- 1Institute of Biology, University of Neuchâtel, Neuchâtel, Switzerland
- 2Institute of Systematic Botany, Zurich, Switzerland
- 3European Biological Control Laboratory, United States Department of Agriculture, Agricultural Research Service, Montferrier-sur-Lez, France
Plants produce distinct blends of volatile compounds that attract pollinators (floral odors) or natural enemies of insect herbivores (herbivore-induced plant volatiles). The admixture of these blends in the atmosphere may alter the attraction of insect mutualists and ultimately affect plant fitness. Here, using synthetic blends of Brassica rapa floral volatiles and real B. rapa flowers, we investigated how floral odors impact the foraging behavior of parasitoids. In an olfactometer setting, floral odors reduced the attractiveness of plants infested by herbivores to parasitoids by 43.5% and affected four out of five parasitoid species tested. Additionally, experiments with the parasitoid Cotesia glomerata revealed that the effects of floral odors are dose-dependent and that floral odors were less disruptive under wind tunnel conditions than under olfactometer conditions. Electroantennogram recordings showed that C. glomerata antennae do respond to floral compounds, but that floral compounds do not inhibit antennal responses to herbivore-induced leaf volatiles. In conclusion, floral odors can act as background pollutants decreasing the attractiveness of chemical blends used by natural enemies to locate their hosts. Under natural conditions, such interferences could affect the outcome of tritrophic interactions and may play an important role in the evolution of plant volatile signaling.
Introduction
Plants produce and release an immense diversity of volatile organic compounds (VOCs) into the atmosphere (Dudareva et al., 2006; Raguso, 2008; Mumm and Dicke, 2010). These compounds can be perceived and used as information by a variety of organisms, such as neighboring plants (Karban et al., 2000; Moreira et al., 2016), herbivores (Unsicker et al., 2009), members of the third trophic level (Turlings and Wäckers, 2004; Mumm and Dicke, 2010), and members of the fourth trophic level (Poelman et al., 2012). Some plant volatiles have evolved as signals conveying specific information to plant mutualists (Dicke and Baldwin, 2010; Schiestl and Johnson, 2013). For instance, the main ecological function of floral odors is to attract pollinators, and the floral blend may carry information about the identity of the flower and the quality of the nectar reward (Raguso, 2008; Knauer and Schiestl, 2015). Similarly, it is thought that some herbivore-induced plant volatiles (HIPVs) have evolved as a means to attract natural enemies (i.e., predators and parasitoids), which in turn fend off plant attackers and reduce herbivore damage (Dicke and Loon, 2000; Turlings and Wäckers, 2004; Turlings and Erb, 2018). This type of indirect plant defense has received extensive scientific attention (Godfray, 1994; Wajnberg et al., 2008) although the fitness benefits plants gain from attracting natural enemies are not easy to demonstrate (Allison and Hare, 2009; Hare, 2011; Kessler and Heil, 2011; Kaplan, 2012b). The volatiles of flowers and plants damaged by herbivores are likely to interact with each other in the atmosphere, contributing to the complexity of the olfactory landscape (Hilker and McNeil, 2008; Wäschke et al., 2013). Floral odors and HIPVs can involve common, ubiquitous compounds or, on the contrary, highly specific compounds or ratios of compounds (Dudareva et al., 2006; Heil, 2014), And their admixture may affect the behavioral response of receiving organisms (Kessler and Halitschke, 2009; Randlkofer et al., 2010; Desurmont et al., 2015).
When insects search for chemical cues to locate a specific resource, background odors are of key importance (Hilker and McNeil, 2008; Schröder and Hilker, 2008; Wäschke et al., 2013). Schröder and Hilker (2008) define three main types of background odors: irrelevant odors that play no role in insect foraging behavior, masking odors that neutralize the behavioral response of an insect toward an otherwise attractive odor source, and enhancing odors that increase the attractiveness of an odor source. Masking background odors may interfere with the perception of plant signals by blocking or competing with chemoreceptors at the antennal level (Schiestl and Roubik, 2003; Hilker and McNeil, 2008; Schröder and Hilker, 2008), or, alternatively, during the processing of the information in the central nervous system (Bruce et al., 2005; Gadenne et al., 2016). Ultimately, sensitivity to background odors is likely to depend on the mode of discrimination among odors blends used by the insect: parasitoids that cue on the presence or absence of certain specific compounds within a blend might be less sensitive to the presence of other background compounds than parasitoids that use ratio-specific or whole-blend recognition patterns (McCormick et al., 2012). As a result of detrimental admixtures between floral odors and HIPVs, plants may become less attractive to mutualists, which may have negative fitness consequences and ultimately impact the evolution of plant signaling over evolutionary time (Kessler et al., 2011).
It has been frequently reported that herbivore-damaged plants are less attractive to pollinators than undamaged plants (Adler et al., 2001; Cardel and Koptur, 2010; Danderson and Molano-Flores, 2010; Kessler et al., 2011; Schiestl et al., 2014), but the part played by direct interference between floral odors and HIPVs is often unclear because herbivory can directly impact the production of floral odors and/or the quality of the nectar reward to pollinators (Kessler et al., 2011). Similarly, herbivores can suffer less attacks by natural enemies when feeding on flowering plants than on vegetative plants (Lucas-Barbosa et al., 2011; Lucas-Barbosa et al., 2014), but the involvement of floral odors “masking” the presence of herbivores to natural enemies has been hard to assess for two reasons. First, herbivores may be physically less detectable to natural enemies when they feed on flowers (Bächtold and Alves-Silva, 2013). Second, flowering plants may produce less attractive blends of HIPVs compared to vegetative plants (Desurmont et al., 2015).
Plant volatile emissions are highly dynamic and context-dependent in nature. Therefore, the evaluation of the effects of admixtures of certain compounds on the behavior of insects under controlled conditions may be best done using synthetic compounds to create and manipulate specific blends and concentrations of plant odors (Schröder and Hilker, 2008; Schiestl et al., 2014). In a previous study involving the plant B. rapa L. (Brassicaceae), the specialist herbivore Pieris brassicae L. (Lepidoptera: Pieridae), and its main parasitoid Cotesia glomerata L. (Hymenoptera: Braconidae), we found that floral odors directly decrease the attractiveness of herbivore-infested plants to C. glomerata (Desurmont et al., 2015). Here, we test the hypothesis that this effect is general and evaluate its magnitude with five parasitoid species possessing different life histories and foraging strategies. In addition, we investigated the modalities and context-dependency of the effects of floral odors on the behavior of C. glomerata. Firstly, we recorded the behavior of C. glomerata in a wind tunnel when exposed to host-infested plants next to real flowers or next to odorless decoy flowers. Secondly, we tested the hypothesis that the effects of floral odors on parasitoid behavior are dose-dependent by comparing the effects of several concentrations of floral odors in an olfactometer setting and in a wind tunnel setting. Thirdly, we tested the hypothesis that floral compounds prevent the perception of HIPVs at the antennal level by conducting electroantennogram (EAG) tests with C. glomerata. For these latter tests, parasitoids were exposed to floral compounds and HIPVs of B. rapa, alone or in various mixes, and the overall responsiveness of parasitoid antennae was measured. Specifically, we asked the following questions: (1) Do floral odors of B. rapa affect the attractiveness of herbivore-infested plants in five parasitoid species? (2) Do B. rapa flowers affect the behavior of C. glomerata under wind tunnel conditions? (3) Do floral odors interfere with HIPVs in a dose-dependent manner? (4) Are floral odors detected by parasitoids at the antennal level and do they affect the responsiveness of antennal neurons to herbivore-induced volatiles?
Materials and Methods
Insect and Plant Material
The plants used for the experiments with all parasitoids, except for tests with Diaeretiella rapae M’Intosh (Hymenoptera: Braconidae), came from a wild accession of B. rapa; seeds were collected in 2012 and 2013 near Maarssen, Netherlands. Plants used for experiments with D. rapae were Arabidopsis thaliana (L.) (wild-type, Columbia-0) instead of B. rapa because the aphid and parasitoid colony had been maintained on that plant at the University of Zurich. All plants were grown in controlled growth chambers under 16/8 L: D light regime at constant 25°C, light intensity 240–260 μmol. They were grown in cylindrical plastic pots (4 × 10 cm), with fertilized commercial soil (Ricoter Aussaaterde, Aarberg, Switzerland) and were watered every other day without supplemental nutrients. Plants used for experiments were approximately 3 weeks old; at that age, B. rapa plants had three to five fully expanded leaves and A. thaliana had 10–15 rosette leaves.
A total of five parasitoids species, all naturally occurring in Europe and representative of a range of ecological characteristics regarding their host range, host life stage targeted, and known use of plant volatiles were used in the study. Specifically, we used the following species: C. glomerata, Hyposoter ebeninus (Gravenhorst) (Hymenoptera: Ichneumonidae), Pteromalus puparum L. (Hymenoptera: Pteromalidae), Microplitis mediator (Haliday) (Hymenoptera: Braconidae), and D. rapae (Hymenoptera: Braconidae) (Table 1 and Figure 2). Three of these species (C. glomerata, H. ebeninus, and M. mediator) parasitize larvae of caterpillars, one (D. rapae) parasitizes aphids, and the fifth one (P. puparum) parasitizes lepidopteran pupae. The use of HIPVs for host location is well documented for parasitoids of caterpillars and aphids in general (Godfray, 1994), and particularly well for C. glomerata (Geervliet et al., 1996; Bukovinszky et al., 2012; de Rijk et al., 2016; Desurmont et al., 2016a, b) and D. rapae (Reed et al., 1995; Girling et al., 2006; Blande et al., 2007) but remains poorly known for pupal parasitoids (Wajnberg et al., 2008). In regard to their degree of dietary specialization, two of the species (C. glomerata and H. ebeninus) are considered to be oligophagous parasitoids that can only attack a few host species within the family Pieridae (Lepidoptera) (Harvey et al., 2010b), whereas the three other species are generalist species able to parasitize hosts belonging to different insect families (Peck, 1963; Arthur and Mason, 1986; Pike et al., 1999). Parasitoids used for the study originated from laboratory colonies maintained on P. brassicae L. (Lepidoptera: Pieridae) larvae (parasitoid species C. glomerata and H. ebeninus), P. brassicae pupae (parasitoid species P. puparum), and Brevicoryne brassicae (Hemiptera: Aphididae) (parasitoid species D. rapae). P. brassicae caterpillars originated from a laboratory rearing maintained in our laboratory on B. rapa for oviposition and Brassica oleracea (Brassicales: Brassicaceae) for larval development. The rearing was initiated with field-collected individuals from various locations in Switzerland. B. brassicae aphids came from a laboratory rearing maintained at the University of Zurich.
