5.0°C by 2100 under RCP scenarios (Esgf-CoG, 2017). Forests and pasture lands cover 70 and 20% of the watershed, respectively (Figure 1C; Biodiversity Center of Japan, 2017). The Bekambeushi River wetland was listed in the Ramsar Convention in 1993 (Akkeshi town, 2019a). In the national forest in the northern areas, the dominant species is Larix kaempferi (Lamb.) Carrière. In the private forest in the southern areas, the dominant species are Sakhalin fir [Abies sachalinensis (F. Schmid)] Mast.] and a mixed forest of Sakhalin fir and Japanese oak (Quercus crispula Blume). In this study area, deer browsing damages to agriculture and forestry are the major natural disturbances, and local government are working to capture them (Akkeshi town, 2018c).
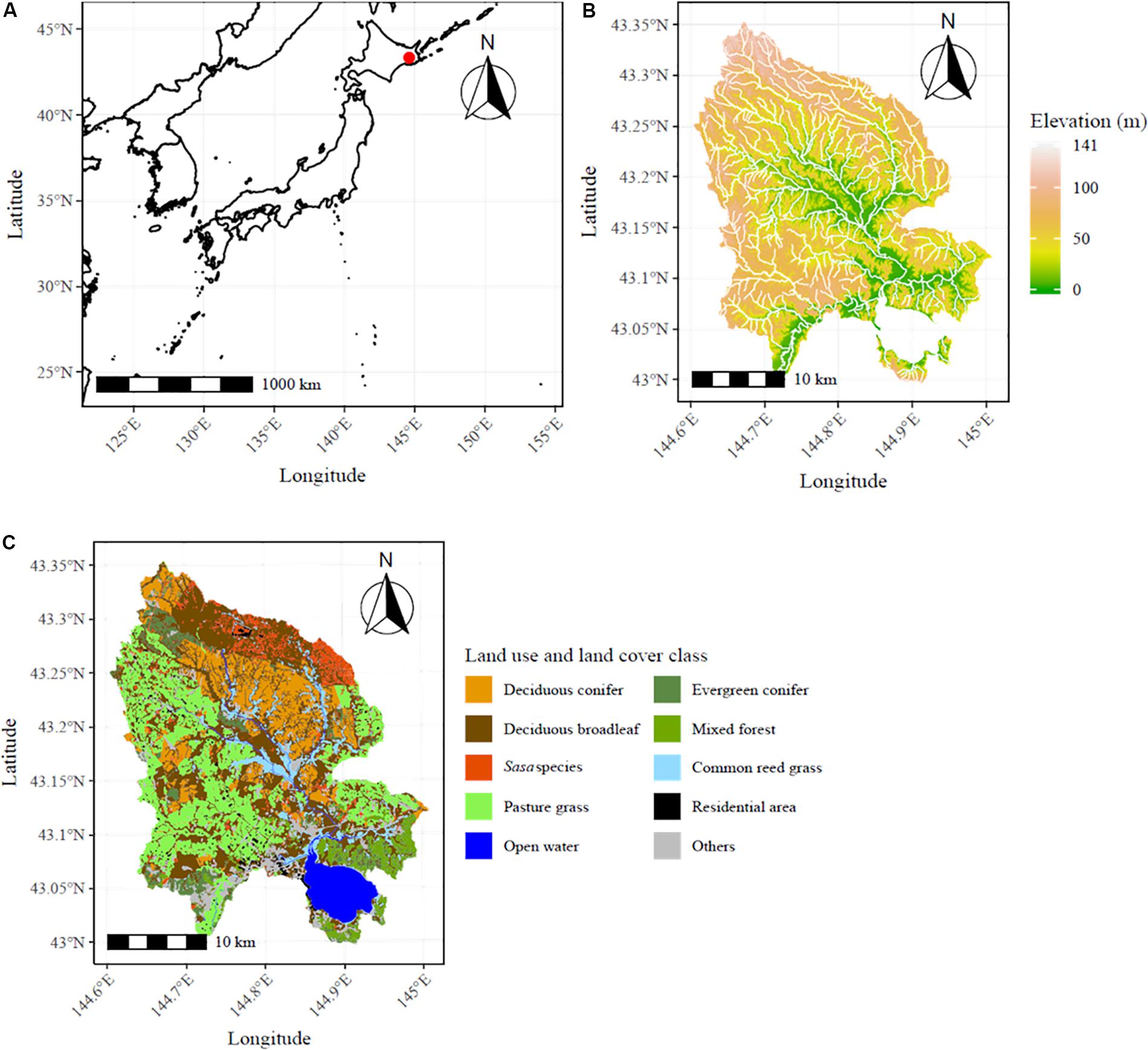
Figure 1. Description of the study area. (A) The location of the Bekambeushi River watershed (red dot), (B) the digital elevation model and distribution of rivers (white lines), and (C) the vegetation distribution.
The main industries are fisheries and aquaculture in Akkeshi Bay, Lake Akkeshi, and the offshore area; forestry in the national and private forests; and dairy farming (Akkeshi town, 2018b), but the rapid decline in primary industries is of major concern in the region. The total residential population in the watershed was 8,604 in 2010 and is projected to be 4,980 by 2050 (National Land Numerical Information [NLNI], 2017). The area of the clear-cutting and thinning of larch and Sakhalin fir for timber and pulp production has declined in recent years (Hokkaido Prefecture, 2019). The region’s administrative documents reflect concern that the abandonment of pastureland will increase because of the declining population (Akkeshi town, 2019b). This decline is a baseline trend in Japan (National Institute of Population and Social Security Research [NIPSSR], 2018; Saito et al., 2018), especially in rural areas (Matsui et al., 2019).
Model Description
In this study, the LANDIS-II model, version 7.0 (Scheller et al., 2007), a widely applied FLM, was used to simulate the ecological impact of the introduction of RE. LANDIS-II is a modeling platform comprising a suite of extensions to simulate various establishment, growth, and disturbance processes. This modeling platform currently attempts to integrate growth and material cycles by referring to mechanistic models, such as the CENTURY soil organic matter model and PnET-II to expand its applicability to robust climate change impact assessment (Scheller et al., 2011; de Bruijn et al., 2014). The model computes vegetation succession at landscape scales by representing landscapes as grid cells. The state of vegetation in each grid cell is represented as a species–age cohort.
The Net Ecosystem Carbon and Nitrogen (NECN) Succession version 6.3 (Scheller et al., 2011), was used to simulate vegetation dynamics under climate change. The extension computes cohort establishment and biomass growth as functions of the environmental condition. The probability of establishment for each grid cell for each species is defined by a function of the minimum January temperature, soil and water, growing degree days (GDD), and light availability. The biomass growth is calculated as the balance between monthly net primary productivity (NPP) and mortality. The monthly NPP was computed by multiplying the monthly maximum NPP by the environmental limits in a grid cell: monthly mean temperature, plant-available soil N, the water and light availability, and density effects. Species and functional group parameters were determined in reference to Haga et al. (2018) and the default value of the CENTURY soil organic matter model (see Supplementary Material 1). We calibrated the NECN succession extension by comparing the simulated and observed aboveground biomass (AGB) growth and litterfall (see Supplementary Material 2).
Settings for the Introduction of Renewable Energy Scenarios
In a shrinking society, there are great uncertainties around the presence of unused land and how to use it (Shoyama et al., 2018). Therefore, we designed 31 scenarios of land use and RE introduction from 2016 to 2100 comprising varied speeds of pastureland abandonment expansion and ratios of solar PV plant installation to biomass energy use (Table 1). First, six scenarios for pastureland abandonment expansion speed were set (Table 1). The lowest speed scenario was 0 ha year–1, maintaining all pastureland to 2100. In the highest speed scenario, we assumed that pastureland would be abandoned at the same speed of population decline. Therefore, the area of abandoned pastureland increased to 42% of the current pastureland in 2050 according to a population decline of 58%. The other four scenarios were set at equal intervals between 0 and 223 ha year–1 (Table 1).
For each pastureland abandonment scenario, the locations of the abandoned pastureland were determined using a Manageability index that considered the multiple socio-geographical conditions of each grid cell. The index assumes that a grid cell with a lower population in the nearest residential area, a farther the distance to the residential area and the nearest road, a larger slope, higher elevation, and the smaller the pastureland patch area, the lower the Manageability (Haga et al., 2018; Kobayashi and Nakamura, 2018). Pastureland grid cells with lower Manageability were abandoned first. Manageability was calculated as follows:
where Manageabilityi is the manageability of grid cell i; popi; and distToResii are the population and the distance to the nearest residential grid cell in 2050 from the grid cell i (m), respectively (National Land Numerical Information [NLNI], 2017); and distToRoadiis the distance to the nearest road from the grid cell i (m). meaSlopei and meaElevi denote the mean slope (degree) and elevation (m) of grid celli, respectively. patchAreai is the area of the pastureland patch in which the grid cell i belongs (m2).
The following two types of abandoned pastureland were excluded from any RE introduction: abandoned pastureland (1) within 300 m of a river or (2) with a wetland history. The former was to conserve riparian forest, which is an ecologically and culturally important ecosystem (Nakaoka et al., 2018), and the latter was because the pastureland has the potential to become wetland (Morimoto et al., 2017). Wetland history was identified using a 1920s historical topographical map (Kaneko et al., 2008).
Abandoned pastureland was categorized as two types of land use according to the distance to the nearest forest for each year: land for producing solar energy and land for obtaining woody biomass energy. This allocation was based on the development policy that solar PV plants are initially installed on abandoned pastureland far away from forests to minimize impacts on wildlife, with the balance of the abandoned pastureland used for biomass energy production. Six ratios were used to represent the mixture of solar PV plants and woody biomass energy scenarios (Table 1).
The AGB of all tree species in the abandoned pastureland used for biomass energy production was harvested. Under the current climate condition, Japanese white birch (Betula platyphylla Sukaczev var. japonica) is the representative regional pioneer species expected to establish on the abandoned pastureland. Therefore, we clear-cut all the tree species in the abandoned pastureland for biomass energy production where it contained 25-year-old Japanese white birch, the maximum growth period. No species were planted after the clear-cutting because natural regeneration was expected. On the abandoned pastureland used for solar PV plants, all the grass and tree species were removed every year for the maintenance of the solar PV panels.
