- 1Guangdong Key Laboratory of Animal Conservation and Resource Utilization, Guangdong Public Laboratory of Wild Animal Conservation and Utilization, Guangdong Institute of Applied Biological Resources, Guangzhou, China
- 2Department of Livestock Production, University of Veterinary and Animal Sciences, Pattoki, Pakistan
- 3College of Animal Science and Technology, Huazhong Agricultural University, Wuhan, China
- 4Department of Biotechnology, University of Sargodha, Sargodha, Pakistan
- 5National Key Laboratory of Crop Genetic Improvement, College of Plant Science and Technology, Huazhong Agricultural University, Wuhan, China
- 6Animal Production Department, Faculty of Agriculture, Benha University, Benha, Egypt
Animal domestication is considered a complex and multistage process that altered behaviorally, morphologically, and physiologically the domesticates relative to their wild ancestors. Ever since Darwin, scientists have been concerned about the history of domestication. To determine the domestication origins of the species, it is crucial to discover their ancestors and identify the approximate local domestication. Domestication has been the focus of several studies from different specialties. Studying when, where, and how domestication happened is essential to understand the origins of civilizations and the evolution of domesticated species. The development of both humans and domestic animals is hard to justify, and the genetic variations that occurred during the early animal domestication process remain vague. The recent and potential applications of evolutionary biology may deliver answers for main social challenges. It is important to examine the relationship among the environment and the traits of organisms that have been influenced through the adaptation to modern environments and the patterns of selection triggered by their environments during domestication period. Once domestication occurred, several events such as gene flow and selective pressures occurred, leading to genomic and phenotypic alterations. In this review, we discuss the current knowledge about the spatiotemporal outlines of domestication and debates surrounding the intent, speed, and evolutionary landscapes of this event. We also focus on the core challenges for future research. In conclusion, we argue that although the current growth in domestication information has been remarkable, the next era will produce even more significant insights into not only how domestication occurred but also where and when it did so.
Introduction
Domestication is an evolutionary process by which animals are artificially selected and undergo huge phenotypic behavioral and physiological alterations (Trut et al., 2009). These transformations occurred at the same time in several regions with a tremendous impact on human societies (Neolithization) (Vigne, 2011). Domestication is well known not only for its slow course but also for its extreme techno-economic alterations, from hunting-gathering to food production (Price, 1999). It depends on many important factors, such as strong demographic transition, cultivation, and husbandry of valuable domesticates (Bocquet-Appel, 2008), along with profound social and spiritual changes (Price, 2002).
Dogs (Canis lupus familiaris) were the earliest species domesticated by Asian and European gatherers during the late glacial period approximately 17–15 thousand years (kyrs) before the present (BP) (Pionnier-Capitan et al., 2011; Frantz et al., 2016) followed later by the domestication of livestock and crops (Mignon-Grasteau et al., 2005). Interestingly, while in some species (dogs and cattle), domestication was a human-driven process, for other species (cat, rat, and house sparrow), it occurred naturally (Driscoll et al., 2009). Nowadays, several authors agree that there are approximately 40 animal species domesticated in different geographic areas (Scherf and Pilling, 2015; Leroy et al., 2018).
Differences in morphological features and behavior of most domestic animals from their wild counterparts came about by controlling breeding and persistent animal husbandry. These practices also designed and shaped the diverse genetic makeup among different breeding populations. The variation in the phenotypes of the domesticated animal led to the basis of the Darwinian evolutionary study, which highlighted several queries for further studies, namely: when, where, and how did the domestication of these animals start, and what are the genetic origins of domestication development (Darwin, 1859). Darwin’s initial observations in “On the Origin of Species,” and his variation under domestication began an essential debate for future works (Darwin, 2010). Commonly, for different domestic animals, there are two evolutionary phases: an ancient domestication event where a wild ancestor become a domesticated species followed by a modern breeding process (Driscoll et al., 2009). Ancient domestication occurred approximately 12,000–14,000 years ago, during the agricultural surge of the initial Neolithic period, along with the domestication of major crops (Wang et al., 2014b). On the other hand, modern breeding occurred only in the past 300 years (since the eighteenth century) and started with the selection of breeding animals based on their demand by human societies (Crowley and Adelman, 1998).
In the current review, we try to summarize the existing information on domestication from the literature. We start by addressing the evolution of animal domestication through an evolutionary perspective. We address the roots, demographic history, and impacts on domestication, genetic architecture, and methodologies taken to monitor domestication procedures by selective pressures. We also conclude presenting the challenges to the research and a preview of future directions for this thematic.
Animal Domestication
Animal domestication is the process of a prompt, artificial, and intensive selection of wild animals that, over the last 11,500 years, has altered the Earth’s biosphere, shaped human evolution, and influenced the size of the human population. The foundation of domestication is linked to the cultural progression from hunting to farming in ancient civilizations during the Neolithic period, possibly with the exclusion of dogs, which were the earliest domesticated animals (Savolainen et al., 2002) and diverge from other species regarding both location and timing of domestication (Beja-Pereira et al., 2006; Liu et al., 2006; Driscoll et al., 2009). The periods where animal domestication occurred were determined through archeological clues and reflected selection forces generated by human activities and by human-adapted environments. During the last years, several studies (Chen et al., 2007; Wright, 2015; Hunter, 2018) have focused on animal domestication, and nowadays, it is possible to establish a timeline for the domestication of several animals (Figure 1). Despite this, there are still several questions regarding the timing, location, and the evolutionary domestication process (Larson and Fuller, 2014).
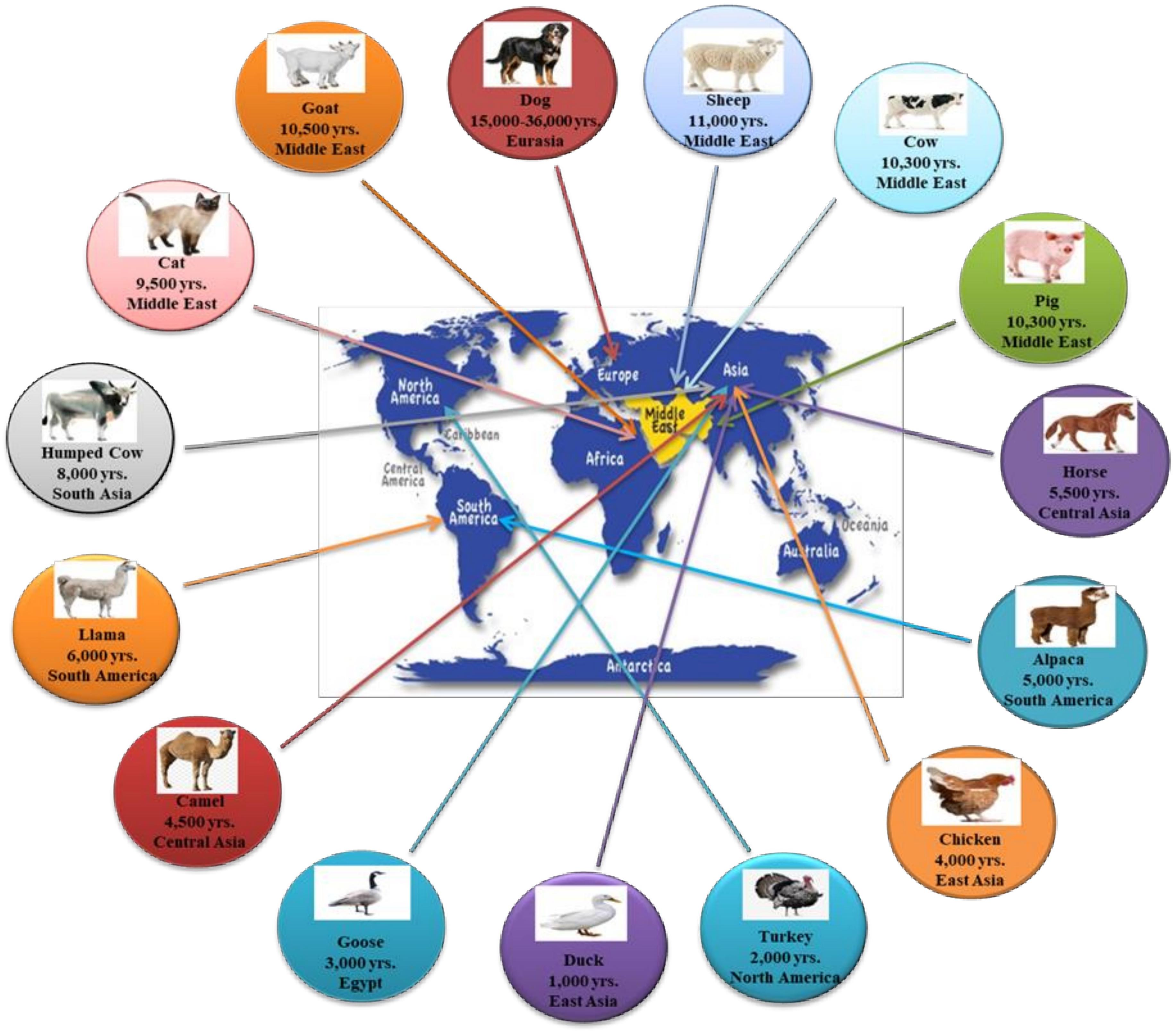
Figure 1. The timeline of animal domestication (adapted from Zeder, 2008).
Since the Neolithic period, humans struggled to domesticate wild animals and use them as food sources (milk and meat), commodity manufacturers (silk and wool), protection, and transportation. There are three pathways described for domestication: commensal, prey, and direct pathways (Zeder, 2012; Figure 2). In the commensal pathway, the wild animals were attracted to anthropogenic habitats, mainly for human food waste or small prey, establishing a commensal relationship with humans. Dogs, cats, or chickens are some of the species that followed this pathway. In the prey pathway, humans start hunting some species like pigs and cattle for their meat in response to depletion of the local stock of these animals. Over time, these game management strategies developed into controlled breeding of these species. In the direct pathway, humans captured wild animals (horses, donkeys, and camelids) to obtain some resources by controlling their movements, their nutrition, and reproduction, which lead to a dramatic bottleneck (Zeder, 2012).
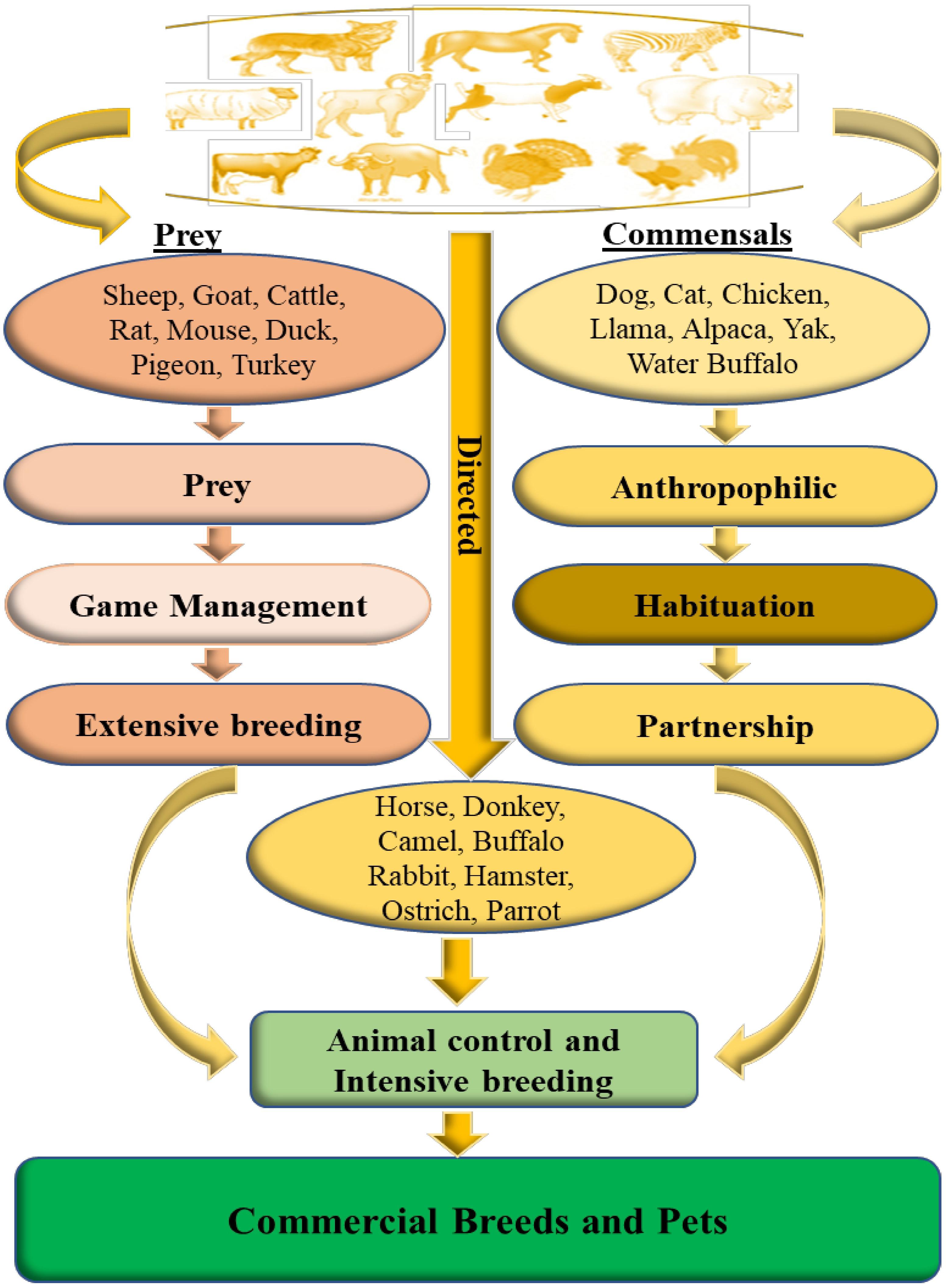
Figure 2. Different pathways from wild to domestic animals (adapted from Larson and Burger, 2013).
Dogs
Dogs were the first animal to be domesticated by humans more than 15,000 years ago. Their wild ancestor is the extinct gray wolf, and despite being intensively studied, there are still questions regarding their geographical and temporal origins and events of domestication. In the literature, there are several places of origin of dogs, including Europe (Thalmann et al., 2013), the Central, Middle East, and East Asia (Pollinger et al., 2010; Shannon et al., 2015; Wang et al., 2016). More recently, a study using mtDNA states that dogs may have been domesticated independently in Eastern and Western Eurasia from different wolf populations. Later the eastern dogs accompanied humans through their dispersion to Western Europe, where they replaced the Western Eurasian and European Paleolithic dogs (Frantz et al., 2016). The same event occurred for American (Leathlobhair et al., 2018) and African (Liu et al., 2018) dogs that arrived with human expansions. These expansions through the world, and the evolutionary dog history, involve bottlenecks and gene flow.