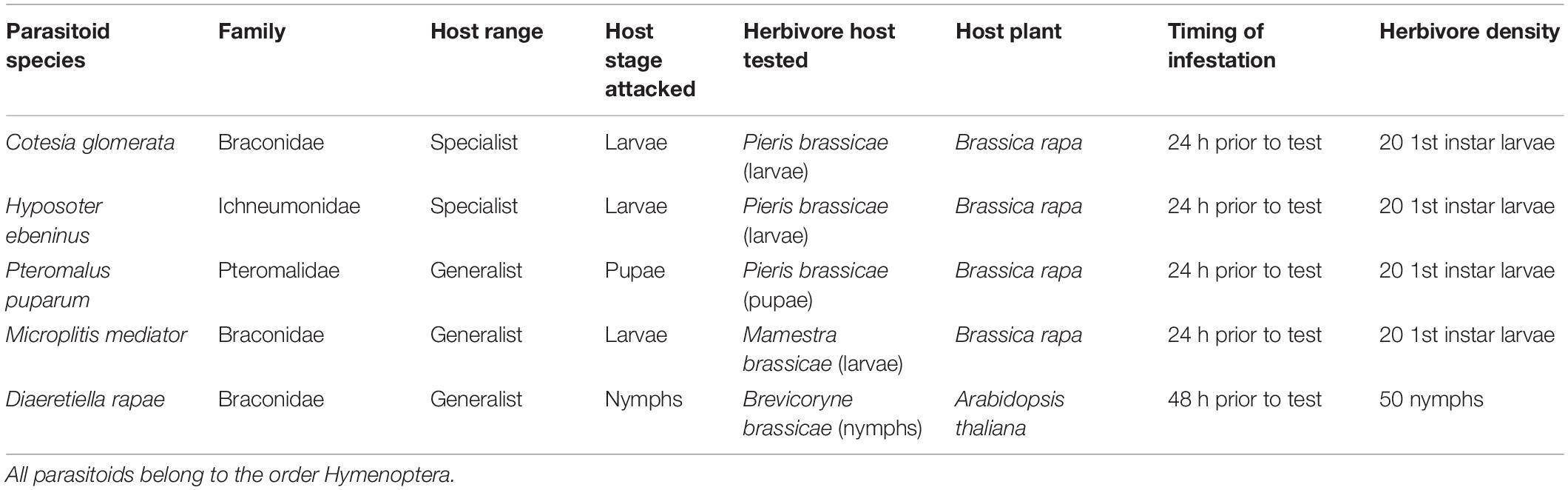
Table 1. Parasitoid species, their respective herbivore hosts, and details of the experiment setup (host plant, infestation timing, and herbivore density) used for olfactometer bioassays.
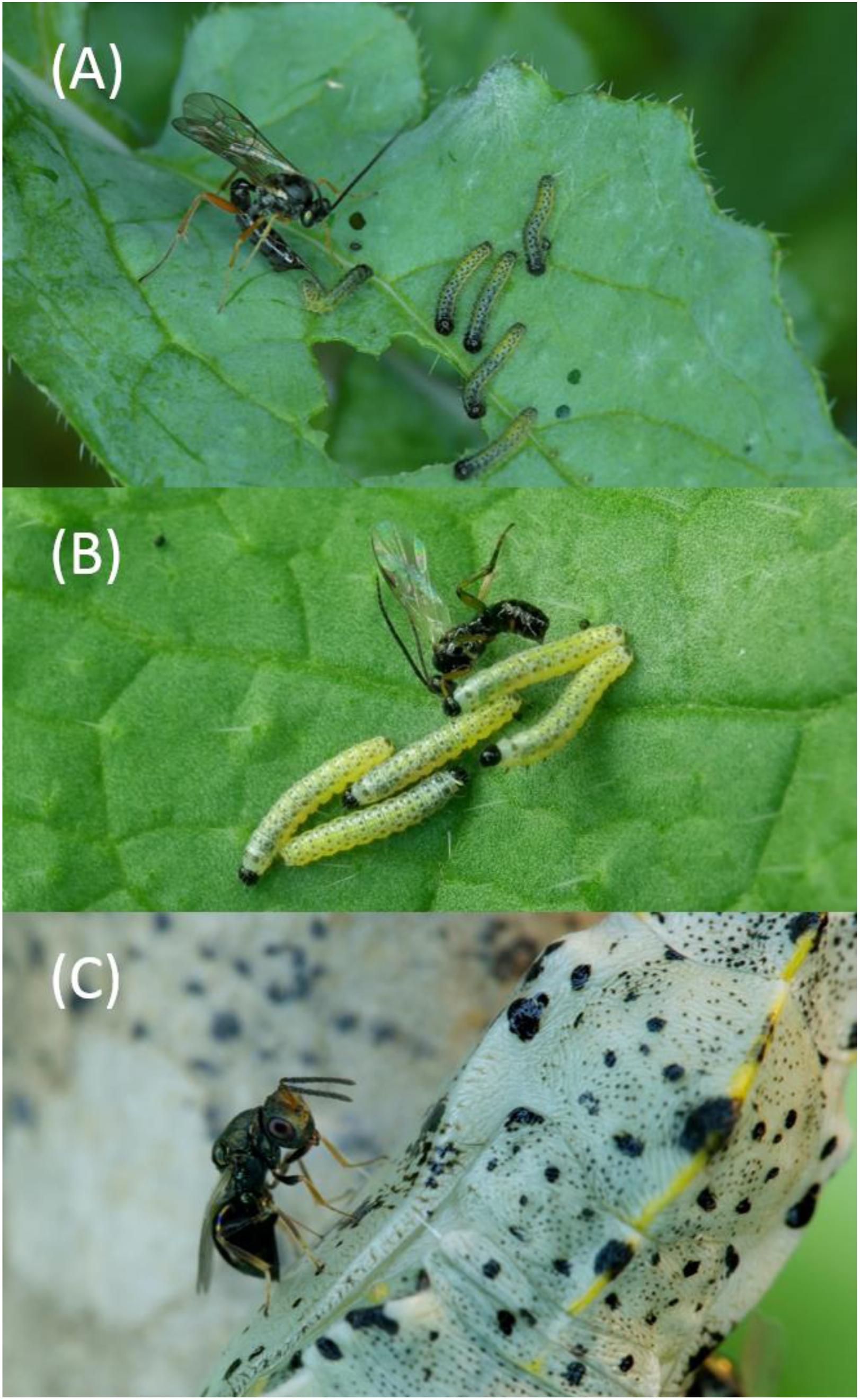
Figure 1. Three of the five parasitoid species used in the study. (A) Hyposoter ebeninus (Hymenoptera: Ichneumonidae) and (B) Cotesia glomerata (Hymenoptera: Braconidae) attacking Pieris brassicae (Lepidoptera: Pieridae) caterpillars. (C) Pteromalus puparum (Hymenoptera: Pteromalidae) on a P. brassicae pupa (photos: Thomas Degen).
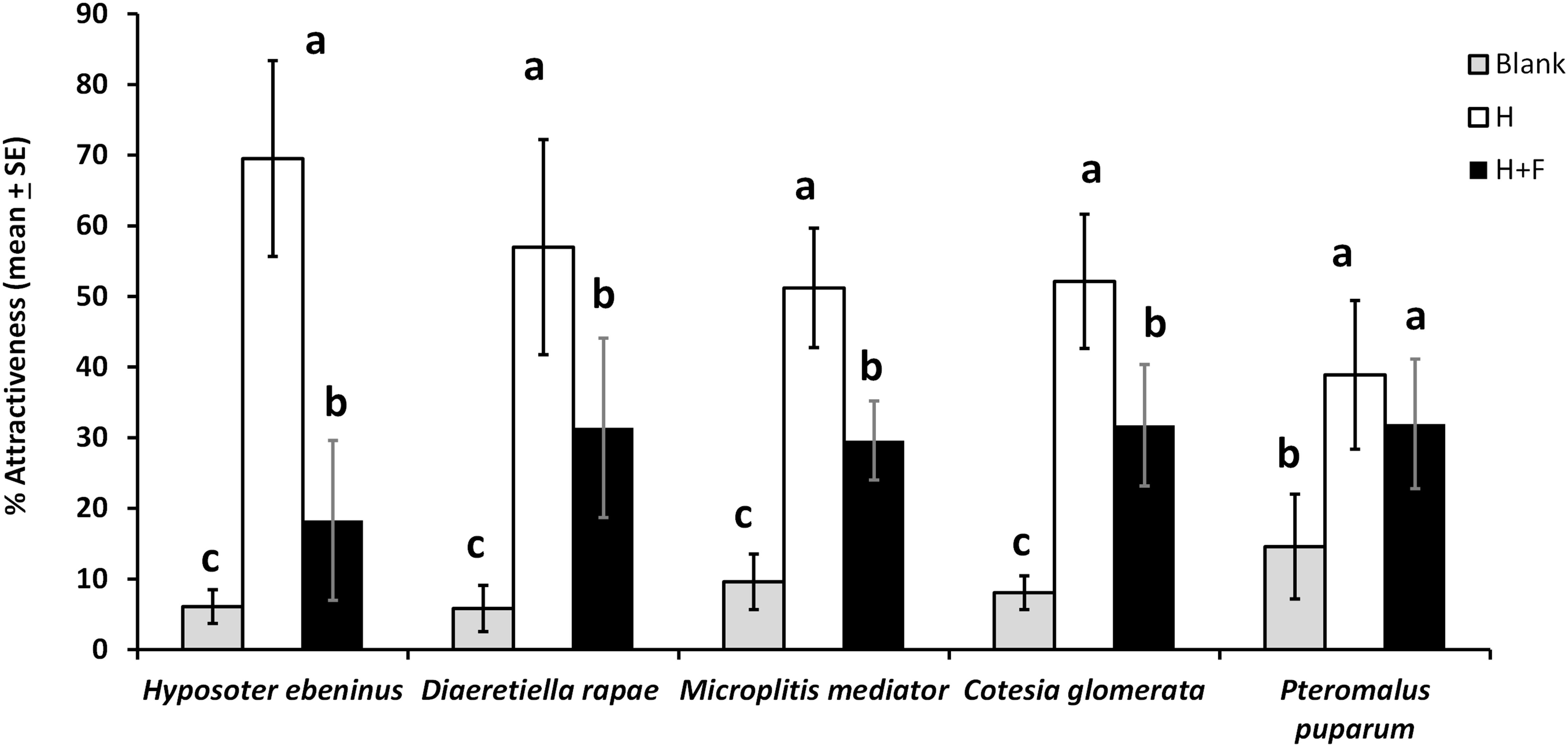
Figure 2. Parasitoid attraction (%, mean + SE) toward empty olfactometer arms (blank), plants infested by host herbivores (H), and plants infested by host herbivores with the addition of floral odors (H + F), in a 4-arm olfactometer for five parasitoid species (N = 6–9 per species). For each species, treatments with a different letter are statistically different from each other (Wilcoxon test, α = 0.01). The concentration of the floral mixture for these tests was equivalent to one Brassica rapa inflorescence (1 IE).
Cotesia glomerata originated from field-collected individuals from the Wageningen area (Netherlands) later supplemented with individuals collected in the Neuchâtel and Zurich areas (Switzerland). P. puparum and H. ebeninus originated from individuals collected in the field in the Neuchâtel area. M. mediator originated from individuals collected in the Frick area (Switzerland). Finally, D. rapae originated from individuals collected in the Zurich area. Parasitoids were maintained under similar conditions before being used for experimental purposes: they were collected as cocoons or adults after emergence from their hosts and were placed in cages (30 cm × 30 cm × 30 cm, model: bugdorm DP1000, MegaView Science Co., Taichung, Taiwan) without any host or plant material. They were provided water and droplets of honey and were kept in an incubator at 25°C and a 12:12 (l:d) photoperiod for 48 h to allow mating. Then the cages were transferred to an incubator at 13°C and a 12:12 (l:d) photoperiod. In the case of C. glomerata, most males were removed from the cages before the transfer at 13°C. Mated naïve females (i.e., females that had never been exposed to plant odors) that were 2–4 weeks old were used for all experiments. This rearing protocol was used with success in previous studies investigating the behavior of C. glomerata (Desurmont et al., 2016a, b).