Simulation Settings Common for All Scenarios
The NECN succession extension requires spatially explicit initial conditions for both the age and AGB for each plant species. We created an initial tree species distribution at 100 m resolution. Plant species distribution was obtained from a vegetation map that recorded community names (Biodiversity Center of Japan, 2017). The dominant plant communities, which cover 95% of the watershed, were selected for the simulation (Supplementary Table 4), and seven tree species and two grass species were selected as a result (Table 2). The initial age and AGB were set according to the forest registers of the national, prefectural, and private forests (Hokkaido Prefecture, 2017a, b; Ministry of Agriculture Forestry and Fisheries [MAFF], 2017). Soil organic matter (SOM) content, soil depth and properties related to soil carbon and water dynamics were determined uniformly for each forest and pasture from the literature (Hokkaido National Agricultural Experiment Station, 1983). The decomposition rates of SOM were set according to the calibration criteria proposed in the previous study (Lucash et al., 2019).
The Biomass Harvest extension, version 4.3, was used to apply the same forest management practice to all scenarios referring to the regional standard plan (Akkeshi town, 2017; Hokkaido Prefecture, 2017c; Supplementary Table 4). The target species were larch, Sakhalin fir, and Japanese oak. In the timber production forest, the target species were clear-cut and replanted. In the conservation area, such as the riparian forest, selective cutting and planting of the target species were conducted. Thinning was applied to cohorts that reached the thinning age used as standard practice in this area (Akkeshi town, 2017; Hokkaido Prefecture, 2017c).
Representative concentration pathway (RCP) 2.6 and 8.5 scenarios (IPCC, 2013) calculated by the MRI-CGCM3 model (Esgf-CoG, 2017) were selected as the climate data to evaluate uncertainty corresponding to climate change. To correct for bias between the MRI-CGCM3 data and the observations, monthly mean temperature and monthly precipitation offsets were determined by comparing data from the model and the Ota Meteorological Observatory (Japan Meteorological Agency, 2018; Supplementary Table 5). In total, 62 simulations, a combination of the 31 RE introduction scenarios and the two climate scenarios were conducted from 2016 to 2100.
Evaluation Indicators
Renewable energy production and three ecological indicators were evaluated as follows using the simulated land use and land cover (LULC) changes, AGB, and harvested biomass. R, version 3.6.3 (R Core Team, 2019) was used for the analysis.
Renewable Energy Supply
The amount of RE supply was estimated as heat energy. First, the heat energy obtained by burning the woody biomass (TJ year–1) was calculated (Tatebayashi et al., 2015) by assuming that all harvested AGB (oven-dry kg biomass year–1) obtained from the abandoned pastureland and forest thinning was used for pellets. The moisture content, yield rate, and lower calorific value were set to 10, 80%, and 16.0 MJ kg–1 (Japan Wood Pellet Association, 2017). Harvested biomass is storable, so a 5 years moving average was used to evaluate the expected amount of heat energy per year.
Second, the amount of the electrical energy generated by the solar PV plants was calculated by multiplying the total area of the solar PV plants per year (m2) with a basic unit of annual power generation. We used 61.58 kWh m–2 year–1 as the basic unit, as used in a previous RE estimation procedure (Ministry of the Environment, Japan [MOE], 2010). This generated electrical energy was then converted to heat energy (TJ year–1) by multiplying with a conversion unit of 3.6 × 106.
Ecological Impacts
Three ecological indicators were evaluated: plant species diversity and two habitat suitability indices (HSI), the mountain hawk-eagle (Spizaetus nipalensis orientalis) and the Blakiston’s fish owl (Ketupa blakistoni blakistoni).
The Shannon–Wiener diversity indices for the plant species were calculated using the simulated AGB for each grid cell. Abandoned pastureland converted to solar PV plants was regarded as a zero diversity index. Abandoned pastureland with a wetland history was also set to zero because the land was excluded from the LANDIS-II simulation.
We calculated the habitat suitability indices for the mountain hawk-eagle and the Blakiston’s fish owl, which have different ecological niches, as representative species affected by the land use changes. The mountain hawk-eagle is a raptorial bird, 70–80 cm in length, that lives in steeply sloping mountain forests (Ministry of the Environment, Japan [MOE], 2012). The eagle uses mature forests and forest edges to prey on small to medium reptiles, birds, and mammals living in the forest (Ministry of the Environment, Japan [MOE], 2012). A habitat suitability index for the mountain hawk-eagle was calculated with a 1 km resolution using the following (Itoh et al., 2012):
where ps is the habitat suitability index for the mountain hawk-eagle for each 1 km grid cell; X1 and X2 are the mean elevation (m) and slope (degree) of the grid cell, respectively; X3 is the occupancy of the broadleaf forest and mixed forest area of the grid cell; X4 is the occupancy of the plantation Cryptomeria japonica (L.f.) D. Don and Chamaecyparis obtusa (Siebold et Zucc.) Endl. of the grid cell; X5 is the occupancy of open area suitable for foraging activities in the grid cell; and X6 is the length of forest edge (m) between the open area and forests in the grid cell. For X3, if the AGB of the broadleaf species occupy more than 30% of the total AGB for each 1 km grid, the grid was identified as broadleaf and mixed forest. X4 was set to zero for all grids because these tree species are not distributed in the study area. Pastureland, grassland, and wetland were regarded as open areas for X5 and X6. A previous study reported that solar PV plants contribute to increases in the populations of small grassland birds (Kitazawa et al., 2019). However, because there is concern that the feeding behavior of raptors could be hindered by solar PV plants (Walston et al., 2016), we excluded the solar PV plants.
The Blakiston’s fish owl is a nocturnal raptor with a total length of ∼70 cm. In 2018, the owls were only living in Hokkaido, Japan, and are classified as a critically endangered species (Ministry of the Environment, Japan [MOE], 2019c). This owl preys mainly on fish and amphibians and normally nests in tree cavities in riparian forests (Yoshii et al., 2018), however, successful cases of nesting in artificially installed nest boxes have been confirmed (Takenaka, 2018). Therefore, habitat suitability for the Blakiston’s fish owl was calculated for each grid with a 4 km resolution using the following (Yoshii et al., 2018):
where pkis the habitat suitability index for the owl for each 4 km grid cell, Y1 is the total area (m2) of riparian natural forest within 300 m of rivers (National Land Numerical Information [NLNI], 2009) for each grid cell, and Y2 is the total length (m) of rivers for each grid cell. The current species composition in natural forests in this region is Abies sachalinensis, Abies sachalinensis-Quercus crispula, Ulmus davidiana, Alnus japonica, and Alnus japonica-Fraxinus mandshurica communities (Biodiversity Center of Japan, 2017). If riparian forests within 300 m of rivers consisted of these species and had not been either clear-cut or planted, we categorized them as natural forests. Regular maintenance activities for solar PV systems near riparian forests may diminish the breeding success of the owl; therefore, we excluded riparian natural forests within 300 m of solar PV plants in the calculation (Takenaka, 2018).
Finally, 62 scenarios were summarized into three groups by referring to the balance between the mean RE production from 2090 to 2100 and the total energy demand of the area in 2010. We evaluated the relative ecological impacts of land use change, RE introduction, and climate change for each scenario by comparing mean values from 2090 to 2100 for the three ecological indicators.
Results
Changes in LULC and Aboveground Biomass
In the A0 scenario, which maintains all pastureland, the changes in both LULC and AGB differed between the RCP scenarios (Figure 2). However, Japanese white birch forest shifted to the other broadleaf forests regardless of the RCP scenario, but this trend was accelerated in the RCP 8.5 scenario (Figure 2A). The birch forest declined from 137 to 26 km2 in 2100 and shifted to Japanese alder after 2050 in the RCP 8.5 scenario (Figure 2A and Supplementary Table 6). The total changes, including both an increase and a decrease in area, were the highest among the pioneer species, such as the Japanese birch and Japanese alder, and the target forestry species, such as larch and Sakhalin fir (Figure 2A; total change in Supplementary Table 6). These two pioneer species and the larch forest shifted to the other species (net change in Supplementary Table 6). The total area of Sakhalin fir forest was maintained (Supplementary Table 6). With an increasing area of alder forest, the total AGB within the watershed reached 55.8 and 53.7 Gg biomass in the RCP 2.6 and 8.5 scenarios, respectively (Figure 2B). In the RCP 8.5 scenario, the AGB of Sakhalin fir, Japanese alder, and Japanese ash declined by 20–40%, and the AGB of Japanese white birch and Japanese oak increased by 22–36% (Figure 2B).