Cattle
Since ancient times, wild cattle and humans are interconnected, and during the last 10,500 years, the number of domesticated bovine species is approximately five (Helmer et al., 2005), resulted in human benefits of meat and milk to drought animals. Recently wild cattle are the source of the genetic pool for domestic breeds’ adaptation to changing pressures of climate and infectious diseases (Melletti and Burton, 2014). Current knowledge of cattle domestication is predominantly based on mitochondrial DNA analysis (Groeneveld et al., 2010). Taurine cattle domestication reported back to the wild and now extinct in the near east, about 8500 years ago, after sheep and goat domestication (Helmer et al., 2005; Bollongino et al., 2012).
Cattle with Longhorn phenotype were the first one to be domesticated; still, this phenotype is common in the number of British, French, Mediterranean, and African breeds to date (Schafberg and Swalve, 2015). First cattle with short horns reported back to 3000 years BC; the phenotype was fit to these habitats and switched by next wave of migrants (Bradley and Magee, 2006). Britain took the most long-horn forms from Asia and neighboring continents about 1000–2000 years BC (Epstein, 1984). In Europe, the most common type of cattle was short-horn until around 1000 BC (Lenstra et al., 2014). Several ecosystems in the various regions of the world are attributed to the domestication and distribution of cattle to and their adaptation to local environments (Lenstra et al., 2014). Moreover, several “agrotypes,” generated by human selection, let the breeds differ in coat color, development of horns, and docility (Ajmone-Marsan et al., 2010). Systematics has accelerated the cattle diversity in the last 200 years and stretched the castles to the main breeds, like with dairy production (Barker et al., 1991) cattle acquired the large udder. The process of the domestication resulted in a continuous decrease of size until the Middle Ages, but it was less pronounced in long-horned Italian forms and draught cattle (Lenstra et al., 2014).
Sheep
Primarily sheep were raised for meat around 4000–5000 years ago but later specified for other foodstuffs. Developments in animal husbandry and application of direct mating systems have evolved a variety of current sheep breeds, not only the most adaptable to the range of climates but also specific to the production of milk, meat, and wool (Chessa et al., 2009; Kijas et al., 2012). Mongolian sheep origin is the wild Argali sheep from highland areas of Central Asia. More than 2,000 years ago, many populations had moved to the south of the Great wall for several reasons; hence the most present Chinese breeds are linked to Mongolian sheep (Huang et al., 2017). However, with exposure to a changing environment, and feeding situations to various eco-regions across the country than normal habitat, Mongolian Sheep faced extensive artificial selection in diverse orders (Liu et al., 2016).
The subspecies of Mongolian sheep display substantial changes in several traits, particularly associated with reproduction, but how species diverge natively relative to these characters is not well interpreted (Huang et al., 2017). These studies identified the genes of significant importance for domestication process (Petersen et al., 2013b; Carneiro et al., 2014), capability to withstand the harsh climates (Gou et al., 2014; Wang et al., 2014a), or conspicuous economic characters (Choi et al., 2014; Yang et al., 2014; Wang et al., 2015). Tibet wild pig (Li et al., 2013) and Tibet mastiffs (Gou et al., 2014; Wang et al., 2014a) have been a subject for popular studies from Tibetan Plate, and linked many genes for adoption to high altitude and hypoxia. Several studies have explored the genome-wide difference among various indigenous breeds to fix the molecular basis of various physical traits of vital importance in the livestock, such as chicken (Fan W.-L. et al., 2013), pig (Yang et al., 2014; Wang et al., 2015), and cattle (Choi et al., 2014). However, the studies for Chinese short fat tail sheep are limited.
Goat
Fertile Crescent Land has reported as the first domestication habitat for goat (Capra hircus) in Near East almost < 10,000 years ago by various genetic and archeological studies (Pringle, 1998). The domestication of goats has a significant role in human society by providing valued products such as milk, meat, furs, and fiber, predominantly in China and other developing countries (Joshi et al., 2004). During the epoch, these domestic breeds rich in genetic assets incited us to pay more consideration; consequently, keeping the domestic animal variety is imperative to accomplish the forthcoming necessities.
Concerning precise economic and environmental features, China has started focusing on conservation strategies for these native breeds, including specified conservation zone, conservation farms, and the gene bank of the genetic reserve for distinctive breeds (Wei et al., 2014). These highlands had allocated West China into Southwest and Northwest China, and consequence to the diverse climatic regions and ecological structure. China is an extensive subcontinent of merged topographical locations, so Chinese goat breeds exhibit a great range of variation in productivity, milk production, meat, and fiber; draught ability; heat tolerance; and disease resistance. Besides, several preceding studies on Chinese goats were conducted in the restricted number of trials within fewer breeds and counties (Di et al., 2011; Wei et al., 2014).
Cat
A recent evolutionary study of domesticated cats using DNA analysis suggested thousand years of interaction between the cats and humans before their domestication, and this interaction let the cats’ genes changed pretentiously from those of wild cats except the development of unique stripes and spots of the tabby (O’Brien and Johnson, 2007). Evaluation of the DNA of cats bridging the last 9,000 years – counting the remains, mummies, and specimens of cats from ancient Roman, Egypt, and modern African wildcat – suggested the current domestic feline have a great contribution of two major cat lines (Ottoni et al., 2017).
Thus early 4400 B.C., earlier ancestors of our domestic cats spread from Southwest Asia and into Europe (Ottoni et al., 2017). Farming communities settled the cats to control the rodent patrol. Mice and rats were drawn to the crops, and the rodent populations were possibly pursued by cats (Montague et al., 2014). In turn, both often came close to the human settlement. Hence, the domestication changed the wild cats to a domesticated human companion without changing much, says Eva-Maria Geigl, the evolutionary geneticist and article co-author (Ottoni et al., 2017). Domesticated cats look like wildcats, but they are not lonely and capable of tolerating both humans and other animals (O’Brien and Johnson, 2007).
Horses
The date of horse domestication is conceived from the definition of domestications (Cieslak et al., 2010). Two theories of domestication are as follows: first, the human control over breeding depicted by changes in the size and variability of ancient skeletal samples from ancient horse populations, and second includes broader evidence of skeletal and dental activity weapons, arts, and spiritual artifacts; and patterns of human lifestyles (Ross-Ibarra, 2004). Evidence claim horses were kept as meat animals before trained as working animals (Hausberger et al., 2008). The concept of isolated genotypes between domesticated and wild populations leads to exploring the domestication attempts using genetics or physical traits examination (Rollin, 2011). But these methods could only find the latest footprints of domestication but failed to determine uncertain primitive gene flow between the two groups (which occurs naturally as long as the domesticated population remains within wildlife habitat) (Vilà et al., 2001). Furthermore, being descendent of captive-fledged ancestors, all the domesticated horses, and feral horses are capable of retaining either characteristic (Dobbie and Braysher, 1993). Time frames chosen for the horses’ domestication are influenced equally, either following the narrower zoological concept of domestication or the broader cultural definition, i.e., the combination of zoological and archeological facts (Aberle and Distl, 2004). The date of 4000 BCE is based on evidence that includes the appearance of bite-related dental pathologies, changes in butchering practices, changes in humans’ economies and patterns of settlement, the depiction of horses as symbols of power in artifacts, and the appearance of horse bones in human graves (Larson and Burger, 2013).
Buffalo
The domestication process led to the adaptation of various bovine species to an agricultural environment, and the most important species are indicated and taurine cattle followed by swamp and riverine and buffalo (Barker, 2014). These species have ranged to several regions, while the domestic forms of gaur and yak are grouped near their wild ancestors’ sharing areas (Groves, 1981). The genus Bubalus distribution was initially started in the Pleistocene, Europe, and South Asia, but was later constrained to southeast Asia and the Indian subcontinent (Flamand et al., 2003). In ancient times, the wild Asian buffalo (Choudhury and Barker, 2014) spread across southeast Asia to Indo-China. It is presently listed as an endangered species, with a world population lesser than 4,000, possibly fewer than 2001. Domestic water buffalo (B. bubalis) has been grouped into two types: river and swamp based on behavioral and morphological criteria (Barker, 2014). Some have referred to these as distinct subspecies, naming swamp buffalo B. bubalis carabenesis and riverine B. bubalis bubalis. However, because they resulted from different domestications, here they are designated distinctly. The same applies to the swamp and river types of water buffalo, the cross-fertile subspecies of the wild Bubalus arnee (Groeneveld et al., 2010; Yindee et al., 2010).
Chicken
The history of domestic chicken species can be discussed in three different periods of time. The longest period is the evolutionary history, shared with other species before speciation, and along with various courses after speciation. The currently shared ancestor of birds and mammals existed 300 my ago (Tixier-Boichard et al., 2011). The most common ancestor between chicken and quail lived 40 my ago (Mwacharo et al., 2013). The second period of species domestication starts several thousand years ago and led to domestic species diversification. The recent period of rigorous production trait selection applied to a subset of these breeds is shorter, with just a few decades (Kanginakudru et al., 2008). Therefore, on an evolutionary scale, domestication and, more significantly, a good selection for higher levels of production are very important (MacDonald, 1992). Current domestic chickens’ genome diversity is a result of the founding effects at the time of domestication, the long-term domestication process, subsequent breed differentiation, and recent strong production selection (Nishibori et al., 2005). The cumulative effect of human-made domestication and subsequent selection has given rise to a remarkable phenotypic diversification of the chicken, both at the molecular level (MacDonald, 1992).
Domestication and Evolution
Domestication has been a core question of interdisciplinary scientific research, and those traits that enhance the survival or the reproductive competence of the organisms are subject to selection and transferred to the next generation to increase the population prevalence (Vitti et al., 2013). Since 2006, the study the domestication through complete genome sequence has become possible, and it has been associated with the detection of selection in a large number of genomic loci that have likely evolved by selective pressures (Carneiro et al., 2014; Larson et al., 2014). Carneiro et al. (2014) studied genes involved in brain and neuronal development and proposed that domestic animals evolved by accumulating several mutations with small effects instead of critical alterations in a few loci. Later in 2016, Messer et al. (2016) reviewed some limitations to this model and provided some examples of studies using fishes, bugs, and reptiles where the phenotypic traits altered in a few generations. Strong animal husbandry practices and controlled breeding have shaped the behavior, the morphological features, and the genetic diversity of domestic animals when compared to their wild ancestors (Wang et al., 2014b). Several alterations were observed across different domestic species and captivated researchers at least since Darwin’ findings, namely lack of fear; enhanced reproductive system (able to reproduce in any season); disparities in the coat length, texture, and color; modifications in skull form, tooth crowding, and corporal sizes; floppy ears, and rolled tails (Driscoll et al., 2009; Trut et al., 2009; Larson and Fuller, 2014; Wilkins et al., 2014). These characteristics make domesticated animals valued models for different areas with highlights in genetics and biomedical research (Andersson, 2016; Wolf et al., 2018): descendants are commonly known, samples are usually unlimited, many breeds are inbred, gene variants responsible for particular phenotypic characters are fixed, and the genetic differences (a requirement for selection) are limited (Andersson, 2001; Gentry et al., 2004; Lyons et al., 2016). All these differences are associated with genetic events as inbreeding, gene flow, and selection pressures. Inbreeding leads to a decrease in genetic diversity and is associated with the isolation of small populations at the beginning of the domestication process (Cieslak et al., 2011). There is evidence of long-term gene flow between wild and domestic animals such as donkeys, horses, camelids, pigs, wolves, cats, and the reindeer (Marshall et al., 2014; Frantz et al., 2015; Bolstad et al., 2017). Once domestication became established, a relaxation in the natural selective pressures (both environmental and induced by humans) enabled the increase of new mutations (mostly non-synonymous mutations), leading to even more differentiated species (Petersen et al., 2013a). Despite this, and due to the multitude of selective pressures involved, it is difficult to isolate any causal factors that result in specific genetic differences (Zeder, 2015). Over the past 40 years, several species (mostly livestock) have been intensively selected, and notable phenotypic variations have been observed. Since many of these genetic mutations lead to phenotypic alterations, identification of the signatures of positive selection is considered a valuable tool to recognize genes that might underlie important traits allowing to link genetic variants to a particular phenotype (Consortium, 2009).
As some authors suggest, domestication started unconsciously (Tchernov and Horwitz, 1991), and later a conscious selection of human-defined traits led to a high level of diversity (Trut et al., 2009). Indeed, during the initial period of their domestication, horses were mainly used for meat and milk (Outram et al., 2009), and later they became important for transportation, warfare, and sport horseracing. Another example is the dog domestication process that focused not only on preferred physical characteristics, such as body thickness and body length, texture, skull shape, tail size and shape (Wayne, 1986), but also on improved behavioral patterns, with advanced features of guarding, herding, speed, agility, and companionship (Ostrander et al., 2000). Indeed, all over the domestication process, studies suggest that humans did not maintain constant selective pressures. Most likely, they selected different traits in different places at different times. Thus, extra care must be taken since the discovery of certain traits in current breeds does not necessarily mean that the trait was a target during the early domestication (MacHugh et al., 2017).
Domestication Studies
Domestication has fascinated scientists from different fields through its importance as a model of evolutionary and demographic change (Zeder et al., 2006). Ever since Darwin, scientists have been concerned about the history of domestication. To determine the domestication origins of the species, it is crucial to discover their ancestors and identify the approximate local domestication. For example, dogs were domesticated before the start of agriculture from gray wolves (Larson and Fuller, 2014). However, gray wolves were disseminated across the Northern hemisphere, hampering the findings on how, why, when, and where dogs were domesticated and if this process occurred just once or independently at different times (Larson, 2017). Over the years, different genetic methodologies have been used to explore these questions (Larson, 2011).