Preparation of Synthetic Floral Odor Mixes
The method of using odor blends created by mixing pure synthetic compounds in behavioral bioassays is ideal to work with consistent blends whose concentrations can be easily changed and has been used with success in previous studies (Schiestl et al., 2014; Desurmont et al., 2015). The synthetic floral odor mix used for parasitoid behavioral bioassays was based on the six most abundant compounds produced by flowers of this B. rapa population found in headspace collections (Schiestl et al., 2014; Desurmont et al., 2015). The concentration of each compound in the solution was adjusted so that two rubber septa (GR-2, 5 mm Supelco, Bellefonte, PA, United States) soaked in the floral mix solution would emit the emission rate of each compound comparable to one inflorescence of B. rapa (ca. 30 flowers, hereafter named 1 inflorescence equivalent, IE), as described in a previous study (Schiestl et al., 2014). The six compounds composing the floral mix were: phenylacetaldehyde (≥90% purity, Sigma-Aldrich, Buchs, Switzerland) 3 μL/mL, nonanal (Givaudan, Dübendorf, Switherland) 9 μL/mL, decanal (Givaudan) 4 μL/mL, acetophenone (Givaudan) 24.5 μL/mL, p-anisaldehyde (≥99% purity, Sigma-Aldrich) 27 μL/mL, and α-farnesene (mixture of isomers, Sigma-Aldrich) 492 μL/mL, diluted in dichloromethane (HPLC grade, Sigma-Aldrich). Rubber septa (GR-2, 5 mm Supelco, Bellefonte, PA, United States) were left to soak in the floral mix solution for a duration of 1 h, then were allowed to dry for 2 h before being used for olfactometer or wind tunnel bioassays with parasitoids.
Olfactometer Experiments
The preferences of parasitoids for different odors were measured in a 4-arm olfactometer setting (D’alessandro and Turlings, 2005). In this setting, wasps were given the choice between four odor sources (= treatments) contained in separated glass bottles. Individual air flows were connected to each odor source and all converged to a central glass piece, where the wasps were released. After 30 min, wasps were recollected and the treatment they chose was recorded. For all parasitoids, except for H. ebeninus, one olfactometer test (= 1 replicate) consisted of five consecutive releases of five wasps (25 wasps tested per replicate) with the same set of plants. For H. ebeninus, which is significantly larger than the other species tested, parasitoids were released in groups of three instead of five, and one replicate consisted of five consecutive releases of three wasps with the same set of plants. Parasitoids were given the choice among two olfactometer arms carrying only purified air (= blanks), one arm with the odor of a vegetative plant infested by their host herbivore (herbivore treatment), and one arm with the odor of a vegetative plant infested by their host with the addition of synthetic floral odors (herbivore + floral odors treatment). To add the floral odor, two rubber septa spiked with a blend of volatiles (see Materials and Methods section: preparation of synthetic floral odor mixes) were placed above each plant from the herbivore + floral odors treatment prior to the tests. Two control septa that had been treated only with solvent were added to each plant from the herbivore treatment. Plants were changed and the olfactometer glassware was cleaned between replicates, and a total of six replicates was done for each parasitoid species tested (totaling 6 × 25 or 6 × 15 wasps per species), except for C. glomerata (nine replicates; 9 × 25 wasps). The modalities of plant infestation by herbivores prior to the tests (timing of infestation, number of herbivores used) is summarized in Table 1.
Olfactomer tests conducted to investigate dose-dependent effects of floral odors on C. glomerata attraction used the same 4-arm olfactometer setup. For these tests, parasitoids has to choose among two odor sources with purified air (= blanks), one vegetative plant infested by their host (herbivore treatment), and one vegetative plant infested by their host with the addition of synthetic floral odors (herbivore + floral odors treatment). Five concentrations of floral odors were tested: 0.25, 0.5, 1, 2, and 4 IE (see section “Materials and Methods”: preparation of synthetic floral odor mixes). One replicate consisted in five consecutive releases of five wasps with the same set of plants (25 wasps tested per replicate), and a number of five to nine replicates per concentration were conducted (0.25 IE, N = 7; 0.5 IE, N = 8, 1 IE, N = 9; 2 IE, N = 5, 4 IE, N = 5).
Wind Tunnel Experiments
For these behavioral tests, a 200 cm × 80 cm × 80 cm wind tunnel was used. A speed-controller fan (D340/E1, FDR32, Neunkirchen, Germany) pushed air through the tunnel, and the air velocity of 0.35 m s–1. Four charcoal filters (145.457 mm, carbon thickness 16 mm, Camfil Farr, Reinfeld, Germany) cleaned the incoming air. The tests were conducted during daytime at ambient room temperature (ca. 22°C). Naïve parasitoid females were tested individually in the wind tunnel. They were introduced in the wind tunnel in open plastic tubes placed on a stand at the downwind side of the tunnel. For the first experiment (effects of real B. rapa flowers on the behavior of C. glomerata), a 3-week old vegetative B. rapa plant with 3–5 fully expanded leaves in a cylindrical plastic pot (4 cm × 10 cm) infested with 15 first instar P. brassicae 24 h prior to the test was placed at the upwind end of the wind tunnel. Either a blooming B. rapa plant with one inflorescence (ca 30 flowers) or a yellow cardboard-made odorless decoy inflorescence of the same dimensions was placed 5 cm behind the infested B. rapa plant. Once an individual parasitoid flew away from the open tube to track an odor plume, the following variables were recorded: the total flight duration until landing, the outcome of parasitoid foraging after landing (flew away or attacked a P. brassicae caterpillar) and, for parasitoids that did attack a caterpillar, the duration between landing on the plant and attacking a caterpillar. The parasitoid was then removed from the wind tunnel. For each herbivore-infested B. rapa plant, five parasitoids were tested with a blooming B. rapa plant and five were tested with an odorless decoy, then the plants were replaced. The experiment was repeated five times (N = 5, 10 parasitoids tested per replicate). For the second experiment (effects of different concentrations of floral odors on the behavior of C. glomerata), two 3-week old vegetative B. rapa plants infested with 15 first instar P. brassicae were placed at the upwind end of the wind tunnel. The two plants were placed side by side and were separated by a distance of 20 cm. Two rubber septa soaked in floral odors prior to the experiment were placed along a vertical metal rod placed on a support behind one of the two B. rapa plants, and two septa that had been soaked only in solvent were placed along a vertical metal rod behind the second B. rapa plant. Once an individual parasitoid flew away from the open tube to track an odor plume, the plant it landed on was recorded and the parasitoid was removed. To avoid positional biases (i.e., parasitoid flying preferentially to one side of the wind tunnel), the position (left/right) of the rods or the position of the B. rapa plants was switched in the wind tunnel every five parasitoids tested so that all possible positional combinations were covered every 20 parasitoids tested. The B. rapa plants and the septa were changed once 20 parasitoids were tested (= 1 replicate), and the experiment was replicated five times per concentration of floral odors. Four concentrations of floral odors were tested (0.5, 1, 2, and 4 IE) for a total of 100 individual parasitoids tested per concentration. For all wind tunnel tests, parasitoids that did not fly after 5 min or flew directly to the ceiling or the side of the tunnel without tracking an odor plume were discarded and not counted in the total of parasitoids tested.
Electrophysiology
Mated C. glomerata females were anesthetized using CO2 and their head was excised below the prothoracic segment with micro scissors. The segment was inserted into the recording electrode (AgCl electrodes bathed in 0.1 M KCl solution contained in borosilicate glass capillaries, OD = 1.5 mm, ID = 1.05 mm, Hilgenberg, Malsfeld, Germany). Subsequently, the distal annulum of both antennae was cut off and the ends were inserted into the indifferent electrode. The head was held in a humidified, charcoal-filtered air stream (90–100% RH) delivered at 1 m/s via a metal tube (ID 6.9 mm) whose outlet was about 1 cm from the preparation. EAG responses were recorded on a computer using Syntech hardware and software (Syntech, Kirchzarten, Germany). The responsiveness of antennae was tested at the start with an air puff from a 5-mL stimulus syringe containing 1 μg of (Z)-3-hexenol on a filter paper strip introduced into the metal tube through a teflon tape-covered hole (Taneja and Guerin, 1997). If the EAG response to this 1 s air puff (1 mL/s) from the stimulus syringe was less than 6 mV, another head was mounted. Headspace collections were obtained by placing a 3-week old vegetative B. rapa plant with 3–5 fully expanded leaves that had been infested with 15 1st instar P. brassicae caterpillars 24 h prior in a volatile collection setup (Ton et al., 2007) for 6 h. In this setup, plant volatiles were collected using trapping filters containing 25 mg of 80–100 mesh SuperQ absorbent and extracted from the filters at the end of the collection with 150 μL of dichloromethane. Solvent extracts were obtained by rinsing leaves of vegetative B. rapa plants that had been infested with 15 1st instar P. brassicae caterpillars for 24 h directly with dichloromethane. Solutions containing synthetic plant volatiles (0.1 μg/μL) and headspace collections were placed in an aliquot of 10 μL on a filter paper strip that was placed in the stimulus syringes after evaporation of the solvent, and solvent extracts were applied to rubber septa similar to the ones used for behavioral bioassays and introduced into the stimulus syringe after being allowed to dry for 30 min.
In order to test how mixtures of B. rapa floral volatiles affect the EAG responses of C. glomerata females to leaf volatiles, three synthetic flower compounds separately or in mixture (flower mix) were presented to C. glomerata females in combination with three synthetic leaf compounds separately or in mixture (leaf mix) or in combination with headspace collections and solvent extractions of herbivore infested B. rapa leaves. The synthetic leaf chemicals used were three common leaf volatiles produced by herbivore-infested B. rapa plants (Danner et al., 2017; Desurmont et al., 2018): benzyl nitrile, (E)-2-hexenal, and (Z)-3-hexenyl acetate, and the synthetic flower chemicals used were three common compounds produced by B. rapa flowers: p-anisaldehyde, (E,E)-α-farnesene, and phenylacetaldehyde (Schiestl et al., 2014). The following stimuli were tested: solvent control (dichloromethane, N = 27), benzyl nitrile (benznit, N = 9), (E)-2-hexenal (e2hex, N = 9), (Z)-3-hexenyl acetate (z3ac, N = 10), mixture of benzyl nitrile, (E)-2-hexenal and (Z)-3-hexenyl acetate (leafmix, N = 10), p-anisaldehyde (anis, N = 9), mixture of leafmix and anis (leafmixanis, N = 10), α-farnesene (farn, N = 9), mixture of leafmix and farn (N = 10), phenylacetaldehyde (paa, N = 10), mixture of leafmix and paa (leafmixpaa, N = 9), mixture of α-farnesene, p-anisaldehyde, and phenylacetaldehyde (flower mix, N = 10), a mixture of leafmix and flower mix (N = 10), headspace collection of B. rapa leaves (headspace, N = 9), a mixture of headspace and flower mix (N = 10), solvent extract of B. rapa leaves (extract, N = 9), a mixture of flower mix and extract (extractflower, N = 9). Odor stimuli were tested in a random order. For each odor stimulus 9–10 C. glomerata females were used.