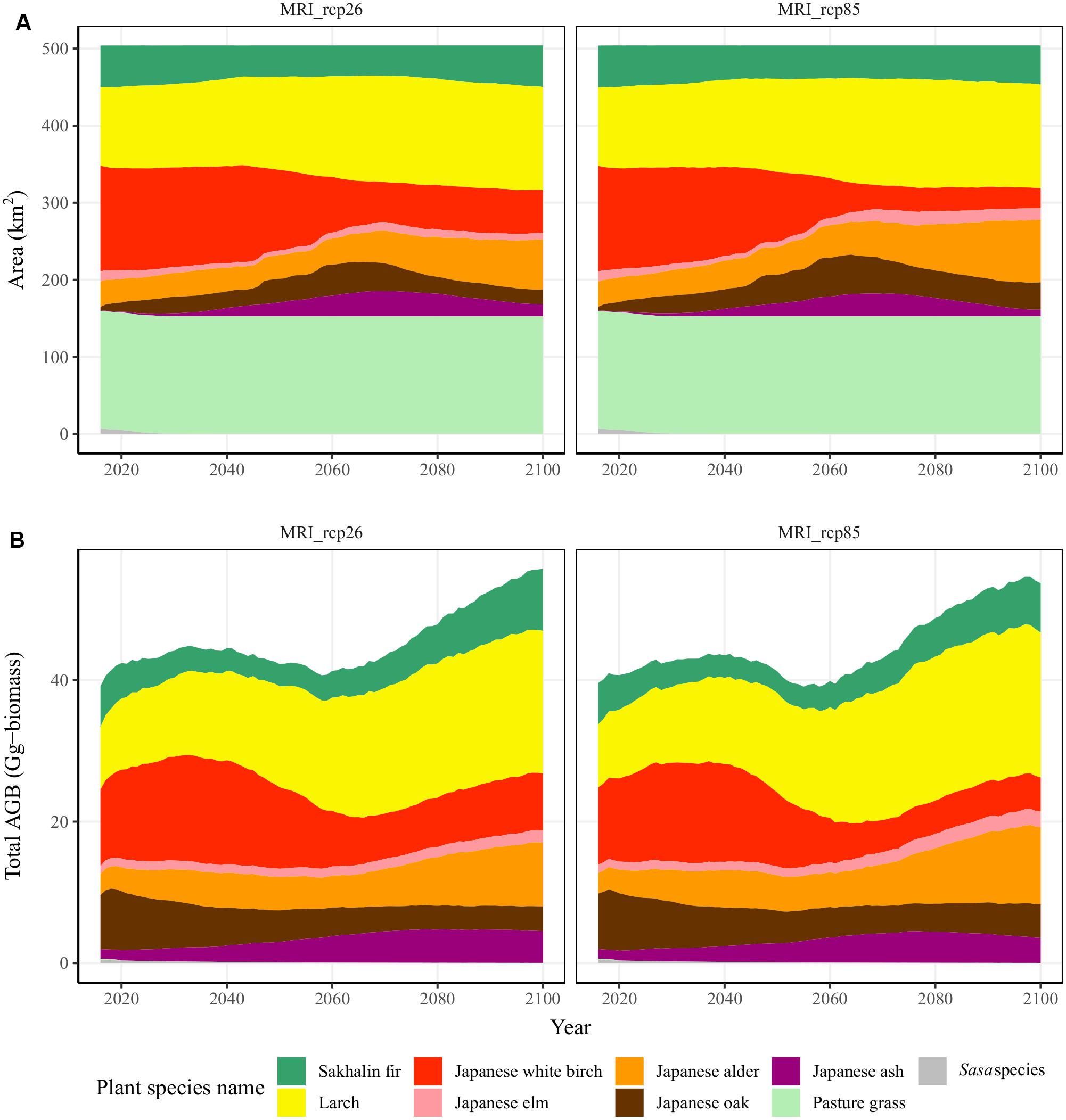
Figure 2. Changes in the (A) land use and land cover and (B) the total aboveground biomass (AGB) for the scenario with no pastureland abandonment expansion (A0) for two climate scenarios (left = IPCC RCP 2.6 and right = IPCC RCP 8.5). The colors indicate the nine plant species simulated in this study.
In the RE introduction scenarios, the abandoned pastureland was shifted to broadleaf forests and wetlands or converted to solar PV plants (Supplementary Figures 7A,B). In the 1920s, 8% of the pastureland was wetland, and these converted pasturelands were returned to wetlands after being abandoned. In the abandoned pastureland converted to tree biomass energy production, pioneer species, such as Japanese white birch and Japanese alder, were established. Like the changes in the forests, the AGB of alder increased toward 2100 in the RCP 8.5 scenario (Supplementary Figures 7A,B). In the riparian forest, especially, the total AGB increased up to 3 and 5 Gg biomass with increasing Japanese alder in the RCP 2.6 and 8.5 scenarios, respectively. In the abandoned pastureland used for tree biomass energy production, Japanese white birch declined in the RCP 8.5 scenario, and thus, the total AGB in 2100 was 15 and 13 Gg biomass in the RCP 2.6 and 8.5 scenarios, respectively.
The distribution of Japanese white birch and Japanese alder in Japan ranges between 958–2,598 and 1,066–2,842 degrees in annual GDD, respectively (5°C base threshold). The Japanese alder is thus distributed in a warmer environment than the Japanese white birch (Forestry Agency of Japan, 2019; National Land Numerical Information [NLNI], 2012). The Japanese white birch is the representative pioneer plant species in this region under the current climate (Resco de Dios et al., 2005). In the RCP 2.6 scenario, the Japanese white birch successfully established on abandoned pastureland until 2100 because the temperature changes were relatively small (Figure 2 and Supplementary Figures 5, 9). However, only the Japanese alder had the potential to establish because the mean annual temperature rose by 5°C in 2100 in the RCP 8.5 scenario compared with the current climate (Figure 2 and Supplementary Figures 5, 9).
Ecological Impacts
Figure 3 shows the trends of the three ecological indicators and the RE supply for each scenario.
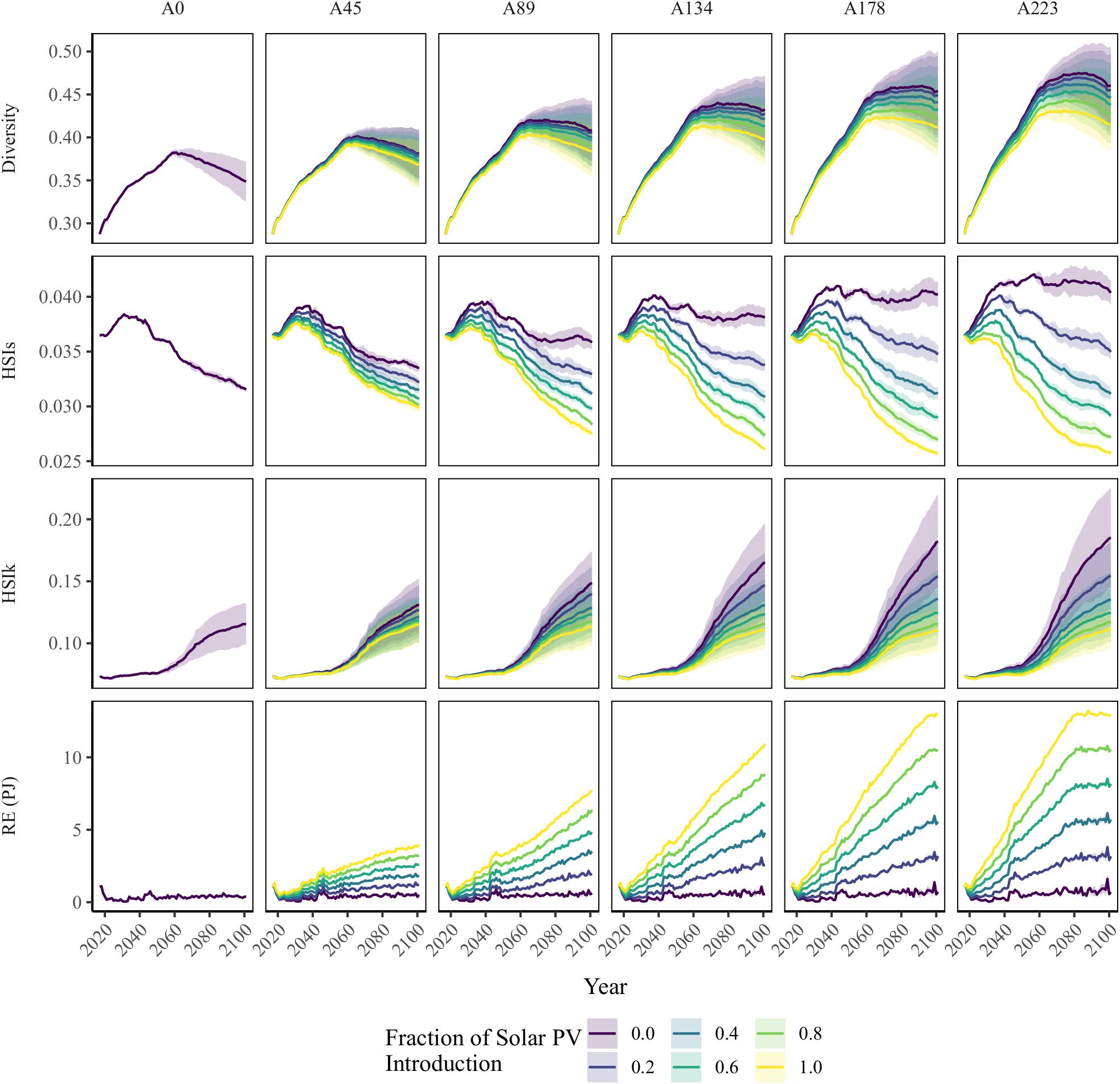
Figure 3. IPCC RCP 2.6 and 8.5 scenarios for the three ecological indicators and renewable energy (RE) production potential from 2016 to 2100 for the 62 scenarios. Each line represents the mean between the two RCP scenarios, and the difference between the scenarios is represented by shading. A0–A223 represent the pastureland abandonment expansion speed, and the colors indicate the solar PV plant fraction (Table 1). Diversity = Shannon–Wiener diversity index, HSIs = habitat suitability index for the mountain hawk-eagle (Spizaetus nipalensis orientalis), HSIk = habitat suitability index for Blakiston’s fish owl (Ketupa blakistoni blakistoni), and RE = the total renewable energy supply from tree biomass utilization and solar PV plants.
In the no pastureland abandonment scenario (A0), selective cutting and planting of broadleaf species increased the Shannon–Wiener diversity index, increasing the mean value within the watershed from 0.04 to 0.08 toward 2100 (Figure 3). After 2060, the diversity index decreased due to the increased occupancy of Japanese alder. The same trend was observed for the A45–A223 scenarios. In the faster pastureland-abandonment expansion speed scenarios, the diversity index linearly increased with the expansion of secondary forest established on the abandoned pastureland. The introduction of solar PV plants decreased the diversity index compared with the same expansion speed scenarios. In the RCP 8.5 scenario, higher temperatures increased the AGB of Japanese alder, and the diversity index was lower than for the RCP 2.6 scenario.