In the first studies, DNAs were extracted from samples of a given species (different locations, breeds, and populations) and used to amplify and sequence control-regions of the mitochondrial genome. Additionally, phylogenetic trees and haplotypes networks were generated (Larson, 2011). Mitochondrial DNA (mtDNA) is considered an ideal marker, being extremely mutable within species and has been used to study demographic expansion, genetic diversity, and phylogenetic structure (Bruford et al., 2003). However, these sequences are derived from the maternally inherited genome and have a limited power to identify and quantify hybridization between different populations (Larson, 2011). This approach was intensively used for several species as dogs (Verginelli et al., 2005), pigs (Giuffra et al., 2000), horses (Jansen et al., 2002), and cattle (Loftus et al., 1994), however with no deep insights into their domestication process. Some authors suggest that recent selective breeding may contribute to undermining the signatures of mtDNA between domesticates and their ancestors (Librado et al., 2016). Additionally, quantitative trait loci (QTL) mapping has been used to identify candidate genes associated with domestication traits. With these analyses, there have been some improvements in the domestication process, namely for fox (Kukekova et al., 2011), chickens (Fallahsharoudi et al., 2017), pigs (Rodriguez et al., 2005), and cattle (Khatkar et al., 2004).
GWAS, NGS, Microarray, and mtDNA
In domestic animals, the genome-wide association studies (GWAS) has become an important method to study the genomic regions involved in traits of concern with the sequencing of the pig, cow, and dog genomes (Lindblad-Toh et al., 2005; Groenen et al., 2012; Hekman et al., 2015). This method investigates the likelihood of the association of the genetic markers with a specific trait in domestic animals (Cadieu et al., 2009). The variation in these traits is due to intensive selective pressure elucidated by a small number of loci in these species (Boyko et al., 2010). Consequently, in several domesticated species, GWAS have effectively recognized contributing genes both for complex traits and Mendelian traits controlled by loci with big influence size (Hekman et al., 2015). The use of genome-wide methods initiated the microarray gene expression studies in the search for a group of genes or gene linkages involved in composite phenotypes in domesticated animals (Everts et al., 2005). However, the use of microarray techniques is narrow due to their dependencies on the use of identified probes, needing species-specific markers for the most precise results (Hekman et al., 2015). The introduction of next-generation sequencing (NGS) technologies has revolutionized the gene expression research by removing the need for prior probes for transcripts. RNA-seq or RNA sequencing requires the high-throughput reads generated by NGS to characterize the whole transcriptome: in other words, all transcripts generated in a tissue sample plus novel isoforms and formerly uncharacterized recorded sequences (Allen et al., 2010). RNA-seq is generally used for a range of applications, such as to compare the group of differentially expressed genes in tissue samples or samples from different experimental groups or populations (Bottomly et al., 2011; Roy et al., 2013). Based on NGS or RNA-seq data, the individuals can be grouped into healthy or diseased by identifying the gene linkages associated with hereditary diseases or other genetic traits or by mapping various genetic loci (Gautier et al., 2012; Tonomura et al., 2015). Furthermore, mtDNA technology was used to recognize the genomic regions associated with important phenotypic traits as well as to identify the evolutionary history and the origin of domestication in animal species as compared with nuclear markers (MacHugh and Bradley, 2001; Akey et al., 2010). Since 2000, sequences of mtDNA fragments such as D-loop and cytochrome b regions have been used to study distribution of different domesticated animals, including dogs (Savolainen et al., 2002), sheep (Hiendleder et al., 2002), pigs (Giuffra et al., 2000), cattle (Troy et al., 2001), goats (Luikart et al., 2001), horses (Jansen et al., 2002), chickens (Liu et al., 2006), donkeys (Beja-Pereira et al., 2004), and cats (Driscoll et al., 2007), as well as those with more restricted dispersals, such as water buffalo (Kierstein et al., 2004), and zebu cattle (Chen et al., 2010). mtDNA research has provided a viewpoint, at least from the parental side, as to the probable ancestors and candidate pedigrees involved in the domestication of species (Wang et al., 2014b).
Understanding the genetic basis of phenotypic variations is the major aim of genetics. Domestic animals offer a subjective opening for making significant improvement toward the goal of minimizing the gap between human biology and traditional model species (Qanbari and Simianer, 2014). The continuous progress of high throughput sequencing technologies and modern bioinformatics approaches provide the complete genetic variation map of an individual, and it is now likely to test for phenotypic variation caused by single nucleotide polymorphisms (SNPs). The genome-wide association studies (GWAS) and candidate gene approach are two primary methods that are currently followed to recognize genomic regions or genes affecting the particular trait. Population genomics has presented a new model for linking DNA with a phenotype that has been revealed as a selection signature analysis. This is a genome to phenotype method that includes the statistical assessment of population genomic data irrespective of phenotype to find out targets of the previous selection. Selection analysis can be employed in the natural population so numerous species (Akey et al., 2010) for which a high-density genetic record is available. An additional benefit is that it can detect selection if the preferred allele is previously fixed, while GWAS fails in such a condition (Qanbari and Simianer, 2014).
SNP Chip
In modern researches, through using recent genomics technologies, it has become likely to explore the micro-evolutionary developments underlying animal domestication at the molecular level. In this regard, various studies that have been done to produce domestic red fox, silver foxes (Vulpes vulpes), and rats (Rattus norvegicus) have provided valuable understandings that were introduced during the middle of the twentieth century (Albert et al., 2009, 2011; Trut et al., 2009). Furthermore, functional genomics, quantitative real-time PCR, microarray and reverse transcription studies of brain tissues from domestic dogs and wolves, transcriptional profiling through RNA-sequencing of rat brains combining genome mapping studies have identified numerous candidate genes and putative regulatory regions that have influenced docility and viciousness in animals (Heyne et al., 2014). It is necessary to annotate that the acute changes in gene expression linked to domestication possibly affect the growing stages in a specific tissue and will require extensive work to be conclusive (Carneiro et al., 2014). Gene enrichment analysis recognized that neurobiology is affected by the loci of those genes that were over-represented and targeted by directional selection, and principle functional analyses exposed that derived single nucleotide polymorphisms in developmental genes (PAX2 and SOX2) were possible to be fixed within, or close to, regulatory sequences. Most remarkably, it was concluded that domestication was predominantly associated with selective sweeps causing genetic variations on regulatory regions throughout the animal genome, therefore indicating micro-evolutionary developments during the initial periods of domestication of vertebrate species (Carneiro et al., 2014). Understanding the significance of single disease-associated SNP alleles itself is neither necessary nor enough in causal of a disease. Rather, it is possibly the collective consequence of a set of SNP alleles enclosed by key genes, plus environmental factors that jointly conclude whether an individual experiences a certain disease. The association study process includes the frequency determination of test factor (e.g., an SNP allele) among several patients and in the race and age-matched controls. The determination of the validity of this test crucially depends on appropriate patient-to-control matching (population stratification). Another way of performing association studies more efficiently is by limiting the SNPs through pre-selection by testing the pathogenic effects of SNPs. For the betterment of association studies design, one way is to exploit pathogenic allele’s linkage disequilibrium. When there is strong linkage disequilibrium among the SNP marker and an unknown pathogenic allele, both can show a parallel association with the disease (Pruvost et al., 2011; Corbett-Detig et al., 2015).
Nuclear Genes Epigenetics
More recently (since 2006), “next-generation sequencing” (NGS) technologies allowed access to the whole-genome sequences and gained new information about timing and location of domestication (Wang et al., 2014b). These approaches expanded the scope of comparative genomics from single genes to gene families and entire genomes. Mutations in DNA can vary from a single polymorphism to gene duplication or even complete genome duplication leading to many consequences, including phenotypic alterations. Additionally, a single gene can be involved in multiple, unrelated phenotypes (Hodgkin, 2002; Hartl, 2009), and genes of polygenic traits can act in combination to produce a single phenotype. The variations within the gene could be triggered by nucleotide exchange (non-synonymous or synonymous) or indels, which can generate adaptive, negative, or neutral alterations in the gene. More recently, these approaches allowed the availability of genomes for several species and permitted the association of genes to specific traits of domestication in cattle (Yurchenko et al., 2018), rabbits (Carneiro et al., 2014), sheep (Zamani et al., 2018), pigs (Rubin et al., 2012), horses (Zhang et al., 2018), dogs (Pendleton et al., 2018), and ducks (Zhou et al., 2018). Several other studies focus on the search for signatures of selection in those genes already described as important for the domestication process (Neves et al., 2014; Ahmad et al., 2017a, b).
Paleogenomics, also known as genome-wide ancient DNA (aDNA) analysis, gives valuable information and has been crucial to investigate when, where, and how rapidly adaptive alleles spread in the populations (Irving-Pease et al., 2018; Brunson and Reich, 2019). Indeed, recent studies using this approach provide new insights into the evolution and history of the cave bear (Barlow et al., 2018) and horses (Gaunitz et al., 2018). In livestock, biotechnology and conservative tools have contributed considerably to improve productivity, preserve genetic diversity, and enhance the adaptation to the environment (Ko and Takahashi, 2006). Furthermore, functional genomics, quantitative real-time PCR, microarray, and reverse transcription studies identified many candidate genes and putative regulatory regions that have influenced docility and viciousness in animals (Heyne et al., 2014).
With these advances in technology, the genetic architecture of domestication and the domestication process in some species became clearer (Caliebe et al., 2017; Kemp et al., 2017; Pitt et al., 2019). For example, it was possible to discover that pig populations were domesticated in one place and then moved to new areas successively gained the mitochondrial signature of native wild populations (Ottoni et al., 2012). The same applies to other taxa. These new approaches also allowed to verify that African cattle are mixtures of “taurine” and “indicine” that possess both Y-chromosome signature and mitochondrial signals (Larson and Fuller, 2014).
Genomics and the Domestication Process
The development of genome technologies such as genome assembly by sequencing, whole-genome shotgun (WGS) method (Lindblad-Toh et al., 2005), NGS (Dong et al., 2013), and third-generation single-molecule sequencing tools (Koren et al., 2012) are outstanding approaches are advancing our understanding to study animal domestication. Genome sequencing of domesticated species not only provides important resources to answer the queries raised by Darwin but also provides prospects to discover the genetic origin of profitable traits in domesticated species (Hillier et al., 2014). The current information on genome projects highlights the demographic history, origins, and the artificial selection of domesticated animal species (Anthony et al., 1986). Additionally, it was determined with a preview of future guidelines for animal domestication. Various techniques were established to allow the de novo assembly of genomes during the human genome project (Venter et al., 2015). The most effective technique was WGS sequencing, along with the building of physical maps. By 2009, four domesticated animals (horse, cat, dog, and taurine cattle) genomes and one wild species genome (the red jungle fowl) were sequenced and assembled based on this method (Wallis et al., 2004).
The domestication process is demonstrated through the detection of selection at a very large number of genomic loci that have likely evolved by natural and artificial selection (Carneiro et al., 2014; Larson et al., 2014). Recently, it has become possible to explore the domestication process by complete genome sequences analyses at high resolution to define how it has formed modern domestic animal. Several genomic loci such as the DGAT1 gene that is associated with lactation traits comprise a major quantitative trait nucleotide has been identified putatively under the selection through genome-wide comparisons of data from modern taurine and indicine cattle (Park et al., 2015). Furthermore, 106 candidate genes were found under selection using population genomics approaches that were particularly involved in muscle development, growth, function, and immunity. This first genome-wide selection analyses detected genes that are considered as important candidates of domestication (Ludwig et al., 2015). However, many methods are available to retrieve and sequence genomes routinely and recognize hundreds of genomic loci under selection through genotyping. It is revealed that paleogenomics techniques will be used to investigate when, where, and how rapidly adaptive alleles spread at the population in domestic animals (MacHugh et al., 2017). Several arithmetical methods have been established by scientists to reveal diverse features of how to accomplish variations from and what is anticipated with respect to genetic differences in the neutral model (Voight et al., 2006). While all statistical methods are based on neutral genomic differences, not all of them are based on similar information. Most of the methods were established for complete sequence data and not for genome-wide pools of predetermined SNPs, which are presently accessible in few livestock species (Corbett-Detig et al., 2015).
Epigenetics in the Evolution of the Domestic Traits
Evidence illustrates that in addition to genetic factors, epigenetic factors can affect the behavioral phenotypes as well as other traits within breed or species (Jensen, 2015; Bélteky et al., 2018). For example, the difference in the behavior of the great tit is statistically linked to the DNA methylation at dopamine receptor genes (Verhulst et al., 2016). Similarly, maternal behavior affects the DNA methylation of the hippocampal glucocorticoid receptor gene in rats (Weaver et al., 2004). In domestic chickens, variances in DNA methylation are linked to disease exposure (Tian et al., 2013), immune reactions (Berghof et al., 2013), metabolism, and growth (Hu et al., 2013).
Furthermore, epigenetic changes can occur just after individuals are exposed to various rearing environments (Pértille et al., 2017). Although cell division maintains the DNA methylation patterns, sometimes these could be regulated by external stimuli (Raynal et al., 2012). The DNA methylation changes that are controlled by the environment can be transferred through the germline (Guerrero-Bosagna et al., 2010) and remain unchanged over generations in somatic tissues (Franklin et al., 2010; Goerlich et al., 2012). Somatic epigenetic changes can affect phenotypic traits, whether selected deliberately or involuntarily or formed by the environment. Therefore, mechanisms of epigenetic changes could be an essential factor in the development of prompt phenotypic variations that arise during domestication. For example, these methylation changes are controlled by substantial hyper-methylation in domestic white Leghorn chickens as compared to the red jungle fowl (Nätt et al., 2012), in pedigree dogs compared to wolves (Janowitz Koch et al., 2016), and in domestic compared to wild worms (Xiang et al., 2013).
Domestication Genes
As described above, selection played a crucial role during domestication accelerating phenotypic variations. Skin and coat color are considered the only domestic traits subject to early selection by humans, thus becoming an essential genetic marker. Coat color can have patterned (spotted, striped) and non-patterned (solid colors) phenotypes that are defined by the proportion of two pigments: eumelanin (black/brown) and pheomelanin (red/yellow) (Cieslak et al., 2011; Koseniuk et al., 2018). During the past years, this has been a central question in domestication studies, and MC1R, ASIP, TYRP1, CBD103, KIT, and PMEL17 genes were associated with different traits in several species (Table 1). A variety of mutations, including single nucleotide polymorphisms (SNPs), insertions, deletions, and duplications are responsible for coat color discrepancies in both domestic and wild populations (Cui et al., 2018).