Statistical Analysis
Olfactometer bioassays: preferences of C. glomerata females were analyzed for each test using a generalized linear model (GLM) with a Poisson distribution fitted by maximum quasi-likelihood estimation (Turlings et al., 2004), with the number of wasps counted in the different branches of the olfactometer as the dependent variable. Means were then compared using a non-parametric multiple comparison Wilcoxon test (α = 0.01, JMP12). The number of wasps tested in each olfactometer test varied depending on the species tested (15 wasps per replicate for H. ebeninus, 25 per replicate for all other parasitoids), and the olfactometer data were analyzed for each parasitoid species separately. Results are presented as percentage attractiveness in the figures illustrating olfactometer tests for easier comprehension: percentage attractiveness of a treatment was calculated as the number of wasps that chose a treatment divided by the total number of wasps that made a choice during the test × 100. Wasps that did not make a choice during the olfactometer tests were not included in the analyses but were included to calculate parasitoid participation (i.e., number of parasitoids making a choice divided by the number of parasitoids tested × 100) after each test. The attractiveness values for the two empty odor sources (Blank1 and Blank2) were averaged in Figures 2, 4 to facilitate the visual representation of the data.
Wind tunnel bioassays: Data from the bioassays with real B. rapa flowers were analyzed using a student t-test (α = 0.05) testing the hypothesis that the parasitoids spend more time flying toward a plant and foraging on a plant in presence of floral odors than in their absence. Replicate was used as a blocking factor. The proportion of parasitoids that attacked a caterpillar after landing on a plant in presence and in absence of floral odors was compared using a Chi-square test (α = 0.05). Data from the bioassays comparing the effects of different concentrations of synthetic floral odors on the attractiveness of infested B. rapa plants were analyzed with a paired t-test (α = 0.05) comparing the number of parasitoids that landed on the plant with floral odors and the plant without floral odors for each positional combination of every replicate.
Electrophysiology: All multiple comparisons were analyzed in R (R Core Team, 2013). Analysis of variance (α = 0.05) was used to test whether the electroantenogram responses were significantly different between the different odor sources. When the ANOVA was significant (P < 0.05) multiple comparisons (R-package: Multcomp) were made using Tukey-contrasts with Bonferroni correction.
Results
Effects of Floral Odors on Five Parasitoid Species
There was a 43.5% overall decrease in the attractiveness of host-infested plants to parasitoids due to the addition of floral odors in 4-arm olfactometer tests (Figure 2). Specifically, floral odors caused a 44.9% decrease in plant attractiveness to D. rapae, 39.1% to C. glomerata, 73.7% to H. ebeninus, 42.2% to M. mediator, and 17.8% (non-significant decrease) to P. puparum. The pattern of parasitoid preferences was the same for four parasitoid species: parasitoids preferred the “herbivore” treatment more than the “herbivore + floral odors” treatment, and the two blanks were the least attractive treatments (D. rapae: df = 8, χ2 = 65.5, P < 0.0001; C. glomerata: df = 11, χ2 = 119.5, P < 0.0001; H. ebeninus: df = 8, χ2 = 80.9, P < 0.0001; M. mediator: df = 8, χ2 = 64.0, P < 0.0001). For the fifth parasitoid species, P. puparum the host and host + floral odors treatments were comparably attractive, and the two blanks were less attractive (df = 8, χ2 = 27.8, P < 0.001) (Figure 2). The percentage participation (percentage of parasitoids choosing one of the olfactometer arms) of the parasitoids was as follows: 48% for D. rapae, 77% for M. mediator, 85% for C. glomerata, 91% for H. ebeninus, and 96% for P. puparum.
Wind Tunnel Bioassays With Real Flowers
In the wind tunnel no-choice bioassays, C. glomerata females took 66% more time flying to the caterpillar-infested plant when in presence of a real inflorescence than in presence of an odorless decoy (df = 44, T = 1.73, P = 0.04) (seconds, mean + SE). After landing, the proportion of wasps attacking a caterpillar and the proportion of wasps leaving the plant without attacking a caterpillar were comparable in presence of a real inflorescence and in presence of a decoy (df = 1, χ2 = 2.2, P = 0.14). Finally, for the subset of observations where parasitoids attacked a caterpillar after landing on a plant, time spend foraging on the plant between landing and attacking a caterpillar was comparable in presence of a real inflorescence and in presence of a decoy (df = 45, T = 1.2, P = 0.88) (Figure 3).
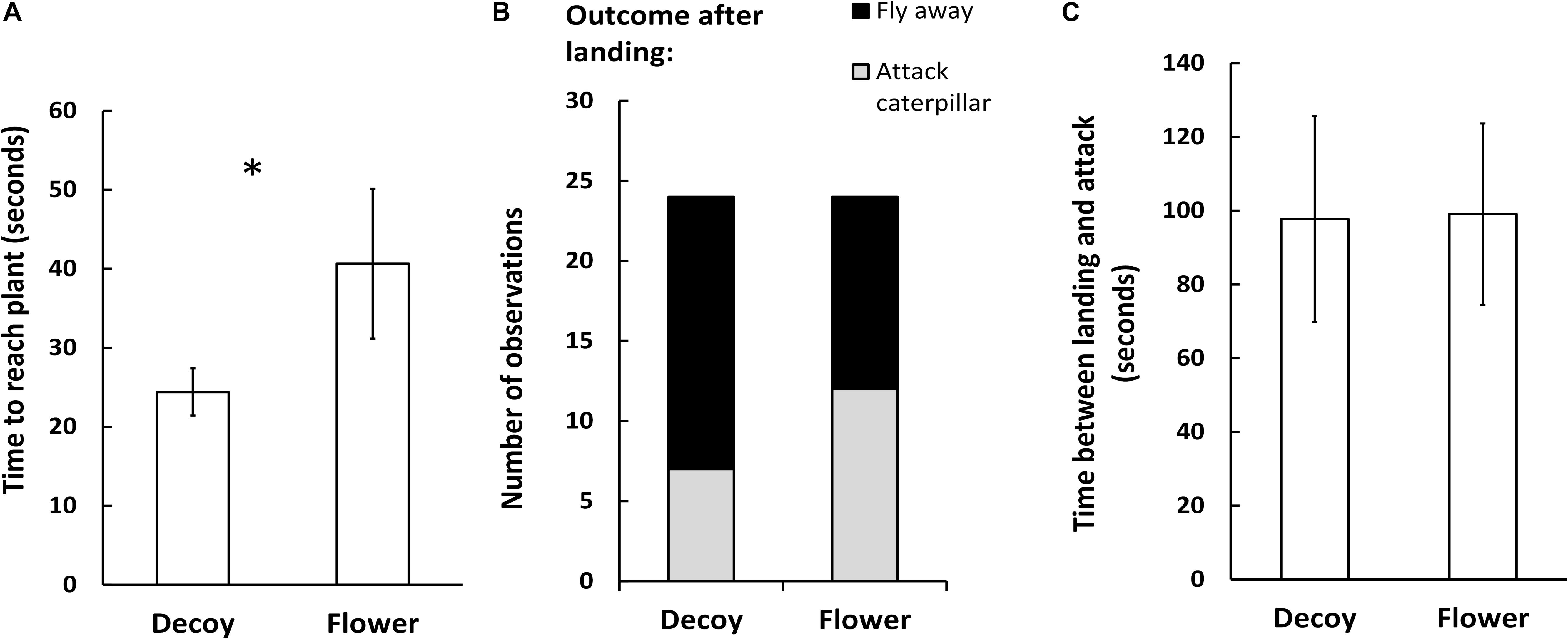
Figure 3. Foraging behavior of the parasitoid Cotesia glomerata in a wind tunnel in presence of a herbivore-infested Brassica rapa plant placed in front of a real B. rapa inflorescence (flower), or a cardboard-made odorless decoy inflorescence (decoy) (N = 25). (A) Time to reach the infested plant (seconds, mean ± SE), (B) outcome of the foraging behavior after landing (attacking a caterpillar or leaving the plant) (N = 24), and (C) time between landing on a plant and attacking a caterpillar (flower: N = 12; decoy: N = 7). Asterisk indicates a statistically significant difference (Student t-test α = 0.05).
Dose-Dependent Effects of Floral Odors on C. glomerata Attraction
In our 4-arm olfactometer tests, the effect of floral odors on plant attractiveness was dose-dependent: it was negligible at concentrations of floral odors inferior to one IE, and became increasingly significant at higher floral odors concentrations (39.1% reduction in attractiveness at 1 IE, 60.3% at 2 IE, and 86.4% at 4 IE). At the two highest concentrations (2 and 4 IE), the attractiveness of the herbivore + floral odors treatment was as low as the blanks (Figure 4). Specifically, the patterns of parasitoid preferences were as follows: the herbivore and herbivore + floral odors treatments were the most attractive and the blanks were least attractive at concentration 0.25 IE (df = 9, χ2 = 80.2, P < 0.0001); the herbivore treatment was the most attractive, the herbivore + floral odors treatment was not significantly different from the other treatments, and the blanks were the least attractive treatment at concentration 0.5 IE (df = 10, χ2 = 41.4, P < 0.0001); the herbivore treatment was the most attractive, the herbivore + floral odors treatment was intermediate, and the blanks were the least attractive at concentration 1 IE (df = 11, χ2 = 119.5; P < 0.0001); the herbivore treatment was the most attractive and the herbivore + floral odors and the blanks were comparably less attractive at concentration 2 IE (df = 7, χ2 = 55.87, P < 0.0001) and 4 IE (df = 7, χ2 = 123.3, P < 0.0001) (Figure 4).