Without pastureland abandonment, the habitat suitability index for the mountain hawk-eagle increased toward 2030 and then decreased to 0.03 by the end of this century because the area of broadleaf forest and mixed forest decreased due to the increase in larch and Sakhalin fir biomass (Figure 3 and Supplementary Figure 8). In the scenarios that used abandoned pastureland to supply only woody biomass (S0.0), the HSIs increased with increasing pastureland abandonment expansion speed. The A223-S0.0 scenario resulted in the highest HSI (0.04) among the RE introduction scenarios in 2100. The HSI decreased with the introduction of solar PV plants compared with scenarios with the same abandonment rate, and the difference in index values between the RCP scenarios was the smallest among the ecological indicators (Figure 3).
The mean of the HSI for the Blakiston’s fish owl (i.e., HSIk) increased from 0.07 to 0.12 toward 2100 in the A0 scenario (Figure 3). In the A45–A223 scenarios, the riparian forest area increased with the expansion of pastureland abandonment and the mean value increased (Figure 3). The higher temperature of the RCP 8.5 scenario increased the AGB of Japanese alder, categorized as natural forest, within 300 m of rivers (Supplementary Figure 7B). The mean HSI value for the owl thus increased, especially, in the RCP 8.5 scenario, however, the greater introduction of solar PV plants increased the area of riparian forests adjacent to the solar panels (Supplementary Figure 8), decreasing the index value (Figure 3).
Renewable Energy Production
The total RE supply in 2100 varied from 3.6 × 10–1 PJ in the A0 scenario to 1.3 × 10 PJ in the A223-S1.0 scenario (Figure 3 and Supplementary Table 10). Tree biomass harvesting from abandoned pastureland emerged around 2040. The amount of harvested AGB fluctuated yearly because the spatial distribution of AGB from abandoned pastureland was affected by seed dispersal from surrounding forests. The amount of energy provided by solar PV plants increased in proportion to the expansion of abandoned pastureland.
Features of the 62 Scenarios
The total energy demand in this watershed was 1.3 PJ in 2010 (Hori et al., 2016). As shown in Figure 3, RE introduction scenarios had the potential to supply 28–998% of the regional energy demand. The 62 scenarios were thus classified into three scenarios: scenario 1, where energy production was less than the energy demand (N = 14); scenario 2, where RE production satisfied the energy demand and was less than five times the demand (N = 29); and scenario 3, where RE production satisfied the energy demand and was more than five times the RE demand (N = 19) (Figure 4 and Supplementary Table 10).
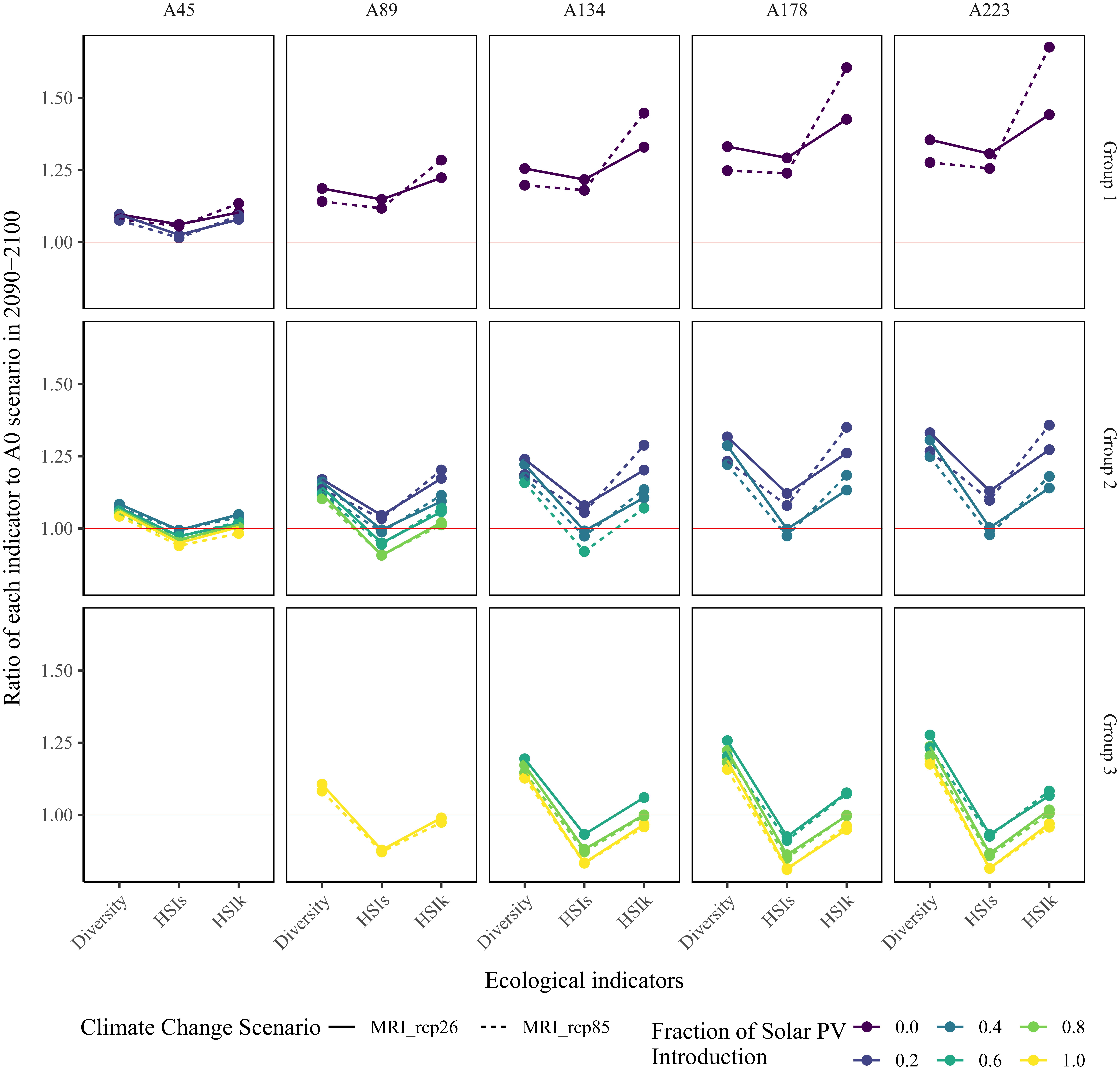
Figure 4. Ratio of each ecological indicator to the no pastureland abandonment scenario (A0) from 2090 to 2100 by pastureland abandonment expansion speed and level of renewable energy production. The red line indicates the same indicator value as the A0 scenario. Values over 1.0 indicate that the ecological indicators are better than the A0 scenario. Three scenario groups were identified based on the balance between renewable energy (RE) supply and demand: group 1, where energy production is less than energy demand; group 2, where RE production satisfies the energy demand and is less than five times the demand; and group 3, where RE production satisfies the energy demand and is more than five times the RE demand. Diversity = Shannon–Wiener diversity index, HSIs = habitat suitability index for the mountain hawk-eagle (Spizaetus nipalensis orientalis), HSIk = habitat suitability index for Blakiston’s fish owl (Ketupa blakistoni blakistoni). A0–A223 represents the pastureland abandonment expansion speed, and the colors indicate the solar PV plant fraction (Table 1).
Group 1 included the scenarios where the solar PV mixture rate was zero (S0.0) and the A45-S0.2 scenario where the area of abandoned pastureland was small and the number of installed solar PV plants was low (Figure 4 and Supplementary Table 10). The amount of RE supply ranged from 3.6 × 10–1 PJ in the A0-S0.0 scenario to 1.3 PJ in the A45-S0.2 scenario (Figure 3). In group 1, all three ecological indicators around 2100 were higher than for the A0 scenario (Figure 4). In group 2, scenarios with a lower solar PV plant fraction (S0.2) maintained the three ecological indicators, whereas the higher fraction (S0.4-1.0) diminished the HSI of the mountain hawk-eagle (Figure 4 and Supplementary Table 10). The habitat suitability of the Blakiston’s fish owl also declined with increasing solar PV plant fraction but was higher than that of the A0 scenario (Figure 4 and Supplementary Table 10). In group 3, the solar PV plant fractions for all scenarios were greater than 60% (Figure 4). The HSI for the mountain hawk-eagle was lower than for the A0 scenario in all scenarios in group 3 (Figure 4). Scenarios that relied on only solar PV plants demonstrated a greater decline in the HSI for the Blakiston’s fish owl than for the A0 scenario (Supplementary Table 10).
Discussion
This study simulated a vegetation succession by considering multiple disturbances, climate change, pastureland abandonment, and two types of RE introduction and visualized the impact on plant species diversity and the habitat suitability of two raptorial birds. The following sections identify the nexuses among these disturbances and the vegetation and wildlife habitat quality and discuss the ecosystem management required to strike a balance between RE introduction and biodiversity conservation.
Climate–Vegetation–Habitat Quality Nexus on Abandoned Pasturelands
The differences in both the establishment probability and seed dispersal ability of the two pioneer species affected the total AGB of Japanese alder from 2050 as the difference in temperatures between the two climatic scenarios became larger (Supplementary Figure 5) and created trade-offs between the plant species diversity indices, the HSI for the Blakiston’s fish owl, and the total biomass of the abandoned pastureland (Figure 2). On the abandoned pasturelands converted for biomass energy production, the Japanese alder biomass also increased in the RCP 8.5 scenario, but the total biomass was lower than for the RCP 2.6 scenario (Supplementary Figure 7A). In these abandoned pasturelands, it became harder for seeds supplied by the Japanese birch that had been distributed near the pasturelands to establish, resulting in a decrease in total biomass (Supplementary Figure 7A). Previous studies have estimated the potential habitat of broadleaf tree species in eastern Asia under future climate change using species distribution models (see, for example, Tanaka et al., 2012; Nakao et al., 2013). To understand nexus among climate change and biodiversity, our results further demonstrate the need to model climate change impacts on multiple species dynamics considering species traits, distribution ability, and spatial distributions of plant species at finer scales.