With the advances in technology and the knowledge on domestication, recent studies were able to associate Tph1 and Gabra5 genes to tameness and to produce tamed animals, namely foxes (Vulpes vulpes) and rats (Rattus norvegicus) (Albert et al., 2009, 2011; Trut et al., 2009). Besides, several other genes have been associated with different phenotypes in different animals. In dogs, genes associated with wrinkled skin (HAS2), body size (IGF1), leg length (FGF4), and fur growth and texture (FGF5, RSPO2, and KRT71) were identified and reported (Sutter et al., 2007; Cadieu et al., 2009; Parker et al., 2009; Hellström et al., 2010). In cattle (Grobet et al., 1997), sheep (Clop et al., 2006), pigs (Stinckens et al., 2008), goat (Zhang et al., 2012), horses (Dall’olio et al., 2010), and dogs (Mosher et al., 2007), a mutation in the MSTN gene is related to increased muscular development (Ahad et al., 2017). Also, in cattle, the DGAT1 and ABCG2 genes are responsible for variations in milk production and composition, respectively (Ogorevc et al., 2009). The HMGA2 and LCORL-NCAPG genes are associated with stature and body size in cattle (Pryce et al., 2011), rabbits (Carneiro et al., 2017), pigs (Rubin et al., 2012), horses (Frischknecht et al., 2015), and dogs (Jones et al., 2008). Another trait that has been the focus of several studies is the meat tenderness with genes associated with cattle (CAST2, HSP90AA1, DNAJA1, and HSPB1) (Malheiros et al., 2018) and pigs (CAST, HAL, RYR1, and RN).
Challenges and Prospects
Ancient information-rich biomolecules such as DNA and proteins have long been under discussion and systematically analyzed by the scientists (Pääbo et al., 1989). In the early 1980s, the DNA cloning was made possible through a cumbersome molecular cloning approach (Higuchi et al., 1984), which eventually proved unreliable, particularly making fake DNA sequences from a 2400-year-old Egyptian mummy (Pääbo, 1985). In 1980s, the amplification of a DNA from archeological material and museum samples using polymerase chain reaction (PCR) method brought an important revolution (Pääbo et al., 1988). However, it was a big challenge that got attention in scientific community to recover reliable and reproducible DNA (Thomas et al., 1989). Therefore, the DNA field has been affected with major technical hurdles such as the presence of inhibitors of enzymatic reactions, post-mortem loss, contamination of preserved samples, and all the influences that can irreversibly contain the validity and reproducibility of a DNA amplified from archeological specimens (Lindahl, 1997; Cooper and Poinar, 2000). However, the field of archaeo-genetics has been developed over the last four decades, scientists have scientifically tackled the methodological challenges related to DNA recovery from long-dead materials, and it is now well-known that an authentic and reproducible genetics information can be produced from the fossils of vertebrates. Thus a DNA research has had an ancient concern in understanding the biology and evolution of domestic animals and their wild ancestors (Troy et al., 2001; Leonard et al., 2002).
Domestication research has challenged scientists since Darwin, and despite the amount of new literature published every year, there are still many questions regarding this thematic. This endless process began several million years ago and included diverse pressures that shaped animals in different ways and differentiate them from their ancestors. Genes linked with coat color were the ones associated with early domestication, being widely studied. With this exception, no other genes were associated with the early stages of animal domestication. Recent studies described that animals were firstly selected based on behavioral characteristics, making hard the research when compared to morphological traits. Indeed, if we look at the crop and plant domestication where the insights obtained in the past years, with the same approaches, are significant when comparing to animals.
With the advances in sequencing and assembly technologies, genomes from different domestic and wild animals are becoming accessible. In addition to these, genomes from ancient populations are becoming available. Genetic research has a wide range of toolkits to explain not only the relationships between the domesticated animals and their wild ancestors but also the domestication traits and their genetic architecture. These results are essential to compare the patterns in the modern populations with those of the previous generations and data from phylogeography and also to identify new genes and associate them with specific traits. Methylomics and transcriptomic analyses are essential to study the epigenetic factors and expression present in wild and domesticated animals to support the variations linked to domestication.
The recent and potential applications of evolutionary biology may deliver answers for main social challenges. It is important to examine the relationship among the environment and the traits of organisms that have been influenced through the adaptation to modern environments and the patterns of selection triggered by their environments during domestication period. A conceptual perspective connecting all of these environmental, genetic, and developmental manipulations is expected to lead to better application and cross-disciplinary incorporation of functional evolutionary approaches to study domestication of animals and their relationship to wild ancestors. It is important to highlight the evolutionary plans/policies that may be used to accomplish required targets of sustainable development for better health, use of natural resource and biodiversity conservation, including how domestication conflicts have been reduced to accomplish preferred outcomes. Therefore, the facts of building a more integrated field of evolutionary biology must be underscored to address global challenges of domestication. Human impact on the biosphere has deep consequences for both direction and the rate of evolution during evolution. At the same time, animals and other organisms that are worthy for ecological, aesthetic or economic reasons are often not able to adapt rapidly enough to keep pace with changes of the environment impacted by human activities. These modern dilemmas progressively threaten animal health, biological diversity, and domestication history. Meanwhile, the problem of earth’s sixth mass extinction of species becomes impending as species are inept to adapt quickly to environmental variations. A developing interest of evolutionary biology may help us to improve our skills to cope with challenges to solve most of pressing problems of domestication of animal species during the twenty-firstcentury.
Future works should apply these technologies and obtain genomes of a large number of individuals inside different species worldwide in order to better comprehend the genetic, morphological, and behavioral characteristics of different species. With these achievements, we hope to fully understand when, where, and how animals where domesticated and consequently understand the human civilizations.
Conclusion
Although the genetic and geographic pattern of early animal domestication is poorly understood, a clear background for understanding the evolutionary routes of domesticated animals is progressing. The early stages of animal domestication show an extended coevolutionary progression with various phases along diverse trajectories that enhanced the reproduction and survival of domesticates. Natural selective pressures relaxed, and new mutations arose and allowed for unique traits.
Our understanding of the genetic basis of animal domestication facilitates improvements through breeding using new techniques. Defining important events of domestication delivers a unique aspect in studying the linkage between humans and the natural world and determines the events that drive human cultural evolution to interact with that leading biological evolution.
Author Contributions
JC, NA, AE, SA, and MA: conception and design of the work. HA: data acquisition. HA, MA, and FJ: analysis. SA: writing of the manuscript. HA, NA, and FJ: critical review.
Funding
This work was funded by the GDAS project of Science and Technology Development (2019-GDASYL-0103059 and 2018GDASCX-0107).
Conflict of Interest
The authors declare that the research was conducted in the absence of any commercial or financial relationships that could be construed as a potential conflict of interest.
Acknowledgments
We thankful to reviewers for their comments and critical reading of the manuscript.
Footnotes
References
Aberle, K. S., and Distl, O. (2004). Domestication of the horse: results based on microsatellite and mitochondrial DNA markers. Archiv. Fur. Tierzucht. 47, 517–536.
Ahad, W. A., Andrabi, M., Beigh, S. A., Bhat, R. A., and Shah, R. A. (2017). Applications of myostatin (MSTN) gene in the livestock animals and humans: a review. Int. J. Curr. Microbiol. App. Sci. 6, 1807–1811.
Ahmad, H. I., Liu, G., Jiang, X., Liu, C., Chong, Y., and Huarong, H. (2017a). Adaptive molecular evolution of MC 1R gene reveals the evidence for positive diversifying selection in indigenous goat populations. Ecol. Evol. 7, 5170–5180. doi: 10.1002/ece3.2919
Ahmad, H. I., Liu, G., Jiang, X., Liu, C., Fangzheng, X., Chong, Y., et al. (2017b). Adaptive selection at agouti gene inferred breed specific selection signature within the indigenous goat populations. Asian Austr. J. Anim. Sci. doi: 10.5713/ajas.16.0994
Ajmone-Marsan, P., Garcia, J. F., and Lenstra, J. A. (2010). On the origin of cattle: how aurochs became cattle and colonized the world. Evol. Anthropol. 19, 148–157.
Akey, J. M., Ruhe, A. L., Akey, D. T., Wong, A. K., Connelly, C. F., Madeoy, J., et al. (2010). Tracking footprints of artificial selection in the dog genome. Proc. Natl. Acad. Sci. U.S.A. 107, 1160–1165. doi: 10.1073/pnas.0909918107
Albert, F. W., Carlborg, O., Plyusnina, I., Besnier, F., Hedwig, D., Lautenschläger, S., et al. (2009). Genetic architecture of tameness in a rat model of animal domestication. Genetics 182, 541–554. doi: 10.1534/genetics.109.102186
Albert, F. W., Hodges, E., Jensen, J., Besnier, F., Xuan, Z., Rooks, M., et al. (2011). Targeted resequencing of a genomic region influencing tameness and aggression reveals multiple signals of positive selection. Heredity 107, 205–214. doi: 10.1038/hdy.2011.4
Allen, H. L., Estrada, K., Lettre, G., Berndt, S. I., Weedon, M. N., Rivadeneira, F., et al. (2010). Hundreds of variants clustered in genomic loci and biological pathways affect human height. Nature 467, 832–838. doi: 10.1038/nature09410
Almathen, F., Elbir, H., Bahbahani, H., Mwacharo, J., and Hanotte, O. (2018). Polymorphisms in MC1R and ASIP genes are associated with coat color variation in the arabian camel. J. Hered. 109, 700–706. doi: 10.1093/jhered/esy024
Andersson, L. (2001). Genetic dissection of phenotypic diversity in farm animals. Nat. Rev. Genet. 2:130. doi: 10.1038/35052563
Andersson, L. (2003). Melanocortin receptor variants with phenotypic effects in horse, pig, and chicken. Ann. N. Y. Acad. Sci. 994, 313–318. doi: 10.1111/j.1749-6632.2003.tb03195.x
Andersson, L. (2016). Domestic animals as models for biomedical research. UPSALA J. Med. Sci. 121, 1–11. doi: 10.3109/03009734.2015.1091522
Anistoroaei, R., Krogh, A., and Christensen, K. (2013). A frameshift mutation in the LYST gene is responsible for the Aleutian color and the associated Chédiak–Higashi syndrome in American mink. Anim. Genet. 44, 178–183. doi: 10.1111/j.1365-2052.2012.02391.x
Anthony, D. W., Bogucki, P., Comşa, E., Gimbutas, M., Jovanović, B., Mallory, J., et al. (1986). The Kurgan culture, indo-european origins, and the domestication of the horse: a reconsideration and comments and replies. Curr. Anthropol. 27, 291–313.
Barker, J. T., Tan, S. G., and Mukherjee, T. (1991). Future studies of genetic differentiation among swamp buffalo and native goat populations. Buffalo and goats in Asia: genetic diversity and its application. 34:144.
Barlow, A., Cahill, J. A., Hartmann, S., Theunert, C., Xenikoudakis, G., Fortes, G. G., et al. (2018). Partial genomic survival of cave bears in living brown bears. Nat. Ecol. Evol. 2:1563. doi: 10.1038/s41559-018-0654-8
Beja-Pereira, A., Caramelli, D., Lalueza-Fox, C., Vernesi, C., Ferrand, N., Casoli, A., et al. (2006). The origin of European cattle: evidence from modern and ancient DNA. Proc. Natl. Acad. Sci. U.S.A. 103, 8113–8118. doi: 10.1073/pnas.0509210103
Beja-Pereira, A., England, P. R., Ferrand, N., Jordan, S., Bakhiet, A. O., Abdalla, M. A., et al. (2004). African origins of the domestic donkey. Science 304, 1781–1781.
Bellone, R. R., Forsyth, G., Leeb, T., Archer, S., Sigurdsson, S., Imsland, F., et al. (2010). Fine-mapping and mutation analysis of TRPM1: a candidate gene for leopard complex (LP) spotting and congenital stationary night blindness in horses. Briefings Funct. Genom. 9, 193–207. doi: 10.1093/bfgp/elq002
Bélteky, J., Agnvall, B., Bektic, L., Höglund, A., Jensen, P., and Guerrero-Bosagna, C. (2018). Epigenetics and early domestication: differences in hypothalamic DNA methylation between red junglefowl divergently selected for high or low fear of humans. Genet. Select. Evol. 50:13. doi: 10.1186/s12711-018-0384-z
Berghof, T., Parmentier, H., and Lammers, A. (2013). Transgenerational epigenetic effects on innate immunity in broilers: An underestimated field to be explored? Poul. Sci. 92, 2904–2913. doi: 10.3382/ps.2013-03177
Bocquet-Appel, J.-P. (2008). “Explaining the Neolithic demographic transition,” in The Neolithic Demographic Transition And Its Consequences, eds J. P. Bar-Yosef and O. Bocquet-Appel (Dordrecht: Springer), 35–55.
Bollongino, R., Burger, J., Powell, A., Mashkour, M., Vigne, J.-D., and Thomas, M. G. (2012). Modern taurine cattle descended from small number of Near-Eastern founders. Mol. Biol. Evol. 29, 2101–2104. doi: 10.1093/molbev/mss092
Bolstad, G. H., Hindar, K., Robertsen, G., Jonsson, B., Saegrov, H., Diserud, O. H., et al. (2017). Gene flow from domesticated escapes alters the life history of wild Atlantic salmon. Nat. Ecol. Evol. 1:0124. doi: 10.1038/s41559-017-0124
Bottomly, D., Walter, N. A., Hunter, J. E., Darakjian, P., Kawane, S., Buck, K. J., et al. (2011). Evaluating gene expression in C57BL/6J and DBA/2J mouse striatum using RNA-Seq and microarrays. PLoS One 6:e17820. doi: 10.1371/journal.pone.0017820
Boyko, A. R., Quignon, P., Li, L., Schoenebeck, J. J., Degenhardt, J. D., Lohmueller, K. E., et al. (2010). A simple genetic architecture underlies morphological variation in dogs. PLoS Biol. 8:e1000451. doi: 10.1371/journal.pbio.1000451
Bradley, D. G., and Magee, D. A. (2006). Genetics and the origins of domestic cattle. Document. Domest. 22, 317–328.