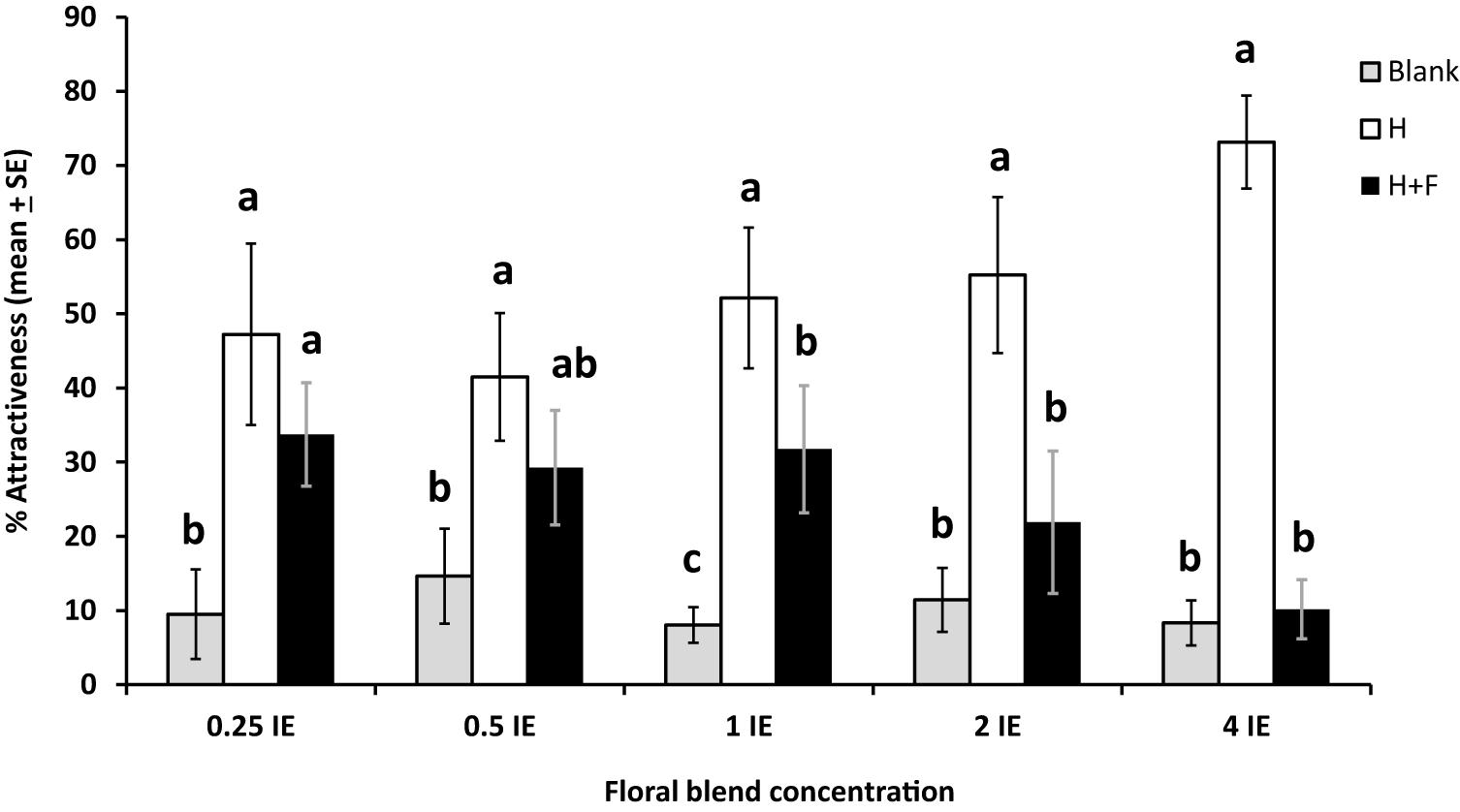
Figure 4. Effects of different concentrations of a mixture of synthetic floral odors on the foraging behavior of the parasitoid Cotesia glomerata in a 4-arm olfactometer (% attractiveness, mean ± SE) (N = 5–9 per concentration). Concentrations are given in Brassica rapa inflorescence equivalents (IE). Blank, empty olfactometer arms; H: plant infested by herbivores; H + F: plant infested by herbivores with the addition of floral odors. For each concentration, treatments with a different letter are statistically different from each other (Wilcoxon test, α = 0.01).
In a wind tunnel setting, the effect of floral odors on plant attractiveness to parasitoids was also dose-dependent. Indeed, plants with and without floral odors were similarly attractive to parasitoids when the concentration of floral odors was inferior or equal to 1 IE (df = 19, T = 0.29, P = 0.39 for concentration 0.5 IE; df = 19, T = 0.48, P = 0.68 for concentration 1 IE), and plants without floral odors were more attractive than plant with floral odors when the concentration of floral odors was superior to 1E (df = 19, T = 2.26, P = 0.02 for concentration 2 IE; df = 19, T = 2.44, P = 0.01 for concentration 4 IE) (% attraction, mean ± SE), with a reduction in attractiveness due to the addition of floral odors of 46% for concentration 2 IE and 53% for concentration 4 IE (Figure 5).
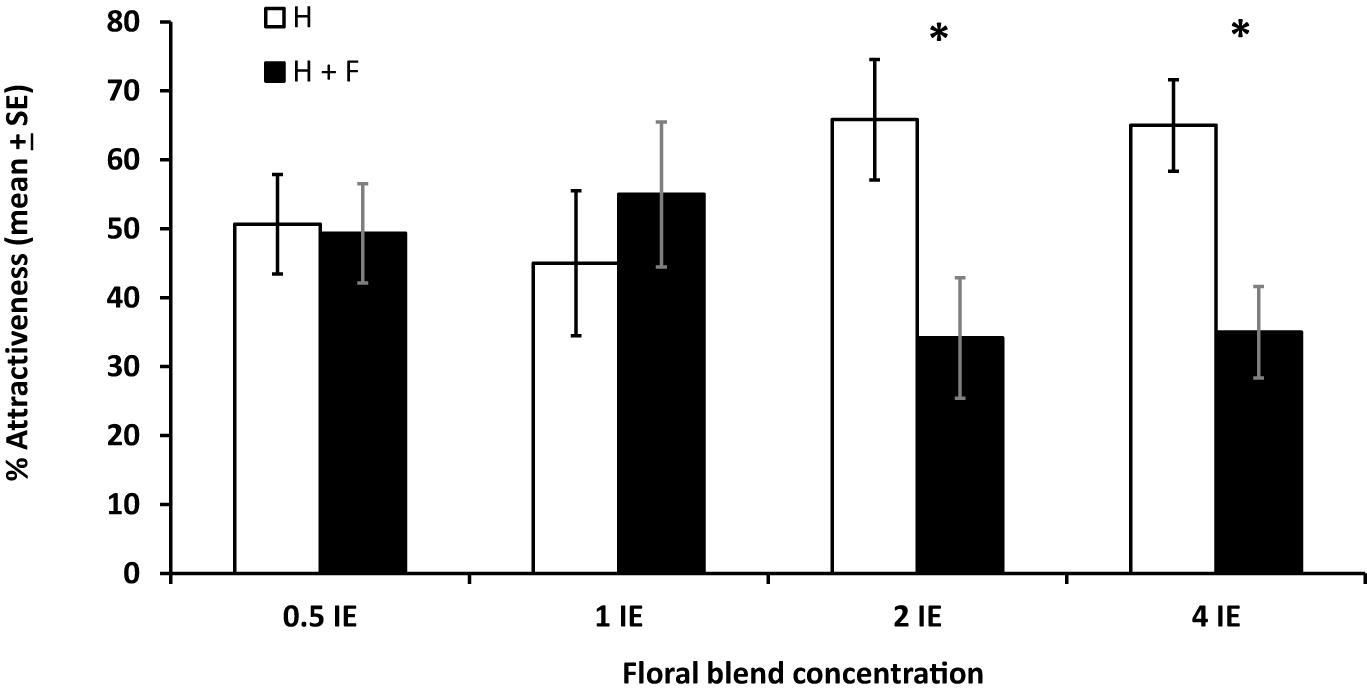
Figure 5. Effects of different concentrations of a mixture of synthetic floral odors on the foraging behavior of the parasitoid Cotesia glomerata in a wind tunnel (% attractiveness, mean ± SE). Concentrations are given in Brassica rapa inflorescence equivalents (IE). H: plant infested by herbivores; H + F: plant infested by herbivores with the addition of floral odors. For each concentration, treatments with an asterisk are statistically different from each other (paired t-test, α = 0.05).
Electrophysiology
The three B. rapa leaf volatiles, benzyl nitrile, (E)-2-hexenal, (Z)-3-hexenylacetate and a mixture of the three compounds (leafmix, a proximate for a leaf volatile blend) elicited significantly higher EAG responses in C. glomerata females than dichloromethane alone (F4,60 = 36.5, P < 0.001, Figure 6A). Pairwise comparisons between the four odor stimuli indicated that the leafmix did not elicit higher EAG responses, except compared to (Z)-3-hexenylacetate (Tukey post hoc, P < 0.05). In order to test if floral volatiles of B. rapa affect the EAG responses of C. glomerata females to leaf volatiles, p-anisaldehyde, α-farnesene and phenylacetaldehyde were presented in combination with the leafmix. While p-anisaldehyde on its own did not elicit significantly higher EAG responses compared to the control the compound significantly increased the EAG response of C. glomerata females to the leafmix (F3,52 = 68.3, P < 0.001, Tukey post hoc, P < 0.05, Figure 6B). The same pattern was observed with α-farnesene, with the compound not eliciting significantly different EAG responses singly compared to the control but increasing the EAG response to the leafmix (F3,52 = 65.9, P < 0.001, Tukey post hoc, P < 0.05, Figure 6C). Phenylacetaldehyde elicited significantly higher EAG responses when presented singly compared to the solvent control and increased the EAG response of C. glomerata females to the leafmix as well (F3,52 = 94.4, P < 0.001, Tukey post hoc, P < 0.05, Figure 6D).
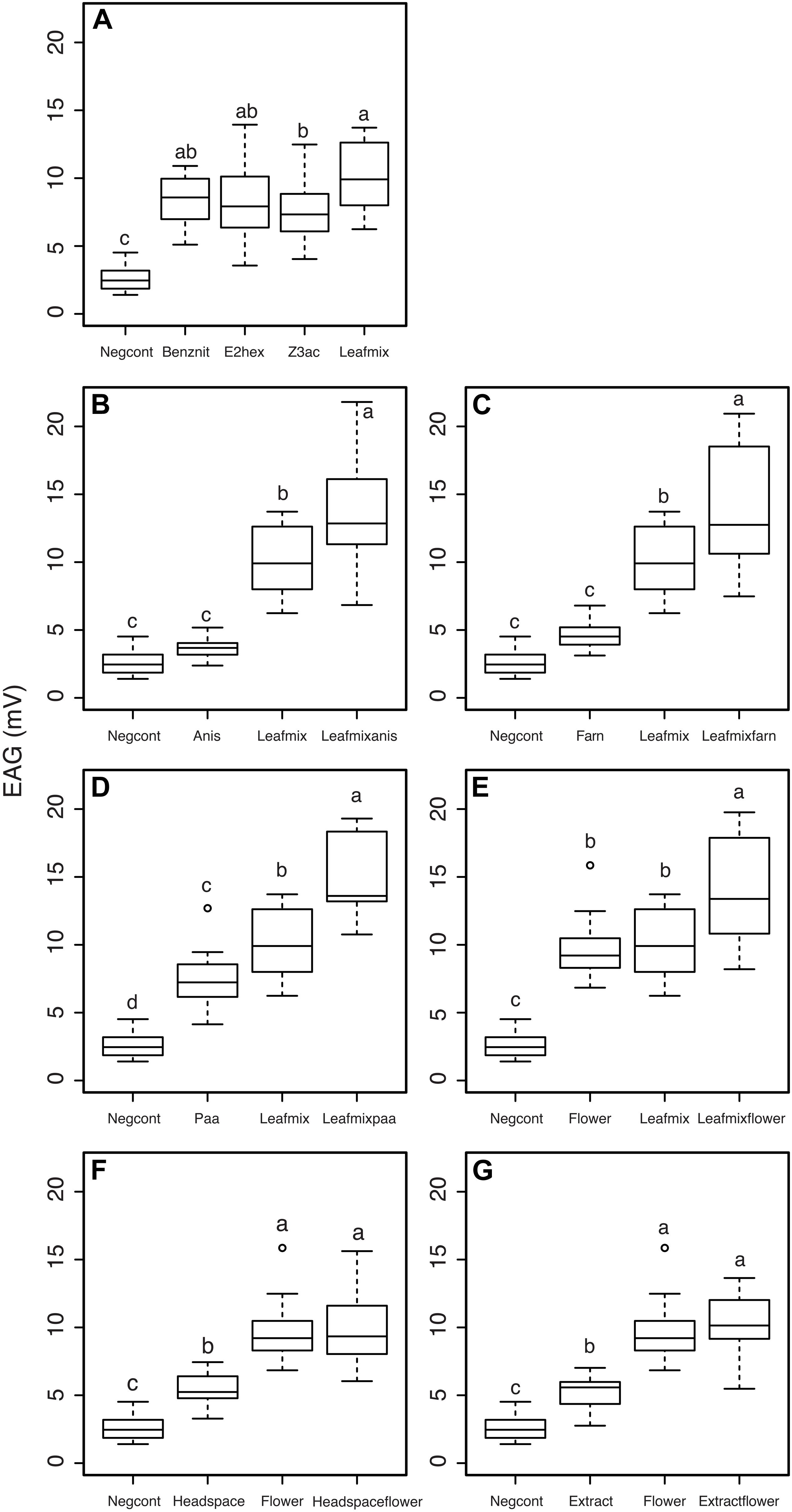
Figure 6. Electroantennogram responses of mated Cotesia glomerata females to plant volatiles. (A) Responses to leaf volatiles; (B) effects of anisaldehyde; (C) effects of alpha-farnesene; (D) effects of phenylacetaldehyde; (E) effects of a mixture of floral odors; (F) effects of headspace collections of leaf volatiles; (G) effects of solvent extracts of leaf volatiles. Negcont: solvent control, N = 27; benznit: benzyl nitrile, N = 9; E2hex: (E)-2-hexenal, N = 9; Z3ac: (Z)-3-hexenyl acetate, N = 10; leafmix: mixture of benzylnitrile, (E)-2-hexenal and (Z)-3-hexenyl acetate, N = 10; anis: anisaldehyde, N = 9; leafmixanis: mixture of leafmix and anis, N = 10; Farn: α-farnesene, N = 9; leafmixfarn: mixture of leafmix and farn, N = 10; paa: phenylacetaldehyde, N = 10; leafmixpaa: mixture of leafmix and paa, N = 9; flower: mixture of α-farnesene, p-anisaldehyde, and phenylacetaldehyde, N = 10; leafmixflower: mixture of leafmix and flower, N = 10; headspace: headspace collection of Brassica rapa leaves, N = 9; headspaceflower: mixture of headspace and flower, N = 10; extract: solvent extract of B. rapa leaves, N = 9; extractflower: mixture of flower and extract, N = 9. For each panel, treatments with a different letter are statistically different from each other (α = 0.05).