Previous studies have shown that the effects of climate change become more significant and uncertain toward the end of this century (Lucash et al., 2019; Ohashi et al., 2019), and our results are also consistent with this finding (Figure 3). Moreover, pioneer species dynamics were dominant in this study, suggesting the need for longer-term simulations beyond 2100. Although governments and international organizations often develop future social scenario narratives for toward 2030 or 2050 (United Nations, 2015; Predicting and Assessing Natural Capital and Ecosystem Services [PANCES], 2016; IPBES, 2020), our work implies that longer-term future vision and ecosystem management plans are required for pre-emptive actions.
Pastureland Abandonment–Renewable Energy–Raptorial Bird Habitat Nexus
Pastureland abandonment led to the expansion of mixed forests of broadleaf and conifer at landscape scales and, thus, increased the diversity and habitat suitability of the two raptorial birds (Figure 3). However, the decrease in the area of managed pastureland caused the loss of open areas and forest edge, which are used by the mountain hawk-eagle. For this reason, the HSI for the mountain hawk-eagle diminished or remained the same after 2030 (Figure 3 and Supplementary Figure 8). In particular, the change of pastureland to solar PV plants reduced the open area and length of forest edge suitable for foraging environments (Supplementary Figure 8). As societies shrink, local managers need to select important pasturelands and maintain a mosaic of different ecosystems on them to conserve biodiversity hotspots.
Solar PV plants were located away from forests and more than 300 m from riversides to conserve the foraging environment of the mountain hawk-eagle and the nesting sites of the Blakiston’s fish owl. Therefore, in scenarios with the small fractions of solar PV plants, the three ecological indicators were improved compared with the A0 scenario, which maintained the current land use (Figure 4). These indicators were improved because the contribution of transitional forests on abandoned pastureland exceeded the ecological impacts associated with solar PV installation at the landscape scale. Our results suggest that a better understanding of the nexus between pastureland abandonment, the introduction of multiple RE sources, and raptorial bird habitat would enable land managers to design local RE energy implementations to mitigate ecological impacts.
Renewable Energy–Biodiversity Nexus
By integrating the previous studies on RE mixes, spatially explicit ecological impact assessment, and process-based landscape modeling, this study has explored scenarios to meet both RE utilization and biodiversity conservation on an energy demand basis that is of interest to local stakeholders. Scenario 1 converted 80–100% of abandoned pastureland into biomass energy production, supplied 28–80% of the current regional energy demand, and improved the three ecosystem indicators. The residential population in the watershed is estimated to decrease to 4,118 by 2050 (48% of 2010) (National Land Numerical Information [NLNI], 2017). Considering that the energy demand will decrease from the current level as the population declines, group 1 could fulfill the regional energy demand, however, depending only on biomass energy would result in annual energy supply fluctuations (Figure 3). Therefore, mixing with other RE, as in the scenario of A45-S0.2, is recommended. In group 3, where the energy production far exceeded the current demand, more than 60% of abandoned pasture was converted to solar PV plant installation (Figure 4). The habitat suitability indices for the two raptorial birds, especially the mountain hawk-eagle, diminished compared with the A0 scenario, which maintained the current land use (Figure 4). In contrast, group 2, where the energy production and the current demand were almost of the same order, promotes the mixed use of solar PV plants and biomass energy. Group 2 produced more energy than the local demand and has the potential to supply energy to surrounding areas under a shrinking society. Concurrently, this mixed use of the two RE sources minimized the impact on the two habitat suitability indices (Figure 4). Therefore, both (1) combining multiple renewable energy sources and (2) arranging the spatial distribution of solar PV plant installations to avoid habitat degradation are essential for the production of sufficient energy while suppressing the impact on the ecosystem, as in groups 1 and 2. A participatory approach supported by scientific evidence can be effective in developing local future energy visions (Belmonte et al., 2015; Hori et al., 2019). Because our approach explicitly addresses not only ecological status but also energy supply potentials, it is useful for designing desirable future visions with different stakeholders to explore future visions that satisfy RE utilization and biodiversity conservation.
Conclusion and Future Perspectives
We identified nexuses between climate, vegetation, the habitat of the two raptorial bird species, and energy and successfully explored three future scenario groups: group 1 that improved plant species diversity and the habitat of the two raptorial bird species with less energy production than the current regional demand, group 2 that supplied sufficient energy production from the mixed use of woody biomass and solar PV systems while minimizing impacts on the three ecological indicators, and group 3 that resulted in high dependency on solar PV systems and the diminished habitat of the two raptorial bird species. Our quantitative modeling provides scientific information about energy–biodiversity nexuses for local stakeholder meetings, which contribute to developing land use and energy strategies.
To promote the use of quantitative forest landscape modeling to other areas, such as the Asia Pacific region, a reliable species parameter database is required for robust simulations. Our study suggests that the need for such a dataset is required, especially, for pioneer species. Currently, large accumulated datasets of traits (e.g., TRY, 2020) and occurrence maps of plant species (see, for example, GBIF, 2020; Tanaka and Matsui, 2007; Forestry Agency of Japan, 2019; Ministry of the Environment, Japan [MOE], 2020; Long Term Ecological Research [LTER], 2020) enable us to systematically prepare the standard parameters required for forest landscape simulation models. These standard datasets facilitate forest model intercomparison practice (Erickson and Strigul, 2019) and reduce uncertainties when simulating the vegetation dynamics of region-specific tree species under climate change.
Our results underestimate the effects of natural disturbance regimes, such as deer browsing and windthrow. In Japan, Sasa species play a key role in regeneration dynamics by preventing the establishment of other plant species. Because the vegetation map and forest registers used in this study lack detailed spatial distribution information of Sasa species, it is necessary to estimate its initial density from the overstory tree density (Tatsumi and Owari, 2013). Climate change and deer browsing affect the survival of Sasa species (Yokoyama and Shibata, 1998; Tsuyama et al., 2011, 2012). More frequent windthrow events and post-windthrow management under future climate will affect species composition and carbon dynamics (Lucash et al., 2019; Morimoto et al., 2019; Hotta et al., 2020). Therefore, integrative modeling of the nexus between (1) natural disturbances at a broader scale, (2) the distribution of Sasa species, and (3) regeneration dynamics under future climate change is a fundamental future perspective.
Recently, SSPs scenarios (O’Neill et al., 2014) have been developed in the climate change domain, and research is progressing to downscale global-scale scenarios to national scales (Frame et al., 2018; Guo et al., 2019; Chen et al., 2020). IPBES is also developing a Nature Futures Framework as a unified future scenario from the aspect of biodiversity conservation (PBL, 2018; IPBES, 2020). Local scenario analysis is thus expected to couple seamlessly with socio-economic conditions provided by such external scenarios. Through the modeling of the nexus between SDG goals 13 (Climate action) and 15 (Life on land), to which forest landscape modeling can contribute, nexus structures are expected to be identified for people, the planet, prosperity, peace, and partnership in collaboration with other research fields.
Data Availability Statement
Input data and analysis scripts have deposited in our GitHub repository: https://github.com/hagachi/Project-RE-biodiversity- nexus-2020. The species parameters and calibration procedure can be found in the Supplementary Material. Redistribution of the forest registers, which were used for initializing landscapes, are restricted by the Forest Management Bureau of Hokkaido Prefecture. Requests to access the datasets should be directed to the bureau: https://www.rinya.maff.go.jp/hokkaido/keikaku/map/map.html (in Japanese).
Author Contributions
CH, MM, TMt, and TMc contributed the conception, design, and analysis. WH, TI, and JM organized the vegetation and environmental dataset. CH, TI, and HS contributed the localization of forest landscape model. MN arranged the field survey. SH and OS provided the ideas and discussion for the design scenarios. CH and MM wrote the first draft of the manuscript. All authors contributed to the revision of the manuscript. CH and TMt finalized the submitted manuscript with input from all the authors.
Funding
This research was funded by the Environment Research and Technology Development Fund (S-15 “Predicting and Assessing Natural Capital and Ecosystem Services” (PANCES), Ministry of the Environment, Japan); JSPS KAKENHI Grant Numbers JP18J20266 and JP17H01516 and Grant Program for Doctoral Course Students from Sompo Environment Foundation.
Conflict of Interest
The authors declare that the research was conducted in the absence of any commercial or financial relationships that could be construed as a potential conflict of interest.
Acknowledgments
The data for the 1920s distribution of wetlands was provided by Dr. Masami Kaneko, Rakuno Gakuen University, Japan. This research contributes to Japan Long Term Ecological Research (JaLTER) and the International Long Term Ecological Research Network (ILTER). The authors would like to thank Enago (www.enago.jp) for their English language review.
Supplementary Material
The Supplementary Material for this article can be found online at: https://www.frontiersin.org/articles/10.3389/fevo.2020.00155/full#supplementary-material
References
Abbasi, S. A., and Abbasi, N. (2000). The likely adverse environmental impacts of renewable energy sources. Appl. Energy 65, 121–144. doi: 10.1016/S0306-2619(99)00077-X
Akkeshi town (2017). Forest Management Plan in Akkeshi Town (in Japanese). Available online at https://www.akkeshi-town.jp/file/contents/302/5279/h29-39shinrinseibikeikaku.pdf (accessed March 23, 2020).
Akkeshi town (2018a). Call for Proposals for the Project for Establishing a Resource Recycling-type Woody Biomass Utilization Plan to Connect Primary Industries in Akkeshi (in Japanese). Available online at https://www.akkeshi-town.jp/oshirase/1835/ (accessed March 23, 2020).
Akkeshi town (2018b). Statistics of Akkeshi Town 2017 (in Japanese). Available online at: https://www.akkeshi-town.jp/gyosei/tokei/tokeisho/ (accessed March 23, 2020).
Akkeshi town (2018c). Wildlife Damage Prevention Plan in Akkeshi Town (in Japanese). Available online at: https://www.akkeshi-town.jp/file/contents/287/5198/2019-2021chojukeikaku.pdf (accessed March 23, 2020).