Bruford, M. W., Bradley, D. G., and Luikart, G. (2003). DNA markers reveal the complexity of livestock domestication. Nat. Rev. Genet. 4:900. doi: 10.1038/nrg1203
Brunberg, E., Andersson, L., Cothran, G., Sandberg, K., Mikko, S., and Lindgren, G. (2006). A missense mutation in PMEL17 is associated with the Silver coat color in the horse. BMC Genet. 7:46. doi: 10.1186/1471-2156-7-46
Brunson, K., and Reich, D. (2019). The promise of paleogenomics beyond our own species. Trends Genet. 35, 319–329. doi: 10.1016/j.tig.2019.02.006
Cadieu, E., Neff, M. W., Quignon, P., Walsh, K., Chase, K., Parker, H. G., et al. (2009). Coat variation in the domestic dog is governed by variants in three genes. Science 326, 150–153. doi: 10.1126/science.1177808
Caliebe, A., Nebel, A., Makarewicz, C., Krawczak, M., and Krause-Kyora, B. (2017). Insights into early pig domestication provided by ancient DNA analysis. Sci. Rep. 7:44550. doi: 10.1038/srep44550
Carneiro, M., Hu, D., Archer, J., Feng, C., Afonso, S., Chen, C., et al. (2017). Dwarfism and altered craniofacial development in rabbits is caused by a 12.1 kb deletion at the HMGA2 locus. Genetics 205, 955–965. doi: 10.1534/genetics.116.196667
Carneiro, M., Rubin, C.-J., Di Palma, F., Albert, F. W., Alföldi, J., Barrio, A. M., et al. (2014). Rabbit genome analysis reveals a polygenic basis for phenotypic change during domestication. Science 345, 1074–1079. doi: 10.1126/science.1253714
Chen, K., Baxter, T., Muir, W. M., Groenen, M. A., and Schook, L. B. (2007). Genetic resources, genome mapping and evolutionary genomics of the pig (Sus scrofa). Intern. J. Biol. Sci. 3:153. doi: 10.7150/ijbs.3.153
Chen, S., Lin, B.-Z., Baig, M., Mitra, B., Lopes, R. J., Santos, A. M., et al. (2010). Zebu cattle are an exclusive legacy of the South Asia Neolithic. Mol. Biol. Evol. 27, 1–6. doi: 10.1093/molbev/msp213
Cheng, Y., Prickett, M. D., Gutowska, W., Kuo, R., Belov, K., and Burt, D. W. (2015). Evolution of the avian β-defensin and cathelicidin genes. BMC Evol. Biol. 15:188. doi: 10.1186/s12862-015-0465-3
Chessa, B., Pereira, F., Arnaud, F., Amorim, A., Goyache, F., Mainland, I., et al. (2009). Revealing the history of sheep domestication using retrovirus integrations. Science 324, 532–536. doi: 10.1126/science.1170587
Choi, J.-W., Liao, X., Stothard, P., Chung, W.-H., Jeon, H.-J., Miller, S. P., et al. (2014). Whole-genome analyses of Korean native and Holstein cattle breeds by massively parallel sequencing. PLoS One 9:e101127. doi: 10.1371/journal.pone.0101127
Choudhury, A., and Barker, J. (2014). Wild water buffalo Bubalus arnee (Kerr, 1792). Ecol. Evol. Behav. Wild Cattle 255–301.
Cieslak, M., Pruvost, M., Benecke, N., Hofreiter, M., Morales, A., Reissmann, M., et al. (2010). Origin and history of mitochondrial DNA lineages in domestic horses. PLoS One 5:e15311. doi: 10.1371/journal.pone.0015311
Cieslak, M., Reissmann, M., Hofreiter, M., and Ludwig, A. (2011). Colours of domestication. Biol. Rev. 86, 885–899.
Clop, A., Marcq, F., Takeda, H., Pirottin, D., Tordoir, X., Bibé, B., et al. (2006). A mutation creating a potential illegitimate microRNA target site in the myostatin gene affects muscularity in sheep. Nat. Genet. 38:813. doi: 10.1038/ng1810
Consortium, B. H. (2009). Genome-wide survey of SNP variation uncovers the genetic structure of cattle breeds. Science 324, 528–532. doi: 10.1126/science.1167936
Cooper, A., and Poinar, H. N. (2000). Ancient DNA: do it right or not at all. Science 289, 1139–1139. doi: 10.1126/science.289.5482.1139b
Corbett-Detig, R. B., Hartl, D. L., and Sackton, T. B. (2015). Natural selection constrains neutral diversity across a wide range of species. PLoS Biol. 13:e1002112. doi: 10.1371/journal.pbio.1002112
Crowley, J., and Adelman, B. (1998). The Complete Dog Book: Official Publication Of The American Kennel Club. New York, NY: Howell House.
Cui, Y., Yan, H., Wang, K., Xu, H., Zhang, X., Zhu, H., et al. (2018). Insertion/deletion within the KDM6A gene is significantly associated with litter size in goat. Front. Genet. 9:91. doi: 10.3389/fgene.2018.00091
Dall’olio, S., Fontanesi, L., Nanni Costa, L., Tassinari, M., Minieri, L., and Falaschini, A. (2010). Analysis of horse myostatin gene and identification of single nucleotide polymorphisms in breeds of different morphological types. Biomed. Res. Intern. 2010:542945. doi: 10.1155/2010/542945
Darwin, C. (2010). The Variation Of Animals And Plants Under Domestication. Cambridge: Cambridge University Press.
Demars, J., Iannuccelli, N., Utzeri, V., Auvinet, G., Riquet, J., Fontanesi, L., et al. (2018). New insights into the melanophilin (MLPH) gene affecting coat color dilution in rabbits. Genes 9:430. doi: 10.3390/genes9090430
Di, R., Farhad Vahidi, S., Ma, Y., He, X., Zhao, Q., Han, J., et al. (2011). Microsatellite analysis revealed genetic diversity and population structure among Chinese cashmere goats. Anim. Genet. 42, 428–431. doi: 10.1111/j.1365-2052.2010.02072.x
Dobbie, W. R., and Braysher, M. (1993). Managing Vertebrate Pests: Feral Horses. Canberra: Australian Government Publishing Service.
Dong, Y., Xie, M., Jiang, Y., Xiao, N., Du, X., Zhang, W., et al. (2013). Sequencing and automated whole-genome optical mapping of the genome of a domestic goat (Capra hircus). Nat. Biotechnol. 31, 135–141. doi: 10.1038/nbt.2478
Driscoll, C. A., Macdonald, D. W., and O’brien, S. J. (2009). From wild animals to domestic pets, an evolutionary view of domestication. Proc. Natl. Acad. Sci. U.S.A. 106, 9971–9978. doi: 10.1073/pnas.0901586106
Driscoll, C. A., Menotti-Raymond, M., Roca, A. L., Hupe, K., Johnson, W. E., Geffen, E., et al. (2007). The Near Eastern origin of cat domestication. Science 317, 519–523. doi: 10.1126/science.1139518
Epstein, H. (1984). “Cattle,” in Evolution of Domesticated Animals, ed. I. L. Mason (New York, NY: Longman).
Everts, R. E., Band, M. R., Liu, Z. L., Kumar, C. G., Liu, L., Loor, J. J., et al. (2005). A 7872 cDNA microarray and its use in bovine functional genomics. Vet. Immunol. Immunopathol. 105, 235–245. doi: 10.1016/j.vetimm.2005.02.003
Fallahsharoudi, A., De Kock, N., Johnsson, M., Bektic, L., Ubhayasekera, S. K. A., Bergquist, J., et al. (2017). Genetic and targeted eQTL mapping reveals strong candidate genes modulating the stress response during chicken domestication. G3 7, 497–504. doi: 10.1534/g3.116.037721
Fan, R., Xie, J., Bai, J., Wang, H., Tian, X., Bai, R., et al. (2013). Skin transcriptome profiles associated with coat color in sheep. BMC Genomics 14:389. doi: 10.1186/1471-2164-14-389
Fan, W.-L., Ng, C. S., Chen, C.-F., Lu, M.-Y. J., Chen, Y.-H., Liu, C.-J., et al. (2013). Genome-wide patterns of genetic variation in two domestic chickens. Genome Biol. Evol. 5, 1376–1392. doi: 10.1093/gbe/evt097
Flamand, J., Vankan, D., Gairhe, K., Duong, H., and Barker, J. (2003). Genetic identification of wild Asian water buffalo in Nepal. Anim. Conserv. Forum 6, 265–270.
Fontanesi, L., Scotti, E., Allain, D., and Dall’olio, S. (2014a). A frameshift mutation in the melanophilin gene causes the dilute coat colour in rabbit (Oryctolagus cuniculus) breeds. Anim. Genet. 45, 248–255. doi: 10.1111/age.12104
Fontanesi, L., Vargiolu, M., Scotti, E., Latorre, R., Pellegrini, M. S. F., Mazzoni, M., et al. (2014b). The KIT gene is associated with the English spotting coat color locus and congenital megacolon in Checkered Giant rabbits (Oryctolagus cuniculus). PLoS One 9:e93750. doi: 10.1371/journal.pone.0093750
Fontanesi, L., Tazzoli, M., Beretti, F., and Russo, V. (2006). Mutations in the melanocortin 1 receptor (MC1R) gene are associated with coat colours in the domestic rabbit (Oryctolagus cuniculus). Anim. Genet. 37, 489–493. doi: 10.1111/j.1365-2052.2006.01494.x
Franklin, T. B., Russig, H., Weiss, I. C., Gräff, J., Linder, N., Michalon, A., et al. (2010). Epigenetic transmission of the impact of early stress across generations. Biol. Psychiatry 68, 408–415. doi: 10.1016/j.biopsych.2010.05.036
Frantz, L. A., Mullin, V. E., Pionnier-Capitan, M., Lebrasseur, O., Ollivier, M., Perri, A., et al. (2016). Genomic and archaeological evidence suggest a dual origin of domestic dogs. Science 352, 1228–1231. doi: 10.1126/science.aaf3161
Frantz, L. A., Schraiber, J. G., Madsen, O., Megens, H.-J., Cagan, A., Bosse, M., et al. (2015). Evidence of long-term gene flow and selection during domestication from analyses of Eurasian wild and domestic pig genomes. Nat. Genet. 47:1141. doi: 10.1038/ng.3394
Frischknecht, M., Jagannathan, V., Plattet, P., Neuditschko, M., Signer-Hasler, H., Bachmann, I., et al. (2015). A non-synonymous HMGA2 variant decreases height in Shetland ponies and other small horses. PLoS One 10:e0140749. doi: 10.1371/journal.pone.0140749
Galov, A., Fabbri, E., Caniglia, R., Arbanasiæ, H., Lapalombella, S., Florijanèiæ, T., et al. (2015). First evidence of hybridization between golden jackal (Canis aureus) and domestic dog (Canis familiaris) as revealed by genetic markers. R. Soc. Open Sci. 2:150450. doi: 10.1098/rsos.150450
Gaunitz, C., Fages, A., Hanghøj, K., Albrechtsen, A., Khan, N., Schubert, M., et al. (2018). Ancient genomes revisit the ancestry of domestic and Przewalski’s horses. Science 360, 111–114. doi: 10.1126/science.aao3297
Gautier, E. L., Shay, T., Miller, J., Greter, M., Jakubzick, C., Ivanov, S., et al. (2012). Gene-expression profiles and transcriptional regulatory pathways that underlie the identity and diversity of mouse tissue macrophages. Nat. Immunol. 13:1118. doi: 10.1038/ni.2419
Gentry, A., Clutton-Brock, J., and Groves, C. P. (2004). The naming of wild animal species and their domestic derivatives. J. Archaeol. Sci. 31, 645–651.
Giuffra, E., Kijas, J., Amarger, V., Carlborg, O., Jeon, J.-T., and Andersson, L. (2000). The origin of the domestic pig: independent domestication and subsequent introgression. Genetics 154, 1785–1791.
Goerlich, V. C., Nätt, D., Elfwing, M., Macdonald, B., and Jensen, P. (2012). Transgenerational effects of early experience on behavioral, hormonal and gene expression responses to acute stress in the precocial chicken. Horm. Behav. 61, 711–718. doi: 10.1016/j.yhbeh.2012.03.006
Gou, X., Wang, Z., Li, N., Qiu, F., Xu, Z., Yan, D., et al. (2014). Whole-genome sequencing of six dog breeds from continuous altitudes reveals adaptation to high-altitude hypoxia. Genome Res. 24, 1308–1315. doi: 10.1101/gr.171876.113
Grobet, L., Martin, L. J. R., Poncelet, D., Pirottin, D., Brouwers, B., Riquet, J., et al. (1997). A deletion in the bovine myostatin gene causes the double–muscled phenotype in cattle. Nat. Genet. 17:71. doi: 10.1038/ng0997-71
Groenen, M. A., Archibald, A. L., Uenishi, H., Tuggle, C. K., Takeuchi, Y., Rothschild, M. F., et al. (2012). Analyses of pig genomes provide insight into porcine demography and evolution. Nature 491, 393–398. doi: 10.1038/nature11622
Groeneveld, L., Lenstra, J., Eding, H., Toro, M., Scherf, B., Pilling, D., et al. (2010). Genetic diversity in farm animals–a review. Anim. Genet. 41, 6–31. doi: 10.1111/j.1365-2052.2010.02038.x
Groves, C. (1981). Systematic relationships in the bovini (Artiodactyla, Bovidae). J. Zool. Syst. Evol. Res. 19, 264–278.
Guerrero-Bosagna, C., Settles, M., Lucker, B., and Skinner, M. K. (2010). Epigenetic transgenerational actions of vinclozolin on promoter regions of the sperm epigenome. PLoS One 5:e13100. doi: 10.1371/journal.pone.0013100
Guibert, S., Girardot, M., Leveziel, H., Julien, R., and Oulmouden, A. (2004). Pheomelanin coat colour dilution in French cattle breeds is not correlated with the TYR, TYRP1 and DCT transcription levels. Pigment. Cell Res. 17, 337–345. doi: 10.1111/j.1600-0749.2004.00152.x
Gunnarsson, U., Hellström, A. R., Tixier-Boichard, M., Minvielle, F., Bed’hom, B., Ito, S. I., et al. (2007). Mutations in SLC45A2 cause plumage color variation in chicken and Japanese quail. Genetics 175, 867–877. doi: 10.1534/genetics.106.063107
Haase, B., Brooks, S., Tozaki, T., Burger, D., Poncet, P. A., Rieder, S., et al. (2009). Seven novel KIT mutations in horses with white coat colour phenotypes. Anim. Genet. 40, 623–629. doi: 10.1111/j.1365-2052.2009.01893.x
Haase, B., Brooks, S. A., Schlumbaum, A., Azor, P. J., Bailey, E., Alaeddine, F., et al. (2007). Allelic heterogeneity at the equine KIT locus in dominant white (W) horses. PLoS Genet. 3:e195. doi: 10.1371/journal.pgen.0030195
Haase, B., Signer-Hasler, H., Binns, M. M., Obexer-Ruff, G., Hauswirth, R., Bellone, R. R., et al. (2013). Accumulating mutations in series of haplotypes at the KIT and MITF loci are major determinants of white markings in Franches-Montagnes horses. PLoS One 8:e75071. doi: 10.1371/journal.pone.0075071
Han, J.-L., Min, Y., Guo, T.-T., Yue, Y.-J., Liu, J.-B., Niu, C.-E., et al. (2015). Molecular characterization of two candidate genes associated with coat color in Tibetan sheep (Ovis arise). J. Integr. Agric. 14, 1390–1397. doi: 10.4238/2015.February.6.22
Hartl, D. L. (2009). Essential Genetics: A Genomics Perspective: A Genomics Perspective. Burlington, MA: Jones & Bartlett Publishers.