The flower mix elicited significantly higher EAG responses when presented singly compared to the solvent control and increased the EAG response of C. glomerata females to the leafmix as well (F3,53 = 76.6, P < 0.001, Tukey post hoc, P < 0.05, Figure 6E). While headspace collections from herbivore infested B. rapa leaves elicited significantly higher EAG responses compared to the control, the combination with the flower mix elicited similar EAG responses as the floral mix alone (F3,52 = 58, P < 0.001, Tukey post hoc, P < 0.05, Figure 6F). The same pattern was observed with solvent extracts of herbivore-infested B. rapa leaves, with the blend eliciting significantly higher EAG responses compared to the control but not eliciting different EAG responses when added to the floral mix compared to the floral mix alone (F3,51 = 7.32, P < 0.001, Tukey post hoc, P < 0.05, Figure 6G).
Discussion
In nature, the olfactory landscape is a complex and highly dynamic environment where scents produced by flowers and by herbivore-damaged plants are, among other sources of VOCs, likely to interact and mix, creating endless variations of volatile blends. Although the qualitative composition of an odor plume originating from a single source may be conserved over long distances (Vickers, 2000), the interactions with other plumes create background noise for plant mutualists that orient toward specific resource-indicating plumes (Beyaert and Hilker, 2014). Our results show that the odors of B. rapa flowers negatively affect parasitoids searching for hosts in two ways. Firstly, the presence of floral odors increases the flight time spent by female wasps tracking a plume of HIPVs (Figure 3). Secondly, the presence of floral odors directly decreases the attractiveness of herbivore-infested plants. This behavioral response to the addition of B. rapa floral odors was similar for four out of the five parasitoid species, independent of the host plant used to produce herbivore-infested plants (Figure 2), which suggests that it is a general effect relevant for many species of natural enemies. The behavior of the specialist ichneumonid H. ebeninus was by far the most affected, with a 73.7% decrease in attraction due to floral odors. Two out of the three generalist parasitoid species tested were affected, showing that this effect is not restricted to specialists. The only species that did not discriminate between host-infested plants with and without floral odors was P. puparum. It is currently unclear to what extent this pupal parasitoid uses volatiles induced by caterpillars in its host searching behavior. It should be noted that for all parasitoid species herbivore-infested plants with floral odors were always more attractive than clean odor sources (blanks). This shows that floral odors do not act as a repellent that fully neutralizes the behavioral response of parasitoids.
Electrophysiology bioassays did not support the hypothesis that floral volatiles and HIPVs compete for olfactory receptors at the antennal level. The parasitoid antennae responded to both HIPVs and floral compounds separately and in mixtures, and the addition of floral compounds separately or as a mixture to a mixture of HIPVs resulted in significantly higher antennal stimulation than HIPVs alone (Figure 6). This suggests that floral compounds do not inhibit responses to HIPVs. Therefore, the decrease in attractiveness associated with the presence of floral odors is more likely to be linked with how the chemical information is integrated by the central nervous system of the insect (Sandoz et al., 2007; Beyaert and Hilker, 2014).
The “background noise” created by the presence of floral odors appears to be dose-dependent as evidenced by bioassays with C. glomerata in the two experimental settings (olfactometer and wind tunnel) (Figures 4, 5). Interestingly, higher doses of floral odors were needed to cause a decrease in attractiveness under wind tunnel conditions compared to olfactometer conditions. This indicates that HIPVs and floral odors did not interact in the same way or that the behavior of the parasitoids was different in the two settings. In the olfactometer, the air flow is constricted and turbulences are presumably higher, likely resulting in a strong blending of HIPVs and floral odors. In the wind tunnel setting, the blends of HIPVs and floral odors had space to form distinct odor plumes. Moreover, the parasitoids had more space to fly and manoeuver in the wind tunnel, which might have helped them to distinguish between odor plumes. In nature, B. rapa plants produce a flowering stalk before blooming, which extends the distance between the leaves and the flowers. Thus, HIPVs and floral odors are likely to form distinct odor plumes, which may attenuate interference effects.
Under natural conditions, parasitoids are likely to encounter multiple odor plumes simultaneously when tracking their hosts and they must have evolved ways to navigate such complex olfactory landscapes (Hambäck and Beckerman, 2003; Mumm and Hilker, 2005; Schröder and Hilker, 2008; Randlkofer et al., 2010). Our data suggest that the presence of B. rapa inflorescences in the close vicinity of a host-infested plant would make such a plant less attractive to parasitoids, which may in turn decrease the risk for herbivores to be parasitized (Poelman et al., 2009; Foti et al., 2018). However, several factors may play a role in the realized effect of floral odors on parasitoid behavior in the field. First, parasitoids feed on the nectar and pollen of a variety of flowers (Jervis and Kidd, 1999; Wäckers, 2004), and may switch from searching for hosts to searching for food depending on their hunger state (Wäckers, 1994; Lewis et al., 1998) and mating status (Xu et al., 2017; Xu and Turlings, 2018). In our bioassays, parasitoids were well-fed and presumably eager to search for hosts rather than food. Starved parasitoids may be attracted to floral odors rather than being repelled by them (Belz et al., 2013; Géneau et al., 2013). Second, the spatial and temporal scales at which HIPVs and floral odors interact certainly play a critical role in their interference potential (Szyszka et al., 2012; Riffell et al., 2014). Finally, parasitoids foraging in a flower-rich environment may become habituated to the background odors originating from flowers, which may reduce their physiological response to the compounds present in floral blends (Schröder and Hilker, 2008) and thereby diminish the interferences between HIPVs and floral odors. A recent key study by Foti et al. (2018) showed that parasitism rates in field patches with and without floral borders matched the respective behavioral responses of two parasitoids toward floral odors, indicating that floral odors can affect parasitoid host foraging under natural conditions.
From the parasitoid’s perspective, avoiding herbivore-infested plants in the vicinity of flowers could have an adaptive value, for example, if risks of predation are higher in flower-rich habitats (Shiojiri and Takabayashi, 2005). From the plant’s perspective, negative interference effects between volatiles attracting natural enemies and pollinators may constitute an evolutionary tradeoff leading to contrasting selection pressures between traits favoring defense and those favoring pollination (McCall and Irwin, 2006; Kessler and Halitschke, 2009; Farré-Armengol et al., 2013). Plants may deal with this tradeoff by being plastic in their volatile emissions and adjust volatile production in leaves and flowers depending on which mutualist they need to attract at a particular time (Schiestl et al., 2014; Silveira et al., 2018). Interestingly, in B. rapa, the emission of floral odors is decreased after herbivore damage and floral odors of damaged plants have a lesser deterrent effect on the parasitoid C. glomerata than floral odors from undamaged plants (Schiestl et al., 2014). This implies that the decrease in floral volatile emissions from B. rapa plants after herbivory is adaptive and attenuates the negative effects of floral scents on parasitoid attraction. Conversely, HIPV emissions B. rapa plants in response to P. brassicae herbivory are less strong at the flowering stage than at the vegetative stage (Desurmont et al., 2015), which corroborates findings obtained with Brassica nigra (Lucas-Barbosa et al., 2011, 2014). This may illustrate ontogeny-dependent resource constraints in B. rapa (Boege and Marquis, 2005), or an adaptive strategy of the plants to reduce the negative effects of HIPVs on pollinator attraction. Herbivores could potentially take advantage of this tradeoff between natural enemy attraction and pollinator attraction by preferentially feeding on or nearby scent-producing flowers, effectively masking them from parasitoids (McCall and Irwin, 2006; Lucas-Barbosa et al., 2014).
The idea that infochemical networks may be sensitive to chemical interferences has received recent evidence with synthetic chemical compounds (Lürling and Scheffer, 2007), atmospheric pollutants (Holopainen and Gershenzon, 2010; Pinto et al., 2010), volatiles released by exotic plants (Harvey et al., 2010a; Bezemer et al., 2014), and for volatiles induced by exotic herbivores (Desurmont et al., 2014; Chabaane et al., 2015; Danner et al., 2017), but the current study is the first to closely examine the general role of floral odors as info-disrupters affecting natural enemies. These results have implications for applied pest management strategies that employ semiochemicals, which are receiving considerable attention (Witzgall et al., 2010; Kaplan, 2012a; Pickett and Khan, 2016). The potential impact of interfering background volatiles should be considered in such strategies. This is also relevant for the use of flower strips in agricultural fields. Flower strips serve as sources of food and refuges for natural enemies, which positively affect their impact on various insect pests (Bianchi and Wäckers, 2008; Jonsson et al., 2008). The floral odors emanating for these plants could potentially reduce the foraging efficiency of parasitoids and negatively affect parasitism rates (Foti et al., 2018). The amounts and types of scents emitted by plant species used in these flower strips, as well as their density and distance from pest-infested plants should be taken in consideration to ensure the efficacy of this conservation biological control strategy.
Data Availability Statement
The raw data supporting the conclusions of this article will be made available by the authors, without undue reservation.
Author Contributions
GD and FS designed the study. GD and MA collected and analyzed the data. GD wrote a first draft of the manuscript. All authors contributed to subsequent versions of the manuscript.
Funding
This study was funded by the Swiss National Science Foundation, in the context of the EUROCORES Programme EuroVOL (project InvaVOL) of the European Science Foundation.
Conflict of Interest
The authors declare that the research was conducted in the absence of any commercial or financial relationships that could be construed as a potential conflict of interest.