Akkeshi town (2019a). Bekambeushi Watershed. Available online at: https://www.akkeshi-town.jp/kanko/kanko10/bekannbeushi/ (accessed March 23, 2020).
Akkeshi town (2019b). Self-Reliance Promotion Project in Akkeshi Town (in Japanese). Available online at: https://www.akkeshi-town.jp/file/contents/292/5223/h29-R02kasokeikaku.pdf (accessed March 23, 2020).
Belmonte, S., Escalante, K. N., and Franco, J. (2015). Shaping changes through participatory processes: local development and renewable energy in rural habitats. Renew. Sustain. Energy Rev. 45, 278–289. doi: 10.1016/j.rser.2015.01.038
Biodiversity Center of Japan (2017). GIS Data of 1:25,000 Scale Vegetation Map. Available online at http://gis.biodic.go.jp/webgis/ (accessed March 23, 2020).
Cabinet Office, Government of Japan (2016). Global Warming Countermeasures Plan (in Japanese). Available online at: https://www.env.go.jp/press/files/jp/102816.pdf (accessed March 23, 2020).
Cantarello, E., Newton, A. C., Martin, P. A., Evans, P. M., Gosal, A., and Lucash, M. S. (2017). Quantifying resilience of multiple ecosystem services and biodiversity in a temperate forest landscape. Ecol. Evol. 7, 9661–9675. doi: 10.1002/ece3.3491
Chen, H., Matsuhashi, K., Takahashi, K., Fujimori, S., Honjo, K., and Gomi, K. (2020). Adapting global shared socio-economic pathways for national scenarios in Japan. Sustain. Sci. 15, 985–1000. doi: 10.1007/s11625-019-00780-y
Costanza, J. K., Abt, R. C., McKerrow, A. J., and Collazo, J. A. (2015). Linking state-and-transition simulation and timber supply models for forest biomass production scenarios. AIMS Environ. Sci. 2:180. doi: 10.3934/environsci.2015.2.180
Creutzburg, M. K., Scheller, R. M., Lucash, M. S., Evers, L. B., LeDuc, S. D., and Johnson, M. G. (2016). Bioenergy harvest, climate change, and forest carbon in the Oregon Coast Range. GCB Bioenergy 8, 357–370. doi: 10.1111/gcbb.12255
de Bruijn, A., Gustafson, E. J., Sturtevant, B. R., Foster, J. R., Miranda, B. R., Lichti, N. I., et al. (2014). Toward more robust projections of forest landscape dynamics under novel environmental conditions: embedding PnET within LANDIS-II. Ecol. Modell. 287, 44–57. doi: 10.1016/j.ecolmodel.2014.05.004
de Vries, B. J. M., van Vuuren, D. P., and Hoogwijk, M. M. (2007). Renewable energy sources: their global potential for the first-half of the 21st century at a global level: an integrated approach. Energy Policy 35, 2590–2610. doi: 10.1016/j.enpol.2006.09.002
Duden, A. S., Verweij, P. A., Junginger, H. M., Abt, R. C., Henderson, J. D., Dale, V. H., et al. (2017). Modeling the impacts of wood pellet demand on forest dynamics in southeastern United States. Biofuels Bioprod. Biorefin. 11, 1007–1029. doi: 10.1002/bbb.1803
Duveneck, M. J., and Thompson, J. R. (2019). Social and biophysical determinants of future forest conditions in New England: effects of a modern land-use regime. Glob. Environ. Change 55, 115–129. doi: 10.1016/j.gloenvcha.2019.01.009
Erickson, A., and Strigul, N. (2019). A forest model intercomparison framework and application at two temperate forests along the east coast of the United States. Forests 10:180. doi: 10.3390/f10020180
Esgf-CoG (2017). ESGF-CoG: CMIP-5. Available online at: https://esgf-node.llnl.gov/search/cmip5/ (accessed March 23, 2020).
Field, C. B., Campbell, J. E., and Lobell, D. B. (2008). Biomass energy: the scale of the potential resource. Trends Ecol. Evol. 23, 65–72. doi: 10.1016/j.tree.2007.12.001
Forestry Agency of Japan (2019). Forest Ecosystem Diversity Basic Survey (in Japanese). Available online at: https://www.rinya.maff.go.jp/j/keikaku/tayouseichousa/index.html (accessed March 23, 2020).
Frame, B., Lawrence, J., Ausseil, A.-G., Reisinger, A., and Daigneault, A. (2018). Adapting global shared socio-economic pathways for national and local scenarios. Clim. Risk Manage. 21, 39–51. doi: 10.1016/j.crm.2018.05.001
Gasparatos, A., Doll, C. N. H., Esteban, M., Ahmed, A., and Olang, T. A. (2017). Renewable energy and biodiversity: Implications for transitioning to a Green Economy. Renew. Sustain. Energy Rev. 70, 161–184. doi: 10.1016/j.rser.2016.08.030
GBIF (2020). Global Biodiversity Information Facility. Available online at: https://www.gbif.org/ (accessed March 23, 2020).
Geosptial Information Authority of Japan [GSI] (2019). Degital Elevation Model in 10 m Resolution. Available online at: https://fgd.gsi.go.jp/download/menu.php (accessed March 23, 2020).
Gibson, L., Wilman, E. N., and Laurance, W. F. (2017). How green is ‘green’ energy? Trends Ecol. Evol. 32, 922–935. doi: 10.1016/j.tree.2017.09.007
Guo, A., Ding, X., Zhong, F., Cheng, Q., and Huang, C. (2019). Predicting the future chinese population using shared socioeconomic pathways, the sixth national population census, and a PDE model. Sustainability 11:3686. doi: 10.3390/su11133686
Gustafson, E. J., Bruijn, A. M. G. D., Pangle, R. E., Limousin, J.-M., McDowell, N. G., Pockman, W. T., et al. (2015). Integrating ecophysiology and forest landscape models to improve projections of drought effects under climate change. Global Change Biol. 21, 843–856. doi: 10.1111/gcb.12713
Haga, C., Inoue, T., Hotta, W., Shibata, R., Hashimoto, S., Kurokawa, H., et al. (2018). Simulation of natural capital and ecosystem services in a watershed in Northern Japan focusing on the future underuse of nature: by linking forest landscape model and social scenarios. Sustain. Sci. 14, 89–106. doi: 10.1007/s11625-018-0623-9
Harper, A. B., Powell, T., Cox, P. M., House, J., Huntingford, C., Lenton, T. M., et al. (2018). Land-use emissions play a critical role in land-based mitigation for Paris climate targets. Nat. Commun. 9:2938. doi: 10.1038/s41467-018-05340-z
Hof, C., Voskamp, A., Biber, M. F., Böhning-Gaese, K., Engelhardt, E. K., Niamir, A., et al. (2018). Bioenergy cropland expansion may offset positive effects of climate change mitigation for global vertebrate diversity. Proc. Natl. Acad. Sci. U.S.A. 115, 13294–13299. doi: 10.1073/pnas.1807745115
Hokkaido National Agricultural Experiment Station (1983). Soil Survey of Kushiro Sub Prefecture and Nemuro city (in Japanese). Available online at: http://www.naro.affrc.go.jp/harc/dosei/026087.html (accessed March 23, 2020).
Hokkaido Prefecture (2017a). Forest Register for Prefectural Forest (in Japanese). Available online at: http://www.pref.hokkaido.lg.jp/sr/dyr/DOP.htm (accessed March 23, 2020).
Hokkaido Prefecture (2017b). Forest Register for Private Forest (in Japanese). Available online at: http://www.pref.hokkaido.lg.jp/sr/srk/OPD.htm (accessed March 23, 2020).
Hokkaido Prefecture (2017c). Regional Forest Plan in Kushiro Nemuro (in Japanese). Available online at: http://www.pref.hokkaido.lg.jp/sr/srk/sinrin/02-03keikakusyotokekomi.htm (accessed March 23, 2020).
Hokkaido Prefecture (2019). Statistics of Forestry in Hokkaido Prefecture (in Japanese). Available online at: http://www.pref.hokkaido.lg.jp/sr/sum/kcs/rin-toukei/rin-toukei-index.htm (accessed March 23, 2020).
Hori, K., Kim, J., Kawase, R., Kimura, M., Matsui, T., and Machimura, T. (2019). Local energy system design support using a renewable energy mix multi-objective optimization model and a co-creative optimization process Renew. Energy (in press). doi: 10.1016/j.renene.2019.11.089
Hori, K., Matsui, T., Hasuike, T., Fukui, K., and Machimura, T. (2016). Development and application of the renewable energy regional optimization utility tool for environmental sustainability: REROUTES. Renew. Energy 93, 548–561. doi: 10.1016/j.renene.2016.02.051
Hotta, W., Morimoto, J., Inoue, T., Suzuki, S. N., Umebayashi, T., Owari, T., et al. (2020). Recovery and allocation of carbon stocks in boreal forests 64 years after catastrophic windthrow and salvage logging in northern Japan. For. Ecol. Manage. 468:118169. doi: 10.1016/j.foreco.2020.118169
IPBES (2019). Summary for Policymakers of the Global Assessment Report on biodiversity and Ecosystem Services of the Intergovernmental Science-Policy Platform on Biodiversity and Ecosystem Services, eds S. Díaz, J. Settele, E. S. Brondízio, H. T. Ngo, M. Guèze, J. Agard, et al. Bonn: IPBES secretariat, 56.
IPBES (2020). Building on the Scenarios and Models Assessment. Available online at: https://ipbes.net/scenarios-phase-2-activities (accessed March 23, 2020).
IPCC (2013). Climate Change 2013: The Physical Science Basis. Contribution of Working Group I to the Fifth Assessment Report of the Intergovern-mental Panel on Climate Change, eds T.F. Stocker, D. Qin, G.-K. Plattner, M. Tignor, S.K. Allen, J. Boschung, A. Nauels, Y. Xia, V. Bex, and P.M. Midgley Cambridge: Cambridge University Press, 1535.