Hausberger, M., Roche, H., Henry, S., and Visser, E. K. (2008). A review of the human–horse relationship. Appl. Anim. Behav. Sci. 109, 1–24.
Hauswirth, R., Haase, B., Blatter, M., Brooks, S. A., Burger, D., Drögemüller, C., et al. (2012). Mutations in MITF and PAX3 cause “splashed white” and other white spotting phenotypes in horses. PLoS Genet. 8:e1002653. doi: 10.1371/journal.pgen.1008321
Hekman, J. P., Johnson, J. L., and Kukekova, A. V. (2015). Transcriptome analysis in domesticated species: challenges and strategies. Bioinform. Biol. Insights 9:S29334. doi: 10.4137/BBI.S29334
Hellström, A. R., Sundstrom, E., Gunnarsson, U., Bed’hom, B., Tixier-Boichard, M., Honaker, C. F., et al. (2010). Sex-linked barring in chickens is controlled by the CDKN2A/B tumour suppressor locus. Pigment Cell Melanom. Res. 23, 521–530. doi: 10.1111/j.1755-148X.2010.00700.x
Helmer, D., Gourichon, L., Monchot, H., Peters, J., and Segui, M. S. (2005). Identifying Early Domestic Cattle From Pre-Pottery Neolithic Sites On The Middle Euphrates Using Sexual Dimorphism. Oxford: Oxbow Books.
Heyne, H. O., Lautenschläger, S., Nelson, R., Besnier, F., Rotival, M., Cagan, A., et al. (2014). Genetic influences on brain gene expression in rats selected for tameness and aggression. Genetics 198, 1277–1290. doi: 10.1534/genetics.114.168948
Hiendleder, S., Kaupe, B., Wassmuth, R., and Janke, A. (2002). Molecular analysis of wild and domestic sheep questions current nomenclature and provides evidence for domestication from two different subspecies. Proc. R. Soc. Lond. Ser. B Biol. Sci. 269, 893–904. doi: 10.1098/rspb.2002.1975
Higuchi, R., Bowman, B., Freiberger, M., Ryder, O. A., and Wilson, A. C. (1984). DNA sequences from the quagga, an extinct member of the horse family. Nature 312, 282–284. doi: 10.1038/312282a0
Hillier, L. W., Miller, W., Birney, E., Warren, W., Hardison, R. C., Ponting, C. P., et al. (2014). Sequence and comparative analysis of the chicken genome provide unique perspectives on vertebrate evolution. Nature 423, 695–777. doi: 10.1038/nature03154
Hu, Y., Xu, H., Li, Z., Zheng, X., Jia, X., Nie, Q., et al. (2013). Comparison of the genome-wide DNA methylation profiles between fast-growing and slow-growing broilers. PLoS One 8:e56411. doi: 10.1371/journal.pone.0056411
Huang, Y.-F., Zhao, Y.-J., and He, J.-N. (2017). Genetic diversity of three Chinese native sheep breeds. Russia. J. Genet. 53, 118–127.
Irving-Pease, E. K., Ryan, H., Jamieson, A., Dimopoulos, E. A., Larson, G., and Frantz, L. A. (2018). “Paleogenomics of animal domestication,” in Paleogenomics Aleogenomics Population Genomics, eds C. Rajora and O. Lindqvist (Cham: Springer), 225–272.
Janowitz Koch, I., Clark, M. M., Thompson, M. J., Deere-Machemer, K. A., Wang, J., Duarte, L., et al. (2016). The concerted impact of domestication and transposon insertions on methylation patterns between dogs and grey wolves. Mol. Ecol. 25, 1838–1855. doi: 10.1111/mec.13480
Jansen, T., Forster, P., Levine, M. A., Oelke, H., Hurles, M., Renfrew, C., et al. (2002). Mitochondrial DNA and the origins of the domestic horse. Proc. Natl. Acad. Sci. U.S.A. 99, 10905–10910. doi: 10.1073/pnas.152330099
Jensen, P. (2015). Adding ‘epi-’to behaviour genetics: implications for animal domestication. J. Exp. Biol. 218, 32–40. doi: 10.1242/jeb.106799
Johnson, J., Kozysa, A., Kharlamova, A., Gulevich, R., Perelman, P., Fong, H., et al. (2015). Platinum coat color in red fox (V ulpes vulpes) is caused by a mutation in an autosomal copy of KIT. Anim. Genet. 46, 190–199. doi: 10.1111/age.12270
Jones, P., Chase, K., Martin, A., Davern, P., Ostrander, E. A., and Lark, K. G. (2008). Single-nucleotide-polymorphism-based association mapping of dog stereotypes. Genetics 179, 1033–1044. doi: 10.1534/genetics.108.087866
Joshi, M. B., Rout, P. K., Mandal, A. K., Tyler-Smith, C., Singh, L., and Thangaraj, K. (2004). Phylogeography and origin of Indian domestic goats. Mol. Biol. Evol. 21, 454–462. doi: 10.1093/molbev/msh038
Kanginakudru, S., Metta, M., Jakati, R., and Nagaraju, J. (2008). Genetic evidence from Indian red jungle fowl corroborates multiple domestication of modern day chicken. BMC Evol. Biol. 8:174. doi: 10.1186/1471-2148-8-174
Karlsson, A. C., Kerje, S., Hallböök, F., and Jensen, P. (2009). The Dominant white mutation in the PMEL17 gene does not cause visual impairment in chickens. Vet. Ophthalmol. 12, 292–298. doi: 10.1111/j.1463-5224.2009.00714.x
Kemp, B. M., Judd, K., Monroe, C., Eerkens, J. W., Hilldorfer, L., Cordray, C., et al. (2017). Prehistoric mitochondrial DNA of domesticate animals supports a 13th century exodus from the northern US southwest. PLoS One 12:e0178882. doi: 10.1371/journal.pone.0178882
Kerje, S., Sharma, P., Gunnarsson, U., Kim, H., Bagchi, S., Fredriksson, R., et al. (2004). The dominant white, dun and Smoky color variants in chicken are associated with insertion/deletion polymorphisms in the PMEL17 gene. Genetics 168, 1507–1518. doi: 10.1534/genetics.104.027995
Khatkar, M. S., Thomson, P. C., Tammen, I., and Raadsma, H. W. (2004). Quantitative trait loci mapping in dairy cattle: review and meta-analysis. Genet. Select. Evol. 36:163. doi: 10.1186/1297-9686-36-2-163
Kierstein, G., Vallinoto, M., Silva, A., Schneider, M. P., Iannuzzi, L., and Brenig, B. (2004). Analysis of mitochondrial D-loop region casts new light on domestic water buffalo (Bubalus bubalis) phylogeny. Mol. Phylogenet. Evol. 30, 308–324. doi: 10.1016/s1055-7903(03)00221-5
Kijas, J. W., Lenstra, J. A., Hayes, B., Boitard, S., Neto, L. R. P., San Cristobal, M., et al. (2012). Genome-wide analysis of the world’s sheep breeds reveals high levels of historic mixture and strong recent selection. PLoS Biol. 10:e1001258. doi: 10.1371/journal.pbio.1001258
Ko, C. H., and Takahashi, J. S. (2006). Molecular components of the mammalian circadian clock. Hum. Mol. Genet. 15, R271–R277. doi: 10.1007/978-3-642-25950-0_1
Komáromy, A. M., Rowlan, J. S., La Croix, N. C., and Mangan, B. G. (2011). Equine Multiple Congenital Ocular Anomalies (MCOA) syndrome in PMEL17 (Silver) mutant ponies: five cases. Vet. Ophthalmol. 14, 313–320. doi: 10.1111/j.1463-5224.2011.00878.x
Körberg, I. B., Sundström, E., Meadows, J., Pielberg, G. R., Gustafson, U., Hedhammar, A., et al. (2014). A simple repeat polymorphism in the MITF-M promoter is a key regulator of white spotting in dogs. PLoS One 9:e104363. doi: 10.1371/journal.pone.0104363
Koren, S., Schatz, M. C., Walenz, B. P., Martin, J., Howard, J. T., Ganapathy, G., et al. (2012). Hybrid error correction and de novo assembly of single-molecule sequencing reads. Nat. Biotechnol. 30:693. doi: 10.1038/nbt.2280
Koseniuk, A., Ropka-Molik, K., Rubiś, D., and Smołucha, G. (2018). Genetic background of coat colour in sheep. Archiv. Anim. Breed. 61, 173–178.
Kristjansson, T., Bjornsdottir, S., Sigurdsson, A., Andersson, L., Lindgren, G., Helyar, S., et al. (2014). The effect of the ‘Gait keeper’mutation in the DMRT3 gene on gaiting ability in Icelandic horses. J. Anim. Breed. Genet. 131, 415–425. doi: 10.1111/jbg.12112
Kukekova, A. V., Trut, L. N., Chase, K., Kharlamova, A. V., Johnson, J. L., Temnykh, S. V., et al. (2011). Mapping loci for fox domestication: deconstruction/reconstruction of a behavioral phenotype. Behav. Genet. 41, 593–606. doi: 10.1007/s10519-010-9418-1
Kunieda, T., Nakagiri, M., Takami, M., Ide, H., and Ogawa, H. (1999). Cloning of bovine LYST gene and identification of a missense mutation associated with Chediak-higashi syndrome of cattle. Mamm. Genome 10, 1146–1149. doi: 10.1007/s003359901181
Larson, G. (2011). Genetics and domestication: important questions for new answers. Curr. Anthropol. 52, S485–S495.
Larson, G. (2017). Reconsidering the distribution of gray wolves. Zool. Res. 38:115. doi: 10.24272/j.issn.2095-8137.2017.021
Larson, G., and Burger, J. (2013). A population genetics view of animal domestication. Trends Genet. 29, 197–205. doi: 10.1016/j.tig.2013.01.003
Larson, G., and Fuller, D. Q. (2014). The evolution of animal domestication. Ann. Rev. Ecol. Evol. Syst. 45, 115–136.
Larson, G., Piperno, D. R., Allaby, R. G., Purugganan, M. D., Andersson, L., Arroyo-Kalin, M., et al. (2014). Current perspectives and the future of domestication studies. Proc. Natl. Acad. Sci. U.S.A. 111, 6139–6146. doi: 10.1073/pnas.1323964111
Leathlobhair, M. N., Perri, A. R., Irving-Pease, E. K., Witt, K. E., Linderholm, A., Haile, J., et al. (2018). The evolutionary history of dogs in the Americas. Science 361, 81–85. doi: 10.1126/science.aao4776
Lenstra, J. A., Ajmone-Marsan, P., Beja-Pereira, A., Bollongino, R., Bradley, D. G., Colli, L., et al. (2014). Meta-analysis of mitochondrial DNA reveals several population bottlenecks during worldwide migrations of cattle. Diversity 6, 178–187.
Leonard, J. A., Wayne, R. K., Wheeler, J., Valadez, R., Guillén, S., and Vila, C. (2002). Ancient DNA evidence for old world origin of new world dogs. Science 298, 1613–1616. doi: 10.1126/science.1076980
Leroy, G., Baumung, R., Boettcher, P., Besbes, B., From, T., and Hoffmann, I. (2018). Animal genetic resources diversity and ecosystem services. Glob. Food Securi. 17, 84–91.
Li, M., Tian, S., Jin, L., Zhou, G., Li, Y., Zhang, Y., et al. (2013). Genomic analyses identify distinct patterns of selection in domesticated pigs and Tibetan wild boars. Nat. Genet. 45, 1431–1438. doi: 10.1038/ng.2811
Librado, P., Fages, A., Gaunitz, C., Leonardi, M., Wagner, S., Khan, N., et al. (2016). The evolutionary origin and genetic makeup of domestic horses. Genetics 204, 423–434. doi: 10.1534/genetics.116.194860
Lindahl, T. (1997). Facts and artifacts of ancient DNA. Cell 90, 1–3. doi: 10.1016/s0092-8674(00)80306-2
Lindblad-Toh, K., Wade, C. M., Mikkelsen, T. S., Karlsson, E. K., Jaffe, D. B., Kamal, M., et al. (2005). Genome sequence, comparative analysis and haplotype structure of the domestic dog. Nature 438, 803–819. doi: 10.1038/nature04338
Liu, Y.-H., Wang, L., Xu, T., Guo, X., Li, Y., Yin, T.-T., et al. (2018). Whole-genome sequencing of African dogs provides insights into adaptations against tropical parasites. Mol. Biol. Evol. 35, 287–298. doi: 10.1093/molbev/msx258
Liu, Y.-P., Wu, G.-S., Yao, Y.-G., Miao, Y.-W., Luikart, G., Baig, M., et al. (2006). Multiple maternal origins of chickens: out of the Asian jungles. Mol. Phylogenet. Evol. 38, 12–19. doi: 10.1016/j.ympev.2005.09.014
Liu, Z., Ji, Z., Wang, G., Chao, T., Hou, L., and Wang, J. (2016). Genome-wide analysis reveals signatures of selection for important traits in domestic sheep from different ecoregions. BMC Genomics 17:863. doi: 10.1186/s12864-016-3212-2
Loftus, R. T., Machugh, D. E., Bradley, D. G., Sharp, P. M., and Cunningham, P. (1994). Evidence for two independent domestications of cattle. Proc. Natl. Acad. Sci. U.S.A. 91, 2757–2761. doi: 10.1073/pnas.91.7.2757
Ludwig, A., Reissmann, M., Benecke, N., Bellone, R., Sandoval-Castellanos, E., Cieslak, M., et al. (2015). Twenty-five thousand years of fluctuating selection on leopard complex spotting and congenital night blindness in horses. Philos. Trans. R. Soc. B 370:20130386. doi: 10.1098/rstb.2013.0386
Lühken, G. (2012). Genetic testing for phenotype-causing variants in sheep and goats. Mol. Cell. Probes 26, 231–237. doi: 10.1016/j.mcp.2012.04.005
Luikart, G., Gielly, L., Excoffier, L., Vigne, J.-D., Bouvet, J., and Taberlet, P. (2001). Multiple maternal origins and weak phylogeographic structure in domestic goats. Proc. Natl. Acad. Sci. U.S.A. 98, 5927–5932. doi: 10.1073/pnas.091591198
Lyons, S. K., Amatangelo, K. L., Behrensmeyer, A. K., Bercovici, A., Blois, J. L., Davis, M., et al. (2016). Holocene shifts in the assembly of plant and animal communities implicate human impacts. Nature 529:80. doi: 10.1038/nature19104
MacDonald, K. C. (1992). The domestic chicken (Gallus gallus) in sub-Saharan Africa: a background to its introduction and its osteological differentiation from indigenous fowls (Numidinae and Francolinus sp.). J. Archaeol. Sci. 19, 303–318.