Acknowledgments
The authors thank Diane Laplanche, Marie-Jeanne Tschudi, and Hao Xu for their help with rearing the herbivores and parasitoids used in the study, Tom de Jong (Leiden University) for providing the B. rapa seeds used in this study, Thomas Degen for providing pictures of parasitoids, and Elodie Belz for providing M. mediator parasitoids.
References
Adler, L. S., Karban, R., and Strauss, S. Y. (2001). Direct and indirect effects of alkaloids on plant fitness via herbivory and pollination. Ecology 82, 2032–2044. doi: 10.1086/303374
Allison, J. D., and Hare, J. D. (2009). Learned and naive natural enemy responses and the interpretation of volatile organic compounds as cues or signals. New Phytol. 184, 768–782. doi: 10.1111/j.1469-8137.2009.03046.x
Arthur, A., and Mason, P. (1986). Life history and immature stages of the parasitoid Microplitis mediator (Hymenoptera: Braconidae), reared on the bertha armyworm Mamestra configurata (Lepidoptera: Noctuidae). Can. Entomol. 118, 487–491. doi: 10.4039/Ent118487-5
Bächtold, A., and Alves-Silva, E. (2013). Behavioral strategy of a lycaenid (Lepidoptera) caterpillar against aggressive ants in a Brazilian savanna. Acta Ethol. 16, 83–90.
Belz, E., Kölliker, M., and Balmer, O. (2013). Olfactory attractiveness of flowering plants to the parasitoid Microplitis mediator: potential implications for biological control. Biocontrol 58, 163–173.
Beyaert, I., and Hilker, M. (2014). Plant odour plumes as mediators of plant–insect interactions. Biol. Rev. 89, 68–81. doi: 10.1111/brv.12043
Bezemer, T. M., Harvey, J. A., and Cronin, J. T. (2014). Response of native insect communities to invasive plants. Annu. Rev. Entomol. 59, 119–141. doi: 10.1146/annurev-ento-011613-162104
Bianchi, F. J., and Wäckers, F. L. (2008). Effects of flower attractiveness and nectar availability in field margins on biological control by parasitoids. Biol. Control 46, 400–408.
Blande, J., Pickett, J., and Poppy, G. (2007). A comparison of semiochemically mediated interactions involving specialist and generalist Brassica-feeding aphids and the braconid parasitoid Diaeretiella rapae. J. Chem. Ecol. 33, 767–779. doi: 10.1007/s10886-007-9264-7
Boege, K., and Marquis, R. J. (2005). Facing herbivory as you grow up: the ontogeny of resistance in plants. Trends Ecol. Evol. 20, 441–448. doi: 10.1016/j.tree.2005.05.001
Bruce, T. J., Wadhams, L. J., and Woodcock, C. M. (2005). Insect host location: a volatile situation. Trends Plant Sci. 10, 269–274. doi: 10.1016/j.tplants.2005.04.003
Bukovinszky, T., Poelman, E. H., Kamp, A., Hemerik, L., Prekatsakis, G., and Dicke, M. (2012). Plants under multiple herbivory: consequences for parasitoid search behaviour and foraging efficiency. Anim. Behav. 83, 501–509.
Cardel, Y. J., and Koptur, S. (2010). Effects of florivory on the pollination of flowers: an experimental field study with a perennial plant. Int. J. Plant Sci. 171, 283–292.
Chabaane, Y., Laplanche, D., Turlings, T. C., and Desurmont, G. A. (2015). Impact of exotic insect herbivores on native tritrophic interactions: a case study of the African cotton leafworm, Spodoptera littoralis and insects associated with the field mustard Brassica rapa. J. Ecol. 103, 109–117.
D’alessandro, M., and Turlings, T. C. (2005). In situ modification of herbivore-induced plant odors: a novel approach to study the attractiveness of volatile organic compounds to parasitic wasps. Chem. Senses 30, 739–753. doi: 10.1093/chemse/bji066
Danderson, C. A., and Molano-Flores, B. (2010). Effects of herbivory and inflorescence size on insect visitation to Eryngium yuccifolium (Apiaceae) a prairie plant. Am. Midl. Nat. 163, 234–246.
Danner, H., Desurmont, G. A., Cristescu, S. M., and Van Dam, N. M. (2017). Herbivore-induced plant volatiles accurately predict history of coexistence, diet breadth, and feeding mode of herbivores. New Phytol. 220, 726–738. doi: 10.1111/nph.14428
de Rijk, M., Wang, Q., Papagiannaki, E., Dicke, M., and Poelman, E. H. (2016). Herbivore species identity rather than diversity of the non-host community determines foraging behaviour of the parasitoid wasp C otesia glomerata. Entomol. Exp. Appl. 161, 20–30.
Desurmont, G. A., Guiguet, A., and Turlings, T. C. J. (2018). Invasive insect herbivores as disrupters of chemically-mediated tritrophic interactions: effects of herbivore density and parasitoid learning. Biol. Invasions 20, 195–206.
Desurmont, G. A., Harvey, J., Van Dam, N. M., Cristescu, S. M., Schiestl, F. P., Cozzolino, S., et al. (2014). Alien interference: disruption of infochemical networks by invasive insect herbivores. Plant Cell Environ. 37, 1854–1865. doi: 10.1111/pce.12333
Desurmont, G. A., Laplanche, D., Schiestl, F. P., and Turlings, T. C. (2015). Floral volatiles interfere with plant attraction of parasitoids: ontogeny-dependent infochemical dynamics in Brassica rapa. BMC Ecol. 15:17. doi: 10.1186/s12898-015-0047-7
Desurmont, G. A., Xu, H., and Turlings, T. C. J. (2016a). Powdery mildew suppresses herbivore-induced plant volatiles and interferes with parasitoid attraction in Brassica rapa. Plant Cell Environ. 39, 1920–1927. doi: 10.1111/pce.12752
Desurmont, G. A., Zemanova, M. A., and Turlings, T. C. J. (2016b). The gastropod menace: slugs on Brassica plants affect caterpillar survival through consumption and interference with parasitoid attraction. J. Chem. Ecol. 42, 183–192. doi: 10.1007/s10886-016-0682-2
Dicke, M., and Baldwin, I. T. (2010). The evolutionary context for herbivore-induced plant volatiles: beyond the ‘cry for help’. Trends Plant Sci. 15, 167–175. doi: 10.1016/j.tplants.2009.12.002
Dicke, M., and Loon, J. J. (2000). Multitrophic effects of herbivore-induced plant volatiles in an evolutionary context. Entomol. Exp. Appl. 97, 237–249.
Dudareva, N., Negre, F., Nagegowda, D. A., and Orlova, I. (2006). Plant volatiles: recent advances and future perspectives. Crit. Rev. Plant Sci. 25, 417–440.
Farré-Armengol, G., Filella, I., Llusia, J., and Peñuelas, J. (2013). Floral volatile organic compounds: between attraction and deterrence of visitors under global change. Perspect. Plant Ecol. Evol. Syst. 15, 56–67.
Foti, M. C., Peri, E., Wajnberg, E., Colazza, S., and Rostás, M. (2018). Contrasting olfactory responses of two egg parasitoids to buckwheat floral scent are reflected in field parasitism rates. J. Pest Sci. 92, 747–756.
Gadenne, C., Barrozo, R. B., and Anton, S. (2016). Plasticity in insect olfaction: to smell or not to smell? Annu. Rev. Entomol. 61, 317–333. doi: 10.1146/annurev-ento-010715-023523
Geervliet, J. B., Vet, L. E., and Dicke, M. (1996). Innate responses of the parasitoids Cotesia glomerata and C. rubecula (Hymenoptera: Braconidae) to volatiles from different plant-herbivore complexes. J. Insect Behav. 9, 525–538.
Géneau, C. E., Wäckers, F. L., Luka, H., and Balmer, O. (2013). Effects of extrafloral and floral nectar of Centaurea cyanus on the parasitoid wasp Microplitis mediator: olfactory attractiveness and parasitization rates. Biol. Control 66, 16–20.
Girling, R., Hassall, M., Turner, J., and Poppy, G. (2006). Behavioural responses of the aphid parasitoid Diaeretiella rapae to volatiles from Arabidopsis thaliana induced by Myzus persicae. Entomol. Exp. Appl. 120, 1–9. doi: 10.1073/pnas.0710305105
Godfray, H. C. J. (1994). Parasitoids: Behavioral and Evolutionary Ecology. Princeton, NJ: Princeton University Press.
Hambäck, P. A., and Beckerman, A. P. (2003). Herbivory and plant resource competition: a review of two interacting interactions. Oikos 101, 26–37.
Hare, J. D. (2011). Ecological role of volatiles produced by plants in response to damage by herbivorous insects. Annu. Rev. Entomol. 56, 161–180. doi: 10.1146/annurev-ento-120709-144753
Harvey, J. A., Bukovinszky, T., and Van Der Putten, W. H. (2010a). Interactions between invasive plants and insect herbivores: a plea for a multitrophic perspective. Biol. Conserv. 143, 2251–2259. doi: 10.1016/j.biocon.2010.03.004
Harvey, J. A., Poelman, E. H., and Gols, R. (2010b). Development and host utilization in Hyposoter ebeninus (Hymenoptera: Ichneumonidae), a solitary endoparasitoid of Pieris rapae and P. brassicae caterpillars (Lepidoptera: Pieridae). Biol. Control 53, 312–318. doi: 10.1016/j.biocontrol.2010.02.004
Heil, M. (2014). Herbivore-induced plant volatiles: targets, perception and unanswered questions. New Phytol. 204, 297–306.
Hilker, M., and McNeil, J. (2008). “Chemical and behavioral ecology in insect parasitoids: how to behave optimally in a complex odorous environment,” in Behavioral Ecology of Insect Parasitoids: From Theoretical Approaches to Field Applications, eds E. Wajnberg, C. Bernstein, and J. J. M. van Alphen (Oxford: Blackwell Publishing), 92–112.
Holopainen, J. K., and Gershenzon, J. (2010). Multiple stress factors and the emission of plant VOCs. Trends Plant Sci. 15, 176–184. doi: 10.1016/j.tplants.2010.01.006
Jervis, M. A., and Kidd, N. A. (1999). “Parasitoid adult nutritional ecology: implications for biological control,” in Theoretical Approaches to Biological Control, eds B. Hawkins and H. Cornell (Cambridge: Cambridge University Press), 131–151.
Jonsson, M., Wratten, S. D., Landis, D. A., and Gurr, G. M. (2008). Recent advances in conservation biological control of arthropods by arthropods. Biol. Control 45, 172–175.
Kaplan, I. (2012a). Attracting carnivorous arthropods with plant volatiles: the future of biocontrol or playing with fire? Biol. Control 60, 77–89.
Kaplan, I. (2012b). Trophic complexity and the adaptive value of damage-induced plant volatiles. PLoS Biol. 10:e1001437. doi: 10.1371/journal.pbio.1001437
Karban, R., Baldwin, I. T., Baxter, K. J., Laue, G., and Felton, G. (2000). Communication between plants: induced resistance in wild tobacco plants following clipping of neighboring sagebrush. Oecologia 125, 66–71. doi: 10.1007/PL00008892
Kessler, A., and Halitschke, R. (2009). Testing the potential for conflicting selection on floral chemical traits by pollinators and herbivores: predictions and case study. Funct. Ecol. 23, 901–912.