IPCC (2014). Climate Change 2014: Synthesis Report. Contribution of Working Groups I, II and III to the Fifth Report Intergovernmental Panel on Climate Change, eds Core Writing Team, R.K. Pachauri and L.A. Meyer Geneva: IPCC, 151.
IPCC (2018). Global Warming of 1.5°C. An IPCC Special Report on the Impacts of Global Warming of 1.5°C Above Pre-industrial Levels and Related Global Greenhouse Gas Emission Pathways, in the Context of Strengthening the Global Response to the Threat of Climate Change, Sustainable Development, and Efforts to Eradicate Poverty, eds V. Masson-Delmotte, V. P. Zhai, H.-O. Pörtner, D. Roberts, J. Skea, P.R. Shukla, et al. Geneva: IPCC.
Itoh, F., Nagasawa, R., and Hioki, Y. (2012). Estimation of the potential habitat of Mountain Hawk-Eagle (Spizaetus nipalensis) and examination about the habitat conservation in Tottori prefecture using GIS. Landsc. Ecol. Manage. 17, 7–17. doi: 10.5738/jale.17.7
Japan Meteorological Agency (2018). Past Climate Data (in Japanese). Available online at: http://www.data.jma.go.jp/gmd/risk/obsdl/index.php (accessed March 23, 2020).
Japan Wood Pellet Association (2017). Wood Pellet Quality Standards (in Japanese). Available online at: https://w-pellet.org/hinshitsu-2/ (accessed March 23, 2020).
Kaneko, M., Suzuki, T., Nakatani, Y., and Ono, T. (2008). Estimation of Wetland Area Using Multi-Temporal Topographical Map. Kyoto: GIS association of Japan, 17.
Keane, R. E., McKenzie, D., Falk, D. A., Smithwick, E. A. H., Miller, C., and Kellogg, L.-K. B. (2015). Representing climate, disturbance, and vegetation interactions in landscape models. Ecol. Modell. 30, 33–47. doi: 10.1016/j.ecolmodel.2015.04.009
Kienast, F., Huber, N., Hergert, R., Bolliger, J., Moran, L. S., and Hersperger, A. M. (2017). Conflicts between decentralized renewable electricity production and landscape services – A spatially-explicit quantitative assessment for Switzerland. Renew. Sustain. Energy Rev. 67, 397–407. doi: 10.1016/j.rser.2016.09.045
Kitazawa, M., Yamaura, Y., Senzaki, M., Kawamura, K., Hanioka, M., and Nakamura, F. (2019). An evaluation of five agricultural habitat types for openland birds: abandoned farmland can have comparative values to undisturbed wetland. Ornithol. Sci. 18, 3–16. doi: 10.2326/osj.18.3
Kobayashi, Y., and Nakamura, F. (2018). “The possibility of using abandoned farmlands for habitat restoration in societies with decreasing populations,” in Biodiversity Conservation Using Umbrella Species: Blakiston’s Fish Owl and the Red-crowned Crane Ecological Research Monographs, ed. F. Nakamura (Singapore: Springer), 185–196. doi: 10.1007/978-981-10-7203-1_13
Laranjeiro, T., May, R., and Verones, F. (2018). Impacts of onshore wind energy production on birds and bats: recommendations for future life cycle impact assessment developments. Int. J. Life Cycle Assess. 23, 2007–2023. doi: 10.1007/s11367-017-1434-4
Long Term Ecological Research [LTER] (2020). LTER (website). Available online at: https://lternet.edu/ (accessed March 23, 2020).
Lucash, M. S., Ruckert, K. L., Nicholas, R. E., Scheller, R. M., and Smithwick, E. A. H. (2019). Complex interactions among successional trajectories and climate govern spatial resilience after severe windstorms in central Wisconsin, USA. Landsc. Ecol. 34, 2897–2915. doi: 10.1007/s10980-019-00929-1
Matsui, T., Haga, C., Saito, O., and Hashimoto, S. (2019). Spatially explicit residential and working population assumptions for projecting and assessing natural capital and ecosystem services in Japan. Sustain Sci. 14, 23–37. doi: 10.1007/s11625-018-0605-y
Nikkei XTECH (2018). 8 Regional Banks to Finance 11.5 Billion yen for the Mega-Solar Power Plant in Akkeshi Town, Hokkaido (in Japanese). Available online at: https://xtech.nikkei.com/dm/atcl/news/16/031910894/?ST=msb (accessed March 23 2020).
Millennium Ecosystem Assessment [MA] (2005). Ecosystem and Human Well-Being – Summary for Decision Makers. Washington, DC: Island Press.
Ministry of Agriculture Forestry and Fisheries [MAFF] (2017). Forest Register for National Forest (in Japanese). Tokyo: Ministry of Agriculture Forestry and Fisheries [MAFF].
Ministry of the Environment, Japan [MOE] (2010). Report on the Potential for Renewable Energy Introduction (in Japanese). Available online at: https://www.env.go.jp/earth/report/h23-03/ (accessed March 23, 2020).
Ministry of the Environment, Japan [MOE] (2012). How to Protect Birds of Prey (revised edition) (in Japanese). Available online at: https://www.env.go.jp/press/files/jp/22992.pdf (accessed March 23, 2020).
Ministry of the Environment, Japan [MOE] (2018). The Fifth Environmental Basic Plan (in Japanese). Available online at: https://www.env.go.jp/press/files/jp/108982.pdf (accessed March 23, 2020).
Ministry of the Environment, Japan [MOE] (2019a). Long-Term Strategy as a Growth Strategy Based on the Paris Agreement (in Japanese). Available online at: https://www.env.go.jp/press/106869.html (accessed March 23, 2020).
Ministry of the Environment, Japan [MOE] (2019b). National Greenhouse Gas Inventory Report of JAPAN. Available online at: http://www-gio.nies.go.jp/aboutghg/nir/2019/NIR-JPN-2019-v3.0_GIOweb.pdf (accessed March 23, 2020).
Ministry of the Environment, Japan [MOE] (2019c). Red List 2019. Available online at: http://www.env.go.jp/press/106383.html (accessed March 23, 2020).
Ministry of the Environment, Japan [MOE] (2020). Monitoring site 1000 (in Japanese). Available online at: http://www.biodic.go.jp/moni1000/ (accessed March 23, 2020).
Moore-O’Leary, K. A., Hernandez, R. R., Johnston, D. S., Abella, S. R., Tanner, K. E., Swanson, A. C., et al. (2017). Sustainability of utility-scale solar energy – critical ecological concepts. Front. Ecol. Environ. 15:385–394. doi: 10.1002/fee.1517
Morimoto, J., Shibata, M., Shida, Y., and Nakamura, F. (2017). Wetland restoration by natural succession in abandoned pastures with a degraded soil seed bank. Restor. Ecol. 25, 1005–1014. doi: 10.1111/rec.12516
Morimoto, J., Umebayashi, T., Suzuki, S. N., Owari, T., Nishimura, N., Ishibashi, S., et al. (2019). Long-term effects of salvage logging after a catastrophic wind disturbance on forest structure in northern Japan. Landsc. Ecol. Eng. 15, 133–141. doi: 10.1007/s11355-019-00375-w
Nakao, K., Higa, M., Tsuyama, I., Matsui, T., Horikawa, M., and Tanaka, N. (2013). Spatial conservation planning under climate change: using species distribution modeling to assess priority for adaptive management of Fagus crenata in Japan. J. Nat. Conserv. 21, 406–413. doi: 10.1016/j.jnc.2013.06.003
Nakaoka, M., Sudo, K., Namba, M., Shibata, H., Nakamura, F., Ishikawa, S., et al. (2018). TSUNAGARI: a new interdisciplinary and transdisciplinary study toward conservation and sustainable use of biodiversity and ecosystem services. Ecol. Res. 33, 35–49. doi: 10.1007/s11284-017-1534-4
National Institute of Population and Social Security Research [NIPSSR] (2018). Regional Population Projections for Japan: 2015-2045 (in Japanese). Available online at: http://www.ipss.go.jp/pp-shicyoson/e/shicyoson18/t-page.asp (accessed March 23, 2020).
National Land Numerical Information [NLNI] (2009). River Data (in Japanese). Available online at: http://nlftp.mlit.go.jp/ksj/gml/datalist/KsjTmplt-W05.html (accessed March 23, 2020).
National Land Numerical Information [NLNI] (2012). Climate Data at 1 km Mesh. Available online at: http://nlftp.mlit.go.jp/ksj/gml/datalist/KsjTmplt-G02.html (accessed March 23, 2020).
National Land Numerical Information [NLNI] (2017). Future population Estimation at 1 km Mesh (in Japanese). Available online at: http://nlftp.mlit.go.jp/ksj/gml/datalist/KsjTmplt-mesh1000.html (accessed March 23, 2020).
Obara, H., Takata, Y., Kohyama, K., Ohkura, T., Maejima, Y., Watabayashi, S., et al. (2016). A new soil map of japan based on comprehensive soil classificationsystem of japan first approximation. Bull. Nat. Inst. Agro-Environ. Sci. 37, 133–148.
Ohashi, H., Hasegawa, T., Hirata, A., Fujimori, S., Takahashi, K., Tsuyama, I., et al. (2019). Biodiversity can benefit from climate stabilization despite adverse side effects of land-based mitigation. Nat. Commun. 10, 1–11. doi: 10.1038/s41467-019-13241-y
O’Neill, B. C., Kriegler, E., Riahi, K., Ebi, K. L., Hallegatte, S., Carter, T. R., et al. (2014). A new scenario framework for climate change research: the concept of shared socioeconomic pathways. Clim. Change 122, 387–400. doi: 10.1007/s10584-013-0905-2
Palomo, I., Dujardin, Y., Midler, E., Robin, M., Sanz, M. J., and Pascual, U. (2019). Modeling trade-offs across carbon sequestration, biodiversity conservation, and equity in the distribution of global REDD+ funds. Proc. Natl. Acad. Sci. U.S.A. 116, 22645–22650. doi: 10.1073/pnas.1908683116
PBL (2018). Report on the Workshop ‘Next Steps in Developing Nature Futures’. The Hague: PBL Netherlands Environmental Assessment Agency.