MacHugh, D. E., and Bradley, D. G. (2001). Livestock genetic origins: goats buck the trend. Proc. Natl. Acad. Sci. U.S.A. 98, 5382–5384. doi: 10.1073/pnas.111163198
MacHugh, D. E., Larson, G., and Orlando, L. (2017). Taming the past: ancient DNA and the study of animal domestication. Ann. Rev. Anim. Biosci. 5, 329–351. doi: 10.1146/annurev-animal-022516-022747
Malheiros, J. M., Enríquez-Valencia, C. E., Da Silva Duran, B. O., De Paula, T. G., Curi, R. A., De Vasconcelos Silva, J., et al. (2018). Association of CAST2, HSP90AA1, DNAJA1 and HSPB1 genes with meat tenderness in Nellore cattle. Meat Sci. 138, 49–52. doi: 10.1016/j.meatsci.2018.01.003
Manceau, M., Domingues, V. S., Linnen, C. R., Rosenblum, E. B., and Hoekstra, H. E. (2010). Convergence in pigmentation at multiple levels: mutations, genes and function. Philos. Trans. R. Soc. B Biol. Sci. 365, 2439–2450. doi: 10.1098/rstb.2010.0104
Mao, H., Ren, J., Ding, N., Xiao, S., and Huang, L. (2010). Genetic variation within coat color genes of MC1R and ASIP in Chinese brownish red Tibetan pigs. Anim. Sci. J. 81, 630–634. doi: 10.1111/j.1740-0929.2010.00789.x
Marshall, F. B., Dobney, K., Denham, T., and Capriles, J. M. (2014). Evaluating the roles of directed breeding and gene flow in animal domestication. Proc. Natl. Acad. Sci. U.S.A. 111, 6153–6158. doi: 10.1073/pnas.1312984110
Melletti, M., and Burton, J. (2014). Ecology, Evolution And Behaviour Of Wild Cattle: Implications For Conservation. Cambridge: Cambridge University Press.
Messer, P. W., Ellner, S. P., and Hairston, N. G. Jr. (2016). Can population genetics adapt to rapid evolution? Trends Genet. 32, 408–418. doi: 10.1016/j.tig.2016.04.005
Mignon-Grasteau, S., Boissy, A., Bouix, J., Faure, J.-M., Fisher, A. D., Hinch, G. N., et al. (2005). Genetics of adaptation and domestication in livestock. Livestock Product. Sci. 93, 3–14.
Montague, M. J., Li, G., Gandolfi, B., Khan, R., Aken, B. L., Searle, S. M., et al. (2014). Comparative analysis of the domestic cat genome reveals genetic signatures underlying feline biology and domestication. Proc. Natl. Acad. Sci. U.S.A. 111, 17230–17235. doi: 10.1073/pnas.1410083111
Mosher, D. S., Quignon, P., Bustamante, C. D., Sutter, N. B., Mellersh, C. S., Parker, H. G., et al. (2007). A mutation in the myostatin gene increases muscle mass and enhances racing performance in heterozygote dogs. PLoS Genet. 3:e79. doi: 10.1371/journal.pgen.0030079
Mwacharo, J. M., Bjørnstad, G., Han, J., and Hanotte, O. (2013). The history of African village chickens: an archaeological and molecular perspective. Afr. Archaeol. Rev. 30, 97–114. doi: 10.1007/s10437-013-9128-1
Nätt, D., Rubin, C.-J., Wright, D., Johnsson, M., Beltéky, J., Andersson, L., et al. (2012). Heritable genome-wide variation of gene expression and promoter methylation between wild and domesticated chickens. BMC Genomics 13:59. doi: 10.1186/1471-2164-13-59
Neves, F., Abrantes, J., Steinke, J. W., and Esteves, P. J. (2014). Maximum-likelihood approaches reveal signatures of positive selection in IL genes in mammals. Innate Immun. 20, 184–191. doi: 10.1177/1753425913486687
Nishibori, M., Shimogiri, T., Hayashi, T., and Yasue, H. (2005). Molecular evidence for hybridization of species in the genus Gallus except for Gallus varius. Anim. Genet. 36, 367–375. doi: 10.1111/j.1365-2052.2005.01318.x
Norris, B. J., and Whan, V. A. (2008). A gene duplication affecting expression of the ovine ASIP gene is responsible for white and black sheep. Genome Res. 18, 1282–1293. doi: 10.1101/gr.072090.107
Ogorevc, J., Kunej, T., Razpet, A., and Dovc, P. (2009). Database of cattle candidate genes and genetic markers for milk production and mastitis. Anim. Genet. 40, 832–851. doi: 10.1111/j.1365-2052.2009.01921.x
Ollivier, M., Tresset, A., Hitte, C., Petit, C., Hughes, S., Gillet, B., et al. (2013). Evidence of coat color variation sheds new light on ancient canids. PLoS One 8:e75110. doi: 10.1371/journal.pone.0075110
Ostrander, E. A., Galibert, F., and Patterson, D. F. (2000). Canine genetics comes of age. Trends Genet. 16, 117–124. doi: 10.1016/s0168-9525(99)01958-7
Ottoni, C., Girdland Flink, L., Evin, A., Geörg, C., De Cupere, B., Van Neer, W., et al. (2012). Pig domestication and human-mediated dispersal in western Eurasia revealed through ancient DNA and geometric morphometrics. Mol. Biol. Evol. 30, 824–832. doi: 10.1093/molbev/mss261
Ottoni, C., Van Neer, W., De Cupere, B., Daligault, J., Guimaraes, S., Peters, J., et al. (2017). The palaeogenetics of cat dispersal in the ancient world. Nat. Ecol. Evol. 1, 1–7.
Outram, A. K., Stear, N. A., Bendrey, R., Olsen, S., Kasparov, A., Zaibert, V., et al. (2009). The earliest horse harnessing and milking. Science 323, 1332–1335. doi: 10.1126/science.1168594
Pääbo, S. (1985). Molecular cloning of ancient Egyptian mummy DNA. Nature 314, 644–645. doi: 10.1038/314644a0
Pääbo, S., Gifford, J. A., and Wilson, A. C. (1988). Mitochondrial DNA sequences from a 7000-year old brain. Nucleic Acids Res. 16, 9775–9787. doi: 10.1093/nar/16.20.9775
Pääbo, S., Higuchi, R. G., and Wilson, A. C. (1989). Ancient DNA and the polymerase chain reaction: the emerging field of molecular archaeology (Minireview). J. Biol. Chem. 264, 9709–9712.
Pan, Z., Li, S., Liu, Q., Wang, Z., Zhou, Z., Di, R., et al. (2018). Whole-genome sequences of 89 Chinese sheep suggest role of RXFP2 in the development of unique horn phenotype as response to semi-feralization. Gigascience 7:giy019. doi: 10.1093/gigascience/giy019
Park, S. D., Magee, D. A., Mcgettigan, P. A., Teasdale, M. D., Edwards, C. J., Lohan, A. J., et al. (2015). Genome sequencing of the extinct Eurasian wild aurochs, Bos primigenius, illuminates the phylogeography and evolution of cattle. Genome Biol. 16:234. doi: 10.1186/s13059-015-0790-2
Parker, H. G., Vonholdt, B. M., Quignon, P., Margulies, E. H., Shao, S., Mosher, D. S., et al. (2009). An expressed fgf4 retrogene is associated with breed-defining chondrodysplasia in domestic dogs. Science 325, 995–998. doi: 10.1126/science.1173275
Pendleton, A. L., Shen, F., Taravella, A. M., Emery, S., Veeramah, K. R., Boyko, A. R., et al. (2018). Comparison of village dog and wolf genomes highlights the role of the neural crest in dog domestication. BMC Biol. 16:64. doi: 10.1186/s12915-018-0535-2
Pereira, G. L., De Matteis, R., Regitano, L. C., Chardulo, L. A. L., and Curi, R. A. (2016). MSTN, CKM, and DMRT3 gene variants in different lines of quarter horses. J. Equine Vet. Sci. 39, 33–37.
Pértille, F., Brantsaeter, M., Nordgreen, J., Coutinho, L. L., Janczak, A. M., Jensen, P., et al. (2017). DNA methylation profiles in red blood cells of adult hens correlate with their rearing conditions. J. Exp. Biol. 220, 3579–3587. doi: 10.1242/jeb.157891
Petersen, J. L., Mickelson, J. R., Cothran, E. G., Andersson, L. S., Axelsson, J., Bailey, E., et al. (2013a). Genetic diversity in the modern horse illustrated from genome-wide SNP data. PLoS One 8:e54997. doi: 10.1371/journal.pone.0054997
Petersen, J. L., Mickelson, J. R., Rendahl, A. K., Valberg, S. J., Andersson, L. S., Axelsson, J., et al. (2013b). Genome-wide analysis reveals selection for important traits in domestic horse breeds. PLoS Genet. 9:e1003211. doi: 10.1371/journal.pgen.1003211
Philipp, U., Hamann, H., Mecklenburg, L., Nishino, S., Mignot, E., Günzel-Apel, A.-R., et al. (2005). Polymorphisms within the canine MLPH gene are associated with dilute coat color in dogs. BMC Genet. 6:34. doi: 10.1186/1471-2156-6-34
Pionnier-Capitan, M., Bemilli, C., Bodu, P., Célérier, G., Ferrié, J.-G., Fosse, P., et al. (2011). New evidence for upper palaeolithic small domestic dogs in south-western Europe. J. Archaeol. Sci. 38, 2123–2140.
Pitt, D., Sevane, N., Nicolazzi, E. L., Machugh, D. E., Park, S. D., Colli, L., et al. (2019). Domestication of cattle: Two or three events? Evol. Appl. 12, 123–136. doi: 10.1111/eva.12674
Pollinger, J. P., Lohmueller, K. E., Han, E., Parker, H. G., Quignon, P., Degenhardt, J. D., et al. (2010). Genome-wide SNP and haplotype analyses reveal a rich history underlying dog domestication. Nature 464, 898–902. doi: 10.1038/nature08837
Price, E. O. (1999). Behavioral development in animals undergoing domestication. Appl. Anim. Behav. Sci. 65, 245–271.
Promerová, M., Andersson, L., Juras, R., Penedo, M., Reissmann, M., Tozaki, T., et al. (2014). Worldwide frequency distribution of the ‘G ait keeper’mutation in the DMRT 3 gene. Anim. Genet. 45, 274–282. doi: 10.1111/age.12120
Pruvost, M., Bellone, R., Benecke, N., Sandoval-Castellanos, E., Cieslak, M., Kuznetsova, T., et al. (2011). Genotypes of predomestic horses match phenotypes painted in Paleolithic works of cave art. Proc. Natl. Acad. Sci. U.S.A. 108, 18626–18630. doi: 10.1073/pnas.1108982108
Pryce, J. E., Hayes, B. J., Bolormaa, S., and Goddard, M. E. (2011). Polymorphic regions affecting human height also control stature in cattle. Genetics 187, 981–984. doi: 10.1534/genetics.110.123943
Qanbari, S., and Simianer, H. (2014). Mapping signatures of positive selection in the genome of livestock. Livestock Sci. 166, 133–143.
Raynal, N. J.-M., Si, J., Taby, R. F., Gharibyan, V., Ahmed, S., Jelinek, J., et al. (2012). DNA methylation does not stably lock gene expression but instead serves as a molecular mark for gene silencing memory. Cancer Res. 72, 1170–1181. doi: 10.1158/0008-5472.CAN-11-3248
Reissmann, M., and Ludwig, A. (2013). Pleiotropic effects of coat colour-associated mutations in humans, mice and other mammals. Semin. Cell Dev. Biol. 24, 576–586. doi: 10.1016/j.semcdb.2013.03.014
Ren, J., Duan, Y., Qiao, R., Yao, F., Zhang, Z., Yang, B., et al. (2011). A missense mutation in PPARD causes a major QTL effect on ear size in pigs. PLoS Genet. 7:e1002043. doi: 10.1371/journal.pgen.1002043
Rieder, S., Taourit, S., Mariat, D., Langlois, B., and Guérin, G. (2001). Mutations in the agouti (ASIP), the extension (MC1R), and the brown (TYRP1) loci and their association to coat color phenotypes in horses (Equus caballus). Mamm. Genome 12, 450–455. doi: 10.1007/s003350020017
Rodriguez, C., Tomas, A., Alves, E., Ramirez, O., Arque, M., Munoz, G., et al. (2005). QTL mapping for teat number in an Iberian-by-Meishan pig intercross. Anim. Genet. 36, 490–496. doi: 10.1111/j.1365-2052.2005.01358.x
Rollin, B. (2011). Putting the Horse Before Descartes: My Life’s Work On Behalf Of Animals. Philadelphia: Temple University Press.
Ross-Ibarra, J. (2004). The evolution of recombination under domestication: a test of two hypotheses. Am. Nat. 163, 105–112. doi: 10.1086/380606
Roy, M., Kim, N., Kim, K., Chung, W.-H., Achawanantakun, R., Sun, Y., et al. (2013). Analysis of the canine brain transcriptome with an emphasis on the hypothalamus and cerebral cortex. Mamm. Genome 24, 484–499. doi: 10.1007/s00335-013-9480-0
Royo, L., Álvarez, I., Fernandez, I., Arranz, J., Gomez, E., and Goyache, F. (2005). The coding sequence of the ASIP gene is identical in nine wild-type coloured cattle breeds. J. Anim. Breed. Genet. 122, 357–360. doi: 10.1111/j.1439-0388.2005.00541.x
Rubin, C.-J., Megens, H.-J., Barrio, A. M., Maqbool, K., Sayyab, S., Schwochow, D., et al. (2012). Strong signatures of selection in the domestic pig genome. Proc. Natl. Acad. Sci. U.S.A. 109, 19529–19536. doi: 10.1073/pnas.1217149109
Runkel, F., Büssow, H., Seburn, K. L., Cox, G. A., Ward, D. M., Kaplan, J., et al. (2006). Grey, a novel mutation in the murine Lyst gene, causes the beige phenotype by skipping of exon 25. Mamm. Genome 17, 203–210. doi: 10.1007/s00335-005-0015-1
Savolainen, P., Zhang, Y.-P., Luo, J., Lundeberg, J., and Leitner, T. (2002). Genetic evidence for an East Asian origin of domestic dogs. Science 298, 1610–1613. doi: 10.1126/science.1073906
Schafberg, R., and Swalve, H. (2015). The history of breeding for polled cattle. Livestock Sci. 179, 54–70.