Kessler, A., Halitschke, R., and Poveda, K. (2011). Herbivory-mediated pollinator limitation: negative impacts of induced volatiles on plant–pollinator interactions. Ecology 92, 1769–1780. doi: 10.1890/10-1945.1
Kessler, A., and Heil, M. (2011). The multiple faces of indirect defences and their agents of natural selection. Funct. Ecol. 25, 348–357.
Knauer, A. C., and Schiestl, F. P. (2015). Bees use honest floral signals as indicators of reward when visiting flowers. Ecol. Lett. 18, 135–143. doi: 10.1111/ele.12386
Lewis, W., Stapel, J. O., Cortesero, A. M., and Takasu, K. (1998). Understanding how parasitoids balance food and host needs: importance to biological control. Biol. Control 11, 175–183.
Lucas-Barbosa, D., Poelman, E. H., Aartsma, Y., Snoeren, T. A., Van Loon, J. J., and Dicke, M. (2014). Caught between parasitoids and predators–survival of a specialist herbivore on leaves and flowers of mustard plants. J. Chem. Ecol. 40, 621–631. doi: 10.1007/s10886-014-0454-9
Lucas-Barbosa, D., Van Loon, J. J., and Dicke, M. (2011). The effects of herbivore-induced plant volatiles on interactions between plants and flower-visiting insects. Phytochemistry 72, 1647–1654. doi: 10.1016/j.phytochem.2011.03.013
Lürling, M., and Scheffer, M. (2007). Info-disruption: pollution and the transfer of chemical information between organisms. Trends Ecol. Evol. 22, 374–379. doi: 10.1016/j.tree.2007.04.002
McCall, A. C., and Irwin, R. E. (2006). Florivory: the intersection of pollination and herbivory. Ecol. Lett. 9, 1351–1365. doi: 10.1111/j.1461-0248.2006.00975.x
McCormick, A. C., Unsicker, S. B., and Gershenzon, J. (2012). The specificity of herbivore-induced plant volatiles in attracting herbivore enemies. Trends Plant Sci. 17, 303–310. doi: 10.1016/j.tplants.2012.03.012
Moreira, X., Petry, W. K., Hernández-Cumplido, J., Morelon, S., and Benrey, B. (2016). Plant defence responses to volatile alert signals are population-specific. Oikos 125, 950–956.
Mumm, R., and Dicke, M. (2010). Variation in natural plant products and the attraction of bodyguards involved in indirect plant defense. Can. J. Zool. 88, 628–667.
Mumm, R., and Hilker, M. (2005). The significance of background odour for an egg parasitoid to detect plants with host eggs. Chem. Senses 30, 337–343. doi: 10.1093/chemse/bji028
Peck, O. (1963). A catalogue of the nearctic Chalcidoidea (Insecta: Hymenoptera). Mem Entomol. Soc. Canada 95, 5–1092. doi: 10.4039/entm9530fv
Pickett, J. A., and Khan, Z. R. (2016). Plant volatile-mediated signalling and its application in agriculture: successes and challenges. New Phytol. 212, 856–870. doi: 10.1111/nph.14274
Pike, K., Starý, P., Miller, T., Allison, D., Graf, G., Boydston, L., et al. (1999). Host range and habitats of the aphid parasitoid Diaeretiella rapae (Hymenoptera: Aphidiidae) in Washington State. Environ. Entomol. 28, 61–71. doi: 10.1093/ee/28.1.61
Pinto, D. M., Blande, J. D., Souza, S. R., Nerg, A.-M., and Holopainen, J. K. (2010). Plant volatile organic compounds (VOCs) in ozone (O 3) polluted atmospheres: the ecological effects. J. Chem. Ecol. 36, 22–34. doi: 10.1007/s10886-009-9732-3
Poelman, E. H., Bruinsma, M., Zhu, F., Weldegergis, B. T., Boursault, A. E., Jongema, Y., et al. (2012). Hyperparasitoids use herbivore-induced plant volatiles to locate their parasitoid host. PLoS Biol. 10:e1001435. doi: 10.1371/journal.pbio.1001435
Poelman, E. H., Oduor, A. M., Broekgaarden, C., Hordijk, C. A., Jansen, J. J., Van Loon, J. J., et al. (2009). Field parasitism rates of caterpillars on Brassica oleracea plants are reliably predicted by differential attraction of Cotesia parasitoids. Funct. Ecol. 23, 951–962.
R Core Team (2013). R: A Language and Environment for Statistical Computing. Vienna: R Foundation for Statistical Computing. Available online at: http://www.R-project.org/
Raguso, R. A. (2008). Wake up and smell the roses: the ecology and evolution of floral scent. Annu. Rev. Ecol. Evol. Syst. 39, 549–569.
Randlkofer, B., Obermaier, E., Hilker, M., and Meiners, T. (2010). Vegetation complexity—the influence of plant species diversity and plant structures on plant chemical complexity and arthropods. Basic Appl. Ecol. 11, 383–395.
Reed, H., Tan, S., Haapanen, K., Killmon, M., Reed, D., and Elliott, N. (1995). Olfactory responses of the parasitoid Diaeretiella rapae (Hymenoptera: Aphidiidae) to odor of plants, aphids, and plant-aphid complexes. J. Chem. Ecol. 21, 407–418. doi: 10.1007/BF02036738
Riffell, J. A., Shlizerman, E., Sanders, E., Abrell, L., Medina, B., Hinterwirth, A. J., et al. (2014). Flower discrimination by pollinators in a dynamic chemical environment. Science 344, 1515–1518. doi: 10.1126/science.1251041
Sandoz, J.-C., Deisig, N., De Brito Sanchez, M. G., and Giurfa, M. (2007). Understanding the logics of pheromone processing in the honeybee brain: from labeled-lines to across-fiber patterns. Front. Behav. Neurosci. 1:5. doi: 10.3389/neuro.08.005.2007
Schiestl, F. P., and Johnson, S. D. (2013). Pollinator-mediated evolution of floral signals. Trends Ecol. Evol. 28, 307–315. doi: 10.1016/j.tree.2013.01.019
Schiestl, F. P., Kirk, H., Bigler, L., Cozzolino, S., and Desurmont, G. A. (2014). Herbivory and floral signaling: phenotypic plasticity and tradeoffs between reproduction and indirect defense. New Phytol. 203, 257–266. doi: 10.1111/nph.12783
Schiestl, F. P., and Roubik, D. W. (2003). Odor compound detection in male euglossine bees. J. Chem. Ecol. 29, 253–257. doi: 10.1023/a:1021932131526
Schröder, R., and Hilker, M. (2008). The relevance of background odor in resource location by insects: a behavioral approach. AIBS Bull. 58, 308–316.
Shiojiri, K., and Takabayashi, J. (2005). Parasitoid preference for host-infested plants is affected by the risk of intraguild predation. J. Insect Behav. 18, 567–576.
Silveira, T., Sanches, P., Zazycki, L., Costa-Lima, T., Cabezas-Guerrero, M., Favaris, A., et al. (2018). Phloem-feeding herbivory on flowering melon plants enhances attraction of parasitoids by shifting floral to defensive volatiles. Arthropod Plant Interact. 12, 751–760.
Szyszka, P., Stierle, J. S., Biergans, S., and Galizia, C. G. (2012). The speed of smell: odor-object segregation within milliseconds. PLoS One 7:e36096. doi: 10.1371/journal.pone.0036096
Taneja, J., and Guerin, P. M. (1997). Ammonia attracts the haematophagous bug Triatoma infestans: behavioural and neurophysiological data on nymphs. J. Comp. Physiol. A 181, 21–34.
Ton, J., D’alessandro, M., Jourdie, V., Jakab, G., Karlen, D., Held, M., et al. (2007). Priming by airborne signals boosts direct and indirect resistance in maize. Plant J. 49, 16–26. doi: 10.1111/j.1365-313X.2006.02935.x
Turlings, T. C., Davison, A., and Tamò, C. (2004). A six-arm olfactometer permitting simultaneous observation of insect attraction and odour trapping. Physiol. Entomol. 29, 45–55.
Turlings, T. C., and Erb, M. (2018). Tritrophic interactions mediated by herbivore-induced plant volatiles: mechanisms, ecological relevance, and application potential. Annu. Rev. Entomol. 63, 433–452. doi: 10.1146/annurev-ento-020117-043507
Turlings, T. C., and Wäckers, F. (2004). Recruitment of predators and parasitoids by herbivore-injured plants. Adv. Insect Chem. Ecol. 2, 21–75.
Unsicker, S. B., Kunert, G., and Gershenzon, J. (2009). Protective perfumes: the role of vegetative volatiles in plant defense against herbivores. Curr. Opin. Plant Biol. 12, 479–485. doi: 10.1016/j.pbi.2009.04.001
Vickers, N. (2000). Mechanisms of animal navigation in odor plumes. Biol. Bull. 198, 203–212. doi: 10.2307/1542524
Wäckers, F. (1994). The effect of food deprivation on the innate visual and olfactory preferences in the parasitoid Cotesia rubecula. J. Insect Physiol. 40, 641–649.
Wäckers, F. (2004). Assessing the suitability of flowering herbs as parasitoid food sources: flower attractiveness and nectar accessibility. Biol. Control 29, 307–314.
Wajnberg, E., Bernstein, C., and Van Alphen, J. (2008). Behavioral Ecology of Insect Parasitoids: From Theoretical Approaches to Field Applications. Oxford: Blackwell Pub.
Wäschke, N., Meiners, T., and Rostás, M. (2013). “Foraging strategies of parasitoids in complex chemical environments,” in Chemical Ecology of Insect Parasitoids, 1st Edn, eds E. Wajnberg and S. Colazza (Chichester: Wiley), 37–63.
Witzgall, P., Kirsch, P., and Cork, A. (2010). Sex pheromones and their impact on pest management. J. Chem. Ecol. 36, 80–100. doi: 10.1007/s10886-009-9737-y
Xu, H., Desurmont, G., Degen, T., Zhou, G., Laplanche, D., Henryk, L., et al. (2017). Combined use of herbivore-induced plant volatiles and sex pheromones for mate location in braconid parasitoids. Plant Cell Environ. 40, 330–339. doi: 10.1111/pce.12818
Keywords: chemical ecology, indirect defense, infochemical networks, plant signaling, VOCs, behavioral ecology
Citation: Desurmont GA, von Arx M, Turlings TCJ and Schiestl FP (2020) Floral Odors Can Interfere With the Foraging Behavior of Parasitoids Searching for Hosts. Front. Ecol. Evol. 8:148. doi: 10.3389/fevo.2020.00148
Received: 19 March 2020; Accepted: 30 April 2020;
Published: 27 May 2020.
Edited by:
Qing-He Zhang, Other, United StatesReviewed by:
Cesar Rodriguez-Saona, Rutgers, The State University of New Jersey, United StatesJunji Takabayashi, Kyoto University, Japan
Copyright © 2020 Desurmont, von Arx, Turlings and Schiestl. This is an open-access article distributed under the terms of the Creative Commons Attribution License (CC BY). The use, distribution or reproduction in other forums is permitted, provided the original author(s) and the copyright owner(s) are credited and that the original publication in this journal is cited, in accordance with accepted academic practice. No use, distribution or reproduction is permitted which does not comply with these terms.
*Correspondence: Gaylord A. Desurmont, Z2Rlc3VybW9udEBhcnMtZWJjbC5vcmc=; Zy5kZXN1cm1vbnRAZ21haWwuY29t