Pogson, M., Hastings, A., and Smith, P. (2013). How does bioenergy compare with other land-based renewable energy sources globally? GCB Bioenergy 5, 513–524. doi: 10.1111/gcbb.12013
Predicting and Assessing Natural Capital and Ecosystem Services [PANCES] (2016). Predicting and Assessing Natural Capital and Ecosystem Services (PANCES)through an Integrated Social-Ecological Systems Approach project (2016) Research Achievements. Available online at: http://pances.net/eng/ (accessed March 23, 2020).
R Core Team (2019). R: A Language and Environment for Statistical Computing. Vienna: R Foundation for Statistical Computing. Available online at: https://www.R-project.org/ (accessed March 23, 2020).
Raoux, A., Dambacher, J. M., Pezy, J.-P., Maze, C., Dauvin, J.-C., and Niquil, N. (2018). Assessing cumulative socio-ecological impacts of offshore wind farm development in the Bay of Seine (English Channel). Mar. Policy 89, 11–20. doi: 10.1016/j.marpol.2017.12.007
Resco de Dios, V., Yoshida, T., and Iga, Y. (2005). Effects of topsoil removal by soil-scarification on regeneration dynamics of mixed forests in Hokkaido, Northern Japan. For. Ecol. Manage. 215, 138–148. doi: 10.1016/j.foreco.2005.05.010
Saito, O., Kamiyama, C., Hashimoto, S., Matsui, T., Shoyama, K., Kabaya, K., et al. (2018). Co-design of national-scale future scenarios in Japan to predict and assess natural capital and ecosystem services. Sustain. Sci. 14, 5–21. doi: 10.1007/s11625-018-0587-9
Santangeli, A., Toivonen, T., Pouzols, F. M., Pogson, M., Hastings, A., Smith, P., et al. (2016a). Global change synergies and trade-offs between renewable energy and biodiversity. GCB Bioenergy 8, 941–951. doi: 10.1111/gcbb.12299
Santangeli, A., Minin, E. D., Toivonen, T., Pogson, M., Hastings, A., Smith, P., et al. (2016b). Synergies and trade-offs between renewable energy expansion and biodiversity conservation – a cross-national multifactor analysis. GCB Bioenergy 8, 1191–1200. doi: 10.1111/gcbb.12337
Scheller, R. M., Domingo, J. B., Sturtevant, B. R., Williams, J. S., Rudy, A., Gustafson, E. J., et al. (2007). Design, development, and application of LANDIS-II, a spatial landscape simulation model with flexible temporal and spatial resolution. Ecol. Modell. 201, 409–419. doi: 10.1016/j.ecolmodel.2006.10.009
Scheller, R. M., Hua, D., Bolstad, P. V., Birdsey, R. A., and Mladenoff, D. J. (2011). The effects of forest harvest intensity in combination with wind disturbance on carbon dynamics in Lake States Mesic Forests. Ecol. Modell. 222, 144–153.
Shifley, S. R., He, H. S., Lischke, H., Wang, W. J., Jin, W., Gustafson, E. J., et al. (2017). The past and future of modeling forest dynamics: from growth and yield curves to forest landscape models. Landsc. Ecol. 32, 1307–1325. doi: 10.1007/s10980-017-0540-9
Shoyama, K., Matsui, T., Hashimoto, S., Kabaya, K., Oono, A., and Saito, O. (2018). Development of land-use scenarios using vegetation inventories in Japan. Sustain. Sci. 14, 39–52. doi: 10.1007/s11625-018-0617-7
Sil, Â, Fernandes, P. M., Rodrigues, A. P., Alonso, J. M., Honrado, J. P., Perera, A., et al. (2019). Farmland abandonment decreases the fire regulation capacity and the fire protection ecosystem service in mountain landscapes. Ecosyst. Serv. 36:100908. doi: 10.1016/j.ecoser.2019.100908
Takenaka, T. (2018). “Ecology and conservation of blakiston’s fish owl in Japan,” in Biodiversity Conservation Using Umbrella Species: Blakiston’s Fish Owl and the Red-crowned Crane Ecological Research Monographs, ed. F. Nakamura (Singapore: Springer), 19–46. doi: 10.1007/978-981-10-7203-1_3
Tanaka, N., and Matsui, T. (2007). PRDB Phytosociological Relevé Database, Forestry and Forest Products Research Institute. Available online at: http://www.ffpri.affrc.go.jp/labs/prdb/index.html (accessed March 23, 2020).
Tanaka, N., Nakao, K., Tsuyama, I., Higa, M., Nakazono, E., and Matsui, T. (2012). Predicting the impact of climate change on potential habitats of fir (Abies) species in Japan and on the East Asian continent. Procedia Environ. Sci. 13, 455–466. doi: 10.1016/j.proenv.2012.01.039
Tarr, N. M., Rubino, M. J., Costanza, J. K., McKerrow, A. J., Collazo, J. A., and Abt, R. C. (2017). Projected gains and losses of wildlife habitat from bioenergy-induced landscape change. GCB Bioenergy 9, 909–923. doi: 10.1111/gcbb.12383
Tatebayashi, K., Matsui, T., Ooba, M., Machimura, T., Tani, Y., Nakao, A., et al. (2015). A development of optimization model of timber production and utilization system for reduction of carbon in areas. J. Jpn. Soc. Civ. Eng. Ser. G (Environ. Res.) 71, II_297–II_308. doi: 10.2208/jscejer.71.II_297
Tatsumi, S., and Owari, T. (2013). Modeling the effects of individual-tree size, distance, and species on understory vegetation based on neighborhood analysis. Can. J. For. Res. 43, 1006–1014.
Thompson, J. R., Lambert, K. F., Foster, D. R., Broadbent, E. N., Blumstein, M., Zambrano, A. M. A., et al. (2016). The consequences of four land-use scenarios for forest ecosystems and the services they provide. Ecosphere 7:e01469. doi: 10.1002/ecs2.1469
TRY (2020). Plant Trait Database. Available online at: https://www.try-db.org/TryWeb/Home.php (accessed March 23, 2020).
Tsuyama, I., Horikawa, M., Nakao, K., Matsui, T., Kominami, Y., and Tanaka, N. (2012). Factors determining the distribution of a keystone understory taxon, dwarf bamboo of the section Crassinodi, on a national scale: application to impact assessment of climate change in Japan. J. For. Res. 17, 137–148. doi: 10.1007/s10310-011-0283-4
Tsuyama, I., Nakao, K., Matsui, T., Higa, M., Horikawa, M., Kominami, Y., et al. (2011). Climatic controls of a keystone understory species, Sasamorpha borealis, and an impact assessment of climate change in Japan. Ann. For. Sci. 68, 689–699. doi: 10.1007/s13595-011-0086-y
UNFCCC (2019). NDC Registry. Available online at: https://www4.unfccc.int/sites/NDCStaging/Pages/All.aspx (accessed March 23, 2020).
United Nations (2015). The Sustainable Development Goals. Available online at: www.un.org/sustainabledevelopment/sustainable-development-goals/ (accessed March 23, 2020).
Walston, L. J., Rollins, K. E., LaGory, K. E., Smith, K. P., and Meyers, S. A. (2016). A preliminary assessment of avian mortality at utility-scale solar energy facilities in the United States. Renew. Energy 92, 405–414. doi: 10.1016/j.renene.2016.02.041
Ward, B. C., Mladenoff, D. J., and Scheller, R. M. (2005). Simulating landscape-level effects of constraints to public forest regeneration harvests due to adjacent residential development in northern wisconsin. For. Sci. 51, 616–632. doi: 10.1093/forestscience/51.6.616
Xi, W., Coulson, R. N., Birt, A. G., Shang, Z.-B., Waldron, J. D., Lafon, C. W., et al. (2009). Review of forest landscape models: types, methods, development and applications. Acta Ecol. Sin. 29, 69–78. doi: 10.1016/j.chnaes.2009.01.001
Yokoyama, S., and Shibata, E. (1998). The effects of sika-deer browsing on the biomass and morphology of a dwarf bamboo, Sasa nipponica, in Mt. Ohdaigahara, central Japan. For. Ecol. Manage. 103, 49–56. doi: 10.1016/S0378-1127(97)00175-8
Yoshii, C., Yamaura, Y., and Nakamura, F. (2018). “Predicting future range expansions of blakiston’s fish owl subject to conservation efforts”,” in Biodiversity Conservation Using Umbrella Species: Blakiston’s Fish Owl and the Red-crowned Crane Ecological Research Monographs, ed. F. Nakamura (Singapore: Springer), 221–236.
Keywords: climate change, renewable energy mix, farmland abandonment, solar power generation, woody biomass energy, LANDIS-II
Citation: Haga C, Maeda M, Hotta W, Inoue T, Matsui T, Machimura T, Nakaoka M, Morimoto J, Shibata H, Hashimoto S and Saito O (2020) Scenario Analysis of Renewable Energy–Biodiversity Nexuses Using a Forest Landscape Model. Front. Ecol. Evol. 8:155. doi: 10.3389/fevo.2020.00155
Received: 30 March 2020; Accepted: 05 May 2020;
Published: 27 May 2020.
Edited by:
Anouschka R. Hof, Wageningen University & Research, NetherlandsReviewed by:
Robert Michael Scheller, North Carolina State University, United StatesIvan Kruhlov, Ivan Franko National University of Lviv, Ukraine
Copyright © 2020 Haga, Maeda, Hotta, Inoue, Matsui, Machimura, Nakaoka, Morimoto, Shibata, Hashimoto and Saito. This is an open-access article distributed under the terms of the Creative Commons Attribution License (CC BY). The use, distribution or reproduction in other forums is permitted, provided the original author(s) and the copyright owner(s) are credited and that the original publication in this journal is cited, in accordance with accepted academic practice. No use, distribution or reproduction is permitted which does not comply with these terms.
*Correspondence: Chihiro Haga, Y2hpaGlyby5oYWdhQGdlLnNlZS5lbmcub3Nha2EtdS5hYy5qcA==