Scherf, B. D., and Pilling, D. (2015). The Second Report On The State Of The World’s Animal Genetic Resources For Food And Agriculture. Rome: FAO.
Schmutz, S. M., and Berryere, T. G. (2007a). Genes affecting coat colour and pattern in domestic dogs: a review. Anim. Genet. 38, 539–549. doi: 10.1111/j.1365-2052.2007.01664.x
Schmutz, S. M., and Berryere, T. G. (2007b). The genetics of cream coat color in dogs. J. Hered. 98, 544–548. doi: 10.1093/jhered/esm018
Schmutz, S. M., Berryere, T. G., and Goldfinch, A. D. (2002). TYRP1 and MC1R genotypes and their effects on coat color in dogs. Mamm. Genome 13, 380–387. doi: 10.1007/s00335-001-2147-2
Schmutz, S. M., and Dreger, D. L. (2013). Interaction of MC1R and PMEL alleles on solid coat colors in H ighland cattle. Anim. Genet. 44, 9–13. doi: 10.1111/j.1365-2052.2012.02361.x
Shannon, L. M., Boyko, R. H., Castelhano, M., Corey, E., Hayward, J. J., Mclean, C., et al. (2015). Genetic structure in village dogs reveals a Central Asian domestication origin. Proc. Natl. Acad. Sci. U.S.A. 112, 13639–13644. doi: 10.1073/pnas.1516215112
Staiger, E. A., Almén, M. S., Promerová, M., Brooks, S., Cothran, E. G., Imsland, F., et al. (2017). The evolutionary history of the DMRT3 ‘Gait keeper’haplotype. Anim. Genet. 48, 551–559. doi: 10.1111/age.12580
Steingrímsson, E., Copeland, N. G., and Jenkins, N. A. (2006). Mouse coat color mutations: from fancy mice to functional genomics. Dev. Dyn. 235, 2401–2411. doi: 10.1002/dvdy.20840
Stinckens, A., Luyten, T., Bijttebier, J., Van Den Maagdenberg, K., Dieltiens, D., Janssens, S., et al. (2008). Characterization of the complete porcine MSTN gene and expression levels in pig breeds differing in muscularity. Anim. Genet. 39, 586–596. doi: 10.1111/j.1365-2052.2008.01774.x
Sutter, N. B., Bustamante, C. D., Chase, K., Gray, M. M., Zhao, K., Zhu, L., et al. (2007). A single IGF1 allele is a major determinant of small size in dogs. Science 316, 112–115. doi: 10.1126/science.1137045
Tchernov, E., and Horwitz, L. K. (1991). Body size diminution under domestication: unconscious selection in primeval domesticates. J. Anthropol. Archaeol. 10, 54–75.
Thalmann, O., Shapiro, B., Cui, P., Schuenemann, V. J., Sawyer, S. K., Greenfield, D., et al. (2013). Complete mitochondrial genomes of ancient canids suggest a European origin of domestic dogs. Science 342, 871–874. doi: 10.1126/science.1243650
Thomas, R. H., Schaffner, W., Wilson, A. C., and Pääbo, S. (1989). DNA phylogeny of the extinct marsupial wolf. Nature 340, 465–467. doi: 10.1038/340465a0
Tian, F., Zhan, F., Vanderkraats, N. D., Hiken, J. F., Edwards, J. R., Zhang, H., et al. (2013). DNMT gene expression and methylome in Marek’s disease resistant and susceptible chickens prior to and following infection by MDV. Epigenetics 8, 431–444. doi: 10.4161/epi.24361
Tixier-Boichard, M., Bed’hom, B., and Rognon, X. (2011). Chicken domestication: from archeology to genomics. C. R. Biol. 334, 197–204. doi: 10.1016/j.crvi.2010.12.012
Tonomura, N., Elvers, I., Thomas, R., Megquier, K., Turner-Maier, J., Howald, C., et al. (2015). Genome-wide association study identifies shared risk loci common to two malignancies in golden retrievers. PLoS Genet. 11:e1004922. doi: 10.1371/journal.pgen.1005339
Troy, C. S., Machugh, D. E., Bailey, J. F., Magee, D. A., Loftus, R. T., Cunningham, P., et al. (2001). Genetic evidence for Near-Eastern origins of European cattle. Nature 410, 1088–1091. doi: 10.1038/35074088
Trut, L., Oskina, I., and Kharlamova, A. (2009). Animal evolution during domestication: the domesticated fox as a model. Bioessays 31, 349–360. doi: 10.1002/bies.200800070
Tryon, R. C., White, S. D., and Bannasch, D. L. (2007). Homozygosity mapping approach identifies a missense mutation in equine cyclophilin B (PPIB) associated with HERDA in the American Quarter Horse. Genomics 90, 93–102. doi: 10.1016/j.ygeno.2007.03.009
Vaez, M., Follett, S. A., Bed’hom, B., Gourichon, D., Tixier-Boichard, M., and Burke, T. (2008). A single point-mutation within the melanophilin gene causes the lavender plumage colour dilution phenotype in the chicken. BMC Genet. 9:7. doi: 10.1186/1471-2156-9-7
Venter, J. C., Smith, H. O., and Adams, M. D. (2015). The sequence of the human genome. Clin. Chem. 61, 1207–1208.
Verginelli, F., Capelli, C., Coia, V., Musiani, M., Falchetti, M., Ottini, L., et al. (2005). Mitochondrial DNA from prehistoric canids highlights relationships between dogs and South-East European wolves. Mol. Biol. Evol. 22, 2541–2551. doi: 10.1093/molbev/msi248
Verhulst, E. C., Mateman, A. C., Zwier, M. V., Caro, S. P., Verhoeven, K. J., and Van Oers, K. (2016). Evidence from pyrosequencing indicates that natural variation in animal personality is associated with DRD 4 DNA methylation. Mol. Ecol. 25, 1801–1811. doi: 10.1111/mec.13519
Vigne, J.-D. (2011). The origins of animal domestication and husbandry: a major change in the history of humanity and the biosphere. C. R. Biol. 334, 171–181. doi: 10.1016/j.crvi.2010.12.009
Vilà, C., Leonard, J. A., Götherström, A., Marklund, S., Sandberg, K., Lidén, K., et al. (2001). Widespread origins of domestic horse lineages. Science 291, 474–477. doi: 10.1126/science.291.5503.474
Vitti, J. J., Grossman, S. R., and Sabeti, P. C. (2013). Detecting natural selection in genomic data. Annu. Rev. Genet. 47, 97–120. doi: 10.1093/molbev/msv334
Voight, B. F., Kudaravalli, S., Wen, X., and Pritchard, J. K. (2006). A map of recent positive selection in the human genome. PLoS Biol. 4:e72. doi: 10.1371/journal.pbio.0040072
Wallis, J. W., Aerts, J., Groenen, M. A., Crooijmans, R. P., Layman, D., Graves, T. A., et al. (2004). A physical map of the chicken genome. Nature 432, 761–764.
Wang, C., Wang, H., Zhang, Y., Tang, Z., Li, K., and Liu, B. (2015). Genome-wide analysis reveals artificial selection on coat colour and reproductive traits in Chinese domestic pigs. Mol. Ecol. Resour. 15, 414–424. doi: 10.1111/1755-0998.12311
Wang, G.-D., Fan, R.-X., Zhai, W., Liu, F., Wang, L., Zhong, L., et al. (2014a). Genetic convergence in the adaptation of dogs and humans to the high-altitude environment of the tibetan plateau. Genome Biol. Evol. 6, 2122–2128. doi: 10.1093/gbe/evu162
Wang, G.-D., Xie, H.-B., Peng, M.-S., Irwin, D., and Zhang, Y.-P. (2014b). Domestication genomics: evidence from animals. Annu. Rev. Anim. Biosci. 2, 65–84. doi: 10.1146/annurev-animal-022513-114129
Wang, X., Zhou, G., Li, Q., Zhao, D., and Chen, Y. (2014). Discovery of SNPs in RXFP2 related to horn types in sheep. Small Rum. Res. 116, 133–136.
Wang, G.-D., Zhai, W., Yang, H.-C., Wang, L., Zhong, L., Liu, Y.-H., et al. (2016). Out of southern East Asia: the natural history of domestic dogs across the world. Cell Res. 26, 21–33. doi: 10.1038/cr.2015.147
Wayne, R. K. (1986). Limb morphology of domestic and wild canids: the influence of development on morphologic change. J. Morphol. 187, 301–319. doi: 10.1002/jmor.1051870304
Weaver, I. C., Cervoni, N., Champagne, F. A., D’alessio, A. C., Sharma, S., Seckl, J. R., et al. (2004). Epigenetic programming by maternal behavior. Nat. Neurosci. 7, 847–854.
Webb, A. A., and Cullen, C. L. (2010). Coat color and coat color pattern-related neurologic and neuro-ophthalmic diseases. Can. Vet. J. 51:653.
Wei, C., Lu, J., Xu, L., Liu, G., Wang, Z., Zhao, F., et al. (2014). Genetic structure of Chinese indigenous goats and the special geographical structure in the Southwest China as a geographic barrier driving the fragmentation of a large population. PLoS One 9:e94435. doi: 10.1371/journal.pone.0094435
Wijesena, H. R., and Schmutz, S. M. (2015). A missense mutation in SLC45A2 is associated with albinism in several small long haired dog breeds. J. Hered. 106, 285–288. doi: 10.1093/jhered/esv008
Wilkins, A. S., Wrangham, R. W., and Fitch, W. T. (2014). The “domestication syndrome” in mammals: a unified explanation based on neural crest cell behavior and genetics. Genetics 197, 795–808. doi: 10.1534/genetics.114.165423
Wilkinson, S., Lu, Z. H., Megens, H.-J., Archibald, A. L., Haley, C., Jackson, I. J., et al. (2013). Signatures of diversifying selection in European pig breeds. PLoS Genet. 9:e1003453. doi: 10.1371/journal.pgen.1003453
Wolf, E., Kind, A., Aigner, B., and Schnieke, A. (2018). Genetically engineered large animals in biomedicine. Anim. Biotechnol. 2, 169–214.
Wong, A., Ruhe, A., Robertson, K., Loew, E., Williams, D., and Neff, M. (2013). A de novo mutation in KIT causes white spotting in a subpopulation of G erman S hepherd dogs. Anim. Genet. 44, 305–310. doi: 10.1111/age.12006
Wright, D. (2015). Article commentary: the genetic architecture of domestication in animals. Bioinform. Biol. Insights 9:S28902.
Xiang, H., Li, X., Dai, F., Xu, X., Tan, A., Chen, L., et al. (2013). Comparative methylomics between domesticated and wild silkworms implies possible epigenetic influences on silkworm domestication. BMC Genomics 14:646. doi: 10.1186/1471-2164-14-646
Yang, S., Li, X., Li, K., Fan, B., and Tang, Z. (2014). A genome-wide scan for signatures of selection in Chinese indigenous and commercial pig breeds. BMC Genet. 15:7. doi: 10.1186/1471-2156-15-7
Yindee, M., Vlamings, B., Wajjwalku, W., Techakumphu, M., Lohachit, C., Sirivaidyapong, S., et al. (2010). Y-chromosomal variation confirms independent domestications of swamp and river buffalo. Anim. Genet. 41, 433–435. doi: 10.1111/j.1365-2052.2010.02020.x
Yurchenko, A. A., Daetwyler, H. D., Yudin, N., Schnabel, R. D., Vander Jagt, C. J., Soloshenko, V., et al. (2018). Scans for signatures of selection in Russian cattle breed genomes reveal new candidate genes for environmental adaptation and acclimation. Sci. Rep. 8:12984. doi: 10.1038/s41598-018-31304-w
Zamani, W., Ghasempouri, S. M., Rezaei, H. R., Naderi, S., Hesari, A. R. E., and Ouhrouch, A. (2018). Comparing polymorphism of 86 candidate genes putatively involved in domestication of sheep, between wild and domestic Iranian sheep. Meta Gene 17, 223–231.
Zeder, M. A. (2008). Domestication and early agriculture in the mediterranean basin: origins, diffusion, and impact. Proc. Natl. Acad. Sci. U.S.A. 105, 11597–11604. doi: 10.1073/pnas.0801317105
Zeder, M. A. (2015). Core questions in domestication research. Proc. Natl. Acad. Sci. U.S.A. 112, 3191–3198.
Zeder, M. A., Emshwiller, E., Smith, B. D., and Bradley, D. G. (2006). Documenting domestication: the intersection of genetics and archaeology. Trends Genet. 22, 139–155. doi: 10.1016/j.tig.2006.01.007
Zhang, C., Liu, Y., Xu, D., Wen, Q., Li, X., Zhang, W., et al. (2012). Polymorphisms of myostatin gene (MSTN) in four goat breeds and their effects on Boer goat growth performance. Mol. Biol. Rep. 39, 3081–3087. doi: 10.1007/s11033-011-1071-0
Zhang, C., Ni, P., Ahmad, H. I., Gemingguli, M., Baizilaitibei, A., Gulibaheti, D., et al. (2018). Detecting the population structure and scanning for signatures of selection in horses (Equus caballus) from whole-genome sequencing data. Evol. Bioinform. 14:1176934318775106. doi: 10.1177/1176934318775106
Keywords: animal domestication, evolution, genes, adaptation, diversification
Citation: Ahmad HI, Ahmad MJ, Jabbir F, Ahmar S, Ahmad N, Elokil AA and Chen J (2020) The Domestication Makeup: Evolution, Survival, and Challenges. Front. Ecol. Evol. 8:103. doi: 10.3389/fevo.2020.00103
Received: 15 October 2019; Accepted: 30 March 2020;
Published: 08 May 2020.
Edited by:
Fulvio Cruciani, Sapienza University of Rome, ItalyReviewed by:
Muniyandi Nagarajan, Central University of Kerala, IndiaPaul Gepts, University of California, Davis, United States
Copyright © 2020 Ahmad, Ahmad, Jabbir, Ahmar, Ahmad, Elokil and Chen. This is an open-access article distributed under the terms of the Creative Commons Attribution License (CC BY). The use, distribution or reproduction in other forums is permitted, provided the original author(s) and the copyright owner(s) are credited and that the original publication in this journal is cited, in accordance with accepted academic practice. No use, distribution or reproduction is permitted which does not comply with these terms.
*Correspondence: Jinping Chen, Y2hlbmpwQGdpYWJyLmdkLmNu